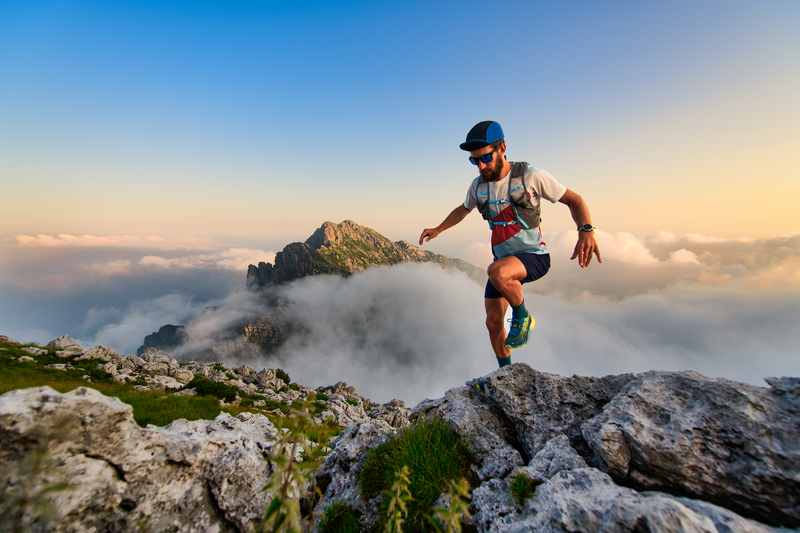
95% of researchers rate our articles as excellent or good
Learn more about the work of our research integrity team to safeguard the quality of each article we publish.
Find out more
HYPOTHESIS AND THEORY article
Front. Astron. Space Sci. , 22 November 2021
Sec. Astrobiology
Volume 8 - 2021 | https://doi.org/10.3389/fspas.2021.759641
This article is part of the Research Topic Bioregenerative Life-Support Systems for Crewed Missions to the Moon and Mars View all 26 articles
Human missions to the Moon and Mars will necessarily increase in both duration and complexity over the coming decades. In the past, short-term missions to low-Earth orbit (LEO) or the Moon (e.g., Apollo) utilized physiochemical life support systems for the crews. However, as the spatial and temporal durations of crewed missions to other planetary bodies increase, physiochemical life support systems become burdened with the requirement of frequent resupply missions. Bioregenerative life support systems (BLSS) have been proposed to replace much of the resupply required of physiochemical systems with modules that can regenerate water, oxygen, and food stocks with plant-based biological production systems. In order to protect the stability and productivity of BLSS modules (i.e., small scale units) or habitats (i.e., large scale systems), an integrated pest management (IPM) program is required to prevent, mitigate, and eliminate both insect pests and disease outbreaks in space-based plant-growing systems. A first-order BLSS IPM program is outlined herein that summarizes a collection of protocols that are similar to those used in field, greenhouse, and vertical-farming agricultural systems. However, the space environment offers numerous unusual stresses to plants, and thus, unique space-based IPM protocols will have to be developed. In general, successful operation of space-based BLSS units will be guided by IPM protocols that (1) should be established early in the mission design phase to be effective, (2) will be dynamic in nature changing both spatially and temporally depending on the successional processes afoot within the crewed spacecraft, plant-growing systems, and through time; and (3) can prevent insect/phytopathology outbreaks at very high levels that can approach 100% if properly implemented.
Human crewed spacecraft since the Mercury and Gemini programs in the 1960’s utilized physical and chemical (i.e., called physiochemical or P/C) life support systems to sustain their occupants. The P/C systems handled CO2 absorption or recycling of the internal spacecraft atmospheres, O2 generation, water purification, pressure control, humidity control, etc. (see reviews by Eckart, 1996; Seedhouse, 2020). Alternatives to P/C life support systems proposed using algae or higher plants in bioregenerative life support systems (BLSS) to recycle water and O2 while producing food stocks for the crews (see reviews by Wheeler, 2004; Escobar and Nabity, 2017; Wheeler, 2017). As mission durations increase in time—or move away from low-earth orbit (LEO)—the energy, launch mass, resupply mass, and economic tradeoffs improve for BLSS compared to decreasing efficiencies of constant resupply for P/C systems. For example, Seedhouse (2020) compares the tradeoffs of 29 life-support factors between BLSS and P/C systems with BLSS approaches surpassing P/C systems when the mission duration exceeds 3 months.
Equivalent system mass (ESM) is a metric used by life-support engineers to contrast and compare mixtures of BLSS and P/C subsystems in order to select the best combination of hardware, materials, energy sources, and operational protocols to optimize life-support while minimizing the energy and overall launch mass of crewed spacecraft and habitats (Drysdale et al., 1999; Drysdale et al., 2001; Ewert et al., 2001; Escobar and Nabity, 2017). When applied to crewed missions in LEO (e.g., Space Shuttle, Skylab, Mir, and the International Space Station [ISS]), ESM modeling suggests that resupply missions for P/C systems are the most economical. Furthermore, in short-duration missions to the Moon (e.g., Apollo) the ESM tradeoffs also argued for a purely P/C approach to life support.
However, we are now entering a new phase of human exploration of the solar system in which long-duration missions to the Moon and Mars begin to argue for ESM tradeoffs that will evolve away from small plant-growth payloads towards larger-scale BLSS habitats. For example, several space-based plant-growth modules are currently operational on the ISS for plant biology research and vegetable production for crews (Zabel et al., 2016). As of this writing, these systems include the Advanced Plant Habitat (APH; Figure 1A; Monje et al., 2020), Vegetable Production System (Veggie; Figure 1B; Massa et al., 2016; Massa et al., 2017), and the Multi-Use Variable-Gravity Platform (MVP; https://techshot.com/aerospace/technology/mvp/). The APH and MVP systems are semi-closed research modules while Veggie is an open-system that utilizes the ISS cabin air for dehumidification and temperature control. As space-based plant-growing systems get more complicated and transition from closed systems to open configurations, the ability to maintain the stability of internal microbiomes on the plants becomes more difficult to achieve. In general, open systems are more subject to microbial disease outbreaks because the crewed habitat microbiome can pass through the plant production modules.
FIGURE 1. Small plant-growth payloads currently onboard the International Space Station (ISS). (A) The Advanced Plant Habitat (APH; Monje et al., 2020) is a semi-closed research unit in which independent environmental controls (e.g., relative humidity, temperature, LED spectral balance) are available to alter the course of a disease outbreak. (B) The Vegetable Production System (Veggie; Massa et al., 2016; Massa et al., 2017) is an open crop production system used to grow fresh edibles for the ISS crew. Astronaut Shane Kimbrough can be seen harvesting lettuce from the Veggie (VEG-03A mission) system on the ISS. (Photos are courtesy of NASA; (A) image oo225510_web; (B) iss055e001010).
Recently, such a scenario occurred on the ISS when cabin air containing infective propagules of the opportunistic phytopathogen, Fusarium oxysporum, caused a severe disease outbreak on Zinnia hybrida plants within a Veggie module (Schuerger et al., 2021a). Disease symptoms developed rapidly during a high-humidity event that created water-soaked leaves and stems within Veggie. The incident supports the conclusion that comprehensive management plans of the ISS and Veggie microbiomes are required to prevent or mitigate severe plant pathology issues in space-based plant growing systems. In essence, we are entering a phase of human exploration in space in which the same microbial and insect pest issues that occur in field, greenhouse, or vertical-farming agricultural operations may occur in future BLSS-supported missions to the Moon and Mars.
Integrated Pest Management (IPM) is a comprehensive approach to managing undesirable microbial and entomological issues that arise during nominal operations of agricultural systems. All aspects of plant production must be considered in an effective IPM program and should include considerations of plant nutrition, horticulture, hardware design, microbial and insect ecology, environmental controls, and operational constraints (to mention a few). First, IPM programs can be both preventive and curative in agricultural systems, are dictated by the pest and disease pressures that are encountered in agricultural systems, and are dynamic in nature. Insect or disease pressures can be defined as the interactions between the incidence (i.e., numerical occurrence) and severity (i.e., levels of damage at the endpoint of the occurrence) of pest/disease outbreaks. As insect or disease pressures increase in incidence and severity, the earlier IPM programs need to be initiated.
An IPM program for space-based plant-growing systems will be proposed here that will be applicable to (1) small-scale plant-growth modules, (2) larger scale plant production systems that have multiple subunits of production but have not yet included waste management, and (3) full-scale BLSS habitats that include plant production and waste management. As the complexities of BLSS modules increase, the complexities of the IPM protocols required to maintain healthy crops will increase. Thus, it is beyond the scope here to propose any sort of comprehensive IPM program with precise specifications or protocols. Instead, the following discussions are intended as a first-order model of the types of IPM protocols that should be considered during currently planned crewed missions to the ISS, the Moon, and Mars over the next 25 years.
Historically, few outbreaks of phytopathogens have been observed in small-scale plant-growth modules, and no insect outbreaks have been noted. The primary reason for the previous success of keeping pests and diseases from developing in space-based plant habitats has been the utilization of closed or semi-closed production modules that were returned to Earth for post-flight processing and sanitation between missions (see reviews by Schuerger, 1998; Schuerger, 2004; Zabel et al., 2016; Schuerger et al., 2021b). Thus, the systems were in general cleaned regularly and rendered free of competing microbiomes separate from the intended research.
However, two naturally occurring disease outbreaks, and one directed plant-pathology experiment in space are noteworthy to discuss here. First, the fungus, Neotyphodium chilense, was identified as the causal agent of disease (i.e., a biological phytopathogen) on wheat seedlings grown in the now decommissioned Plant Growth Unit (PGU; Bishop et al., 1997). The disease occurred on surface-sterilized wheat seeds and was later identified as an endophytic phytopathogen within seeds. Although a heat-treatment of seeds to eliminate the fungus was later developed (Bishop et al., 1997), nearly 50% of the seedlings in the original Space Shuttle STS-63 mission (03-Feb-1995 to 11-Fe-1995) were lost. Second, an international team of scientists conducted the first directed space plant-pathology experiment on the Shuttle STS-87 flight (19-Nov-1997 to 05-Dec-1997) that involved flying infected roots of soybean plants previously inoculated with oospores of Phytophthora sojae; a common root-rotting mycoparasite of soybeans (Ryba-White et al., 2001). Root symptoms, colonization of infected root tissues, and numbers of new oospores observed in infected roots were all significantly higher in space-flown plants than ground controls. And third, Schuerger et al. (2021a) described an outbreak of F. oxysporum on zinnia plants grown in the Veggie VEG-01C mission (16-Nov-2015 to 14-Feb-2016) in which only one of six zinnia plants reached full maturity without being infected by the opportunistic fungus. In all three cases, low-light levels, elevated relative humidities, and the spaceflight environment are likely to have acted synergistically to enhance disease severity in microgravity (µg).
Recently, Schuerger et al. (2021b) described eight broad categories of plausible phytopathogens that should be considered for space-based BLSS modules, regardless of size, including the following (in priority order based on the likelihood of being introduced into space-based BLSS habitats): (1) microbial contaminants, toxins, and secondary metabolites whose presence alone subjects plants to stress without inciting an active disease outbreak; (2) volatile organic compounds—from either abiotic or biotic sources—that alter plant physiology in BLSS modules causing stress; (3) opportunistic phytopathogens that are generally not able to incite disease in healthy plants on Earth; (4) subclinical phytopathogens that might cause stunting and loss of yield without causing overt disease symptoms; (5) storage rots that develop on harvested or stored edible produce; (6) traditional phytopathogens that are accidentally introduced into spacecraft during prelaunch activities and cause the types of disease outbreaks typically encountered in field and greenhouse agricultural systems; and (7) obligate phytopathogens that have unique biotrophic relationships with specific crop cultivars (e.g., rust pathogens on their unique hosts).
To this list we can add several other factors that are likely to impact the health of crops in space-based BLSS. First, the spaceflight environment itself can alter plant and phytopathogen physiologies such that the combination could increase disease severity in space (Khodadad et al., 2020; Schuerger et al., 2021a; Schuerger et al., 2021b). For example, disease severity for the N. chilense/wheat, P. sojae/soybean, and F. oxysporum/zinnia events in LEO all exhibited increased symptoms and signs in the µg environment compared to Earth controls (Bishop, et al., 1997; Ryba-White et al., 2001; Schuerger et al., 2021a; respectively). Recently, Schuerger et al. (2021b) suggested that the increased severity of these three diseases in space may have been caused by up-regulation of phytopathogen virulence genes and down-regulation of plant resistance processes in µg. However, this remains only a hypothesis at this point and requires additional phytopathogen experiments in µg.
Second, the growth of saprophytic fungi on crop detritus during production or harvest cycles might increase the inoculum loads to levels that then overwhelm host resistance. For example, this problem has been observed in greenhouse-grown squash plants in which the typical saprophytic fungus, Choanephora sp., infects squash fruit through colonized senescent flower blossoms still attached to the distal ends of fruit (Figure 2; Schuerger, unpublished). Furthermore, the process of a microbial surge in population (i.e., often called microbial flooding or microbial blooms) can create physical problems to mechanical systems present in BLSS. For example, small or large irrigation tubing can become partially or fully occluded by biofilm formation concomitant to precipitation of nutrient salts (Figure 3; Schuerger, unpublished).
FIGURE 2. The opportunistic fungus, Choanephora sp., infecting squash fruit via a senescent flower blossom (fb). The advancing margin (am) of infected tissue by Choanephora sp. can be observed 2–3 cm above the lawn of black conidia of the phytopathogen. (Photo credit: A.C. Schuerger and courtesy of The Land, Epcot®).
FIGURE 3. Occluded hydroponic irrigation lines after 5.25 years of operation at The Land, Epcot greenhouse facility located in central Florida (circa 1988). (A) Precipitates and microbial biofilms adhered to the insides of nutrient delivery lines. The thickness of the precipitates/biofilms (arrows) was determined by the amount of total flow through the lines. (B) Smaller delivery tubes often had salt precipitates occluding the ends of the tubes where the nutrient/air transition occurred. (C) Shown here are white precipitates removed from 2 mm tubing used for aeration in small hydroponic systems (i.e., from Figure 3B). White precipitates in Figure 3C were rich in Fe (15.35 wt%), Ca (13.53 wt%), PO4 (12.70 wt%), Mg (1.61 wt%), Al (1.16 wt%), K (0.54 wt%), Na (0.44 wt%), and lesser amounts of all ions present in the nutrient solutions (Schuerger, unpublished). (Photo credit: A.C. Schuerger and courtesy of The Land, Epcot®).
And lastly, closed ecosystems, root hypoxia, high humidity, altered gravity, and allelopathy may be concomitant factors that increase plant stress, and thus, potentially alter host resistance to microbial phytopathogens in space. Of these factors, root and canopy hypoxia are well established as plant stressors in µg due to low-shear forces in liquids and gases that result in stratified layers of stagnant water or air with very low gas diffusion rates (e.g., Stout et al., 2001; Kitaya et al., 2003; Maggi and Pallud, 2010).
In summary, a wide range of potential environmental or mechanical stressors—and indeed active phytopathogens—will likely impact the long-term stability of space-based BLSS modules in similar ways as these factors impact field, greenhouse, and vertical-farming agricultural systems on Earth. The spaceflight environment further adds a number of additional stressors that are not typically encountered on terrestrial surfaces due to the lack of a gravity-vector in space. In addition, the full range of physiological, anatomical, and genomic responses of both plants and their associated biological phytopathogens remains mostly unknown for space-based plant-growing systems and should be explored in the near-term with a wide range of directed plant host/phytopathogen experiments.
Spacecraft microbiome studies reveal similar species diversity and bioloads among diverse missions (see reviews by Taylor, 1974; Schuerger, 1998; Schuerger, 2004; Schuerger et al., 2021b). For example, Apollo, Mir, Shuttle, and ISS spacecraft have exhibited similar species of bacteria and fungi because the common factor of these diverse missions are the human crews present for either short-durations or extended tenures in the spacecraft. Prior to circa 2005, the studies were exclusively based on the recovery and identification of culturable bacteria and fungi from spacecraft surfaces and human crews. However, since circa 2010, metagenomic studies of the ISS habitat modules and crews have expanded the known microbiomes in large complex spacecraft to include non-culturable bacteria, fungi, and some archaea (e.g., Mora et al., 2019; Avila-Herrera et al., 2020; Khodadad et al., 2020).
Potential fungal phytopathogens in these studies include species of Alternaria, Aspergillus, Cladosporium, Curvularia, Fusarium, Penicillium, and Verticillium. Potential bacterial phytopathogens recovered from spacecraft include species of Agrobacterium, Burkholderia, Candida, Corynebacterium, Pseudomonas, and Xanthomonas. More recently, a review of the genomic sequences in an ISS microbiome study (Avila-Herrera et al., 2020; as reviewed by Schuerger et al., 2021b) has suggested that phytopathogens like Agrobacterium tumefaciens (crown gall), Alternaria brassicicola (leaf black spot), Erwinia amylovora (soft rot), Erwinia chrysanthemi (wilts), Fusarium oxysporum (crown rot and wilts of vegetables), Pectobacterium carotovorum (soft rot), and Puccinia striiformis (leaf yellow rust of wheat) may already be present in the ISS microbiome. However, no ISS microbiome studies were found in which plant viruses, nematodes, mycoplasmas, or viroids were identified.
The brief discussion above is by no means exhaustive, but it does highlight the possibility that a range of phytopathogens may be introduced into space-based BLSS and crewed habitats. Then why have not more plants succumbed to disease outbreaks? Two factors are in play. First, the question is partially answered by the concept of the disease triangle (Agrios, 2005) in which disease only occurs if the following three factors occur simultaneously: (1) the host must be susceptible to the phytopathogen being considered, (2) the phytopathogen must be virulent and abundant in regards to the susceptible host, and (3) the environment for plant infection and disease development must remain in a conducive range for a long-enough period of time to permit disease development. If any of these factors are interrupted (e.g., resistant cultivars, alteration of the physical environment), then disease development is halted. Second, most plant-growth experiments in space prior to 2015 were conducted in payloads that were prepared prior to launch and maintained during the missions under aseptic conditions. In contrast, ISS plant growth experiments after 2015 included both open (Veggie) and semi-closed (APH) conditions. When under aseptic conditions, the Shuttle, Mir, or ISS microbiomes could not interact with the plants, and thus, disease outbreaks were limited to a single event when wheat seed were contaminated with an endophytic fungus (Bishop et al., 1997).
In contrast to the development of phytopathogens on plants in space, the presence of phytophagus entomological species may be a serious issue because insects that feed on plants are typically generalists without a focused host-range. Although, the emphasis here is to propose IPM practices that are applicable to the development of plant diseases in space-based BLSS, insect pests should not be ignored. For example, the five most common insect pest in greenhouse crops are aphids, leaf miners, spider mites, thrips, and white flies of numerous species (Figure 4; Guillino et al., 2020). As of this writing, it is entirely unknown if these or other phytophagus insects have been introduced into the ISS environment. No reports were found in the literature on the occurrence of these insect pests on the ISS or other spacecraft.
FIGURE 4. Common airborne insect pests (except spider mites) of greenhouse hydroponic systems that are also possible as infestations in space-based BLSS habitats. (A) Green peach aphids (Myzus persicae), (B) thrips (Echinothrips americanus), (C) two-spotted spider mites (Tetranychus urticae), (D) white fly (Bemisia tabaci), (E) shore fly (Scatella stagnalis), (F) fungus gnat (Bradysia sp.), and (G) fruit fly (Drosophila melanogaster). All images were taken under 40× to 60× magnification using stereo-microscopes. (Photo credits: (A, B, E, F) courtesy of L. Osborne, Entomology & Nematology Department, University of Florida; (C) and (D) courtesy of E. Erbe, USDA and colorization of (C) by C. Pooley, USDA; and (G) courtesy of L. Bliss, Entomology & Nematology Dept., University of Florida).
Second, the ability to vector phytopathogens needs to be considered for typically non-phytophagus insects. For example, fruit flies, fungus gnats, and shore flies are well known to act as vectors for a wide range of phytopathogens including Pectobacterium carotovorum (bacterial soft rot; Figure 5; Schuerger and Batzer, 1993), F. oxysporum f. sp. radicis-lycopersici (fungal crown rot of tomato; Gillespie and Menzies, 1993), Pythium aphanidermatum (a root rot eukaryotic mycoparasite of hydroponic crops; Goldberg and Stanghellini, 1990; Hyder et al., 2009; Braun et al., 2012), and Thielaviopsis basicola (fungal root rot of corn-salad, Valerianella locusta; Stanghellini et al., 1999). In addition, insects like aphids, thrips, and white flies are notorious for acting as vectors of plant viruses (e.g., Hogenhout et al., 2008; Whitefield et al., 2015).
FIGURE 5. Drosophila sp. fruit flies feeding on bacterial ooze from an active disease outbreak on squash plants. The fruit flies act as vectors of the bacterial soft-rot phytopathogen, Pectobacterium carotovorum, on greenhouse-grown plants. The image was taken with a 105 mm macrolens on a Nikon 2E single-reflex camera (approx. 7×). (Photo credit: A.C. Schuerger and courtesy of The Land, Epcot®).
In summary, searching for and identifying insects present in the ISS habitat should be a near-term research goal. It is likely that phytophagus insects have been periodically introduced into the Mir and ISS space stations but have not caused problems because they did not survive long enough to colonize plants grown in open plant-growth modules like the Veggie system. However, as crop production becomes a more permanent fixture in space-based crewed habitats, insects that can vector phytopathogens must be studied in order to evaluate the risks to BLSS plant production.
Integrated Pest Management (IPM) is a thoroughly studied approach in field agriculture for insects, nematodes, rodents, and phytopathogens. Less work—but still substantial levels of research—have been developed for IPM programs in greenhouse and vertical-farming agricultural systems (e.g., Jarvis, 1992; Guillino et al., 2020). However, only a half-dozen or so papers have appeared in the literature on IPM programs for space-based BLSS habitats (e.g., Nelson, 1987; Gonzales et al., 1996; Schuerger, 1998; Schuerger, 2004; Schuerger et al., 2021a; Schuerger et al., 2021b).
The following is an outline for an IPM program for space-based plant-growth modules (e.g., APH, Veggie) and large-scale BLSS habitats for future crewed missions to the Moon and Mars. A comprehensive spaceflight IPM program is not specified here because modifications of the concepts below will be achieved through the implementation processes for future missions. Furthermore, aspects of the following IPM program will be adopted differentially for specific missions based on previous flight experience with plant growing systems and the risks of failure to the life-support systems for new missions. As higher plants are adopted for an increasing proportion of the crew’s life-support requirements—and for longer-term missions—pest and disease management should become a priority.
The following concepts will apply to almost all plant-growth systems in space regardless of size. However, as crop-production systems increase in size, complexity, and long-term use, these IPM protocols will become more complex and require significant preplanning for mission success. The following principles for BLSS/IPM protocols are adapted from Agrios (2005), Eckart (1996), Gonzales et al. (1996), Guillino et al. (2020), Jarvis (1992), Marschner (1995), Ming and Henninger (1989), Schuerger (1998), Schuerger (2004), Schuerger et al. (2021a), Schuerger et al. (2021b), and Seedhouse (2020).
Although traditional pesticides are an important component of field and greenhouse agricultural systems, it is proposed here that traditional pesticides should not be used in closed BLSS habitats in space due to the immediate effects (i.e., direct biotoxicity to astronauts) and biomagnification (i.e., increased concentrations through trophic levels) of toxic compounds in the closed ecosystems of spacecraft. However, some materials may be certified for use within closed ecosystems for the sanitation of hardware or plant surfaces. For example, during the F. oxysporum outbreak on zinnia plants on the ISS (Schuerger et al., 2021a), infected plant surfaces were sanitized with citric acid based wipes saturated with 1% Pro-San (Microcide, Inc., Sterling Heights, MI, United States); and hardware surfaces were sanitized with 0.4% benzalkonium chloride wipes (BZK Antiseptic Towelettes, Nice-Pak/PDI Inc., Orangeburg, NY, United States). In both cases, the wipes were precertified for safe use onboard the ISS prior to the disease outbreak; but their efficacy against F. oxysporum was not established prior to the disease event. Thus, the wipes were used because no other IPM protocols were in place when the fungal disease occurred.
Although plant parasitic nematodes, exotic phytopathogens (e.g., mycoplasma, spirochaetes), and many obligate biotrophic phytopathogens (e.g., corn smut, downy mildew, lettuce big vein virus) are likely not to be introduced into BLSS modules due to IPM sanitation and exclusion protocols, it remains plausible that they can be.
Allelopathy may be an issue in crops that share common nutrient loops and should be studied in pre-flight ground research of polycultures.
There are at least four key epidemiological strategies for disease control in BLSS habitats. First, prevent, eliminate, or reduce inoculum loads to tolerable levels. If infective propagules are not present within the crop production systems, then plants can avoid specific phytopathogens (i.e., called escape). Second, slow the rate of disease development by initiating pre-designed and available sanitation, therapeutics, or rogueing protocols. Third, alter the environmental conditions by manipulating the BLSS hardware to reduce the conducive conditions described above in the disease triangle concept. Fourth, IPM protocols must be in place to prevent disease. In most cases, once plants become infected by biological phytopathogens, the plants cannot be rendered free of the infective agents.
Space-based hardware, ISS modules, and full-scale Mars spacecraft are (or will be) assembled under controlled conditions within cleanrooms of increasing stringency of dust mitigation depending on mission criteria (e.g., Figure 6; Columbus module for the ISS). In contrast, greenhouse and vertical-farming agricultural systems are often constructed in an open configuration (e.g., Figure 7; Biosphere 2 complex). In open systems, all types of pests and microbial contamination are possible. For example, the open-construction approach in the Biosphere 2 complex led to serious insect and disease outbreaks (Silverstone and Nelson, 1996; Marino et al., 1999). Insect infestations in the Biosphere 2 complex included outbreaks of ants, aphids, cockroaches, broad mites, spider mites, pill bugs, mealybugs, scale insects, leaf hoppers, white flies, and psyllids. Furthermore, 20 parasitic nematodes were introduced into the agricultural systems with the most devastating being Meloidogyne spp. (root-knot nematodes). Plant diseases were also introduced and included numerous Pythium spp. (root rots of diverse crops). Thus, in the continuum from open-assembly configurations (Biosphere 2) to semi-closed or tightly closed spacecraft assembly facilities (e.g., APH, Columbus, ISS equipment), the more isolation that is possible, the more likely that insects and phytopathogens will be severely constrained in both diversity and abundance. Based on the perceived risks to the missions, tighter controls utilized during assembly will yield better chances that the launched hardware, equipment, and subsystems will not be carriers of the insects and disease phytopathogens outlined above.
FIGURE 6. The Columbus module (launched on 11-Feb-2008) was assembled in a closed-construction cleanroom configuration inside the Operation & Checkout Facility at the Kennedy Space Center, FL. The assembly of most spacecraft and ISS modules are (or will be) assembled under such conditions and will reduce the diversity and bioburden of surface contamination. (Photo courtesy of NASA).
FIGURE 7. The Biosphere 2 complex in Oracle, AZ was assembled in an open-construction configuration that permitted many more insect pests, plant diseases, and microbial communities than the closed-construction configurations of spacecraft, ISS modules (e.g., Figure 6), or future BLSS habitats. (Photo credit: A. C. Schuerger).
Large-scale space BLSS habitats should be compartmentalized with separate nutrient and air recirculation systems in order to manage sets of crops under optimum conditions (e.g., warm- versus cool-temperature crops), and to isolate units if an insect outbreak or disease epidemic occurs.
The following are general principles for the ecology of phytopathogens that individually, or in combination, might be utilized for preventing or mitigating disease outbreaks in space-based BLSS.
i) Most fungi enter hosts tissues actively utilizing their endogenous energy reserves in spores to gain footholds in host tissues.
ii) Most bacteria and viruses enter hosts tissues via vectors or passively through mass flow of water into leaves, stems, or roots.
iii) Excluding vector-mediated infections, most phytopathogens require free moisture on susceptible tissues for infection, and thus, humidity control within a canopy is a key component of any IPM program for BLSS crops.
iv) Over fertilization (e.g., high N and P) will often promote disease development in crops.
v) High K and Ca fertilization will often suppress disease development by strengthening cell wall structures.
vi) Continual use of monocultures over time and space may increase inocula loads of phytopathogens in BLSS modules and could lead to the loss of host resistance. Thus, utilize crop rotation, genetic diversity in crop cultivars, and polycultures to prevent specific phytopathogens from developing into epidemics in BLSS crops. Crop rotation might include changing specific cultivars of one crop-type with divergent resistance genes or rotating to other crop species in time and location.
vii) Optimum temperatures for disease development are typically in the 25–30°C range. Thus, holding crops under slightly cooler conditions (i.e., <25°C) often can suppress the development of many diseases.
viii) Optimum relative humidity (RH) for disease development is >80%, with the presence of free-standing water on leaves and stems the most problematic. Thus, maintaining canopy humidities ≤70% RH will decrease both the incidence and severity of canopy infections.
ix) Phytopathogens are most likely to be introduced into BLSS habitats by the following routes (in priority): (1) airborne; (2) human-transmitted via equipment, food, clothing, or hardware; (3) insects missed by exclusion protocols, (4) seed (most can be sanitized prior to flight), and (5) vegetative transplants (if not properly processed through tissue-culture techniques).
x) There is generally a grace-period early in the operation of greenhouse and other closed agricultural systems in which few insect and phytopathology problems are encountered. However, as the operations of such systems—including space-based BLSS habitats—continue through time, the slow introductions of new materials and personnel will increase the risks of insect pest outbreaks and plant disease epidemics.
xi) Polygenic resistance is regarded as more durable than isogenic resistance (Agrios, 2005). Thus, it is harder for a phytopathogens to mutate multiple gene loci at once in order to attack a host that has a collection of polygenic resistance genes. When selecting crop cultivars for space-based BLSS, choose polygenic host resistance genes, if available.
There are several high-criticality phases for initiating a system-wide BLSS IPM program and include the following: (1) hardware design, (2) horticulture design phase, (3) payload and subsystem construction, (4) pre-launch protocols of assembled hardware, (5) crew training and experience in agricultural activities, (6) crop production, and (7) harvest and waste processing. The following are brief discussions of the IPM protocols that might be adopted during the hardware design phase of the BLSS subsystems.
Use anti-microbial tubing, films, and surfaces to keep microbial biofilms managed within the nutrient and water irrigation subsystems.
However, plan for the eventual occlusion of nutrient and water delivery subsystems, and thus, plant production hardware would benefit from being modular, easily assembled/disassembled, and easily sanitized (i.e., not requiring sophisticated or complex sterilization equipment or protocols).
Excellent air flow through crop canopies would decrease internal canopy humidity levels. BLSS crop-production units would benefit from air flowing upward from the plant crowns; if such air flow dynamics can be easily designed into the systems.
Temperature (<25°C) and humidity (≤70% RH) control systems should be designed into long-term BLSS hardware whenever possible.
Irrigation of plant roots would benefit from minimal splashing or release of liquid nutrient solutions (even in a gravity-well like the Moon or Mars) in order to prevent algal or microbial blooms on hydrated surfaces.
Avoid the design of one large-scale BLSS habitat with common air, water, and nutrient loops. If BLSS modules can be designed with isolated loops, the chances of losing the entire BLSS habitat due to a mechanical or biological failure is significantly reduced. However, it is obvious that the ESM trade-offs between one large BLSS approach versus isolated modular subunits will factor into the eventual design of such systems.
Plant roots require dissolved oxygen in nutrient solutions. The saturated dissolved pO2 at 25°C on Earth at 1 g is approx. 7–8 ppm if nutrient solutions are aerated during recirculation. If a physical substrate is used for irrigation and plant support (e.g., Moon or Mars regolith (Ming and Henninger, 1989); Arcillite in plant pillows in the Veggie hardware (Massa et al., 2016; Massa et al., 2017), then the aeration of the roots is likely satisfied by the interstitial spaces within the materials. However, root hypoxia can still occur in µg due to low-shear forces (Stout et al., 2001; Kitaya et al., 2003; Maggi and Pallud, 2010), and thus, root aeration should be monitored in most systems.
All crops require frequent scouting to maintain optimum conditions for crop health free of insect infestations and disease outbreaks. It is beyond the scope of the current effort to go into detail on scouting protocols. Suffice it to note here that automatic remote sensing systems will likely be required to monitor crop health over time.
And lastly, rapid disease diagnostic tools are required for space-based diagnoses of phytopathogens such that the flight and ground crews can rapidly respond to specific issues (see Haveman and Schuerger, 2021). For example, foliar symptoms of tip-burn on cabbage (Figure 8A; i.e., usually caused by Ca imbalance in rapidly expanding leaves) and soft-rot bacterial disease (Figure 8B; e.g., caused by Pectobacterium carotovorum) appear very similar in the early stages of disease. Not knowing which is the causal agent of disease in Figures 8A,B will significantly hamper the disease control response.
FIGURE 8. Symptoms of calcium tip burn versus bacterial soft rot. (A) Lettuce plant (Lactuca sativa) affected by calcium-tip burn along leaf margins. (B) Chinese cabbage (Brassica rapa) infected with the bacterial soft-rot phytopathogen, Pectobacterium carotovorum. (Photo credits: (A) by R.M. Wheeler and courtesy of NASA; (B) by A.C. Schuerger and courtesy of The Land, Epcot®).
In parallel to the physical design and construction of the BLSS hardware, horticultural activities will be developed to select a diversity of crops that will be compatible with the hardware. It cannot be over-emphasized that the hardware and horticultural design landscapes must be interwoven during the prelaunch phase to optimize all activities for mitigating disease outbreaks. The following suggestions are for the horticultural design phase of the BLSS habitat.
Select crop cultivars with resistance to known or anticipated phytopathogens. For example, to-date Pythium spp. (root rots) have not been recovered from spacecraft or the ISS (see Schuerger, 1998; Schuerger, 2004; Schuerger et al., 2021a; Schuerger et al., 2021b), and thus, efforts to develop crops resistant to this genus may not be fruitful for space-based BLSS modules. In contrast, Fusarium spp. (fungal wilts, rots, and head blights) are predicted to be major problems in space-based BLSS crops (see Schuerger and Mitchell, 1992; Schuerger et al., 2021a; Schuerger et al., 2021b), and thus, selecting crops resistant to known Fusarium spp. would be a logical decision. The lists of active phytopathogens in space-based BLSS habitats are likely to grow over time, and thus, plant breeders and horticulturalist should remain vigilant in testing and improving crop resistance to the unique environmental and biological stressors experienced in spaceflight.
Avoid high-pollen producing crops because dispersed pollen can act as a rich organic food base for weak or opportunistic phytopathogens (see examples in Schuerger et al., 2021b).
Select crops for high harvest indices to reduce waste detritus at harvest. Senescent biomass is another easy source of proliferating saprophytic bacteria and fungi in closed ecosystems, and thus, should be either collected quickly and sterilized or avoided by developing crops with high harvest indices.
Avoid crops with succulent flower blossoms, fruit, or other structures because many phytopathogens (e.g., Choanephora sp.; Figure 2) can take advantage of succulent tissues, especially if high humidities and temperatures are present.
Avoid selecting crops with high levels of leaf guttation because the free water droplets along leaf margins may serve as sites for bacterial or fungal infections.
Crop rotation is a very effective IPM protocol to keep inoculum loads low in crop production systems due to the constantly changing genetic diversity of host tissues.
Select at least three genetically dissimilar cultivars of each crop to provide genetic diversity during the mission. The preferred cultivar could be grown until a phytopathological issue arises, upon which the crew could switch to other cultivars or crops.
Sanitize all seeds for both external microbial contamination and internal endophytic phytopathogens (e.g., Bishop et al., 1997). Seed sanitation protocols must be maintained through time among different seed lots.
Avoid the use of organic-rich rooting substrates (e.g., peat moss) because microbial blooms are more likely to occur in organic rich materials and such blooms can interfere with essential nutrient uptake for plants. Furthermore, peat moss can harbor pathogens and insect pests more easily than inorganic substrates (e.g., rockwool).
Once crop production hardware is constructed, and the crop cultivars selected, the next most crucial phase to mitigate down-stream pathology issues is the pre-launch handling of payloads, spacecraft processing personnel, and astronauts. The following are brief discussions of a number of pre-launch IPM protocols that should be considered for both small-scale payloads (e.g., APH, Veggie) and complex BLSS modules. However, as mission criteria become aligned with utilizing BLSS habitats for significant amounts of regeneration of O2, water, and food stocks, the risks of losing the BLSS functionality will increase, and thus, so too will the utilization of IPM protocols available to the ground and flight crews.
All flight hardware should be sterilized or sanitized prior to launch and kept in sealed bio-isolation wraps or containers to prevent recontamination. Such an approach will assure that the hardware itself is not the source of new phytopathogens.
All personnel handling flight hardware—even if they are not directly involved in handling BLSS crop production equipment—should be aware that they can act as vectors for both insects and phytopathogens into space launch systems, crewed habitats, and crop-production payloads. For example, individuals that handle tobacco products can frequently act as vectors of plant viruses like tomato mosaic virus (Ng and Perry, 2004; Whitefield et al., 2015). In addition, even the colors of clothing and flight suits can have repellant (white or silver) or attractant (yellow or light blue) effects to insects like thrips, white flies, fungus gnats, and aphids (Guillino et al., 2020). For example, the Columbus module engineers depicted in Figure 6 are wearing light-blue cleanroom garments. Thus, flight support personnel would reduce the risks of accidentally introducing many insect pests or their vectored phytopathogens into flight systems by wearing non-attractant colors during handling of all flight hardware.
Insect barriers should be erected at the sites of launch vehicle ingress and egress to prevent flying insect pests from entering spacecraft prior to launch. A single air-curtain might not be adequate in all cases, and a double-exclusion barrier (i.e., a 2-stage cleanroom approach in which the spacecraft technicians and crew swap out street clothes for cleanroom garments in an anteroom before passing through an air-flushing and filtration room) might be considered if the threat from insect pests is documented. If a full-scale BLSS habitat gets a severe insect infestation (e.g., by spider mites or white flies), it would be very difficult to eliminate the infestation short of shutting the whole crop production system down and rebooting the BLSS habitat.
During each mission that utilizes a plant-growth module (e.g., APH and Veggie; Figure 1) or BLSS habitat (e.g., Figure 9), IPM practices will be utilized to (1) attempt to prevent the introduction of insects or phytopathogens, and then (2) mitigate all outbreak events that do occur. A comprehensive IPM program established during the design and construction of BLSS modules and habitats can reduce the incidence and severity of both insect pest and phytopathogen outbreaks by >90%. However, the unusual conditions encountered in microgravity—or the reduced gravity on the Moon (1/6g) and Mars (1/3g)—may create conditions that promote the development of opportunistic phytopathogens (e.g., F. oxysporum on zinnia plants on the ISS; Schuerger et al., 2021a). The following are suggested IPM protocols that should be considered for space-based BLSS modules.
FIGURE 9. A Mars-based BLSS module that uses transparent walls similar to a terrestrial greenhouse can provide significant amounts of water, O2, and food recycling on other planetary bodies. Although humanity may be several decades away from such complex bases on Mars, activities on the ISS are paving the way for the design, flight, and operation of planetary BLSS habitats. (Photo credit: by B. Versteeg/Spacehabs.com).
Environmental manipulation is the best and easiest way to interfere in the disease triangle combination of factors that leads to disease development. Thus, (1) keep ambient RH ≤ 70% in crop canopies, (2) raise or lower temperatures (i.e., ideally with separate root and shoot temperature control subsystems), when feasible to move outside the conducive ranges of the phytopathogens being encountered, (3) avoid BLSS operations that create dew or standing water on crop canopies, and (4) keep root zone pO2 near saturation to promote healthy root systems.
Operate LED lighting systems, air-circulation, horticultural practices, and environmental controls to minimize open wounds on plants created during pruning activities. For example, crops should be pruned when canopies are dry, and leaf pruning should be directed at leaf abscission layers (when possible). If pruning cuts are made distal to the abscission layers of leaves, and leaf canopies are under high-humidity conditions, the cut bases of the petioles can act as sites for colonization by many fungi and bacteria. The microbial colonization of dead tissues that remain connected to stems is an easy route into the main stems of plants for aggressive phytopathogens (e.g., Figures 2, 5).
Frequent sanitation of pruning equipment should be maintained during the missions to avoid the microbial contamination of the cutting surfaces. As the bioburden on the shears or scissors builds up, the chances of vectoring a traditional or opportunistic phytopathogen increases.
Senescent crop detritus should be avoided by selecting crops with high-harvest indices and by removing dead tissues as frequently as possible.
Edible portions of harvested crops should be produced on a grow-and-eat cycle to minimize the storage of edible biomass that might succumb to colonization of airborne storage rot fungi (e.g., Aspergillus, Mucor, Penicillium, and Rhizopus spp.) or bacteria (e.g., soft rot bacteria like Erwinia and Pectobacterium spp.).
Insects can be attracted by UV, blue, and yellow lights. Thus, small-scale and low-power insect traps should be developed that are strategically placed within BLSS habitats to draw flying insects away from crop production modules for capture.
Sanitize the crop production equipment and surfaces between crops. And where feasible, sanitize the internal nutrient flow plumbing to reduce or eliminate microbial biofilms.
Degrade, recycle, or remove crop detritus as quickly as possible after final harvests to prevent colonization of the detritus by saprophytic fungi and bacteria. If this process can be completed quickly, the removal of the detritus will act to suppress microbial blooms within BLSS equipment.
Crop detritus should also be heat-sterilized before reuse within nutrient recapture hardware, waste processing cycles, or incorporation into regolith growing media.
The following brief case studies are given as first-order IPM programs for plant-growth modules (i.e., small scale) and BLSS habitats (i.e., large scale). Most of the IPM protocols or concepts given above are, in principle, applicable to all plant-growing systems currently on the ISS or to be built for future crewed missions to the Moon and Mars. In addition, small-scale modules will always be more easily managed for insect and disease problems than large-scale BLSS habitats. However, IPM programs must be (1) established early in the mission design phase to be effective, (2) will be dynamic in nature changing both spatially and temporally depending on the successional processes afoot within the crewed spacecraft through time; and (3) can prevent insect/phytopathogen outbreaks at very high levels of success that can approach 100% if properly implemented.
A semi-closed plant-growth module like the APH unit (Figure 1A) is the conceptual endmember of IPM modeling that are the easiest to keep free of insect infestations and disease outbreaks. The fundamental approach should be to (1) sanitize or sterilize all components prior to launch, (2) screen seed or tissue-culture propagative materials for both external and endophytic insects and phytopathogens, (3) sanitize seed if such insects/microbes are present, (4) operate the horticultural activities in such a manner as to mitigate against the introduction or dispersal of phytopathogens (e.g., use the same set of dedicated crop-pruning tools that are frequently sanitized), (5) remove and isolate all crop waste at harvest, and (6) sanitize and clean all equipment between cropping cycles. In the past, plant-growth modules were typically returned to the ground for reprocessing between flight experiments. However, the APH is a modular system in which some subsystems might be returned to the ground for reprocessing while other subunits may not. The subunits that remain on the ISS, will become more likely to be problematic over multiple cropping cycles and should be sanitized with the citric acid-based wipes saturated with 1% Pro-San or the 0.4% benzalkonium chloride wipes described in Integrated Pest Management for Space-Based Bioregenerative Life Support Systems. Such sanitation protocols will act to break insect reproductive and disease cycles and lower the overall risks to plants in these systems.
Although open-agricultural systems like Veggie will undergo a short grace-period when first launched—over time—the systems will begin to encounter more problems as the hardware begins to receive microbial contamination from the ISS microbiome. Open systems will require the same startup IPM protocols described above for the APH but will also require additional sanitation and sterilization scrutiny over time including: (1) replacement of uncleanable parts; (2) fallow periods of time to help break insect and pathogen life-cycles; (3) crop-rotation to avoid sequential monocultures of one crop in any specific Veggie unit; (4) multiple cultivars available to respond to disease outbreaks; and (5) remove individual plants that exhibit severe stress, insect infestations, or disease outbreaks as soon as possible after detection.
Currently, there are two Veggie (Figure 1B) units operating on the ISS. Let us assume that eventually this builds up to 10 separate units to provide daily salad greens for an ISS crew of six astronauts and cosmonauts. A big advantage of multiple Veggie units that are sown with diverse crops is that the genetic diversity and staggered cropping cycles help protect the plant-growing area from outbreaks of a given insect pest or phytopathogen. For example, the genetic diversity of five crops sown into a polyculture (i.e., two Veggie units per crop) offers a significant improvement in ecological stability over monocultures. However, if all 10 Veggie units are side-by-side, insect infestations can spread rapidly. Thus, another IPM protocol in a multi-unit system would be to have one-half of all Veggie units located in one ISS module and the second-half in a different module. Such compartmentalization will increase the ecological stability of both growing regions with minimal increased hardware or crew time. Furthermore, if an insect infestation or a phytopathogen with a wide host-range occurs in one subsystem—but not in both—one half of the plant-growing units can be shut down, cleaned, sanitized and repropagated without losing all of the plant production in the ISS.
In the BLSS literature discussed above (e.g., Ming and Henninger, 1989; Eckart, 1996; Wheeler, 2017; Seedhouse, 2020), the authors outline multiple scenarios in which initially there are a few plant-growth modules in prototype spacecraft that evolve into larger systems that provide 50% of crew diets, and in-the-end will be full-scale habitats providing up to 90% of oxygen, water, and crops to support crews. Throughout this continuum, IPM protocols will start simple and develop into more complex programs through the design, construction, flight, operation, and recovery phases of any mission to the Moon and Mars. Most of the IPM protocols discussed above will be applicable to such complex BLSS habitats.
For example, in the BLSS module depicted in Figure 9, the plant production habitat is isolated from the main Mars base but will undoubtedly be connected to recycle water, O2, and edible biomass. Every time materials and people pass between the BLSS habitats and the primary crew quarters, the microbiomes of each must be managed to mitigate against the introduction of microbial phytopathogens. However, if insect infestations do not arise in the first few cropping cycles, it is likely that the IPM prelaunch program was successful in preventing the introduction of eggs, larvae, or adults of the problematic insects. Once a BLSS module is isolated from Earth, insect infestations would be expected to be a non-issue until the next crew arrives. Thus, there will have to be IPM protocols associated with crew-rotations in which new crews do not bring with them fresh materials with new pests or phytopathogens.
In addition, a Mars mission can be broken down into three phases for spacecraft and crew operations: (1) outbound transit from Earth-to-Mars, (2) surface operations using one or more bases and rovers, and (3) an inbound Mars-to-Earth transit. The outbound transit to Mars is the most critical to assure that no insects are hitchhiking along as unwanted interlopers. If the 6–8 months outbound transit phase is free of insect infestations, then the crew rotation protocols will likely not be required. However, if outbound BLSS modules encounter either severe insect infestations and/or disease outbreaks, the crews and BLSS hardware within the outbound leg should be mothballed approx. 3–4 weeks prior to arrival in Mars orbit to break the insect and disease life cycles.
In the Introduction section, the term equivalent system mass was introduced as a necessary modeling approach to examine the trade-offs among diverse spacecraft components, life-support systems, and mission scenarios. All of the IPM practices discussed above—including new protocols yet to be developed—will be evaluated on how they affect the ESM of the final configuration for human missions to the Moon and Mars. The closer the spacecraft and BLSS habitats are to Earth, the more relaxed such an IPM program can be because there are shorter resupply time-lags from Earth, and ground crews can be more responsive within the Earth/Moon system. In contrast, a crewed Mars trip will be a 3-year mission in which all life-support equipment and protocols must work effectively to maintain crew health. As soon as the BLSS approach is designed into bases and missions to provide for essential crew life support activities on Mars, an IPM program must be adopted to assure the long-term success of the systems.
Furthermore, any given set of IPM protocols might also have different levels of intensity within diverse scenarios depending on the risk to the mission and the distance from Earth. For example, the intensity of an inter-crop sanitization cycle might be a quick wipe-down of the Veggie hardware between crops when no problems have occurred; such an approach would be a low-intensity IPM program. In contrast, if an insect infestation occurs during transit between Earth and Mars, a system-wide shut-down, sanitation/sterilization process, and reboot procedure might be considered a very high-intensity IPM program. Thus, ESM modeling of different BLSS habitats with divergent mission goals should be a prelaunch priority and is likely to force different IPM programs to be adopted for the divergent missions. One IPM program will not fit all mission scenarios.
Bioregenerative life support systems that utilize higher plants for the recycling of O2, water, and food biomass have significant ESM advantages over pure P/C systems due to the reductions in resupply missions. Plant production science in the spaceflight environment has a long and storied history that began in the mid-1960’s. However, a full-scale BLSS habitat with fully integrated food production, O2 regeneration, and waste recycling subsystems has not yet been designed, built, or tested. As the science and technologies continue to be developed over the next decade, IPM programs for both insect pests and phytopathogens need to be developed in parallel with plant production technologies to assure crew safety for missions that leave the Earth/Moon system.
The IPM protocols and approaches discussed above represent a first-order IPM model for BLSS hardware and habitats. The individual IPM components can be used alone or in combination to help reduce the risks of losses from insects and phytopathogens. With a carefully integrated IPM program woven into BLSS technologies and operations, the risks of catastrophic failures can be dramatically reduced (i.e., possibly to near zero). Moreover, if an insect pest or phytopathogen outbreak does occur, preestablished IPM protocols on the outbound spacecraft (e.g., sanitation wipes, crop diversity, compartmentalization, etc.) will likely make it manageable without compromising the mission.
The future of space exploration is very exciting with both commercial and government organizations working toward missions to the Moon and Mars. However, as the space transportation systems begin to achieve fruition, the development of sustainable life support systems become imperative. Thus, the next decade will fit into space history as a key phase of human dispersal into the Solar system. We need to be prepared to support increasing populations of humans on multiple missions using BLSS approaches to reduce the Solar System wide ESM of all activities. Integrated Pest Management fits directly into the development of BLSS habitats and offers to significantly increase the ecological stability and reliability of plant-based life support systems.
The original contributions presented in the study are included in the article/supplementary material, further inquiries can be directed to the corresponding author.
Written informed consent was not obtained from the individual in Figure 1 because it is standard NASA policy to name astronauts in public-domain photos. Thus, no consent was required.
AS envisioned, wrote, and edited the entire paper as presented above.
Funding support for the creation of this work was provided by the Southeastern Regional Universities Association (Grant #80KSC017C0012) as partial support for a visiting scientist program to NASA, Kennedy Space Center, FL.
The author declares that the research was conducted in the absence of any commercial or financial relationships that could be construed as a potential conflict of interest.
All claims expressed in this article are solely those of the authors and do not necessarily represent those of their affiliated organizations, or those of the publisher, the editors and the reviewers. Any product that may be evaluated in this article, or claim that may be made by its manufacturer, is not guaranteed or endorsed by the publisher.
The author would like to thank Fred Pettit (Science Director for Epcot, Lake Buena Vista, FL, United States); Lance Osborne and Lyle Buss (Entomology & Nematology Department, University of Florida); NASA; and the United States Department of Agriculture for photographs used in this publication.
Avila-Herrera, A., Thissen, J., Urbaniak, C., Be, N. A., Smith, D. J., Karouia, F., et al. (2020). Crewmember Microbiome May Influence Microbial Composition of ISS Habitable Surfaces. PloS ONE 15 (4), e0231838. doi:10.1371/journal.pone.0231838
Bishop, D. L., Levine, H. G., Kropp, B. R., and Anderson, A. J. (1997). Seedborne Fungal Contamination: Consequences in Space-Grown Wheat. Phytopathology 87, 1125–1133. doi:10.1094/phyto.1997.87.11.1125
Braun, S. E., Sanderson, J. P., and Wraight, S. P. (2012). Larval Bradysia Impatiens (Diptera: Sciaridae) Potential for Vectoring Pythium Root Rot Pathogens. Phytopathology 102, 283–289. doi:10.1094/phyto-09-11-0262
Drysdale, A. E., Ewert, M. K., and Hanford, A. J. (1999). Equivalent System Mass Studies of Missions and Concepts. Warrendale, PA: ICES Paper, 1–4.
Drysdale, A. E., Maxwell, S., Ewert, M. K., and Hanford, A. J. (2001). Systems Analysis of Life Support for Long-Duration Missions, Vol. 2000-01-2394. Warrendale, PA: SAE International.
Eckart, P. (1996). Spaceflight Life Support and Biospherics. Dordrect, Netherlands: Kluwer Academic Publishers & Microcosm Press, 444.
Escobar, C. M., and Nabity, J. A. (2017). “Past, Present, and Future of Closed Human Life Support Ecosystems - A Review,” in ICES-2017-311 International Conference on Environmental Systems, Warrendale, PA, 1–18.
Ewert, M. K., Drysdale, A. E., Hanford, A. J., and Levri, J. (20012001). Life Support Equivalent System Mass Predictions for the Mars Dual lander Reference mission. Warrendale, PA: ICES Paper, 1–11.
Gillespie, D. R., and Menzies, J. G. (1993). Fungus Gnats Vector Fusarium oxysporum f. sp. radicis-lycopersici. Ann. Appl. Biol. 123, 539–544. doi:10.1111/j.1744-7348.1993.tb04926.x
Goldberg, N. P., and Stanghellini, M. E. (1990). Ingestion-Egestion and Aerial Transmission of Pythium aphanidermatum by Shore Flies (Ephydrinae: Scatella stagnalis). Phytopathology 80, 1244–1246. doi:10.1094/phyto-80-1244
Gonzales, A. A., Schuerger, A. C., Barford, C., and Mitchell, R. (1996). Engineering Strategies for the Design of Plant Nutrient Delivery Systems for Use in Space: Approaches to Countering Microbiological Contamination. Adv. Space Res. 18, 5–20. doi:10.1016/0273-1177(95)00857-b
Guillino, M. L., Albajes, R., and Nicot, P. C. (2020). Integrated Pest and Disease Management in Greenhouse Crops. 2nd Edn. New York City, NY: Springer Publishing, 700.
Haveman, N. J., and Schuerger, A. C. (Forthcoming 2021). Diagnosing an Opportunistic Fungal Pathogen on Spaceflight-Grown Plants Using the MinION Sequencing Platform. Astrobiology. doi:10.1089/ast.2021.0049
Hogenhout, S. A., Ammar, E.-D., Whitfield, A. E., and Redinbaugh, M. G. (2008). Insect Vector Interactions with Persistently Transmitted Viruses. Annu. Rev. Phytopathol. 46, 327–359. doi:10.1146/annurev.phyto.022508.092135
Hyder, N., Coffey, M. D., and Stanghellini, M. E. (2009). Viability of Oomycete Propagules Following Ingestion and Excretion by Fungus Gnats, Shore Flies, and Snails. Plant Dis. 93, 720–726. doi:10.1094/PDIS-93-7-0720
Khodadad, C. L. M., Hummerick, M. E., Spencer, L. E., Dixit, A. R., Richards, J. T., Romeyn, M. W., et al. (2020). Microbiological and Nutritional Analysis of Lettuce Crops Grown on the International Space Station. Front. Plant Sci. 11, 199–215. doi:10.3389/fpls.2020.00199
Kitaya, Y., Kawai, M., Tsuruyama, J., Takahashi, H., Tani, A., Goto, E., et al. (2003). The Effect of Gravity on Surface Temperatures of Plant Leaves. Plant Cell Environ 26, 497–503. doi:10.1046/j.1365-3040.2003.00980.x
Maggi, F., and Pallud, C. (2010). Space Agriculture in Micro- and Hypo-Gravity: A Comparative Study of Soil Hydraulics and Biogeochemistry in a Cropping Unit on Earth, Mars, the Moon and the Space Station. Planet. Space Sci. 58, 1996–2007. doi:10.1016/j.pss.2010.09.025
Marino, B. D. V., Mahato, T. R., Druitt, J. W., Leigh, L., Lin, G., Russell, R. M., et al. (1999). The Agricultural Biome of Biosphere 2:. Ecol. Eng. 13, 199–234. doi:10.1016/s0925-8574(98)00100-1
Marschner, H. (1995). Mineral Nutrition of Higher Plants. 2nd Edn. New York City, NY: Academic Press, 889.
Massa, G. D., Dufour, N. F., Carver, J. A., Hummerick, M. E., Wheeler, R. M., Morrow, R. C., et al. (2017). VEG-01: Veggie Hardware Validation Testing on the International Space Station. Open Agric. 2, 33–41. doi:10.1515/opag-2017-0003
Massa, G. D., Wheeler, R. M., Morrow, R. C., and Levine, H. G. (2016). Growth chambers on the International Space Station for Large Plants. Acta Hortic. 1134, 215–222. doi:10.17660/actahortic.2016.1134.29
Ming, D. W., and Henninger, D. L. (1989). Lunar Base Agriculture: Soils for Plant Growth. Madison, WI: American Society of Agronomy, Inc. Crop Science Society of America, Inc. Soil Science Society of America, Inc., 255.
Monje, O., Richards, J. T., Carver, J. A., Dimapilis, D. I., Levine, H. G., Dufour, N. F., et al. (2020). Hardware Validation of the Advanced Plant Habitat on ISS: Canopy Photosynthesis in Reduced Gravity. Front. Plant Sci. 11, 1–15. doi:10.3389/fpls.2020.00673
Mora, M., Wink, L., Kogler, I., Mahnert, A., Rettberg, P., Schwendner, P., et al. (2019). Space Station Conditions Are Selective But Do Not Alter Microbial Characteristics Relevant to Human Health. Nat. Commun. 10, 3990. doi:10.1038/s41467-019-11682-z
Nelson, B. (1987). The Role of Plant Pathology in Development of Controlled Ecological Life Support Systems. Plant Dis. 71, 580–584. doi:10.1094/pd-71-0580
Ng, J. C. K., and Perry, K. L. (2004). Transmission of Plant Viruses by Aphid Vectors. Mol. Plant Pathol. 5, 505–511. doi:10.1111/j.1364-3703.2004.00240.x
Ryba-White, M., Nedukha, O., Hilaire, E., Guikema, J. A., Kordyum, E., and Leach, J. E. (2001). Growth in Microgravity Increases Susceptibility of Soybean to a Fungal Pathogen. Plant Cell Physiol 42, 657–664. doi:10.1093/pcp/pce082
Schuerger, A. C. (1998). Microbial Contamination of Advanced Life Support (ALS) Systems Poses a Moderate Threat to the Long-Term Stability of Space-Based Bioregenerative Systems. Life Support. Biosph. Sci. 5, 325–337.
Schuerger, A. C., Amaradasa, B. S., Dufault, N. S., Hummerick, M. E., Richards, J. T., Khodadad, C. L., et al. (2021a). Fusarium oxysporum as an Opportunistic Fungal Pathogen on Zinnia hybrida Plants Grown on Board the International Space Station. Astrobiology 21 (9), 1029–1048. doi:10.1089/ast.2020.2399
Schuerger, A. C., and Batzer, J. C. (1993). Identification and Host Range of an Erwinia Pathogen Causing Stem Rots on Hydroponically Grown Plants. Plant Dis. 77, 472–477. doi:10.1094/pd-77-0472
Schuerger, A. C. (2004). “Microbial Ecology of the Surface Exploration of Mars with Human-Operated Vehicles,” in Martian Expedition Planning. Editor C. S. Cockell (Escondido, CA: Univelt Publishers), 363–386.
Schuerger, A. C., and Mitchell, D. J. (1992). Effects of Temperature, Hydrogen Ion Concentration, Humidity, and Light Quality on Disease Caused by Fusarium solani f. sp. Phaseoli in Mung Bean. Can. J. Bot. 70, 1798–1808. doi:10.1139/b92-223
Schuerger, A. C., Wheeler, R. M., Levine, H. G., Paul, A.-L., and Ferl, R. J. (Forthcoming 2021b). “Vegetable Health Challenges in Extraterrestrial Production,” in Plant Disease Management: Handbook of Vegetable and Herb Diseases. Editors M. T. McGrath, W. H. Elmer, and R. J. McGovern (New York City, NY: Springer Publishing).
Silverstone, S. E., and Nelson, M. (1996). Food Production and Nutrition in Biosphere 2: Results from the First mission September 1991 to September 1993. Adv. Space Res. 18, 49–61. doi:10.1016/0273-1177(95)00861-8
Stanghellini, M. E., Rasmussen, S. L., and Kim, D. H. (1999). Aerial Transmission of Thielaviopsis basicola, a Pathogen of Corn-Salad, by Adult Shore Flies. Phytopathology 89, 476–479. doi:10.1094/phyto.1999.89.6.476
Stout, S. C., Porterfield, D. M., Briarty, L. G., Kuang, A., and Musgrave, M. E. (2001). Evidence of Root Zone Hypoxia in Brassica rapa L. Grown in Microgravity. Int. J. Plant Sci. 162, 249–255. doi:10.1086/319585
Taylor, G. R. (1974). Recovery of Medically Important Microorganisms from Apollo Astronauts. Aerosp Med. 45, 824–828.
Wheeler, R. M. (2017). Agriculture for Space: People and Places Paving the Way. Open Agric. 2, 14–32. doi:10.1515/opag-2017-0002
Wheeler, R. M. (2004). Horticulture for Mars. Acta Hortic. 642, 201–215. doi:10.17660/actahortic.2004.642.22
Whitefield, A. E., Falk, B. W., and Rotenberg, D. (2015). Insect Vector-Mediated Transmission of Plant Viruses. Virology 479/480, 278–289.
Keywords: Bioregenerative life support system, BLSS, Veggie, Advanced Plant Habitat, phytopathogens, space exploration, integrated pest management, IPM
Citation: Schuerger AC (2021) Integrated Pest Management Protocols for Space-Based Bioregenerative Life Support Systems. Front. Astron. Space Sci. 8:759641. doi: 10.3389/fspas.2021.759641
Received: 16 August 2021; Accepted: 13 October 2021;
Published: 22 November 2021.
Edited by:
Lucie Poulet, NASA Postdoctoral Program, United StatesReviewed by:
Mitchell Roth, The Ohio State University, United StatesCopyright © 2021 Schuerger. This is an open-access article distributed under the terms of the Creative Commons Attribution License (CC BY). The use, distribution or reproduction in other forums is permitted, provided the original author(s) and the copyright owner(s) are credited and that the original publication in this journal is cited, in accordance with accepted academic practice. No use, distribution or reproduction is permitted which does not comply with these terms.
*Correspondence: Andrew C. Schuerger, c2NodWVyZ0B1ZmwuZWR1
Disclaimer: All claims expressed in this article are solely those of the authors and do not necessarily represent those of their affiliated organizations, or those of the publisher, the editors and the reviewers. Any product that may be evaluated in this article or claim that may be made by its manufacturer is not guaranteed or endorsed by the publisher.
Research integrity at Frontiers
Learn more about the work of our research integrity team to safeguard the quality of each article we publish.