- 1Center for the Utilization of Biological Engineering in Space (CUBES), Berkeley, CA, United States
- 2Department of Bioengineering, University of California Berkeley, Berkeley, CA, United States
- 3Department of Chemical and Biomolecular Engineering, University of California Berkeley, Berkeley, CA, United States
- 4Department of Chemical Engineering, University of California Davis, Davis, CA, United States
- 5Department of Civil and Environmental Engineering, Stanford University, Stanford, CA, United States
- 6Department of Mechanical and Aerospace Engineering, University of Florida, Gainesville, FL, United States
- 7Department of Plant and Microbial Biology, University of California Berkeley, Berkeley, CA, United States
- 8Department of Materials Science and Engineering, University of California Berkeley, Berkeley, CA, United States
- 9Department of Chemistry and Biochemistry, Utah State University, Logan, UT, United States
- 10Department of Plants, Soils, and Climate, Utah State University, Logan, UT, United States
- 11Department of Chemistry, Stanford University, Stanford, CA, United States
- 12Department of Horticultural Sciences, Texas A&M University, College Station, TX, United States
- 13Lawrence Berkeley National Laboratory, Berkeley, CA, United States
- 14Global HealthShare Initiative, Davis, CA, United States
- 15Kavli Energy Nanosciences Institute, Berkeley, CA, United States
A crewed mission to and from Mars may include an exciting array of enabling biotechnologies that leverage inherent mass, power, and volume advantages over traditional abiotic approaches. In this perspective, we articulate the scientific and engineering goals and constraints, along with example systems, that guide the design of a surface biomanufactory. Extending past arguments for exploiting stand-alone elements of biology, we argue for an integrated biomanufacturing plant replete with modules for microbial in situ resource utilization, production, and recycling of food, pharmaceuticals, and biomaterials required for sustaining future intrepid astronauts. We also discuss aspirational technology trends in each of these target areas in the context of human and robotic exploration missions.
1 Introduction
Extended human stay in space or upon the surface of alien worlds like Mars introduces new mission elements that require innovation (Musk, 2017); among these are the biotechnological elements (Menezes et al., 2015a; Menezes et al., 2015b; Nangle et al., 2020a) that support human health, reduce costs, and increase operational resilience. The potential for a Mars mission in the early 2030s (Drake et al., 2010) underscores the urgency of developing a roadmap for advantageous space biotechnologies.
A major limiting factor of space exploration is the cost of launching goods into space (Wertz and Larson, 1996). The replicative capacity of biology reduces mission launch cost by producing goods on-demand using in situ resources (Rapp, 2013), recycling waste products (Hendrickx et al., 2006), and interacting with other biological processes for stable ecosystem function (Gòdia et al., 2002). This trait not only lowers initial launch costs, but also minimizes the quantity and frequency of resupply missions that would otherwise be required due to limited food and pharmaceutical shelf-life (Du et al., 2011) on deep space missions. Biological systems also provide robust utility via genetic engineering, which can provide solutions to unforeseen problems and lower inherent risk (Menezes et al., 2015a; Berliner et al., 2019). For example, organisms can be engineered on-site to produce a pharmaceutical to treat an unexpected medical condition when rapid supply from Earth would be infeasible (McNulty et al., 2021). A so-called “biomanufactory” for deep space missions (Menezes, 2018) based on in situ resource utilization and composed of integrated biologically-driven subunits capable of producing food, pharmaceuticals, and biomaterials (Figure 1) will greatly reduce launch and resupply cost, and is therefore critical to the future of human-based space exploration (Menezes et al., 2015a; Nangle et al., 2020a).
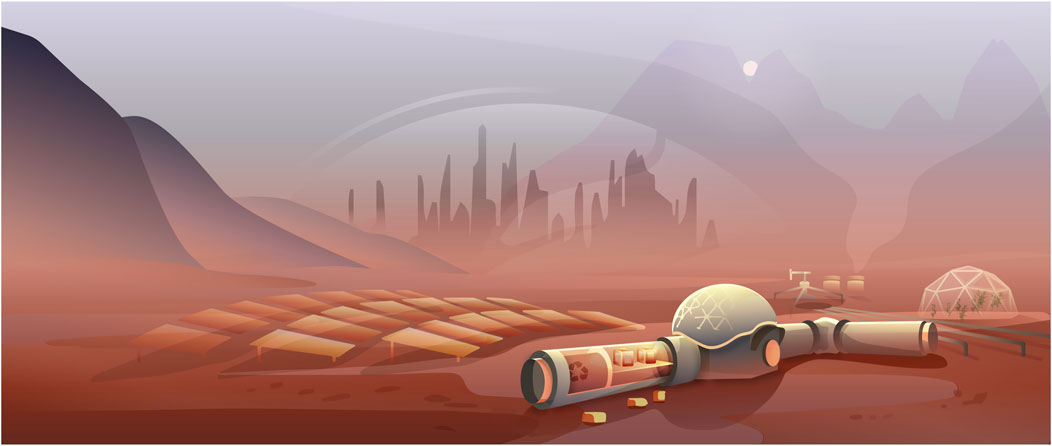
FIGURE 1. Artist’s rendering of a crewed Martian biomanufactory powered by photovoltaics, fed via atmospheric ISRU, and capable of food and pharmaceutical synthesis (FPS), in situ manufacturing (ISM), and biological loop closure (LC). Artwork by Davian Ho.
2 Feasibility, Needs, and Mission Architecture
The standard specifications for Mars exploration from 2009 (Drake et al., 2010) to 2019 (Linck et al., 2019) are not biomanufacturing-driven (Berliner et al., 2019) due to the novelty of space bioengineering. Here, we outline biotechnological support to produce food, medicine, and specialized construction materials on a long-term mission with six crew-members and surface operations for
The proposed biomanufactory would augment processes for air generation and water and waste recycling and purification—typically associated with Environmental Control and Life Support Systems (ECLSS) (Gòdia et al., 2002; Hendrickx et al., 2006)—since its needs overlap but are broader, and drive a wider development of an array of ISRU, in situ manufacturing (ISM), food and pharmaceutical synthesis (FPS), and loop closure (LC) technologies (Figure 2).
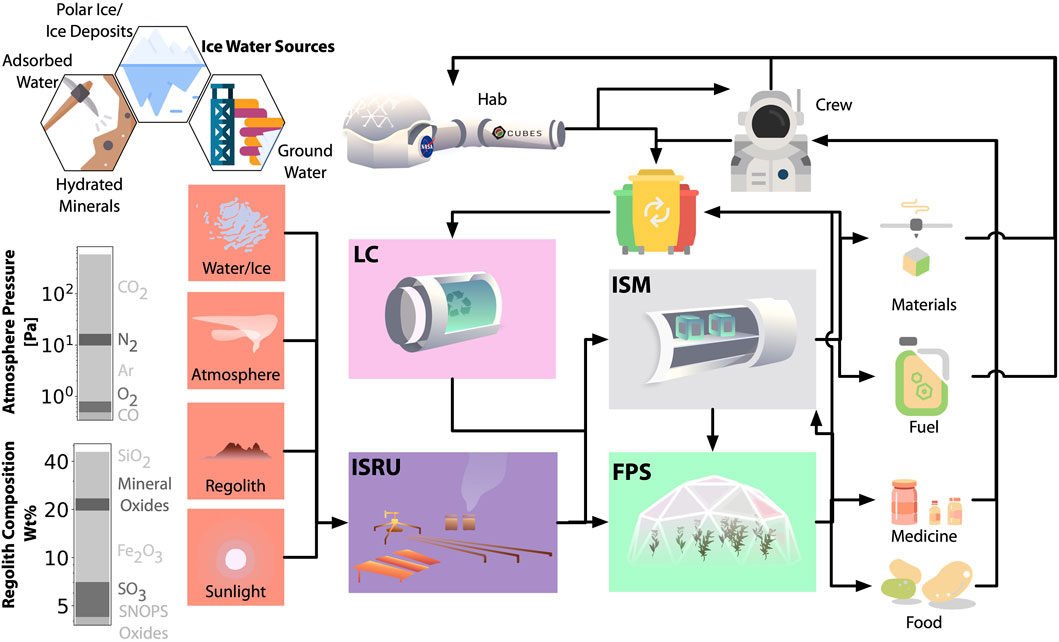
FIGURE 2. Proposed surface operations are drawn from inventories of in situ resources (red) such as ice, atmosphere, regolith, and sunlight. Atmospheric feedstocks of carbon and nitrogen are biologically fixed via the ISRU (in situ resource utilization) biomanufactory components (including abiotic processes, purple), providing the source of biopolymer manufacturing via the ISM (in situ manufacturing) component (grey) and food via the FPS (food and pharmaceutical synthesis) component (green), which are used for astronaut consumption and utilization during mission operations. Waste from each of these elements is collected and fed into the LC (loop closure) element (pink) to maximize efficiency and reduce the cost of supply logistics from Earth.
Food, medicine, and gas exchange to sustain humans imposes important ECLSS feasibility constraints (Yeh et al., 2005; Yeh et al., 2009; Weber and Schnaitmann, 2016). These arise from a crewmember (CM) physiological profile, with an upper-bound metabolic rate of
2.1 Biomanufactory Systems Engineering
Efficiency gains in a biomanufactory come in part from the interconnection (Figure 2) and modularity of various unit operations (Figures 3–6) (Crowell et al., 2018). However, different mission stage requirements for assembly, operation, timing, and productivity can lead to different optimal biomanufactory system configurations. A challenge therefore exists for technology choice and process optimization to address the high flexibility, scalability, and infrastructure minimization needs of an integrated biomanufactory. Current frameworks for biomanufacturing optimization do not dwell on these aspects. A series of new innovations in modeling processes and developing performance metrics specific to ECLSS biotechnology is called for, innovations that can suitably capture risk, modularity, autonomy, and recyclability. Concomitant invention in engineering infrastructure will also be required.
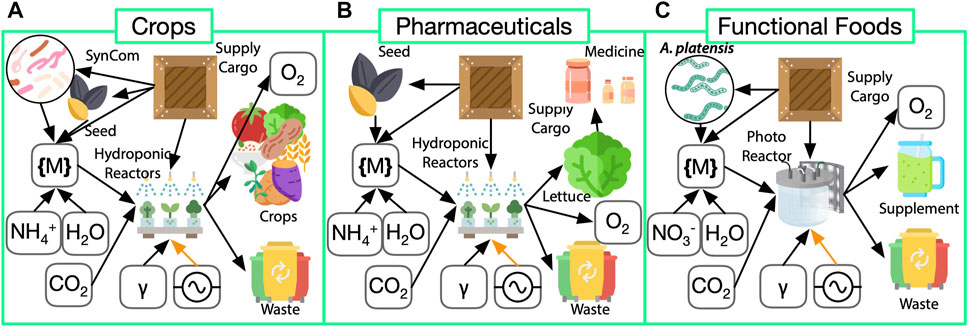
FIGURE 3. FPS (green in Figure 2) system breakdown for biomanufactory elements of (A) crops, (B) a biopharmaceutical, and (C) functional food production. In all cases, growth reactors require power (electrical current symbol ) and light (γ). (A) Crop biomass and oxygen gas (
3 Food and Pharmaceutical Synthesis
An estimated
FPS organisms for Mars use must be optimized for growth and yields of biomass, nutrient, and pharmaceutical accumulation. Providing adequate and appropriate lighting will be a challenge of photoautotrophic-centric FPS on Mars (Massa et al., 2007; Kusuma et al., 2020). Developing plants and algae with reduced chloroplast light-harvesting antenna size has the potential to improve whole-organism quantum yield by increasing light penetration deeper into the canopy, which will reduce the fraction of light that is wastefully dissipated as heat and allow higher planting density (Friedland et al., 2019). Developing FPS organisms for pharmaceutical production is especially complicated, given the breadth of production modalities and pharmaceutical need (e.g., the time window of intervention response, and molecule class) (McNulty et al., 2021). Limited-resource pharmaceutical purification is also a critically important consideration that has not been rigorously addressed. Promising biologically-derived purification technologies (Werner et al., 2006; Mahmoodi et al., 2019) should be considered for processing drugs that require very high purity (e.g., injectables).
Developing FPS growth systems for Mars requires synergistic biotic and abiotic optimization, as indicated by lighting systems and plant microbiomes. For lighting, consider that recent advancements in LED efficiency now make LEDs optimal for crop growth in extraterrestrial systems (Hardy et al., 2020). The ideal spectra from tunable LEDs will likely be one with a high fraction of red photons for maximum production efficiency, but increasing the fraction of shorter wavelength blue photons could increase crop quality (Johkan et al., 2010; Kusuma et al., 2021). Similarly, higher photon intensities increase production rates but decrease production efficiency. Understanding the associated volume and power/cooling requirement tradeoffs will be paramount to increasing overall system efficiency.
For microbiomes, consider that ISS open-air plant cultivation results in rapid and widespread colonization by atypicaly low-diversity bacterial and fungal microbiomes that often lead to plant disease and decreased plant productivity (Khodadad et al., 2020). Synthetic microbial communities (SynComs, Figure 3A) may provide stability and resilience to the plant microbiome and simultaneously improve the phenotype of host plants via the genes carried by community members. A subset of naturally occurring microbes are well known to promote growth of their plant hosts (Hassani et al., 2018), accelerate wastewater remediation and nutrient recycling (Nielsen, 2017), and shield plant hosts from both abiotic and biotic stresses (Caddell et al., 2019), including opportunistic pathogens (Bishop et al., 1997; Ryba-White et al., 2001; Leach et al., 2007). While SynCom design is challenging, the inclusion of SynComs in life support systems represents a critical risk-mitigation strategy to protect vital food and pharma resources. The application of SynComs to Mars-based agriculture motivates additional discussions in tradeoffs between customized hydroponics versus regolith-based farming, both of which will require distinct technology platforms and applied SynComs.
3.1 FPS Integration Into the Biomanufactory
Our biomanufactory FPS module has three submodules: crops, pharmaceuticals, and functional foods (Figure 3). The inputs to all three submodules (Figure 3) are nearly identical in needing
All submodules will have increased risk, modularity, and recyclability relative to traditional technological approaches. Increased risk is associated with biomass loss due to lower-than-expected yields, contamination, and possible growth system failure. Increased modularity over shipping known pharmaceuticals to Mars derives from the programmability of biology, and the rapid response time of molecular pharming in crops for as-needed production of biologics. Increased recyclability stems from the lack of packaging required for shipping food and pharmaceuticals from Earth, as well as the ability to recycle plant waste using anaerobic digestion.
At a systems integration level, FPS organism care will increase the crew time requirements for setup, maintenance, and harvesting compared to advance food and pharmaceutical shipments. However, overall cost impacts require careful scrutiny: crop growth likely saves on shipping costs, whereas pharmaceutical or functional food production on Mars may increase costs relative to shipping drugs and vitamins from Earth.
4 In Situ Materials Manufacturing
Maintaining FPS systems requires cultivation vessels/chambers, support structures, plumbing, and tools. Such physical objects represent elements of an inventory that, for short missions, will likely be a combination of predeployment cargo and supplies from the crewed transit vehicle (Drake et al., 2010). As mission duration increases, so does the quantity, composition diversity, and construction complexity of these objects. The extent of ISM for initial exploration missions is not currently specified (Drake et al., 2010). Nevertheless, recent developments (Owens et al., 2015; Moses and Bushnell, 2016; Owens and De Weck, 2016) imply that ISM will be critical for the generation of commodities and consumables made of plastics (Carranza et al., 2006), metals (Everton et al., 2016), composite-ceramics (Karl et al., 2020), and electronics (Werkheiser, 2015) as mission objects, with uses ranging from functional tools (Grenouilleau et al., 2000) to physical components of the life-supporting habitat (Owens et al., 2015).
Plastics will make up the majority of high-turnover items with sizes on the order of small parts to bench-top equipment, and will also account for contingencies (Prater et al., 2016). Biotechnology—specifically synthetic biology—in combination with additive manufacturing (Rothschild, 2016) has been proposed an a critical element towards the establishment of offworld manufacturing (Snyder et al., 2019) and can produce such polymeric constructs from basic feedstocks in a more compact and integrated way than chemical synthesis, because microbial bioreactors operate much closer to ambient conditions than chemical processes (Malik et al., 2015). The versatility of microbial metabolisms allows direct use of
A class of bioplastics that can be directly obtained from microorganisms (Naik et al., 2008) are polyhydroxyalkanoates (PHAs). While the dominant natural PHA is poly (3-hydroxybutyrate) (PHB), microbes can produce various co-polymers with an expansive range of physical properties (Myung et al., 2017). This is commonly accomplished through co-feeding with fatty acids or hydroxyalkanoates, which get incorporated in the polyester. These co-substrates can be sourced from additional process inputs or generated in situ. For example the PHA poly-lactic acid (PLA) can be produced by engineered Escherichia coli (Jung et al., 2010), albeit to much lower weight percent than is observed in organisms producing PHAs naturally. PHA composition can be modulated in other organisms (Rehm, 2010). The rapid development of synthetic biology tools for non-model organisms opens an opportunity to tune PHA production in high PHB producers and derive a range of high-performance materials.
Before downstream processing (melting, extrusion/molding), the intracellularly accumulating bioplastics need to be purified. The required degree of purity determines the approach and required secondary resources. Fused filament fabrication 3D-printing, which works well in microgravity (Prater et al., 2016; Prater et al., 2018), has been applied for PLA processing and may be extendable to other bio-polyesters. Ideally, additive manufacturing will be integrated in-line with bioplastics production and filament extrusion.
4.1 ISM Integration Into the Biomanufactory
Figure 4 depicts the use of three organism candidates from genera Cupriavidus, Methylocystis, and Halomonas that can meet bioplastic production. This requires a different set of parameters to optimize their deployment, which strongly affects reactor design and operation. These microbes are capable of using a variety of carbon sources for bioplastic production, each with a trade-off. For example, leveraging
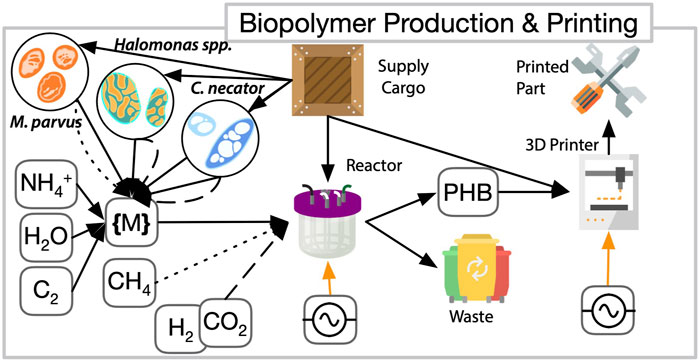
FIGURE 4. ISM (grey in Figure 2) systems breakdown for biomanufactory elements of biopolymer production and 3D-printing. 3D printed parts are fabricated from bioproduced plastics. Biopolyesters such as PHB, along with corresponding waste products, are formed in cargo-supplied reactors with the aid of microorganisms. A variety of available carbon feedstocks can serve as substrates for aerobic auto-, hetero-, or mixotrophic microorganisms such as Cupriavidus necator, Methylocystis parvus and Halomonas spp. All three microbes are capable of using
Bioplastic recovery and purification is a major challenge. To release the intracellular compound, an osmolysis process (Rathi et al., 2013) may be employed with the halophile (Tan et al., 2011; Chen et al., 2017). However, the transfer of cells into purified water and separation of the polyesters from the cell debris, potentially through several washing steps, may require substantial amounts of water. An alternative and/or complement to the common process for extraction of PHAs with halogenated organic solvents, is to use acetate or methanol as solvents (Anbukarasu et al., 2015; Aramvash et al., 2018). This is applicable independent of the organism and the inputs can be provided from other biomanufactory modules.
The high crystallinity of pure PHB makes it brittle and causes it to have a narrow melting range, resulting in warp during extrusion and 3D-printing. Such behavior places operational constraints on processing and hampers applications to precision manufacturing (Marchessault and Yu, 2005). Workarounds may be through additives, biocomposite synthesis, and copolymerization. However, this ultimately depends on what biology can provide (Müller and Seebach, 1993). There is a need to advance space bio-platforms to produce more diverse PHAs through synthetic biology.
ISM of biomaterials can reduce the mission cost, increase modularity, and improve system recyclability compared to abiotic approaches. In an abiotic approach, plastics will be included in the payload, thereby penalizing up-mass at launch. As with elements of FPS and ISRU, ISM increases flexibility and can create contingencies during surface operations, therefore reducing mission risk. The high modularity of independent plastic production, filament formation, and 3D-printing allows for a versatile process, at the cost of greater resources required for systems operations. Overall, this maximizes resource use and recyclability, by utilizing mission waste streams and byproducts for circular resource management.
5 In Situ Resource Utilization
Biomanufacturing on Mars can be supported by flexible biocatalysts that extract resources from the environment and transform them into the complex products needed to sustain human life. The Martian atmosphere contains
Although photosynthetic organisms are attractive for FPS, a higher demand for carbon-rich feedstocks and other chemicals necessitates a more rapid and efficient
Biological
Regolith provides a significant inventory for trace elements (Fe, K, P, S, etc.) and, when mixed with the substantial cellulosic biomass waste from FPS processes, can facilitate recycling organic matter into fertilizer to support crop growth. However, regolith use is hampered by widespread perchlorate (Catling et al., 2010; Cull et al., 2010; Navarro-González et al., 2010), indicating that decontamination is necessary prior to enrichment or use. Dechlorination can be achieved via biological perchlorate reduction using one of many dissimilatory perchlorate reducing organisms (Byrne-Bailey and Coates, 2012; Davila et al., 2013; Wetmore et al., 2015; Bywaters and Quinn, 2016). Efforts to reduce perchlorate biologically have been explored independently and in combination with a more wholistic biological platform (Llorente et al., 2018). Such efforts to integrate synthetic biology into human exploration missions suggest that a number of approaches should be considered within a surface biomanufactory.
5.1 ISRU Integration Into the Biomanufactory
A biomanufactory must be able to produce and utilize feedstocks along three axes as depicted in Figure 5:
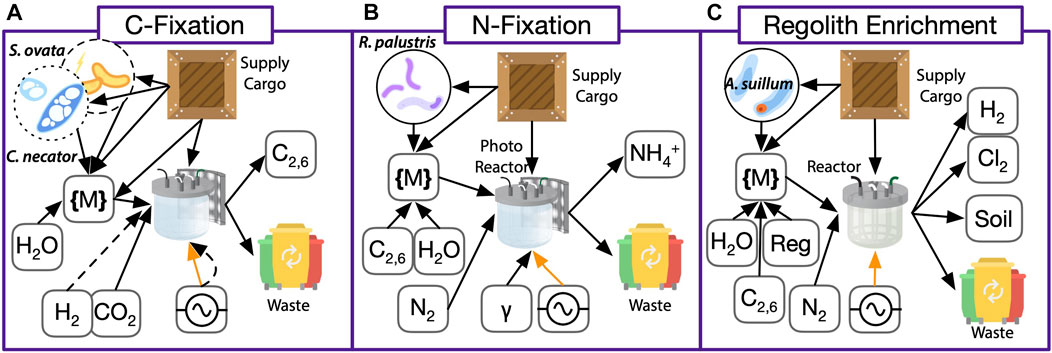
FIGURE 5. ISRU (purple in Figure 2) system breakdown of biomanufactory elements. (A) Carbon fixation with the autotrophic bacteria Sporomusa ovata or Cupriavidus necator through electrosynthesis or lithoautotrophic fixation of
Replicate ISRU bioreactors operating continuously in parallel with back-up operations lines can ensure a constant supply of the chemical feedstocks, commodity chemicals, and biomass for downstream processing in ISM and FPS operations. Integration of ISRU technologies with other biomanufactory elements, especially anaerobic digestion reactors, may enable (near-)complete recyclability of raw materials, minimizing resource consumption and impact on the Martian environment (MacElroy and Wang, 1989; Pogue et al., 2002).
6 Loop Closure and Recycling
Waste stream processing to recycle essential elements will reduce material requirements in the biomanufactory. Typical feedstocks include inedible crop mass, human excreta, and other mission wastes. Space mission waste management traditionally focuses on water recovery and efficient waste storage through warm air drying and lyophilization (Yeh et al., 2005; Anderson et al., 2018). Mission trash can be incinerated to produce
Microbes that recover resources from mission wastes are a viable option to facilitate loop closure. Aerobic composting produces
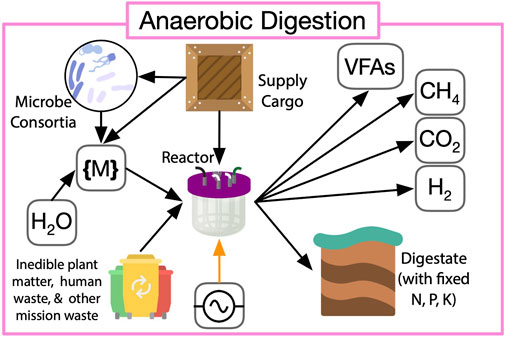
FIGURE 6. LC-based (pink in Figure 2) anaerobic digestion of mission waste such as inedible plant matter, microbial biomass, human, and other wastes produce methane, volatile fatty acids (VFAs), and digestate rich with key elemental nutrients (N, P, K), thereby supplementing ISRU operation.
Because additional infrastructure and utilities are necessary for waste processing, the extent of loop closure that is obtainable from a treatment route must be analyzed to balance yield with its infrastructure and logistic costs. Anaerobic digestion performance is a function of the composition and pretreatment of input waste streams (crop residuals, feces, urine, end-of-life bioproducts), as well as reaction strategies like batch or continuous, number of stages, and operation conditions such as organic loading rate, solids retention time, operating temperature, pH, toxic levels of inhibitors (
6.1 LC Integration Into the Biomanufactory
FPS and ISM waste as well as human waste are inputs for an anaerobic digester, with output recycled products supplementing the ISRU unit. Depending on the configuration of the waste streams from the biomanufactory and other mission elements, the operating conditions of the process can be varied to alter the efficiency and output profile. Open problems include the design and optimization of waste processing configurations and operations, and the identification of optimal end-product distributions based on a loop closure metric (Benvenuti et al., 2020) against mission production profiles, mission horizon, biomanufacturing feedstock needs, and the possible use of leftover products by other mission elements beyond the biomanufactory. A comparison with abiotic waste treatment strategies (incineration and pyrolysis) is also needed, checking power demand, risk, autonomy, and modularity benefits.
7 Discussion and Roadmap
Biomanufactory development must be done in concert with planned NASA missions that can provide critical opportunities to test subsystems and models necessary to evaluate efficacy and technology readiness levels (TRLs) (Mankins, 1995). Figure 7 is our attempt to place critical elements of a biomanufactory roadmap into this context. We label critical mission stages using Reference Mission Architecture (RMA)-S and RMA-L, which refer to Mars surface missions with short (
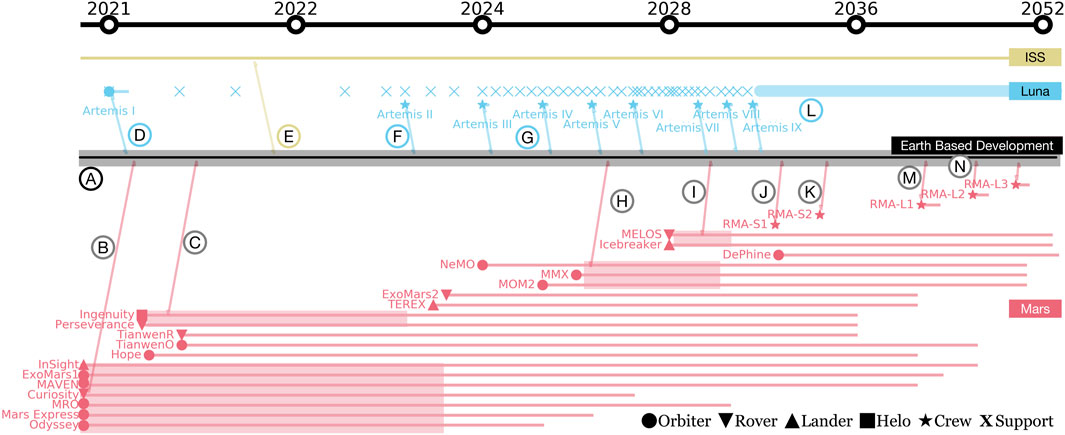
FIGURE 7. Proposed roadmap from 2021 to 2052 in log2-scale time of Earth-based developments (black) and their relationships to ISS (gold), lunar (blue), and Martian (red) missions. Missions range in status from currently operational, to enroute, planned, and proposed. Reference Mission Architecture (RMA)-S is a 30-sol mission, and RMA-L are missions with more than 500 sols of surface operations. RMA-L1 is the mission target for deployment of a biomanufactory. An arrival at target location is denoted with a symbol to indicate its type as orbiter, rover, lander, helicopter, support, or crewed operations. Circled letters are colored by location and correspond to specific milestones or opportunities for biomanufactory development.
Reliance on biotechnology can increase the risk of forward biological contamination (Piaseczny et al., 2019). Beyond contamination, there are ethical issues that concern both the act of colonizing a new land and justifying the cost and benefits of a mission given needs of the many here on earth. Our roadmap begins with the call for an extensive and ongoing discussion of ethics (Figure 7 Ⓐ). Planetary protection policies can provide answers or frameworks to address extant ethical questions surrounding deep-space exploration, especially on Mars (Rivkin et al., 2020; Tavares et al., 2020). Critically, scientists and engineers developing these technologies cannot be separate or immune to such policy development.
7.1 Autonomous Martian Surface Missions
Figure 7 Ⓑ denotes the interconnection between current Martian mission objects (Mars InSight Landing Pres, 2018; Mars Science Laboratory L, 2012; Baldwin et al., 2016; MAVEN Press Kit, 2013; Mars Reconnaissance Orbit, 2006; Mars Express: A Decade of Observing the Red Planet, 2013; Mars Odyssey Arrival Pres, 2001) and Earth-based process development elements for a biomanufactory (Figures 3–6). Together with en route autonomous surface missions (Mars Helicopter/Ingenuity, 2020; Mars 2020 Perseverance La, 2020) (Figure 7 Ⓒ), these missions provide a roadmap for continued mission development based on landing location biosignatures (Bussey and Hoffman, 20162016; Vago et al., 2017). The biomanufactory (Figures 3–6) will require ample water in media, atmospheric gas feedstocks, and power that can be bounded by measurements from autonomous missions. Upcoming sample return missions offer an opportunity to shape the design of ISRU processes such as regolith decontamination from perchlorate and nitrogen enrichment for crop growth. Additional orbiters (Jedrey et al., 2016) and lander/rover pairs (Figure 7 Ⓗ) have been planned and will aid in the selection of a landing site for short term Martian exploration missions (Figure 7 Ⓙ, Ⓚ). Such locations will be determined based on water/ice mining/availability (McKay et al., 2013) as depicted in Figure 7 Ⓘ. These missions can be deployed with specific payloads to experimentally validate biomanufactory elements. Low TRL biotechnologies can be flown as experimental packages on upcoming rovers and landers, offering the possibility for TRL advancement of biology-driven subsystems. Planning for such testing will require coordination with, and validation on, ISS and satellite payloads (Figure 7 Ⓔ), for instance, to understand the impact of Martian gravity, to contrast levels of radiation exposure, and so on.
7.2 Artemis Operations
The upcoming lunar exploration missions, Artemis (Smith et al., 2020) and Gateway (Crusan et al., 2019), provide additional opportunities for integration with Earth-based biomanufactory development. Early support missions (Figure 7 Ⓓ, Ⓕ) will provide valuable experience in cargo predeployment for crewed operations, and is likely to help shape logistics development for short-term (Figure 7 Ⓙ, Ⓚ) as well as long-term Mars exploration missions (Figure 7 Ⓜ) when a biomanufactory can be deployed. Here we present a subset of Artemis efforts as they relate to mission elements with opportunities for testing and maturing biomanufacturing technology. Although ISRU technologies for the Moon and Mars will be sufficiently distinct due to different resource availabilities, crewed Artemis missions (Figure 7 Ⓕ, Ⓖ) provide a testing ground for crewed Mars bioprocess infrastructure. Later Artemis missions (Figure 7 Ⓛ) also provide a suitable environment to test modular, interlocked, scalable reactor design, as well as the design of compact molecular biology labs for DNA synthesis and transformation. Since these technologies are unlikely to be mission critical during Artemis, their TRL can be increased and their risk factors studied through in-space evaluation.
The Artemis missions also provide a testbed to evaluate the space-based evolution of microbes and alterations of seedstocks as a risk inherent to the biological component of the biomanufactory. This risk can be mitigated by incorporating backup seed and microbial freezer stocks to reset the system. However, ensuring that native and/or engineered traits remain robust over time is critical to avoid the resource penalties that are inherent to such a reset. Consequently, while optimal organisms and traits can be identified and engineered prior to a mission, testing their long-term performance on future NASA missions prior to inclusion in life support systems will help to assess whether engineered traits are robust to off-planet growth, whether microbial communities are stable across crop generations, and whether the in situ challenges that astronauts will face when attempting to reset the biomanufacturing system are surmountable. Quantifying these uncertainties during autonomous and crewed Artemis missions will inform tradeoff and optimization studies during the design of an enhanced life support system for Martian surface bio-operations.
7.3 Human Exploration of Mars
Crewed surface operations of
The RMA-S1 and RMA-S2 crews will be exposed for the first time to surface conditions after interplanetary travel, allowing for an initial assessment of health effects that can be contrasted to operations on the lunar surface (Figure 7 Ⓕ), and that may be alleviated by potential biomanufactory pharmaceutical and functional food outputs (Figures 3B,C). The RMA-S1 and RMA-S2 mission ISRU and FPS experiments will also provide insight into the input requirements for downstream biomanufactory processes. ISM technologies such as bioplastic synthesis and additive manufacture (Figure 4) can be evaluated for sufficient TRL. Further, loop closure performance for several desired products can also be tested. This will help estimate the impact of waste stream characteristics changes on recycling (Brémond et al., 2018).
7.4 Moving Forward
We have outlined the design and future deployment of a biomanufactory to support human surface operations during a 500 days manned Mars mission. We extended previous stand-alone biological elements with space use potential into an integrated biomanufacturing system by bringing together the important systems of ISRU, synthesis, and recycling, to yield food, pharmaceuticals, and biomaterials. We also provided an envelope of future design, testing, and biomanufactory element deployment in a roadmap that spans Earth-based system development, testing on the ISS, integration with lunar missions, and initial construction during shorter-term initial human forays on Mars. The innovations necessary to meet the challenges of low-cost, energy and mass efficient, closed-loop, and regenerable biomanufacturing for space will undoubtedly yield important contributions to forwarding sustainable biomanufacturing on Earth. We anticipate that the path towards instantiating a biomanufactory will be replete with science, engineering, and ethical challenges. But that is the excitement—part-and-parcel—of the journey to Mars.
Data Availability Statement
The original contributions presented in the study are included in the article, further inquiries can be directed to the corresponding authors.
Author Contributions
AJB, JMH, AJA, and APA conceived the concept based on the Center for the Utilization of Biological Engineering in Space (CUBES) that was established by AJB, BB, DSC, DCD, AM, SN, RMW, PY, CSC, KAM, LCS, AAM, and APA. All authors wrote and edited the manuscript with grouped efforts led by JMH, AJA, AJB, MJM, NJHA, SSG, and GM.
Funding
This material is based upon work supported by NASA under grant or cooperative agreement award number NNX17AJ31G.
Conflict of Interest
The authors declare that the research was conducted in the absence of any commercial or financial relationships that could be construed as a potential conflict of interest.
References
Abel, A. J., and Clark, D. S. (2020). A Comprehensive Modeling Analysis of Formate-Mediated Microbial Electrosynthesis, ChemSusChem.14, 344–355. doi:10.1002/cssc.202002079
Abel, A. J., Hilzinger, J. M., Arkin, A. P., and Clark, D. S. (2020). Systems-informed Genome Mining for Electroautotrophic Microbial Production. bioRxiv. doi:10.1101/2020.12.07.414987
Anbukarasu, P., Sauvageau, D., and Elias, A. (2015). Tuning the Properties of Polyhydroxybutyrate Films Using Acetic Acid via Solvent Casting. Sci. Rep. 5, 17884. doi:10.1038/srep17884
Anderson, M. S., Ewert, M. K., and Keener, J. F.. Life Support Baseline Values and Assumptions Document (2018).
Aramrueang, N., Rapport, J., and Zhang, R. (2016). Effects of Hydraulic Retention Time and Organic Loading Rate on Performance and Stability of Anaerobic Digestion of Spirulina Platensis. Biosyst. Eng. 147, 174–182. doi:10.1016/j.biosystemseng.2016.04.006
Aramvash, A., Moazzeni Zavareh, F., and Gholami Banadkuki, N. (2018). Comparison of Different Solvents for Extraction of Polyhydroxybutyrate fromCupriavidus Necator. Eng. Life Sci. 18, 20–28. doi:10.1002/elsc.201700102
Averesch, N. J. H. (2021). Choice of Microbial System for In-Situ Resource Utilization on Mars. Front. Astron. Space Sci. 8. doi:10.3389/fspas.2021.700370
[Dataset] Baldwin, E., Clark, S., Scuka, D., and O’Flaherty, K.. ExoMars 2016: Analysing Atmospheric Gases for Signs of Active Biological or Geological Processes on Mars, and Testing Key landing Technologies (2016).
Benton, M. (2008). “Crew and Cargo Landers for Human Exploration of Mars-Vehicle System Design,” in 44th AIAA/ASME/SAE/ASEE Joint Propulsion Conference {\&} Exhibit, 5156. doi:10.2514/6.2008-5156
Benvenuti, A. J., Drecksler, S. R., Sen Gupta, S., Menezes, A. A., J\∼Benvenuti, A., R\∼Drecksler, S., et al. (2020). “Design of Anaerobic Digestion Systems for Closed Loop Space Biomanufacturing,” in 50th International Conference on Environmental Systems (ICES).
Berliner, A. J., Makrygiorgos, G., Mirkovic, M., Menezes, A. A., Mesbah, A., and Arkin, A. P. (2019). Towards a Biomanufacturing-Driven Reference Mission Architecture for Long-Term Human Mars Exploration. LPICo 2089, 6261.
Bishop, D. L., Levine, H. G., Kropp, B. R., and Anderson, A. J. (1997). Seedborne Fungal Contamination: Consequences in Space-Grown Wheat. Phytopathology 87, 1125–1133. doi:10.1094/phyto.1997.87.11.1125
Brandl, H., Knee, E. J., Fuller, R. C., Gross, R. A., and Lenz, R. W. (1989). Ability of the Phototrophic Bacterium Rhodospirillum Rubrum to Produce Various Poly (β-Hydroxyalkanoates): Potential Sources for Biodegradable Polyesters. Int. J. Biol. Macromolecules 11, 49–55. doi:10.1016/0141-8130(89)90040-8
Brémond, U., de Buyer, R., Steyer, J.-P., Bernet, N., and Carrere, H. (2018). Biological Pretreatments of Biomass for Improving Biogas Production: an Overview from Lab Scale to Full-Scale. Renew. Sustain. Energ. Rev. 90, 583–604. doi:10.1016/j.rser.2018.03.103
Bussey, B., and Hoffman, S. J. (2016). “Human Mars landing Site and Impacts on Mars Surface Operations,” in IEEE Aerospace Conference (IEEE), 1–21. doi:10.1109/aero.2016.7500775
Byrne-Bailey, K. G., and Coates, J. D. (2012). Complete Genome Sequence of the Anaerobic Perchlorate-Reducing Bacterium Azospira Suillum Strain PS. J. Bacteriol. 194, 2767–2768. doi:10.1128/jb.00124-12
Bywaters, K. F., and Quinn, R. C. (2016). Perchlorate-Reducing Bacteria: Evaluating the Potential for Growth Utilizing Nutrient Sources Identified on Mars. Lunar Planet. Sci. Conf. 47, 2946.
Caddell, D. F., Deng, S., and Coleman-Derr, D. (2019). Role of the Plant Root Microbiome in Abiotic Stress Tolerance. Seed Endophytes. Springer Nature Switzerland AG, 273–311. doi:10.1007/978-3-030-10504-4_14
Cannon, K. M., and Britt, D. T. (2019). Feeding One Million People on Mars. New Space 7, 245–254. doi:10.1089/space.2019.0018
Caraccio, A. J., Hintze, P., Anthony, S. M., Devor, R. W., Captain, J. G., and Muscatello, A. C. (2013). “Trash-to-gas: Converting Space Trash into Useful Products,” in 43rd International Conference on Environmental Systems (ICES). doi:10.2514/6.2013-3440AIAA 2013-3440
Carranza, S., Makel, D. B., and Blizman, B. (2006). “In Situ manufacturing of Plastics and Composites to Support H{\&}R Exploration,” in AIP Conference Proceedings (American Institute of Physics), 813, 1122–1129.
Catling, D. C., Claire, M. W., Zahnle, K. J., Quinn, R. C., Clark, B. C., Hecht, M. H., et al. (2010). Atmospheric Origins of Perchlorate on Mars and in the Atacama. J. Geophys. Res. Planets 115. doi:10.1029/2009je003425
Chen, J., Li, W., Zhang, Z.-Z., Tan, T.-W., and Li, Z.-J. (2018). Metabolic Engineering of Escherichia coli for the Synthesis of Polyhydroxyalkanoates Using Acetate as a Main Carbon Source. Microb. Cel Fact 17, 102. doi:10.1186/s12934-018-0949-0
Chen, X., Yin, J., Ye, J., Zhang, H., Che, X., Ma, Y., et al. (2017). Engineering Halomonas Bluephagenesis TD01 for Non-sterile Production of Poly(3-Hydroxybutyrate-Co-4-Hydroxybutyrate). Bioresour. Technol. 244, 534–541. doi:10.1016/j.biortech.2017.07.149
Clark, D., and Clark, D. (1997). “In-situ Propellant Production on Mars-A Sabatier/electrolysis Demonstration Plant,” in 33rd Joint Propulsion Conference and Exhibit, 2764.
Cohen, M. M. (2015). “First Mars Habitat Architecture,” in AIAA SPACE 2015 Conference and Exposition, 4517. doi:10.2514/6.2015-4517
Crowell, L. E., Lu, A. E., Love, K. R., Stockdale, A., Timmick, S. M., Wu, D., et al. (2018). On-demand Manufacturing of Clinical-Quality Biopharmaceuticals. Nat. Biotechnol. 36, 988–995. doi:10.1038/nbt.4262
Crusan, J., Bleacher, J., Caram, J., Craig, D., Goodliff, K., Herrmann, N., et al. (2019). “NASA’s Gateway: An Update on Progress and Plans for Extending Human Presence to Cislunar Space,” in 2019 IEEE Aerospace Conference (IEEE), 1–19. doi:10.1109/aero.2019.8741561
Cull, S. C., Arvidson, R. E., Catalano, J. G., Ming, D. W., Morris, R. V., Mellon, M. T., et al. (2010). Concentrated Perchlorate at the Mars Phoenix landing Site: Evidence for Thin Film Liquid Water on Mars. Geophys. Res. Lett. 37. doi:10.1029/2010gl045269
Davila, A. F., Willson, D., Coates, J. D., and McKay, C. P. (2013). Perchlorate on Mars: a Chemical hazard and a Resource for Humans. Int. J. Astrobiology 12, 321–325. doi:10.1017/s1473550413000189
Doloman, A., and Seefeldt, L. C. (2020). An Experimentally Evaluated Thermodynamic Approach to Estimate Growth of Photoheterotrophic Purple Non-sulfur Bacteria. Front. Microbiol. 11, 540378. doi:10.3389/fmicb.2020.540378
Doud, D. F. R., Holmes, E. C., Richter, H., Molitor, B., Jander, G., and Angenent, L. T. (2017). Metabolic Engineering of Rhodopseudomonas Palustris for the Obligate Reduction of N-Butyrate to N-Butanol. Biotechnol. Biofuels 10, 1–11. doi:10.1186/s13068-017-0864-3
Drake, B. G., Hoffman, S. J., and Beaty, D. W. (2010). “Human Exploration of Mars, Design Reference Architecture 5.0,” in Aerospace Conference, 2010 IEEE (IEEE), 1–24. doi:10.1109/aero.2010.5446736
Du, B., Daniels, V. R., Vaksman, Z., Boyd, J. L., Crady, C., and Putcha, L. (2011). Evaluation of Physical and Chemical Changes in Pharmaceuticals Flown on Space Missions. Aaps J. 13, 299–308. doi:10.1208/s12248-011-9270-0
Everton, S. K., Hirsch, M., Stravroulakis, P., Leach, R. K., and Clare, A. T. (2016). Review of Iin-Ssitu Process Monitoring and Iin-Ssitu Metrology for Metal Additive Manufacturing. Mater. Des. 95, 431–445. doi:10.1016/j.matdes.2016.01.099
Friedland, N., Negi, S., Vinogradova-Shah, T., Wu, G., Ma, L., Flynn, S., et al. (2019). Fine-tuning the Photosynthetic Light Harvesting Apparatus for Improved Photosynthetic Efficiency and Biomass Yield. Sci. Rep. 9, 1–12. doi:10.1038/s41598-019-49545-8
Gòdia, F., Albiol, J., Montesinos, J. L., Pérez, J., Creus, N., Cabello, F., et al. (2002). MELISSA: a Loop of Interconnected Bioreactors to Develop Life Support in Space. J. Biotechnol. 99, 319–330. doi:10.1016/s0168-1656(02)00222-5
Grenouilleau, J. C., Housseini, O., and Pérès, F. (2000). In-Situ Rapid Spares Manufacturing and its Application to Human Space Missions. Space 2000, 42–48. doi:10.1061/40479(204)5
Hardy, M., Wheeler, R., Ewert, M., Kusuma, P., and Bugbee, B. (2020). “Providing Photons for Food in Regenerative Life Support: A Comparative Analysis of Solar Fiber Optic and Electric Light Systems,” in 50th International Conference on Environmental Systems (ICES).
Hassani, M. A., Durán, P., and Hacquard, S. (2018). Microbial Interactions within the Plant Holobiont. Microbiome 6, 58. doi:10.1186/s40168-018-0445-0
Heinrich, D., Raberg, M., Fricke, P., Kenny, S. T., Morales-Gamez, L., Babu, R. P., et al. (2016). Synthesis Gas (Syngas)-Derived Medium-Chain-Length Polyhydroxyalkanoate Synthesis in Engineered Rhodospirillum Rubrum. Appl. Environ. Microbiol. 82, 6132–6140. doi:10.1128/aem.01744-16
Hendrickx, L., De Wever, H., Hermans, V., Mastroleo, F., Morin, N., Wilmotte, A., et al. (2006). Microbial Ecology of the Closed Artificial Ecosystem MELiSSA (Micro-ecological Life Support System Alternative): Reinventing and Compartmentalizing the Earth's Food and Oxygen Regeneration System for Long-Haul Space Exploration Missions. Res. Microbiol. 157, 77–86. doi:10.1016/j.resmic.2005.06.014
Hindupur, A., Ball, N., Kagawa, H., Kostakis, A., Levri, J., Sims, K., et al. BioNutrients-1: On-Demand Production of Nutrients in Space (2019).
Hintze, P., Meier, A., and Shah, M. (2018). “Sabatier System Design Study for a Mars ISRU Propellant Production Plant,” in 48th International Conference on Environmental Systems.
Hintze, P. E., Caraccio, A., Anthony, S. M., DeVor, R., Captain, J. G., Tsoras, A., et al. (2013). “Trash-to-gas: Using Waste Products to Minimize Logistical Mass during Long Duration Space Missions,” in AIAA SPACE 2013 Conference and Exposition, 5326. doi:10.2514/6.2013-5326
Hoffman, S. J., and Kaplan, D. I. (1997). Human Exploration of Mars: The Reference mission of the NASA Mars Exploration Study Team, Vol. 6107. National Aeronautics and Space Administration, Lyndon B. Johnson Space Center.
[Dataset] Jedrey, T., Lock, R., and Matsumoto, M. Conceptual Studies for the Next Mars Orbiter (NeMO) (2016).
Johkan, M., Shoji, K., Goto, F., Hashida, S.-n., and Yoshihara, T. (2010). Blue Light-Emitting Diode Light Irradiation of Seedlings Improves Seedling Quality and Growth after Transplanting in Red Leaf Lettuce. horts 45, 1809–1814. doi:10.21273/HORTSCI.45.12.1809
Jung, Y. K., Kim, T. Y., Park, S. J., and Lee, S. Y. (2010). Metabolic Engineering ofEscherichia Colifor the Production of Polylactic Acid and its Copolymers. Biotechnol. Bioeng. 105, 161–171. doi:10.1002/bit.22548
Karl, D., Kamutzki, F., Lima, P., Gili, A., Duminy, T., Zocca, A., et al. (2020). Sintering of Ceramics for clay In Situ Resource Utilization on Mars. Open Ceramics 3. doi:10.1016/j.oceram.2020.100008
Khan, M. A., Ngo, H. H., Guo, W., Liu, Y., Zhang, X., Guo, J., et al. (2018). Biohydrogen Production from Anaerobic Digestion and its Potential as Renewable Energy. Renew. Energ. 129, 754–768. doi:10.1016/j.renene.2017.04.029
Khodadad, C. L. M., Hummerick, M. E., Spencer, L. E., Dixit, A. R., Richards, J. T., Romeyn, M. W., et al. (2020). Microbiological and Nutritional Analysis of Lettuce Crops Grown on the International Space Station. Front. Plant Sci. 11, 199. doi:10.3389/fpls.2020.00199
Kusuma, P., Pattison, P. M., and Bugbee, B. (2020). From Physics to Fixtures to Food: Current and Potential LED Efficacy. Hortic. Res. 7, 1–9. doi:10.1038/s41438-020-0283-7
Kusuma, P., Swan, B., and Bugbee, B. (2021). Does Green Really Mean Go? Increasing the Fraction of Green Photons Promotes Growth of Tomato but Not Lettuce or Cucumber. Plants. 10 (4). doi:10.3390/plants10040637
Kyriacou, M. C., De Pascale, S., Kyratzis, A., and Rouphael, Y. (2017). Microgreens as a Component of Space Life Support Systems: A Cornucopia of Functional Food. Front. Plant Sci. 8, 1587. doi:10.3389/fpls.2017.01587
Landis, G., Kerslake, T., Scheiman, D., and Jenkins, P. (2004). “Mars Solar Power,” in 2nd International Energy Conversion Engineering Conference, 5555. doi:10.2514/6.2004-5555
Landis, G. A. (2000). Solar Cell Selection for Mars. IEEE Aerosp. Electron. Syst. Mag. 15, 17–21. doi:10.1109/62.821659
Leach, J. E., Ryba-White, M., Sun, Q., Wu, C. J., Hilaire, E., Gartner, C., et al. (2007). Plants, Plant Pathogens, and Microgravity-Aa Deadly Trio. Gravit. Space Biol. Bull. 14, 15–23.
Li, H., Opgenorth, P. H., Wernick, D. G., Rogers, S., Wu, T.-Y., Higashide, W., et al. (2012). Integrated Electromicrobial Conversion of CO2 to Higher Alcohols. Science 335, 1596. doi:10.1126/science.1217643
Linck, E., Crane, K. W., Zuckerman, B. L., Corbin, B. A., Myers, R. M., Williams, S. R., et al. (2019). Evaluation of a Human Mission to Mars by 2033. Washington, DC: IDA Science and Technology Policy Institute.
Liskowsky, D. R., and Seitz, W. W. (2010). Human Integration Design Handbook. Washington, DC, Rep. NASA/SP-2010-3407. NASA.
Liu, C., Wang, W., Anwar, N., Ma, Z., Liu, G., and Zhang, R. (2018). Effect of Organic Loading Rate on Anaerobic Digestion of Food Waste under Mesophilic and Thermophilic Conditions. Energy Fuels 31, 2976–2984.
Liu, C., Colón, B. C., Ziesack, M., Silver, P. A., and Nocera, D. G. (2016). Water Splitting-Biosynthetic System with CO2reduction Efficiencies Exceeding Photosynthesis. Science 352, 1210–1213. doi:10.1126/science.aaf5039
Liu, C., Gallagher, J. J., Sakimoto, K. K., Nichols, E. M., Chang, C. J., Chang, M. C. Y., et al. (2015). Nanowire-Bacteria Hybrids for Unassisted Solar Carbon Dioxide Fixation to Value-Added Chemicals. Nano Lett. 15, 3634–3639. doi:10.1021/acs.nanolett.5b01254
Llorente, B., Williams, T., and Goold, H. (2018). The Multiplanetary Future of Plant Synthetic Biology. Genes 9, 348. doi:10.3390/genes9070348
Logan, B. E., Rossi, R., Ragab, A. a., and Saikaly, P. E. (2019). Electroactive Microorganisms in Bioelectrochemical Systems. Nat. Rev. Microbiol. 17, 307–319. doi:10.1038/s41579-019-0173-x
MacElroy, R. D., and Wang, D. (1989). Waste Recycling Issues in Bioregenerative Life Support. Adv. Space Res. 9, 75–84. doi:10.1016/0273-1177(89)90031-8
Mahmoodi, S., Pourhassan-Moghaddam, M., Wood, D. W., Majdi, H., and Zarghami, N. (2019). Current Affinity Approaches for Purification of Recombinant Proteins. Cogent Biol. 5, 1665406. doi:10.1080/23312025.2019.1665406
Malik, M. R., Yang, W., Patterson, N., Tang, J., Wellinghoff, R. L., Preuss, M. L., et al. (2015). Production of High Levels of Poly-3-Hydroxybutyrate in Plastids of Camelina Sativaseeds. Plant Biotechnol. J. 13, 675–688. doi:10.1111/pbi.12290
Marchessault, R. H., and Yu, G. (2005). Crystallization and Material Properties of Polyhydroxyalkanoates PHAs. Biopolymers Online Biol. Chem. Biotechnol. Appl. 3. doi:10.1002/3527600035.bpol3b07
Massa, G. D., Emmerich, J. C., Morrow, R. C., Bourget, C. M., and Mitchell, C. A. (2007). Plant-growth Lighting for Space Life Support: a Review. Gravit. Space Res. 19.
McKay, C. P., Stoker, C. R., Glass, B. J., Davé, A. I., Davila, A. F., Heldmann, J. L., et al. (2013). The Icebreaker Life Mission to Mars: a Search for Biomolecular Evidence for Life. Astrobiology 13, 334–353. doi:10.1089/ast.2012.0878
McNulty, M. J., Xiong, Y., Yates, K., Karuppanan, K., Hilzinger, J. M., Berliner, A. J., et al. (2021). Molecular Pharming to Support Human Life on the Moon, mars, and beyond. Crit. Rev. Biotechnol. 0, 1–16. doi:10.1080/07388551.2021.1888070
Meegoda, J., Li, B., Patel, K., and Wang, L. (2018). A Review of the Processes, Parameters, and Optimization of Anaerobic Digestion. Ijerph 15, 2224. doi:10.3390/ijerph15102224
Meier, A., Shah, M., Hintze, P., Petersen, E., and Muscatello, A. (2017). “Mars Atmospheric Conversion to Methane and Water: An Engineering Model of the Sabatier Reactor with Characterization of Ru/al2o3 for Long Duration Use on mars,” in 47th International Conference on Environmental Systems.
Menezes, A. A. (2018). Realizing a Self-Reproducing Space Factory with Engineered and Programmed Biology. LPICo 2063, 3145.
Menezes, A. A., Cumbers, J., Hogan, J. A., and Arkin, A. P. (2015). Towards Synthetic Biological Approaches to Resource Utilization on Space Missions. J. R. Soc. Interf. 12, 20140715. doi:10.1098/rsif.2014.0715 (The Royal Society)
Menezes, A. A., Montague, M. G., Cumbers, J., Hogan, J. A., and Arkin, A. P. (2015). Grand Challenges in Space Synthetic Biology. J. R. Soc. Interf. 12, 20150803. doi:10.1098/rsif.2015.0803
Miele, A., and Wang, T. (1999). Optimal Transfers from an Earth Orbit to a Mars Orbit. Acta Astronautica 45, 119–133. doi:10.1016/s0094-5765(99)00109-5
Möller, K., and Müller, T. (2012). Effects of Anaerobic Digestion on Digestate Nutrient Availability and Crop Growth: a Review. Eng. Life Sci. 12, 242–257. doi:10.1002/elsc.201100085
Moses, R. W., and Bushnell, D. M.. Frontier Iin-Ssitu Resource Utilization for Enabling Sustained Human Presence on mars (2016).
Müller, H.-M., and Seebach, D. (1993). Poly(hydroxyalkanoates): A Fifth Class of Physiologically Important Organic Biopolymers?. Angew. Chem. Int. Ed. Engl. 32, 477–502. doi:10.1002/anie.199304771
Musk, E. (2017). Making Humans a Multi-Planetary Species. New Space 5, 46–61. doi:10.1089/space.2017.29009.emu
Myung, J., Flanagan, J. C. A., Waymouth, R. M., and Criddle, C. S. (2017). Expanding the Range of Polyhydroxyalkanoates Synthesized by Methanotrophic Bacteria through the Utilization of omega-hydroxyalkanoate Co-substrates. AMB Expr. 7, 118. doi:10.1186/s13568-017-0417-y
Myung, J., Wang, Z., Yuan, T., Zhang, P., Van Nostrand, J. D., Zhou, J., et al. (2015). Production of Nitrous Oxide from Nitrite in Stable Type II Methanotrophic Enrichments. Environ. Sci. Technol. 49, 10969–10975. doi:10.1021/acs.est.5b03385
Naik, S., Venu Gopal, S. K., and Somal, P. (2008). Bioproduction of Polyhydroxyalkanoates from Bacteria: a Metabolic Approach. World J. Microbiol. Biotechnol. 24, 2307–2314. doi:10.1007/s11274-008-9745-z
Nangle, S. N., Wolfson, M. Y., Hartsough, L., Ma, N. J., Mason, C. E., Merighi, M., et al. (2020). The Case for Biotech on Mars. Nat. Biotechnol. 38, 401–407. doi:10.1038/s41587-020-0485-4
Nangle, S. N., Ziesack, M., Buckley, S., Trivedi, D., Loh, D. M., Nocera, D. G., et al. (2020). Valorization of CO2 through Lithoautotrophic Production of Sustainable Chemicals in Cupriavidus Necator. Metab. Eng. 62, 207–220. doi:10.1016/j.ymben.2020.09.002
Navarro-González, R., Vargas, E., de La Rosa, J., Raga, A. C., and McKay, C. P. (2010). Reanalysis of the Viking Results Suggests Perchlorate and Organics at Midlatitudes on Mars. J. Geophys. Res. Planets 115. doi:10.1029/2010je003599
Nielsen, P. H. (2017). Microbial Biotechnology and Circular Economy in Wastewater Treatment. Microb. Biotechnol. 10, 1102–1105. doi:10.1111/1751-7915.12821
Owens, A., and De Weck, O. (2016). Systems Analysis of In-Space Manufacturing Applications for the International Space Station and the Evolvable Mars Campaign. AIAA SPACE 2016, 5394. doi:10.2514/6.2016-5394
Owens, A., Do, S., Kurtz, A., and Weck, Od. (2015). “Benefits of Additive Manufacturing for Human Exploration of mars,” in 45th International Conference on Environmental Systems.
Pandurangan, M., and Kim, D. H. (2015). A Novel Approach for In Vitro Meat Production. Appl. Microbiol. Biotechnol. 99, 5391–5395. doi:10.1007/s00253-015-6671-5
Piaseczny, J., Brecht, A. S., Som, S. M., Tavares, F., and Wilhelm, M. B. (2019). Atmospheric Dispersal of Contamination Sourced from a Putative Human Habitat on Mars. AGUFM 2019, P51C–P08.
Pogue, G. P., Lindbo, J. A., Garger, S. J., and Fitzmaurice, W. P. (2002). MAKING ANALLY FROM ANENEMY: Plant Virology and the New Agriculture. Annu. Rev. Phytopathol. 40, 45–74. doi:10.1146/annurev.phyto.40.021102.150133
Prater, T. J., Bean, Q. A., Beshears, R. D., Rolin, T. D., Werkheiser, N. J., Ordonez, E. A., et al. (2016). Summary Report on Phase I Results from the 3D Printing in Zero G Technology Demonstration mission, Volume I. Tech. Rep..
Prater, T. J., Werkheiser, N. J., and Ledbetter, F. E. (2018). Summary Report on Phase I and Phase II Results from the 3D Printing in Zero-G Technology Demonstration Mission. Tech. Rep. Vol. II.
Ramirez-Perez, J. C., Hogan, J. A., and Strom, P. F. (2007). Inedible Biomass Biodegradation for Advanced Life Support Systems: I. Composting Reactor and Kinetics. habitation (elmsford) 11, 105–122. doi:10.3727/154296607783948713
Ramirez-Perez, J. C., Hogan, J. A., and Strom, P. F. (2008). Inedible Biomass Biodegradation for Advanced Life Support Systems: II. Compost Quality and Resource Recovery. habitation (elmsford) 11, 163–172. doi:10.3727/154296608785908615
Rapp, D. (2013). Mars ISRU Technology. Use of Extraterrestrial Resources for Human Space Missions to Moon or Mars. Springer Nature Switzerland AG, 31–90. doi:10.1007/978-3-642-32762-9_2
Rathi, D.-N., Amir, H. G., Abed, R. M. M., Kosugi, A., Arai, T., Sulaiman, O., et al. (2013). Polyhydroxyalkanoate Biosynthesis and Simplified Polymer Recovery by a Novel Moderately Halophilic Bacterium Isolated from Hypersaline Microbial Mats. J. Appl. Microbiol. 114, 384–395. doi:10.1111/jam.12083
Rehm, B. H. A. (2010). Bacterial Polymers: Biosynthesis, Modifications and Applications. Nat. Rev. Microbiol. 8, 578–592. doi:10.1038/nrmicro2354
Rittmann, B. E., and McCarty, P. L. (2001). Environmental Biotechnology: Principles and Applications. New York, NY: McGraw-Hill Education.
Rivkin, A. S., Milazzo, M., Venkatesan, A., Frank, E., Vidaurri, M. R., Metzger, P., et al. (2020). Asteroid Resource Utilization: Ethical Concerns and Progress. arXiv preprint arXiv:2011.03369.
Rothschild, L. J. (2016). Synthetic Biology Meets Bioprinting: Enabling Technologies for Humans on Mars (And Earth). Biochem. Soc. Trans. 44, 1158–1164. doi:10.1042/BST20160067
Ryba-White, M., Nedukha, O., Hilaire, E., Guikema, J. A., Kordyum, E., and Leach, J. E. (2001). Growth in Microgravity Increases Susceptibility of Soybean to a Fungal Pathogen. Plant Cel Physiol. 42, 657–664. doi:10.1093/pcp/pce082
Schievano, A., Tenca, A., Scaglia, B., Merlino, G., Rizzi, A., Daffonchio, D., et al. (2012). Two-Stage vs Single-Stage Thermophilic Anaerobic Digestion: Comparison of Energy Production and Biodegradation Efficiencies. Environ. Sci. Technol. 46, 8502–8510. doi:10.1021/es301376n
Serio, M., Kroo, E., Florczak, E., Wójtowicz, M., Wignarajah, K., Hogan, J., et al. (2008). Pyrolysis of Mixed Solid Food, Paper, and Packaging Wastes. Tech. Rep.. doi:10.4271/2008-01-2050
Smith, M., Craig, D., Herrmann, N., Mahoney, E., Krezel, J., McIntyre, N., et al. (2020). “The Artemis Program: An Overview of NASA’s Activities to Return Humans to the Moon,” in 2020 IEEE Aerospace Conference (IEEE), 1–10. doi:10.1109/aero47225.2020.9172323
Snyder, J. E., Walsh, D., Carr, P. A., and Rothschild, L. J. (2019). A Makerspace for Life Support Systems in Space. Trends Biotechnology 37, 1164–1174. doi:10.1016/j.tibtech.2019.05.003
Strazzera, G., Battista, F., Garcia, N. H., Frison, N., and Bolzonella, D. (2018). Volatile Fatty Acids Production from Food Wastes for Biorefinery Platforms: A Review. J. Environ. Manag. 226, 278–288. doi:10.1016/j.jenvman.2018.08.039
Tan, D., Xue, Y.-S., Aibaidula, G., and Chen, G.-Q. (2011). Unsterile and Continuous Production of Polyhydroxybutyrate by Halomonas TD01. Bioresour. Technol. 102, 8130–8136. doi:10.1016/j.biortech.2011.05.068
Tavares, F., Buckner, D., Burton, D., McKaig, J., Prem, P., Ravanis, E., et al. (2020). Ethical Exploration and the Role of Planetary Protection in Disrupting Colonial Practices. arXiv preprint arXiv:2010.08344.
Touloupakis, E., Poloniataki, E. G., Ghanotakis, D. F., and Carlozzi, P. (2021). Production of Biohydrogen And/or Poly-β-Hydroxybutyrate by Rhodopseudomonas Sp. Using Various Carbon Sources as Substrate. Appl. Biochem. Biotechnol. 193, 307–318. doi:10.1007/s12010-020-03428-1
Vago, J. L., Westall, F., Coates, A. J., Jaumann, R., Korablev, O., Ciarletti, V., et al. Pasteur Instrument Teams, Landing SPI (2017). Habitability on Early Mars and the Search for Biosignatures with the ExoMars Rover. Astrobiology 17, 471–510. doi:10.1089/ast.2016.1533
Vergari, F., Tibuzzi, A., and Basile, G. (2010). An Overview of the Functional Food Market: from Marketing Issues and Commercial Players to Future Demand from Life in Space. Bio-Farms for Nutraceuticals, 308–321. doi:10.1007/978-1-4419-7347-4_23(Springer)
Verseux, C., Heinicke, C., Ramalho, T. P., Determann, J., Duckhorn, M., Smagin, M., et al. (2021). A Low-Pressure, N2/CO2 Atmosphere Is Suitable for Cyanobacterium-Based Life-Support Systems on Mars. Front. Microbiol. 12, 67. doi:10.3389/fmicb.2021.611798
Weber, J., and Schnaitmann, J. (2016). “A New Human Thermal Model for the Dynamic Life Support System Simulation V-HAB,” in 46th International Conference on Environmental Systems.
Werner, S., Marillonnet, S., Hause, G., Klimyuk, V., and Gleba, Y. (2006). Immunoabsorbent Nanoparticles Based on a Tobamovirus Displaying Protein A. Proc. Natl. Acad. Sci. 103, 17678–17683. doi:10.1073/pnas.0608869103
Wertz, J. R., and Larson, W. J. (1996). Reducing Space mission Cost. Torrance, CA: Microcosm Press Torrance.
Wetmore, K. M., Price, M. N., Waters, R. J., Lamson, J. S., He, J., Hoover, C. A., et al. (2015). Rapid Quantification of Mutant Fitness in Diverse Bacteria by Sequencing Randomly Bar-Coded Transposons. mBio 6, 1–15. doi:10.1128/mBio.00306-15
Wheeler, R. M. (2017). Agriculture for Space: People and Places Paving the Way. Open Agric. 2, 14–32. doi:10.1515/opag-2017-0002
Yeh, H. H. J., Brown, C. B., and Jeng, F. J.. Tool for Sizing Analysis of the Advanced Life Support System (2005).
Keywords: space systems bioengineering, human exploration, in situ resource utilization, life support systems, biomanufacturing
Citation: Berliner AJ, Hilzinger JM, Abel AJ, McNulty MJ, Makrygiorgos G, Averesch NJH, Sen Gupta S, Benvenuti A, Caddell DF, Cestellos-Blanco S, Doloman A, Friedline S, Ho D, Gu W, Hill A, Kusuma P, Lipsky I, Mirkovic M, Luis Meraz J, Pane V, Sander KB, Shi F, Skerker JM, Styer A, Valgardson K, Wetmore K, Woo S-G, Xiong Y, Yates K, Zhang C, Zhen S, Bugbee B, Clark DS, Coleman-Derr D, Mesbah A, Nandi S, Waymouth RM, Yang P, Criddle CS, McDonald KA, Seefeldt LC, Menezes AA and Arkin AP (2021) Towards a Biomanufactory on Mars. Front. Astron. Space Sci. 8:711550. doi: 10.3389/fspas.2021.711550
Received: 18 May 2021; Accepted: 01 July 2021;
Published: 19 July 2021.
Edited by:
Cyprien Verseux, University of Bremen, GermanyReviewed by:
Briardo Llorente, Macquarie University, AustraliaRicardo Amils, Autonomous University of Madrid, Spain
Ivan Glaucio Paulino-Lima, Blue Marble Space Institute of Science (BMSIS), United States
Copyright © 2021 Berliner, Hilzinger, Abel, McNulty, Makrygiorgos, Averesch, Sen Gupta, Benvenuti, Caddell, Cestellos-Blanco, Doloman, Friedline, Ho, Gu, Hill, Kusuma, Lipsky, Mirkovic, Luis Meraz, Pane, Sander, Shi, Skerker, Styer, Valgardson, Wetmore, Woo, Xiong, Yates, Zhang, Zhen, Bugbee, Clark, Coleman-Derr, Mesbah, Nandi, Waymouth, Yang, Criddle, McDonald, Seefeldt, Menezes and Arkin. This is an open-access article distributed under the terms of the Creative Commons Attribution License (CC BY). The use, distribution or reproduction in other forums is permitted, provided the original author(s) and the copyright owner(s) are credited and that the original publication in this journal is cited, in accordance with accepted academic practice. No use, distribution or reproduction is permitted which does not comply with these terms.
*Correspondence: Adam P. Arkin, YXBhcmtpbkBsYmwuZ292; and Aaron J. Berliner, YWFyb24uYmVybGluZXJAYmVya2VsZXkuZWR1
†Present Address: Anna Doloman, Laboratory of Microbiology, Wageningen University, Wageningen, Netherlands