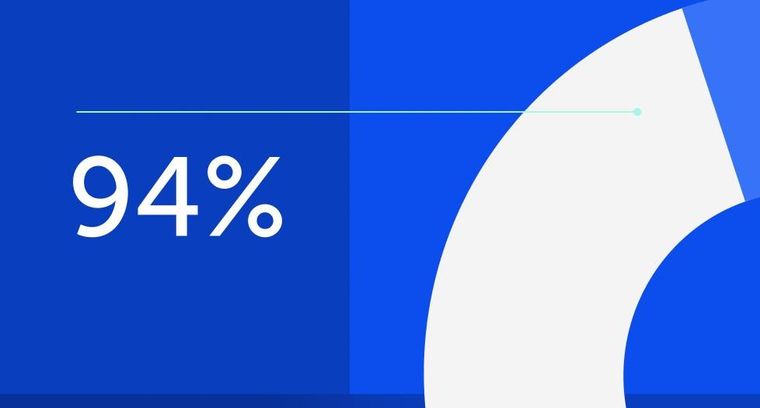
94% of researchers rate our articles as excellent or good
Learn more about the work of our research integrity team to safeguard the quality of each article we publish.
Find out more
REVIEW article
Front. Soft Matter, 13 July 2023
Sec. Biological Soft Matter
Volume 3 - 2023 | https://doi.org/10.3389/frsfm.2023.1219497
This article is part of the Research TopicCelebrating 1 Year of Frontiers in Soft MatterView all 5 articles
Cryopreservation allows the long-term storage of biological material, and has become integral for numerous applications including assisted reproductive technologies, stem cell therapies, blood banking, and species preservation. However, there are still hundreds of cell types that cannot be cryopreserved, and no organs at all. The biggest challenge facing cryopreservation is the ongoing reliance on predominantly just two cryoprotectants (dimethylsulfoxide and glycerol) as well as physical limitations to heating and cooling rates. The field of soft matter can accelerate cryopreservation research by providing insights into the underlying mechanisms and interactions of cells, cryoprotectants, and solvents including the role of temperature. With techniques as far ranging as differential scanning calorimetry, atomic force microscopy, and neutron and X-ray scattering, it is possible to probe multiple length and time scales in order to identify and characterise important interactions. This in turn can lead to the development of new cryoprotectants, and new methods of heating/cooling which could overcome some of the current challenges of cryopreservation.
Cryopreservation is the process of cooling biological samples down to very low temperatures, usually below −80°C, so that they can be stored for a long time (Mazur, 1970; Mazur, 2004). At such low temperatures, biological processes cease to operate, thus allowing the sample to enter a kind of ‘stasis’ where it theoretically remains unchanged until it is warmed (Mazur, 1970, 2004). Generally, there are two broad categories of cryopreservation (Figure 1); slow cooling, whereby the rate of cooling is low enough that extracellular ice forms and some water is transported out of the cells; and vitrification whereby the rate of cooling is so fast that water transport cannot occur and there is no ice formation but rather the entire system solidifies in a non-crystalline state (Mazur, 1970; Pegg, 2007).
FIGURE 1. Two broad categories of cryopreservation: (A) Vitrification or rapid cooling which can be achieved by plunging into liquid nitrogen and does not allow water transport or crystallisation, and (B) Slow cooling where water leaves the cells (causing them to shrink) and extracellular ice crystals form.
In nature there are numerous organisms that have adapted to extreme conditions, including extreme cold, so that they are able to enter a natural kind of “stasis” state. Almost universally, this adaptation includes the production of a cryoprotectant (CPA) that protects the organism’s cells from damage during freezing (Storey, 1990; Fuller et al., 2004). In nature, the primary cryoprotectants are small sugars, in particular the disaccharides trehalose and sucrose. Trehalose conveys both cold and desiccation resistance in many species, including bacteria and tardigrades, while sucrose is predominantly associated with plant freezing tolerance (Koster and Lynch, 1992; Koster et al., 1994). The primary modes of action are the ability to vitrify, combined with their ability to protect membranes at low to moderate dehydrations (Koster et al., 1994; Wolfe and Bryant, 1999; Koster et al., 2000). The mechanisms for membrane protection have been debated, with the resolution (Andersen et al., 2011) appearing to lie in the level of hydration. At the low to moderate hydrations most relevant to cryopreservation, sugars act osmotically to retain water and keep membranes separated during dehydration (Koster and Bryant, 2006; Lenne et al., 2006; Lenne et al., 2007; Lenne et al., 2009). At the very low hydrations mostly relevant for desiccation and freeze-drying, sugars replace water, thus stabilising proteins and lipids (Coutinho et al., 1988; Crowe et al., 1990; Crowe et al., 1992; Kandror et al., 2002; Crowe et al., 2004; Nguyen et al., 2022).
Antifreeze proteins and glycoproteins have been found to play a role in numerous cold and freeze-resistant species, particularly fish and insects (Balcerzak et al., 2014). These solutes act to protect organisms from freezing damage through thermal hysteresis and inhibiting ice recrystallisation (Balcerzak et al., 2014). The effect of this can be significant, for example, the freezing point of blood from artic fishes is routinely 1°C–2°C below zero (Scholander et al., 1957; DeVries et al., 1970; Devries, 1971). Similarly, a xylomannan glycolipid has been found in a diverse range of cold-tolerant taxa including amphibians, insects and plants and is thought to bind to lipid membranes to prevent damage during freezing (Walters et al., 2011). Other species accumulate different chemicals to protect them from freezing, such as wood frogs which produce urea, glycerol and glucose which allows them to tolerate temperatures as low as −16°C (Costanzo et al., 2013; Larson et al., 2014; Costanzo et al., 2015). In addition to animals, some plants have also evolved freeze tolerance, either through dehydration strategies or production of intracellular CPAs, including antifreeze proteins (distinct from those described above) (Hon et al., 1994; Pearce, 2004; Preston and Sandve, 2013).
In nature these molecules are produced inside cells and therefore can act intracellularly. However, the molecules are generally not membrane permeable, and therefore when used for artificial cryopreservation can only act extracellularly. Thus alternative membrane permeating cryoprotectants are needed for most laboratory cryopreservation. Note that cryopreservation for some types of cells and biomolecules can be achieved with non-penetrating CPAs (called extracellular CPAs), discussed briefly below.
Laboratory cryopreservation of microbial and fungal species has been carried out for close to a century (Haines and Barcroft, 1938; Fulton and Smith, 1953; Fennell, 1960), however, this review will focus on cryopreservation of plant and animal cells. The first report of artificial cryopreservation of an animal cell occurred early in the 20th century, although pinning down the very first reference is difficult (Shettles, 1939; Shaffner, 1942). These first studies focused on spermatozoa, which showed excellent recovery when frozen with glycerol—the first significant CPA (Polge et al., 1949). Since then there have been several breakthroughs in cryopreservation research, including the discovery of dimethylsulfoxide (DMSO) as a CPA, which is now the most widely-used for mammalian cells (Lovelock and Bishop, 1959). Research over the last century has made cryopreservation a standard laboratory practice, which varies somewhat between cell types, but broadly involves adding a CPA to the cells, then cooling the mixture at a specified rate followed by low-temperature storage, usually at either −80°C or −196°C (Pegg, 2007). When required, cells are thawed by warming (some variation for different cell types) and usually washed to remove the CPA which would otherwise have toxic effects on the cells (Sputtek and Sputtek, 2004; Pegg, 2007). Cryopreservation along these lines is important in numerous fields and for a wide variety of applications.
Cryopreservation has applications in numerous fields including assisted reproductive technology, species preservation, and biomedicine (Mazur, 1970, 2004; Pegg, 2007; Jang et al., 2017; Xie et al., 2022). Some of these applications are well-developed, while others are just emerging. The applications of cryopreservation are limited only by the types of cells that one wants to store.
One of the first applications of cryopreservation for animal cells was for assisted reproduction (Polge et al., 1949; Hungerford et al., 2023). This technology has come a long way and it is now possible to store sperm, oocytes and even embryos from a range of species (Polge et al., 1949; Bosch et al., 2020; Daly et al., 2020; Ferré et al., 2020; Stoecklein et al., 2021; Hungerford et al., 2023). However, the cryopreservation of reproductive tissue from some species, in particular amphibians, reptiles and marsupials, has remained challenging (Clulow and Clulow, 2016; Rodger, 2019; Campbell et al., 2020).
For application in species preservation, samples of reproductive tissues, embryos, or seeds are taken from live specimens and cryopreserved. This allows for long-term storage of specimens as a “back-up” to their living (and perhaps endangered) counterparts (Walters et al., 2004; Clulow and Clulow, 2016). One of the most outstanding examples of this is the Svalbard Global Seed Vault which contains samples of more than 5,000 species from around the world (Asdal and Guarino, 2018). This facility could allow the re-establishment of plant species in the event of mass extinction.
In addition to the above applications, the discovery and development of cryopreservation techniques has allowed unprecedented advances in science. Cryopreservation enables the storage and long-term access of biological specimens, including immortalised cell-lines (Geraghty et al., 2014). These cell lines are used globally for drug testing, disease investigations, and as therapeutic agents (e.g., stem cells) which in turn has led to unprecedented advances in all of these scientific fields.
Currently, cryopreservation can be used to store a relatively limited number of cell types. The majority of existing cryopreservation protocols rely on a small group of established CPAs, generally require small sample volumes, and there is very little scope for optimisation of cooling and warming protocols. All of these limitations contribute to the current inability to store hundreds of cell types, including some neural cells and cardiomyocytes (Jang et al., 2017), primary natural killer (NK) and T cells (Yao and Matosevic, 2021), as well as an inability to store any complex tissues or organs (Jacobsen et al., 1984; Bakhach, 2009; Lewis et al., 2016). These limitations are outlined below.
The majority of current cryopreservation protocols rely on variables that are not optimised for specific cell types, including CPA and cooling rate. Part of this is due to the complex, and resource-intensive nature of developing new cryopreservation protocols which relies on ready access to significant quantities of good-quality cells, and appropriate facilities.
One of the most difficult challenges to overcome in cryopreservation is the reliance on a limited number of CPAs (see Table 1), the most common being dimethylsulfoxide (DMSO) and glycerol (Best, 2015; Elliott et al., 2017; Raju et al., 2021). Recent evidence suggests that DMSO is more toxic than previously thought (Zenhäusern et al., 2000) and could in fact be causing epigenetic changes to cell lines which may have implications for subsequent applications (Iwatani et al., 2006; Tunçer et al., 2018). Similarly, glycerol leads to oxidative stress (Korrapati et al., 2012) and has been shown to be more toxic than other CPAs for certain cell types such as flounder embryos (Chen and Tian, 2005). Furthermore, a reliance on toxic CPAs such as DMSO is one of the main hurdles preventing storage of complex tissues and organs. As described above, for successful cryopreservation cells must be treated with a CPA prior to freezing. This is not an issue in single-cell suspensions where all cells are exposed to the CPA simultaneously. However, with complex tissues and organs there is a diffusion limitation where extended lengths of time are required for the CPA to penetrate to deeper cell layers (Martinez-Madrid et al., 2004; Pegg, 2007), and in the mean-time the outer layers are damaged by CPA toxicity. Thus, one of the biggest challenges for extending cryopreservation from single-to multi-cell systems is delivery of CPA to inner cell layers without toxic effects to the outer cell layers (Pegg, 2007; Bleisinger et al., 2020).
The second biggest challenge in the field of cryopreservation stems from technological limitations to cooling and heating rates. Theoretically, if a sample could be instantaneously cooled to −196°C and instantaneously warmed to room temperature, there would be little to no risk of freezing damage as there would be no time for ice to form (Gao and Critser, 2000; Mazur, 2004). While the cooling side of cryopreservation has been improved with the invention of controlled-rate freezers and the ability to plunge samples directly into liquid nitrogen or other cryogens (Wolfe and Bryant, 2001; Bojic et al., 2021), thawing generally still relies on convective warming by placing a sample into a 37°C water bath. This process is sufficiently slow that it puts the sample at risk of recrystallisation damage. Furthermore, it is impractical for larger sample vials, or tissues and organs which are multilayered (Manuchehrabadi et al., 2017; Bojic et al., 2021).
Until the issues of CPA toxicity and cooling/warming rates are addressed, extending cryopreservation beyond the cell types that can already be stored will be slow. However, if these, and other, challenges can be overcome, then it is possible that hundreds of new cell types can be stored, and perhaps even tissues and organs. Recent research into the major challenges of cryopreservation is discussed below.
Low temperatures are not in themselves damaging to most cell types. Rather, it is the physical process of freezing which is damaging (Mazur, 1963; Mazur, 1977; Wolfe and Bryant, 2001). This is due to a combination of mechanical damage due to the formation of intra- and extra-cellular ice crystals, and solute damage due to changes in the concentration of electrolytes (Figure 2) (Wolfe and Bryant, 2001).
FIGURE 2. There are multiple mechanisms of freezing damage. The formation of extracellular ice crystals can cause mechanical damage to cells (A) but it also results in concentration of solutes which causes water to flow out of the cells in an attempt to restore osmotic balance. This can lead to desiccation/dehydration of the cells (B). Intracellular ice crystals damage intracellular organelles leading to cell death (C).
At sub-zero temperatures water will form ice. However, this is a stochastic process which means that the probability of ice formation is proportional to the volume of water available; ice is more likely to form in a large volume than a small volume (Bryant, 1995). Thus, when considering cryopreservation of a cell, ice is more likely to form in the extracellular environment than the intracellular (Karow JR., 1969). However, intracellular ice formation is somewhat dependent on cooling rate; at slow cooling rates if ice forms externally, water will be transported out of the cell thus increasing the intracellular solute concentration resulting in dehydration but not freezing of the intracellular space. In contrast, if a cell is cooled too quickly, it will not lose sufficient water, resulting is supercooling and eventual formation of intracellular ice (Mazur, 1977).
The preferential formation of ice in the extracellular environment leads to an increase in solute concentration (salts, sugars, proteins, etc.) within the unfrozen fraction (Lovelock, 1953a; b). This increased concentration can be damaging to cells for a number of reasons, including creating an osmotic imbalance leading to osmotic shock, changing protein conformation, or influencing the bilayer structure of the cell membrane (Mazur, 1970; Steponkus, 1984; Gao and Critser, 2000; Wolfe and Bryant, 2001; Mazur, 2004; Elliott et al., 2017; Zhao and Fu, 2017). Furthermore, as the extracellular unfrozen fraction becomes more concentrated, the osmotic imbalance forces water out of the cell, leading to dehydration which can lead to membrane phase changes and cell damage (Crowe et al., 1990; Crowe et al., 1992; Bryant, 1995; Wolfe and Bryant, 1999; Wolfe and Bryant, 2001; Wolfe et al., 2002).
While extracellular ice formation is generally harmless to dilute cell suspensions, it can become lethal when cells are closely packed as in concentrated suspensions or multi-cellular assemblies (Pegg, 2020). Previous work has demonstrated that extracellular ice causes structural disruption of tissues including muscle (Elford and Walter, 1972; Taylor and Pegg, 1983; Jalal and Zidi, 2018), cartilage disks (Rendal-Vázquez et al., 2001; Pegg et al., 2006) and kidneys (Jacobsen et al., 1984). The location and degree of ice formation is related to the rate of cooling and warming, providing potential mechanisms for controlling ice formation (Fahy, 1990; Pegg, 2020).
The contrasting mechanisms of freeze damage (solute effects vs. ice damage) led Mazur to develop the “Two Factor Hypothesis” (Mazur, 1963; Mazur et al., 1972). This hypothesis relates cooling rates to cell survival, suggesting that there may be an ideal intermediate cooling rate that maximises cell survival. This hypothesis is illustrated in Figure 3. At low cooling rates cells die due to dehydration and toxicity from high solute concentrations. However, at high cooling rates, intracellular ice formation may occur which will also be lethal. Thus, an intermediate cooling rate should lead to maximum cell survival. The value of the ideal cooling rate varies between cell types due to factors such as size and permeability (Mazur, 1963; Mazur, 1977).
FIGURE 3. Cell viability at different cooling rates. Cells that are cooled too slowly die due to dehydration and toxicity effects while cells that are cooled too fast die due to intracellular ice formation. Thus there may be an intermediate “optimal” cooling rate. Extremely rapid cooling, i.e., vitrification may overcome these two mechanisms of freezing damage.
Freezing damage may also be combatted through vitrification. Vitrification is a transition to a solid glass-like state without ice formation and requires very rapid cooling, as shown in Figure 3 (Gao and Critser, 2000; Bojic et al., 2021). In general, vitrification depends on high concentrations of CPA to reduce the cooling/warming rates required to prevent ice formation, which then raises toxicity issues (Bojic et al., 2021).
It should be noted that ice formation and associated freezing damage can happen during warming (often called recrystallisation) as well as cooling. Therefore warming rate is an important consideration in combatting freezing damage (Gao and Critser, 2000; Waters et al., 2020). In particular, a sample that has undergone vitrification may be especially susceptible to ice recrystallization if the warming rate is too slow (Bojic et al., 2021; Zhan et al., 2022).
One of the biggest limitations to heating and cooling rates is heat transfer. Ideally, samples for preservation would be cooled and warmed instantaneously, thus achieving vitrification and re-warming without ice formation. In practice, cooling rates are limited by the physics of heat transfer.
Currently, vitrification relies on directly plunging samples into liquid nitrogen to achieve cooling rates of ∼340°C/min for small organs like ovaries (Courbiere et al., 2006) and 10,000°C/min for single cell suspensions (Nawroth et al., 2002). However, even with this method there is an uneven temperature change for the outside of the sample compared to the inside which for large samples (such as organs) can lead to fracturing and cell death (Steif et al., 2007).
Most cryopreservation protocols rely on slow warming in a hot-water bath which for 1 mL of cells in a 2 mL cryovial results in a warming rate of ∼45 ± 8 °C/min (Baboo et al., 2019) (significantly slower than the cooling rates used in vitrification). This method of warming has two significant limitations: 1) the slow warming rate can lead to ice recrystallisation, especially for samples that have been vitrified (Sharma et al., 2021) and 2) there is an uneven temperature change across samples (outside will be warmer than inside), which becomes more pronounced with larger samples (Manuchehrabadi et al., 2017). Uneven temperatures lead to cracking and fracturing during both cooling and warming (Steif et al., 2007; Sharma et al., 2021). Thus, a protocol which is suitable for a 1 mL solution of cells may result in very low cell recovery if applied to a 5 mL sample.
To overcome the above limitations of convective warming, substantial research is being carried out into new methods of sample warming which can achieve faster warming rates and can simultaneously warm an entire sample, regardless of size. For liquid samples such as blood, this can be achieved by maximising surface area (Sputtek and Mingers, 1999; Sputtek et al., 2007). Other methods include:
• Nanowarming which utilises iron oxide nanoparticles excited by a radio frequency field (Etheridge et al., 2014). This technique has been used to warm vitrified rat kidneys at a rate of 63.7°C/min (Sharma et al., 2021) and resulted in a higher viability of cryopreserved porcine arteries compared to those warmed using traditional convective methods (Manuchehrabadi et al., 2017). Importantly, the warming rates of tissues can be substantially different to the warming rate of the media on its own (Manuchehrabadi et al., 2017).
• Infrared radiation which can achieve various warming rates depending on power and length of exposure (Cao et al., 2022; Zhan et al., 2022). Adherent cells have been successfully thawed using this method with warming rates of 3,000°C/min (Bissoyi and Braslavsky, 2021).
• Laser warming which can achieve phenomenal heating rates up to 10 million °C/min. This technique has been utilised to warm mouse oocytes (Jin et al., 2014) and zebrafish embryos (Khosla et al., 2020).
• Using a water bath with additional microwave warming, which increased the rate from 70.2°C/min to 105°C/min while reducing the temperature gradient (Ruan et al., 2020).
As discussed above, CPAs are usually added to media prior to freezing. These CPAs inhibit ice formation, promote vitrification, and can act as buffers against solute toxicity induced by dehydration (Meryman, 1971; Hubálek, 2003; Fuller, 2004; Best, 2015; Elliott et al., 2017). Table 1 shows a summary of the most commonly used CPAs, along with some novel CPAs that have recently been being explored. The table includes notes on the cell types the CPAs are used for, and their toxicity. CPAs generally fall into two categories; intracellular or permeating and extracellular or non-permeating (Karow JR., 1969; Fuller, 2004; Elliott et al., 2017; Raju et al., 2021). Extracellular cryoprotectants include sucrose, polyvinylpyroldone and hydroxyethyl starch. These molecules are unable to enter cells and instead increase the osmolarity of the extracellular environment which causes cell dehydration and reduces the formation of intracellular ice crystals (Meryman, 1971; Raju et al., 2021).
DMSO and glycerol are two of the most commonly used intracellular CPAs, and both have been known for more than 50 years (Polge et al., 1949; Lovelock and Bishop, 1959). However, despite decades of research, there are still hundreds of cell types that cannot be efficiently preserved using these CPAs, including granulocytes (Sputtek and Sputtek, 2004) and T cells (Yao and Matosevic, 2021). In addition only very simple tissue architectures such as blood vessels have been preserved to date, and these have generally had poor recovery post-cryopreservation (Bakhach, 2009).
One significant limitation to cryopreservation of cells, and in particular tissues and organs is CPA toxicity (Table 1) (Fahy, 1986; Raju et al., 2021). Toxicity of CPAs leads to cell death during incubation and cryopreservation and requires rapid and time-consuming washing steps (Shu et al., 2014). For example, the IC50 (concentration which results in a 50% inhibition in growth) of DMSO for epithelial cells was found to be just 2.9% (Layman, 1987), and near 2% for human leukemic cells (Hajighasemi and Tajic, 2017) which is significantly less than the concentrations commonly used for cryopreservation (5%–10%). In fact DMSO has been linked to epigenetic changes in cultured cells which may impact on downstream applications such as embryo development (Iwatani et al., 2006; Tunçer et al., 2018). In addition, CPA toxicity can impact medical treatments, including adverse reactions. For example, fatal cardiac arrhythmia (Zenhäusern et al., 2000); neurotoxicity (Windrum and Morris, 2003; Ataseven et al., 2017); and respiratory depression (Benekli et al., 2000) have been recorded in patients administered cells stored with DMSO (Shu et al., 2014).
CPAs must, by necessity, interact with water and cellular components in order to facilitate protection during cryopreservation. However, new CPAs with reduced toxicity could overcome many of the existing challenges (Raju et al., 2021). For example, a CPA with little to no toxicity could be perfused through an organ or tissue and allowed time to fully penetrate all cell layers before freezing. This is not an option with current toxic CPAs as outer cell layers which are exposed for an extended period of time will die.
In addition to toxicity, permeability is another challenge for CPAs. As discussed above, naturally occurring CPAs are produced in the cells of freeze-resistant organisms, so do not need to permeate to perform their function. So while these natural compounds are sometimes used as non-permeating CPAs, an intracellular CPA must also usually be included (Dou et al., 2019; Sui et al., 2019).
Thus, one key requirement of effective CPAs is that they are able to permeate into cells. Small, moderately polar molecules can generally enter cells via passive diffusion such as DMSO and glycerol (Yang and Hinner, 2015). Larger, or more polar or ionic species cannot passively diffuse, which is why sugars such as trehalose are non-permeating CPAs (Yang and Hinner, 2015). The likelihood of a molecule being permeable can be roughly estimated using the “rule of 5” which states that poor permeability is likely if: there are more than 5 hydrogen bond donors; there are more than 10 hydrogen bond acceptors; the molecular weight is greater than 500 g/mol; and the lipophilicity (CLogP) is >5 (Lipinski et al., 1997; Yang and Hinner, 2015).
Some molecules can enter cells via transporter-mediated entry including ions, water, some sugars, and most amino acids (Yang and Hinner, 2015). When developing CPAs they must be able to get into the target cell through either passive diffusion or transporter-mediated entry.
One avenue of research is to improve the permeability of existing CPAs. Trehalose has been a particular focus for this strategy. Numerous methods have been applied to trehalose to improve permeation; including chemical modification (e.g., addition of an alkyl chain) (Abazari et al., 2015), nanoparticle delivery (Rao et al., 2015; Stefanic et al., 2017), pseudopeptides (Chen et al., 2020), microinjection (Eroglu et al., 2002) and genetic modification (Guo et al., 2000).
An alternative method is to alter the cell membrane to allow trehalose permeability. This can be achieved by a simple increase in temperature in the case of platelets (Wolkers et al., 2001), through addition of permeability-increasing biopolymers (Lynch et al., 2010), electroporation (Dovgan et al., 2017), or through the application of bacterially-derived pore-forming molecules (Eroglu et al., 2000). In the latter case, an increased survival of mammalian cells was observed with low trehalose concentrations following cryopreservation, but higher concentrations resulted in massive cell death (Eroglu et al., 2000).
Evidently, there are many potential strategies to increase CPA uptake, however, each of these come with disadvantages such as the potential toxicity or downstream effects of altering the cells (either genetically or by introducing pores), difficulties in scaling (e.g., for microinjection), and concentration and toxicity concerns for nanoparticles. Thus a more fruitful avenue of research may be to find new, permeating CPAs.
As demonstrated from the discussion above, there is a vital need for new, permeating, less-toxic CPAs, not only to overcome the issues associated with current CPAs, but also to store new cell types, and perhaps eventually more complex assemblies such as tissues and organs. The ideal CPA will inhibit ice formation and promote vitrification, permeate into cells, and prevent protein/membrane damage, without being itself too toxic. A detailed discussion of the need for rational design of new CPAs is given in our recent review (Raju et al., 2021).
There are several classes of chemicals that have shown promise as CPAs, although more research is required to explore their ability to store new cell types (Raju et al., 2021). These potential new CPAs include anti-freeze proteins (Leclère et al., 2011; Balcerzak et al., 2014; Kuiper et al., 2015), glycolipids (Bendas et al., 1996; Walters et al., 2011), carbohydrate derivatives (Eroglu et al., 2000; Chaytor et al., 2011; Imamura et al., 2014; Abazari et al., 2015; Raju et al., 2019b), ionic liquids (Bryant et al., 2021), deep eutectic solvents (Castro et al., 2018; Jesus et al., 2021; Bryant et al., 2022) and others (Sasaki et al., 2005; Toyosawa et al., 2009; Miyamoto et al., 2012; Verdanova et al., 2014).
Testing the cryoprotective efficacy of new CPAs can be costly and time-consuming. Therefore, rational methods of design and testing must be employed, as described in our recent review (Raju et al., 2021). Soft matter techniques in particular can be used to screen potential CPAs for promising properties before carrying out full-scale testing. These properties include permeability, thermal behaviour, and interactions with cells or model cellular components, as discussed below.
Soft matter encompasses the study of materials that lie somewhere between a liquid and a solid, and by definition includes most biological systems including cells and tissues (Tang, 2022). The multi-disciplinary nature of this field makes it ideally suited to the exploration of cryopreservation because it allows the investigation of the biological, chemical, and physical processes that are at work. Numerous techniques are capable of interrogating soft matter systems, all with different capabilities, accessible length scales and limitations, as outlined below.
There are a broad range of techniques which are standard in the cryopreservation literature as outlined in Table 2. These include optical and electron microscopy techniques, differential scanning calorimetry and so on. This review will focus on techniques which are less widely used, and/or have had significant recent developments which make them exciting techniques for cryobiological investigations.
TABLE 2. Techniques that are standard for cryopreservation research and will not be discussed further in this review.
Permeability is a critical requirement for intracellular CPAs, as discussed above, and can be measured in several ways as reviewed by McGrath (McGrath, 1997). These methods have advantages and disadvantages that depend on cell type, availability, and the conditions being examined. However, one of the simplest methods of measuring CPA permeability is the “shrink-swell” light microscopy experiment. In this technique, changes to cell size on exposure to solutions containing CPAs are observed using microscopy. Addition of a CPA solution causes an osmotic imbalance that forces water out of cells and causes them to shrink. If the CPA is permeable, it will subsequently flow into the cells, reversing the osmotic imbalance and causing the cell to swell again. However, if the CPA cannot permeate, then the cell will remain in its shrunken state (Mazur and Schneider, 1986; Kleinhans, 1998; Bryant et al., 2022). This data is quantified by measuring the cell area over time (e.g., using software such as ImageJ to measure cell area in different frames), converting that to a volume, and then normalising to the starting volume of the cell (Bryant et al., 2021; Bryant et al., 2022), as shown in Figure 4. Most studies rely on time-consuming manual area determinations and data processing, however recent advances in computational image analysis and machine learning provides avenues for automated image analysis which will significantly speed up the process and allow upscaling of the technique (Shu et al., 2016; Tu et al., 2022). A limitation of this method is that it assumes the cells are spherical for conversion of area to volume, which is often not the case. This assumption may lead to erroneous conclusions about shrink-swell behaviour.
FIGURE 4. Schematic showing the change in cell volume during shrink-swell experiments due to osmotic changes. The solid and long dash lines are examples of permeating CPAs with different permeabilities and osmolalities which results in different shrink/swell rates. Illustrations show a cell at each state of a shrink-swell experiment: before addition (top left), directly after addition of CPA (bottom, water leaves cell, causing it to shrink), and after permeation of the CPA (top right, CPA and water have gone back into the cell, causing it to return to its starting volume). The dotted line represents a non-penetrating CPA.
The shrink-swell experiment described above relies on the cells being fixed in place for the duration of the experiment (e.g., by a poly-L-lysine coating). This can be difficult and unreliable, but also may introduce new behaviours that would not occur in unfixed cells. Thus, this experiment can be improved by using microfluidic technologies (Figure 5) (Zhao and Fu, 2017; Raju et al., 2019a). Perfusion chambers (Chen et al., 2007) and single-cell traps (Tan and Takeuchi, 2007; Wlodkowic et al., 2009) have been developed to capture cells and allow permeability measurements without fixation (Chen et al., 2007; Kitson et al., 2012; Edashige, 2017; Fang et al., 2017; Tseng et al., 2022; Tu et al., 2022).
FIGURE 5. Example of a microfluidic device used to trap cells for subsequent shrink-swell analysis. (A) degassing and channel filling, (B) cell loading, (C) cell stabilization, (D) cell shrinking after DMSO addition, (E) cell swelling as DMSO penetrates, (F) cell traps, (G) time-lapse images showing volume changes of a THP- 1 cell, (H) volume kinetics of four THP-1 cells with average shown in black. Reprinted from (Raju et al., 2019a), with the permission of AIP Publishing.
Other methods for determining shrink-swell behaviour include:
• Coulter electronic conductance which measures electrical conductivity between two chambers (Higgins and Karlsson, 2008a; Higgins and Karlsson, 2008b, 2010). The presence of a cell passing through an aperture causes an increase in resistance and results in a measured voltage spike which can be related to the cell size (Hirsch and Gallian, 1968; Model, 2018). The benefit of this method is that it is largely independent of cell shape, unlike the light microscopy method described above which generally assumes spherical cells. However, as the Coulter method relies on conductivity, the results can be complicated by the addition of solvents (as when testing permeability of potential CPAs) as the conductivity of the solvent itself will change.
• Flow cytometry utilises light scattering from particles in a flowing solution and has been applied to monitor cell volume changes (Noiles et al., 1997; Yeung et al., 2002; Tzur et al., 2011; Model, 2018). The technique allows rapid analysis of whole populations, unlike light microscopy which only analyses a handful of cells at a time. However, volume changes to cells can also cause changes to the refractive index, which changes scattering intensity and therefore can interfere with the apparent cell volume.
While cell volume can be measured in a variety of other ways, such as atomic force microscopy (discussed below) and confocal microscopy, these techniques are not appropriate for permeability measurements as the imaging time is too slow. In the case of highly permeable CPAs such as DMSO, the entire shrink-swell process may be over in just 30 s, and therefore only techniques which allow rapid data acquisition are appropriate.
Data from shrink-swell experiments can be modelled to extrapolate the permeability parameter of potential CPAs. Models of this behaviour have progressed from one parameter (solute permeability) (Mazur et al., 1974; Mazur and Miller, 1976), to two (water and solute permeability) (Jacobs, 1933), to three (water and solute permeability, interaction parameter) (Kedem and Katchalsky, 1958). The three-parameter or Kedem-Katchalsky (KK) formalism is most commonly used today as it accounts for the interaction between solute and solvent (Kleinhans, 1998). The resulting permeability parameters can then be used to rationally tailor cryopreservation protocols. First it can be used to exclude molecules which are non-permeating from further consideration, and second it will yield permeability parameters for those that are. If permeation is rapid (as is often the case for DMSO) then the cells will require little to no time to uptake the CPA prior to freezing. However, if the permeability is low, then permeation into the cell will be slow, and therefore the cell may require a period of “incubation” with the CPA prior to freezing in order to allow sufficient uptake to have a protective effect (Bryant et al., 2022).
Langmuir isotherm experiments utilise a simple Langmuir trough (Figure 6) to compress monolayers of amphiphilic molecules on the surface of a subphase. The apparatus usually measures surface pressure using a Wilhelmy plate and is equipped with barriers which can be moved closer together or further apart to control the surface pressure and area. These experiments are frequently used to examine lipid behaviour under different conditions such as temperature and lateral pressure (Oliveira et al., 2022). The lipid of interest is dissolved in a volatile solvent such as ethanol or chloroform and then injected onto the surface of the subphase, usually water (within the “barriers” as shown in Figure 6). The volatile solvent evaporates and the lipid assembles at the air-solvent interface so that the hydrophobic tails are orientated into the air (Oliveira et al., 2022). The barriers can then be moved to measure changes in surface pressure with compression to ensure that a stable monolayer has formed at the surface. At this point changes can be made to the system (e.g., temperature, or the injection of an additive into the subphase) and the response of the monolayer is measured as a change in surface pressure. These values can then be used to calculate the area per molecule of lipid as a function of different conditions.
FIGURE 6. (A) Langmuir trough setup illustrating a lipid monolayer (pink) between two moveable barriers with chemicals of interest dissolved in the subphase (green spheres). (B) Illustrative pressure isotherm showing changing area per molecule with changing surface pressure.
Langmuir isotherms have revealed that sugars with higher complexity have a more significant impact on lipid monolayers than simpler ones (e.g., trehalose compared to glucose) by increasing the apparent headgroup area (Clark et al., 2015). Conversely, the polymer hydroxyethyl starch has no interaction with lipids, consistent with its role as an external non-permeating CPA (Bryant et al., 1994).
Another study found that the effect of permeating CPAs on model lipid monolayers was dependent on the lipid species, with a more significant impact observed for unsaturated compared to saturated lipids (Raju et al., 2020). The same study observed a significant increase in headgroup area for all lipids with addition of glycerol and ethylene glycol, but only for saturated DPPC with addition of DMSO. This finding has implications for other studies that have focused on only one type of lipid as the results may not apply to cell membranes which are composed of many different types of lipids. In contrast to the above, a study utilising octadecanol as the lipid found that DMSO and glycerol did not significantly affect the pressure isotherms, and suggested that the cryoprotective mechanisms came from interactions either with membrane proteins or via interaction with the aqueous environment surrounding the lipids (Williams and Harris, 1977).
Langmuir isotherm experiments offer a simple benchtop approach to investigating the interaction of CPAs with model monolayers. It is limited by relying on a lipid monolayer at the solvent-air interface, rather than a bilayer which may more accurately reflect the cell membrane. The technique is further complicated as the surface pressure can influence the degree of interaction observed between CPAs and the lipid layer, which may not be relevant to real membranes (Dabkowska et al., 2014). Despite this, these experiments can still shed light on interactions with lipid headgroups, such as whether molecules insert into the monolayer or cause an effective dehydration (and thus decrease in apparent headgroup area).
In addition to the uses described above, a Langmuir trough combined with Langmuir-Blodgett deposition can be used to prepare bilayer samples for a range of experiments including neutron and X-ray techniques to extract structural information about how CPAs may affect bilayer structure.
Reflectivity involves measuring the reflections off a surface. This reflectivity is measured as a function of the scattering vector q which can be defined as:
where
FIGURE 7. (A) Schematic of a lipid with head and tail regions highlighted. (B) Schematic showing the principles of a reflectivity measurement from a lipid monolayer at an air-water interface. (C) Schematic showing the principles of a reflectivity measurement from a lipid bilayer at a solid-liquid interface.
Neutron and X-ray reflectivity rely on differences in the scattering length density (SLD) or “scattering power” of components within the sample. The greater the difference in SLD between components (e.g., a lipid head group and the solvent), the greater the resolution will be from these techniques. For neutrons, scattering arises from the nuclear scattering lengths while for x-rays scattering arises from electron density. Thus, in x-ray reflectivity experiments H2O and D2O are indistinguishable, but they are very different in neutron reflectivity experiments (Lakey et al., 2022). Thus, the two techniques can be used in tandem to “see” different parts of a sample. This provides independent measurements of the same parameters (e.g., layer thickness) and allows simultaneous fitting (Vaknin et al., 1991). In this case, known variables are fixed (e.g., SLDs) while the unknown variables are allowed to vary (e.g., layer thickness) but the resulting fit must match both the neutron and X-ray reflectivity data, providing greater precision and reliability in the results. An example is shown in Figure 8.
FIGURE 8. X-ray (XRR) and neutron (NR) reflectivity profiles (left) and associated SLD profiles (right) for POPC lipid monolayers with an aqueous subphase (adapted from Bryant et al., 2023, licensed CC-BY-4.0). The SLD profiles highlight differences in contrast between X-rays and neutrons, which can be used to localise different regions. Deuteration of molecules and/or the aqueous layer provides an additional contrast profile for neutrons.
In neutron reflectivity a technique known as “contrast matching” can also be used to achieve multiple independent measurements of the same variable to improve analysis and fitting results. This technique relies on the significant difference in SLDs between hydrogen and deuterium, providing a stark contrast between H2O (SLD = −5.6 × 10−7 Å−2) and D2O (SLD = 6.3 × 10−6 Å−2). By adjusting the H2O/D2O ratio, the solvent SLD can be adjusted to the desired value, for example, to ‘match out’ the scattering from a component (e.g., the lipid hydrocarbon tail). Thus, the same measurement can be performed using solvents with different SLDs to get several independent measurements from the same system but highlighting different features. Then these multiple patterns can be simultaneously fit (fixing known variables such as the solvent SLD) to obtain a reliable value for the unknown parameters (Bayerl et al., 1990).
Reflectivity techniques have been used to examine lipid monolayers and bilayers under a variety of conditions. Such experiments have yielded insight into the hydration and positioning of the glycerol backbone of lipids in the fluid compared to condensed state (Bayerl et al., 1990) and the positioning of biomolecules such as proteins within a model lipid bilayer (Fragneto et al., 2000; Hossain et al., 2017; Hsieh et al., 2018). This gives insight into behaviours such as preferential partitioning into the headgroup (or tail) region, and broad changes to the lipid layer (e.g., change to thickness).
A handful of papers have used reflectivity to examine the interactions of CPAs with lipids. In one study a lipid monolayer of dipalmitoylphosphatidylcholine (DPPC) exposed to DMSO (0.1 and 0.05 mol fraction) showed no change in tail or headgroup thickness according to neutron reflectivity (Dabkowska et al., 2014). A different study used several techniques, including neutron reflectivity, to explore the interaction of sugars (glucose, glycerol and trehalose) with a DPPC monolayer. They found it difficult to fit their reflectivity data, but found it suggestive of glucose dehydrating the headgroup region (Clark et al., 2015).
Reflectivity offers an underutilised opportunity to better understand the interactions of CPAs with cell membranes. Based on the studies performed to date, it is likely that a bilayer system will yield better resolution and more relevant results than monolayers. However, future studies could utilise reflectivity to explore how potential CPAs interact with a wide range of lipids, including lipid mixtures to better represent real membranes, thus shedding light on interactions of CPAs and laying the groundwork for rational design of next-generation CPAs.
Small and wide angle X-ray scattering (SAXS and WAXS) and small angle neutron scattering (SANS) are carried out in transmission with the scattered beam measured on a 2-dimensional detector (Figure 9). The sample’s structural information is represented in reciprocal space by the elastic scattering pattern (Liu et al., 2022). After radial averaging, the scattering intensity can be given as a function of the scattering vector, q:
FIGURE 9. Schematic showing the principles of small angle scattering whereby an incoming beam passes through the sample and is scattered onto a two-dimensional detector.
Where
SAXS is a widely used technique for studying structure in self-assembling systems, in particular the structure of lipid membranes (Nagle and Tristram-Nagle, 2000; Tyler et al., 2015). However, to date there have been relatively few studies using SAXS to understand cryobiological processes. One study using both SAXS and SANS showed that DMSO acts to dehydrate lipid headgroups (Kiselev et al., 1999; Shashkov et al., 1999). Studies involving one of the authors used SAXS/WAXS to show that the presence of vitrified sugars lowers the lipid gel to fluid transition temperature by tens of degrees (Koster et al., 1994), quantified the effects of different sugar concentrations on the transition temperature (Lenne et al., 2007), and produced a quantitative model to explain the effect (Lenne et al., 2009). A later study used SAXS to understand the interactions between lipid membranes and intrinsically disordered proteins implicated in cold tolerance in plants (Thalhammer et al., 2014).
Several studies have used SANS to explore the interaction of DMSO with lipids, usually in the form of multi- or uni-lamellar vesicles. One study used contrast variation SANS to show that DMSO can induce membrane phase transitions. DMSO also caused a decrease in interbilayer spacing of multilamellar vesicles (Gorshkova and Gordeliy, 2007). Two studies by the same group demonstrated that DMSO interacts strongly with water, causing dehydration of the intermembrane space and a subsequent decrease in repeat spacing (Kiselev et al., 1999; Shashkov et al., 1999).
Studies involving one of the authors have used contrast variation SANS to estimate the location of sugars in lipid/water mixtures (Lenne et al., 2006), and showed that glucose is excluded from the inverse hexagonal phase, which occurs during dehydration in some lipid systems. The utility of SAXS and SANS as tools to study the interactions of cryomolecules with membranes was reviewed in (Garvey et al., 2013).
Membrane diffraction measures X-ray or neutron diffraction from self-assembled stacks of hundreds of bilayers (Wiener and White, 1991; Hauss et al., 2005). In this technique, by varying the deuteration of water, lipid and solute, the specific location of solutes within membranes can be determined to high precision, providing insights into the detailed interactions between the solutes and the membranes. In the context of cryobiology, this has been used to show that the natural cryoprotectants trehalose and sucrose do not preferentially interact with membrane headgroups at moderate hydrations (Kent et al., 2014; Kent et al., 2015), and has been used to measure how deeply cold regulated proteins penetrate into the lipid bilayer (Bremer et al., 2017).
One of the main limitations of neutron (and high precision X-ray) scattering is the requirement for a specialist facility, usually with limited access. For neutrons in particular, fluxes tend to be low, requiring relatively long measurement times (∼ tens of minutes to hours). Furthermore, contrast variation measurements require deuterated components which can be expensive, or completely unavailable, thus limiting the systems that can be explored. Despite these limitations, these techniques are critical tools and show a lot of promise for overcoming challenges in cryopreservation—in particular to understand the interactions of CPAs with model lipid bilayers.
Infrared (IR) and Near-IR spectroscopy measures the absorption, emission, or reflection of IR radiation as it interacts with matter in the region of ∼4,000 to ∼600 cm−1 (Capuano and van Ruth, 2016; Pasquini, 2018). It can be used to interrogate the chemistry of solid (Chabal, 1988), liquid (Arrondo et al., 1993), or gas samples (Sharpe et al., 2004; MacAleese and Maître, 2007), which also encompasses biological materials in solution or deposited onto a solid substrate (Parker, 2012; Beć et al., 2020). Data is realised by recording the variation in energy as a function of the wavelength/frequency of the IR radiation as it interacts with the sample of interest (see Figure 10). Plotting the resulting signal as a function of wavenumber (or wavelength) provides a spectrum, revealing “bands” which yield chemical information as chemical bonds and functional groups have different characteristic absorption frequencies, which can then be used to identify the chemical composition of a material (Liu, 2021). The technique can therefore identify which functional groups or chemical bonds are present in a sample of interest.
FIGURE 10. Synchrotron FTIR images of human keratinocytes using macro-ATR-FTIR. (A) Image showing a cell triplet. (B) Intensity map of the integrated area of the Lipid band (3,010–2,837 cm−1), (C) Intensity map of the integrated area of the Amide I band (1746–1,563 cm−1). (B,C) Red indicates high intensity and blue indicates low intensity. Scale bars are 20 µm.
Fourier-transform IR (FTIR) spectroscopy takes this one step further by illuminating a sample simultaneously with many frequencies of light and performing a Fourier transform to determine the absorption at each wavenumber (Movasaghi et al., 2008). This technique can be coupled with optical microscopy to perform hyperspectral-imaging (micro-FTIR). This generates spatially resolved FTIR maps of a sample, which can provide sub-nanometre resolution of a sample and its corresponding chemical environment (Wood et al., 1996; Wood et al., 2004; Roy et al., 2017; Vongsvivut et al., 2019).
For biological applications, IR spectroscopy can interrogate biochemical signals within bio-molecules (Roggo et al., 2007; Wiercigroch et al., 2017), tissues (Rolfe, 2000; Wood et al., 2004; Movasaghi et al., 2008; Pellicer and del Carmen Bravo, 2011), single celled organisms (Helm et al., 1991; Alvarez-Ordóñez et al., 2011; Cheeseman et al., 2021; Hartnell et al., 2021; Shaw et al., 2022) and mammalian cells (Wood et al., 1996; Arnold et al., 2003; Heraud et al., 2010; Roy et al., 2017), amongst other biological constructs (Mourant et al., 2005; Stuart, 2006; Henriques et al., 2009; Tidy et al., 2017). The spectral regions of interest are often the methylene/methyl (CH2/CH3) region for lipids (3,000–2,800 cm−1), amide I and II bands for proteins (1705–1,600 cm−1 and 1,567–1,448 cm−1 respectively), and fingerprint region for polysaccharides and nucleic acids (1,200–1,000 cm−1). Micro-FTIR has been recently utilised to interrogate the spatial distribution of bio-chemical properties within whole tissues (Wood et al., 2004; Heraud et al., 2010; Hackett et al., 2016), mammalian cells (Wood et al., 1996; Hackett et al., 2013; Roy et al., 2017; Vongsvivut et al., 2019), and single-celled organisms (Pham et al., 2020; Cheeseman et al., 2021; Hartnell et al., 2021; Shaw et al., 2022).
For cryopreservation-based research, IR, near-IR, FTIR, and Micro-FTIR techniques can be used in several ways to evaluate the cryoprotectant—bio interactions: 1) to elucidate the bio-chemical interactions of cryoprotectant molecules with biological materials (whole cells or isolated bio-constructions) (Chopra et al., 2016); 2) to interrogate the bio-chemical alteration of cells in response to the presence of a CPA (Gläfke et al., 2012; Sieme et al., 2015; Wolkers and Oldenhof, 2016); and 3) to assess biochemical changes which occur during freeze-thaw cycles (Wolkers and Oldenhof, 2015; Giugliarelli et al., 2016; Wang et al., 2018).
While IR has the potential to interrogate many biochemical signatures at once, it is limited in absolute resolution. Data is usually an average of the interrogated area which cannot be easily controlled. For example, in the case of cells, individual components (e.g., proteins, membranes, etc.) cannot be isolated—measurements will provide an average from all contributions within a relatively large volume. Furthermore, analysis of IR data can be difficult, and may rely on minute shifts in the spectra that are difficult to quantify.
Atomic force microscopy (AFM) is an in situ scanning probe technique which can image and interrogate a system physically via a cantilever beam with a probe attached to one end (Hansma et al., 1994; Magonov et al., 1997; Cappella and Dietler, 1999; Senden, 2001; Garcia and Perez, 2002; Butt et al., 2005; Parot et al., 2007; García, 2010). The nature of the probe is normally either a sharp-tip or a colloidal entity. Data obtained from the technique can readily provide nanometre spatial resolution (Uchihashi et al., 2005; Higgins et al., 2006; Fukuma et al., 2007; Loh and Jarvis, 2010; Ricci et al., 2014; Elbourne et al., 2015a; Hofmann et al., 2016; Kariuki et al., 2022), as well as probe nanoscale forces at a variety of interfaces (Cappella and Dietler, 1999; Butt et al., 2005; Thordarson et al., 2006; Helenius et al., 2008; Elbourne et al., 2023), including those in liquid environments.
In recent decades, the technique has progressed beyond a simple surface profiling tool, to a multi-facetted surface analysis instrument (see Figure 11), capable of probing soft matter (Wanless and Ducker, 1996; Elbourne et al., 2015a) and biological systems (Wanless and Ducker, 1996; Ducker and Wanless, 1999; Webber et al., 2001; Elbourne et al., 2013; Segura et al., 2013; Page et al., 2014; Elbourne et al., 2015b; Elbourne et al., 2016; McDonald et al., 2016), nanomechanical properties (Radmacher, 1997; Touhami et al., 2003; Parot et al., 2007; Müller and Dufrêne, 2011; Collett et al., 2019; Pham et al., 2020) and in situ interactions, all with nanoscale resolution (Higgins et al., 2006; Takeshi, 2010; Trewby et al., 2016; Ricci et al., 2017; Fukuma and Garcia, 2018; Trewby et al., 2019; Fukuma, 2020; Yurtsever et al., 2021). For biological systems, the AFM can probe tissues (Goksu et al., 2009; Müller and Dufrêne, 2011; Yamashita et al., 2012; Shan and Wang, 2015; Dufrêne et al., 2017), whole cells (bacteria, fungi, and mammalian) (Parot et al., 2007; Müller and Dufrêne, 2011; Dufrêne et al., 2017), bioparticles (viruses, proteins, etc.) (Collett et al., 2019; Collett et al., 2021; Boyton et al., 2022), or isolated cell constructs (membrane mimics, lipid bilayers, excised sections, etc.) (Radmacher et al., 1992; Radmacher, 1997; Goksu et al., 2009; Garvey et al., 2023).
FIGURE 11. Schematic illustration of AFM techniques which can be applied to cryopreservation research. (A,B) show the principle of AFM measurements for cell wall constructs and whole cell/tissues respectively. (C) Shows how the AFM tip can be modified to examine specific interactions including physical, chemical and biological. (D) Shows how a surface can be sequentially scanned to produce spatial maps of nanomechanical data. (E) Shows a height image of a mammalian cell taken using AFM, inset: mechanical stiffness map of the imaged cell.
For cryopreservation, AFM can provide qualitative and quantitative information on morphology and nanomechanical properties of whole cells, tissues, and biological extracts (natural or biomimetic). These measurements can provide ex situ or in situ information on the effect of a CPA on a given system, depending on the experimental approach. For instance, one study monitored the morphological and nanomechanical response of murine fibroblasts to varying concentrations of the traditional CPAs DMSO and glycerol before and after a freeze/thaw cycle (Golan et al., 2018). More recently similar protocols were used to investigate the response of four human cell lines (THP-1, HaCat, PC3, and UG87-MG) to a novel CPA based on deep eutectic solvents (Bryant et al., 2022). Studies in this area are still in their infancy, however, AFM could be used to elucidate molecular mechanisms involved in cryoprotectant-membrane behaviour and structure-function properties of cryoprotectants, as well as the general effect of CPAs on whole cells and tissues.
While AFM can achieve atomic-level resolution its applications can be limited by the time required to take each measurement. As discussed above, this excludes AFM from use in examining permeability kinetics of CPAs. AFM is also limited to determining the external structure (size and shape) and does not provide information on internal structures. AFM also usually relies on samples being fixed to a surface which can be challenging for some materials. The surface can also introduce artifacts or changed interactions that would not occur in a free-floating system.
This review highlights the extensive research currently being carried out utilising soft matter techniques to overcome challenges in cryopreservation, and identify some areas where these techniques have not yet been applied but offer a lot of promise.
There is an obvious need for new CPAs (Raju et al., 2021). More than 50 years of research has demonstrated that the existing standard CPAs (glycerol and DMSO) are unsuitable for many cell types, and are currently limiting the applications of cryopreservation. These new CPAs should be rationally designed with consideration for factors such as permeability, which can to some degree be predicted based on the “rule of 5” described in Section 2.2.2 (Lipinski et al., 1997; Yang and Hinner, 2015). However, it is not enough to test potential new CPAs using protocols that have been optimised over decades for DMSO or glycerol. Instead, new CPAs need to be fully characterised. This includes:
• Determining the ideal heating/cooling rates as well as final storage temperature to avoid freezing and ice recrystallisation (e.g., via DSC).
• Determining permeability in order to optimise the addition and removal steps of the CPAs into the cells of interest (e.g., via shrink-swell measurements).
• Determining biomolecular interactions in order to understand the mechanism of cryopreservation and of potential toxicity effects (e.g., via SANS, reflectometry, FTIR and AFM)
Utilisation of multiple techniques, such as those described in this review, will allow full exploration and characterisation of chemical, thermal, and physical properties relevant to cryopreservation protocols. This understanding will encompass length scales from just a few nanometres up to several microns. Ultimately these explorations will lead to the development of structure-property relationships for CPAs and improved cryopreservation protocols.
Characterisation and optimisation will allow the proper utilisation of new CPAs and may allow the replacement of the current gold standards of DMSO and glycerol for some applications. This in turn will have unprecedented benefits in numerous fields. First, application of new CPAs may allow the storage of new cell types, which can be used for biomedical therapies as well as scientific research. Second, novel CPAs may facilitate the storage of more complex materials such as tissues, plant samples and maybe even organs which would have unprecedented benefits for the medical sector.
This review has focused on some of the less widely used techniques which can help address the challenges in cryopreservation. There are of course many other useful techniques, including the standard techniques detailed in Table 2, as well as well-known chemical techniques such as electron paramagnetic resonance and nuclear magnetic resonance, but these are beyond the scope of this review. The techniques reviewed here have the potential to provide a detailed understanding of the underlying mechanisms of cryopreservation which can then be used for the rational design of cryopreservation protocols. Armed with these techniques it is likely that we will see rapid advancement of the cryopreservation field in the near future.
Writing—original draft preparation, SB; writing—review and editing, SB, AE, TG, and GB; All authors contributed to the article and approved the submitted version.
SB acknowledge support from an Australian Institute of Nuclear Science and Engineering (AINSE) and Early Career Researcher Grant (ECRG). SB and GB acknowledge the support of the ARC Research Council grants DP190101010 and LP160101496. AE is supported by an Australian Research Council (ARC) Discovery Early Career Research Award (DECRA) (DE220100511).
The authors declare that the research was conducted in the absence of any commercial or financial relationships that could be construed as a potential conflict of interest.
All claims expressed in this article are solely those of the authors and do not necessarily represent those of their affiliated organizations, or those of the publisher, the editors and the reviewers. Any product that may be evaluated in this article, or claim that may be made by its manufacturer, is not guaranteed or endorsed by the publisher.
Abazari, A., Meimetis, L. G., Budin, G., Bale, S. S., Weissleder, R., and Toner, M. (2015). Engineered trehalose permeable to mammalian cells. PLOS ONE 10, e0130323. doi:10.1371/journal.pone.0130323
Alvarez-Ordóñez, A., Mouwen, D., López, M., and Prieto, M. (2011). Fourier transform infrared spectroscopy as a tool to characterize molecular composition and stress response in foodborne pathogenic bacteria. J. Microbiol. methods 84, 369–378. doi:10.1016/j.mimet.2011.01.009
Andersen, H. D., Wang, C., Arleth, L., Peters, G. H., and Westh, P. (2011). Reconciliation of opposing views on membrane-sugar interactions. Proc. Natl. Acad. Sci. U. S. A. 108, 1874–1878. doi:10.1073/pnas.1012516108
Arnold, S. A., Crowley, J., Woods, N., Harvey, L. M., and Mcneil, B. (2003). In-situ near infrared spectroscopy to monitor key analytes in mammalian cell cultivation. Biotechnol. Bioeng. 84, 13–19. doi:10.1002/bit.10738
Arrondo, J. L. R., Muga, A., Castresana, J., and Goñi, F. M. (1993). Quantitative studies of the structure of proteins in solution by Fourier-transform infrared spectroscopy. Prog. biophysics Mol. Biol. 59, 23–56. doi:10.1016/0079-6107(93)90006-6
Asdal, Å., and Guarino, L. (2018). The svalbard global seed Vault: 10 Years-1 million samples. Biopreserv Biobank 16, 391–392. doi:10.1089/bio.2018.0025
Ashwood-Smith, M. J., Warby, C., Connor, K. W., and Becker, G. (1972). Low-temperature preservation of mammalian cells in tissue culture with polyvinylpyrrolidone (PVP), dextrans, and hydroxyethyl starch (HES). Cryobiology 9, 441–449. doi:10.1016/0011-2240(72)90161-7
Ataseven, E., Tüfekçi, Ö., Yilmaz, Ş., Güleryüz, H., and Ören, H. (2017). Neurotoxicity associated with dimethyl sulfoxide used in allogeneic stem cell transplantation. J. Pediatr. Hematology/Oncology 39, e297–e299. doi:10.1097/mph.0000000000000784
Awan, M., Buriak, I., Fleck, R., Fuller, B., Goltsev, A., Kerby, J., et al. (2020). Dimethyl sulfoxide: A central player since the dawn of cryobiology, is efficacy balanced by toxicity? Regen. Med. 15, 1463–1491. doi:10.2217/rme-2019-0145
Baboo, J., Kilbride, P., Delahaye, M., Milne, S., Fonseca, F., Blanco, M., et al. (2019). The impact of varying cooling and thawing rates on the quality of cryopreserved human peripheral blood T cells. Sci. Rep. 9, 3417. doi:10.1038/s41598-019-39957-x
Bakhach, J. (2009). The cryopreservation of composite tissues: Principles and recent advancement on cryopreservation of different type of tissues. Organogenesis 5, 119–126. doi:10.4161/org.5.3.9583
Balcerzak, A. K., Capicciotti, C. J., Briard, J. G., and Ben, R. N. (2014). Designing ice recrystallization inhibitors: From antifreeze (glyco)proteins to small molecules. RSC Adv. 4, 42682–42696. doi:10.1039/c4ra06893a
Bayerl, T. M., Thomas, R. K., Penfold, J., Rennie, A., and Sackmann, E. (1990). Specular reflection of neutrons at phospholipid monolayers. Changes of monolayer structure and headgroup hydration at the transition from the expanded to the condensed phase state. Biophysical J. 57, 1095–1098. doi:10.1016/s0006-3495(90)82628-x
Beć, K. B., Grabska, J., and Huck, C. W. (2020). Near-infrared spectroscopy in bio-applications. Molecules 25, 2948. doi:10.3390/molecules25122948
Bendas, G., Wilhelm, F., Richter, W., and Nuhn, P. (1996). Synthetic glycolipids as membrane-bound cryoprotectants in the freezedrying process of liposomes. Eur. J. Pharm. Sci. 4, 211–222. doi:10.1016/0928-0987(95)00054-2
Benekli, M., Anderson, B., Wentling, D., Bernstein, S., Czuczman, M., and Mccarthy, P. (2000). Severe respiratory depression after dimethylsulphoxide-containing autologous stem cell infusion in a patient with AL amyloidosis. Bone Marrow Transplant. 25, 1299–1301. doi:10.1038/sj.bmt.1702452
Best, B. P. (2015). Cryoprotectant toxicity: Facts, issues, and questions. Rejuvenation Res. 18, 422–436. doi:10.1089/rej.2014.1656
Bissoyi, A., and Braslavsky, I. (2021). Adherent cell thawing by infrared radiation. Cryobiology 103, 129–140. doi:10.1016/j.cryobiol.2021.08.002
Bleisinger, N., Dittrich, R., Strahl, O., Brauweiler, R., Hoffmann, I., Beckmann, M. W., et al. (2020). Me2SO perfusion time for whole-organ cryopreservation can be shortened: Results of micro-computed tomography monitoring during Me2SO perfusion of rat hearts. PLoS One 15, e0238519. doi:10.1371/journal.pone.0238519
Bojic, S., Murray, A., Bentley, B. L., Spindler, R., Pawlik, P., Cordeiro, J. L., et al. (2021). Winter is coming: The future of cryopreservation. BMC Biol. 19, 56. doi:10.1186/s12915-021-00976-8
Bosch, E., De Vos, M., and Humaidan, P. (2020). The future of cryopreservation in assisted reproductive technologies. Front. Endocrinol. (Lausanne) 11, 67. doi:10.3389/fendo.2020.00067
Boutron, P. (1992). Cryoprotection of red blood cells by a 2,3-butanediol containing mainly the levo and dextro isomers. Cryobiology 29, 347–358. doi:10.1016/0011-2240(92)90036-2
Boyton, I., Goodchild, S. C., Diaz, D., Elbourne, A., Collins-Praino, L. E., and Care, A. (2022). Characterizing the dynamic disassembly/reassembly mechanisms of encapsulin protein nanocages. ACS Omega 7, 823–836. doi:10.1021/acsomega.1c05472
Bozkurt, Y., Yavaş, I., Bucak, M. N., and Yeni, D. (2019). Effect of different cryoprotectants (glycerol, methanol and dimethyl sulfoxide) on post-thaw quality, viability, fertilization ability and DNA damage of cryopreserved nile Tilapia (Oreochromis niloticus) spermatozoa. Cryo Lett. 40, 11–17.
Bremer, A., Kent, B., Hauss, T., Thalhammer, A., Yepuri, N. R., Darwish, T. A., et al. (2017). Intrinsically disordered stress protein COR15A resides at the membrane surface during dehydration. Biophysical J. 113, 572–579. doi:10.1016/j.bpj.2017.06.027
Bryant, G. (1995). DSC measurement of cell suspensions during successive freezing runs: Implications for the mechanisms of intracellular ice formation. Cryobiology 32, 114–128. doi:10.1006/cryo.1995.1011
Bryant, G., Perez, E., and Sputtek, A. (1994). The influence of hydroxyethyl starch on phospholipid monolayer isotherms. CRYO-LETTERS 15, 299–308.
Bryant, S. J., Awad, M. N., Elbourne, A., Christofferson, A. J., Martin, A. V., Meftahi, N., et al. (2022). Deep eutectic solvents as cryoprotective agents for mammalian cells. J. Mater. Chem. B 10, 4546–4560. doi:10.1039/d2tb00573e
Bryant, S. J., Brown, S. J., Martin, A. V., Arunkumar, R., Raju, R., Elbourne, A., et al. (2021). Cryopreservation of mammalian cells using protic ionic liquid solutions. J. Colloid Interface Sci. 603, 491–500. doi:10.1016/j.jcis.2021.06.096
Bryant, S. J., Shaw, Z. L., Huang, L. Z. Y., Elbourne, A., Abraham, A. N., Vongsvivut, J., et al. (2023). Insights into chemical interactions and related toxicities of deep eutectic solvents with mammalian cells observed using synchrotron macro-ATR-FTIR microspectroscopy. Biophysica 3, 318–336. doi:10.3390/biophysica3020021
Butt, H. J., Cappella, B., and Kappl, M. (2005). Force measurements with the atomic force microscope: Technique, interpretation and applications. Surf. Sci. Rep. 59, 1–152. doi:10.1016/j.surfrep.2005.08.003
Campbell, L., Cafe, S. L., Upton, R., Doody, J. S., Nixon, B., Clulow, J., et al. (2020). A model protocol for the cryopreservation and recovery of motile lizard sperm using the phosphodiesterase inhibitor caffeine. Conserv. Physiol. 8, coaa044. doi:10.1093/conphys/coaa044
Cao, Y., Chang, T., Fang, C., Zhang, Y., Liu, H., and Zhao, G. (2022). Inhibition effect of Ti3C2Tx MXene on ice crystals combined with laser-mediated heating facilitates high-performance cryopreservation. ACS Nano 16, 8837–8850. doi:10.1021/acsnano.1c10221
Cappella, B., and Dietler, G. (1999). Force-distance curves by atomic force microscopy. Surf. Sci. Rep. 34, 1. doi:10.1016/s0167-5729(99)00003-5
Capuano, E., and Van Ruth, S. M. (2016). “Infrared spectroscopy: Applications,” in Encyclopedia of food and health. Editors B. Caballero, P. M. Finglas, and F. Toldrá (Oxford: Academic Press), 424–431.
Castro, V. I. B., Craveiro, R., Silva, J. M., Reis, R. L., Paiva, A., and Duarte, C., (2018). Natural deep eutectic systems as alternative nontoxic cryoprotective agents. Cryobiology 83, 15–26. doi:10.1016/j.cryobiol.2018.06.010
Chabal, Y. J. (1988). Surface infrared spectroscopy. Surf. Sci. Rep. 8, 211–357. doi:10.1016/0167-5729(88)90011-8
Chaytor, J. L., Tokarew, J. M., Wu, L. K., Leclère, M., Tam, R. Y., Capicciotti, C. J., et al. (2011). Inhibiting ice recrystallization and optimization of cell viability after cryopreservation. Glycobiology 22, 123–133. doi:10.1093/glycob/cwr115
Cheeseman, S., Shaw, Z. L., Vongsvivut, J., Crawford, R. J., Dupont, M. F., Boyce, K. J., et al. (2021). Analysis of pathogenic bacterial and yeast biofilms using the combination of synchrotron ATR-FTIR microspectroscopy and chemometric approaches. Molecules 26, 3890. doi:10.3390/molecules26133890
Chen, H.-H., Purtteman, J. J. P., Heimfeld, S., Folch, A., and Gao, D. (2007). Development of a microfluidic device for determination of cell osmotic behavior and membrane transport properties. Cryobiology 55, 200–209. doi:10.1016/j.cryobiol.2007.08.001
Chen, S. L., and Tian, Y. S. (2005). Cryopreservation of flounder (Paralichthys olivaceus) embryos by vitrification. Theriogenology 63, 1207–1219. doi:10.1016/j.theriogenology.2004.06.007
Chen, S., Wu, L., Ren, J., Bemmer, V., Zajicek, R., and Chen, R. (2020). Comb-like pseudopeptides enable very rapid and efficient intracellular trehalose delivery for enhanced cryopreservation of erythrocytes. ACS Appl. Mater. Interfaces 12, 28941–28951. doi:10.1021/acsami.0c03260
Chopra, P., Nayak, D., Nanda, A., Ashe, S., Rauta, P. R., and Nayak, B. (2016). Fabrication of poly (vinyl alcohol)-Carrageenan scaffolds for cryopreservation: Effect of composition on cell viability. Carbohydr. Polym. 147, 509–516. doi:10.1016/j.carbpol.2016.04.027
Clark, G. A., Henderson, J. M., Heffern, C., Akgün, B., Majewski, J., and Lee, K. Y. C. (2015). Synergistic interactions of sugars/polyols and monovalent salts with phospholipids depend upon sugar/polyol complexity and anion identity. Langmuir 31, 12688–12698. doi:10.1021/acs.langmuir.5b02815
Clulow, J., and Clulow, S. (2016). Cryopreservation and other assisted reproductive technologies for the conservation of threatened amphibians and reptiles: Bringing the ARTs up to speed. Reproduction, Fertil. Dev. 28, 1116–1132. doi:10.1071/rd15466
Collett, S., Torresi, J., Earnest-Silveira, L., Christiansen, D., Elbourne, A., and Ramsland, P. A. (2019). Probing and pressing surfaces of hepatitis C virus-like particles. J. Colloid Interface Sci. 545, 259–268. doi:10.1016/j.jcis.2019.03.022
Collett, S., Torresi, J., Earnest-Silveira, L., Khanh Truong, V., Christiansen, D., Tran, B. M., et al. (2021). Investigating virus-host cell interactions: Comparative binding forces between hepatitis C virus-like particles and host cell receptors in 2D and 3D cell culture models. J. Colloid Interface Sci. 592, 371–384. doi:10.1016/j.jcis.2021.02.067
Cordeiro, R. M., Stirling, S., Fahy, G. M., and De Magalhães, J. P. (2015). Insights on cryoprotectant toxicity from gene expression profiling of endothelial cells exposed to ethylene glycol. Cryobiology 71, 405–412. doi:10.1016/j.cryobiol.2015.10.142
Costanzo, J. P., Do Amaral, M. C. F., Rosendale, A. J., and Lee, R. E. (2013). Hibernation physiology, freezing adaptation and extreme freeze tolerance in a northern population of the wood frog. J. Exp. Biol. 216, 3461–3473. doi:10.1242/jeb.089342
Costanzo, J. P., Reynolds, A. M., Do Amaral, M. C., Rosendale, A. J., and Lee, R. E. (2015). Cryoprotectants and extreme freeze tolerance in a subarctic population of the wood frog. PLoS One 10, e0117234. doi:10.1371/journal.pone.0117234
Courbiere, B., Odagescu, V., Baudot, A., Massardier, J., Mazoyer, C., Salle, B., et al. (2006). Cryopreservation of the ovary by vitrification as an alternative to slow-cooling protocols. Fertil. Steril. 86, 1243–1251. doi:10.1016/j.fertnstert.2006.05.019
Coutinho, C., Bernardes, E., Félix, D., and Panek, A. D. (1988). Trehalose as cryoprotectant for preservation of yeast strains. J. Biotechnol. 7, 23–32. doi:10.1016/0168-1656(88)90032-6
Crowe, J. H., Hoekstra, F. A., and Crowe, L. M. (1992). Anhydrobiosis. Annu. Rev. Physiology 54, 579–599. doi:10.1146/annurev.ph.54.030192.003051
Crowe, J., Crowe, L. M., Tablin, F., Wolkers, W., Oliver, A., and Tsvetkova, N. (2004). “Stabilization of cells during freeze-drying: The trehalose myth,” in Life in the frozen state. Editors B. J. Fuller, N. Lane, and E. E. Benson (Boca Raton: CRC Press), 582–601.
Crowe, J. H., Carpenter, J. F., Crowe, L. M., and Anchordoguy, T. J. (1990). Are freezing and dehydration similar stress vectors? A comparison of modes of interaction of stabilizing solutes with biomolecules. Cryobiology 27, 219–231. doi:10.1016/0011-2240(90)90023-w
Dabkowska, A. P., Collins, L. E., Barlow, D. J., Barker, R., Mclain, S. E., Lawrence, M. J., et al. (2014). Modulation of dipalmitoylphosphatidylcholine monolayers by dimethyl sulfoxide. Langmuir 30, 8803–8811. doi:10.1021/la501275h
Daillant, J., Bellet-Amalric, E., Braslau, A., Charitat, T., Fragneto, G., Graner, F., et al. (2005). Structure and fluctuations of a single floating lipid bilayer. Proc. Natl. Acad. Sci. U. S. A. 102, 11639–11644. doi:10.1073/pnas.0504588102
Daly, J., Smith, H., Mcgrice, H. A., Kind, K. L., and Van Wettere, W. (2020). Towards improving the outcomes of assisted reproductive technologies of cattle and sheep, with particular focus on recipient management. Anim. (Basel) 10, 293. doi:10.3390/ani10020293
Damien, M., Luciano, A. A., and Peluso, J. J. (1990). Propanediol alters intracellular pH and developmental potential of mouse zygotes independently of volume change. Hum. Reprod. 5, 212–216. doi:10.1093/oxfordjournals.humrep.a137072
Damjanovic, V., and Thomas, D. (1974). The use of polyvinylpyrrolidone as a cryoprotectant in the freezing of human lymphocytes. Cryobiology 11, 312–316. doi:10.1016/0011-2240(74)90007-8
Desai, K., Spikings, E., and Zhang, T. (2015). Use of methanol as cryoprotectant and its effect on sox genes and proteins in chilled zebrafish embryos. Cryobiology 71, 1–11. doi:10.1016/j.cryobiol.2015.06.009
Devries, A. L. (1971). Glycoproteins as biological antifreeze agents in antarctic fishes. Science 172, 1152–1155. doi:10.1126/science.172.3988.1152
Devries, A. L., Komatsu, S. K., and Feeney, R. E. (1970). Chemical and physical properties of freezing point-depressing glycoproteins from antarctic fishes. J. Biol. Chem. 245, 2901–2908. doi:10.1016/s0021-9258(18)63073-x
Dou, M., Lu, C., Sun, Z., and Rao, W. (2019). Natural cryoprotectants combinations of l-proline and trehalose for red blood cells cryopreservation. Cryobiology 91, 23–29. doi:10.1016/j.cryobiol.2019.11.002
Dovgan, B., Barlič, A., Knežević, M., and Miklavčič, D. (2017). Cryopreservation of human adipose-derived stem cells in combination with trehalose and reversible electroporation. J. Membr. Biol. 250, 1–9. doi:10.1007/s00232-016-9916-z
Ducker, W. A., and Wanless, E. J. (1999). Adsorption of hexadecyltrimethylammonium bromide to mica: Nanometer-scale study of binding-site competition effects. Langmuir 15, 160–168. doi:10.1021/la9710942
Dufrêne, Y. F., Ando, T., Garcia, R., Alsteens, D., Martinez-Martin, D., Engel, A., et al. (2017). Imaging modes of atomic force microscopy for application in molecular and cell biology. Nat. Nanotechnol. 12, 295–307. doi:10.1038/nnano.2017.45
Edashige, K. (2017). Permeability of the plasma membrane to water and cryoprotectants in mammalian oocytes and embryos: Its relevance to vitrification. Reproductive Med. Biol. 16, 36–39. doi:10.1002/rmb2.12007
El-Shewy, H. M., William, F. K., Darrabie, M., Collins, B. H., and Opara, E. C. (2004). Polyvinyl pyrrolidone: A novel cryoprotectant in islet cell cryopreservation. Cell Transplant. 13, 237–243. doi:10.3727/000000004783983927
Elbourne, A., Dupont, M., Kariuki, R., Meftahi, N., Daeneke, T., Greaves, T. L., et al. (2023). Mapping the three-dimensional nanostructure of the ionic liquid–solid interface using atomic force microscopy and molecular dynamics simulations. Adv. Mater. Interfaces 10, 2202110. doi:10.1002/admi.202202110
Elbourne, A., Mcdonald, S., Voïchovsky, K., Endres, F., Warr, G. G., and Atkin, R. (2015a). Nanostructure of the ionic liquid–graphite stern layer. ACS Nano 9, 7608–7620. doi:10.1021/acsnano.5b02921
Elbourne, A., Mclean, B., Voïtchovsky, K., Warr, G. G., and Atkin, R. (2016). Molecular resolution in situ imaging of spontaneous graphene exfoliation. J. Phys. Chem. Lett. 7, 3118–3122. doi:10.1021/acs.jpclett.6b01323
Elbourne, A., Sweeney, J., Webber, G. B., Wanless, E. J., Warr, G. G., Rutland, M. W., et al. (2013). Adsorbed and near-surface structure of ionic liquids determines nanoscale friction. Chem. Commun. 49, 6797–6799. doi:10.1039/c3cc42844c
Elbourne, A., Voitchovsky, K., Warr, G. G., and Atkin, R. (2015b). Ion structure controls ionic liquid near-surface and interfacial nanostructure. Chem. Sci. 6, 527–536. doi:10.1039/c4sc02727b
Elford, B. C., and Walter, C. A. (1972). Effects of electrolyte composition and pH on the structure and function of smooth muscle cooled to −79 °C in unfrozen media. Cryobiology 9, 82–100. doi:10.1016/0011-2240(72)90015-6
Elliott, G. D., Wang, S., and Fuller, B. J. (2017). Cryoprotectants: A review of the actions and applications of cryoprotective solutes that modulate cell recovery from ultra-low temperatures. Cryobiology 76, 74–91. doi:10.1016/j.cryobiol.2017.04.004
Eroglu, A., Russo, M. J., Bieganski, R., Fowler, A., Cheley, S., Bayley, H., et al. (2000). Intracellular trehalose improves the survival of cryopreserved mammalian cells. Nat. Biotechnol. 18, 163–167. doi:10.1038/72608
Eroglu, A., Toner, M., and Toth, T. L. (2002). Beneficial effect of microinjected trehalose on the cryosurvival of human oocytes. Fertil. Steril. 77, 152–158. doi:10.1016/s0015-0282(01)02959-4
Etheridge, M. L., Xu, Y., Rott, L., Choi, J., Glasmacher, B., and Bischof, J. C. (2014). RF heating of magnetic nanoparticles improves the thawing of cryopreserved biomaterials. TECHNOLOGY 02, 229–242. doi:10.1142/s2339547814500204
Fahy, G. M. (1986). The relevance of cryoprotectant "toxicity" to cryobiology. Cryobiology 23, 1–13. doi:10.1016/0011-2240(86)90013-1
Fahy, G. M. (1990). “Vitrification as an approach to organ cryopreservation: Past, present, and future,” in Cryopreservation and low temperature biology in blood transfusion: Proceedings of the fourteenth international symposium on blood transfusion, groningen 1989, organised by the red cross blood bank groningen-drenthe. Editors C. T. Smit Sibinga, P. C. Das, and H. T. Meryman (Boston, MA: Springer US), 255–268.
Fang, C., Ji, F., Shu, Z., and Gao, D. (2017). Determination of the temperature-dependent cell membrane permeabilities using microfluidics with integrated flow and temperature control. Lab a Chip 17, 951–960. doi:10.1039/c6lc01523a
Fennell, D. I. (1960). Conservation of fungous cultures. Botanical Rev. 26, 79–141. doi:10.1007/bf02860481
Ferré, L. B., Kjelland, M. E., Strøbech, L. B., Hyttel, P., Mermillod, P., and Ross, P. J. (2020). Review: Recent advances in bovine in vitro embryo production: Reproductive biotechnology history and methods. Animal 14, 991–1004. doi:10.1017/s1751731119002775
Fragneto, G., Graner, F., Charitat, T., Dubos, P., and Bellet-Amalric, E. (2000). Interaction of the third helix of antennapedia homeodomain with a deposited phospholipid bilayer: A neutron reflectivity structural study. Langmuir 16, 4581–4588. doi:10.1021/la991119s
Fukuma, T., and Garcia, R. (2018). Atomic- and molecular-resolution mapping of solid–liquid interfaces by 3D atomic force microscopy. ACS Nano 12, 11785–11797. doi:10.1021/acsnano.8b07216
Fukuma, T., Higgins, M. J., and Jarvis, S. P. (2007). Direct imaging of lipid-ion network formation under physiological conditions by frequency modulation atomic force microscopy. Phys. Rev. Lett. 98, 106101. doi:10.1103/physrevlett.98.106101
Fukuma, T. (2020). Subnanometer-scale imaging of nanobio-interfaces by frequency modulation atomic force microscopy. Biochem. Soc. Trans. 48, 1675–1682. doi:10.1042/bst20200155
Fuller, B. J. (2004). Cryoprotectants: The essential antifreezes to protect life in the frozen state. Cryoletters 25, 375–388.
Fulton, J. D., and Smith, A. U. (1953). Preservation of entamoeba histolytica at −79°C. In the presence of glycerol. Ann. Trop. Med. Parasitol. 47, 240–246. doi:10.1080/00034983.1953.11685564
Gao, D., and Critser, J. K. (2000). Mechanisms of cryoinjury in living cells. ILAR J. 41, 187–196. doi:10.1093/ilar.41.4.187
García, R. (2010). “Introduction,” in Amplitude modulation atomic force microscopy (Germany: Wiley-VCH Verlag GmbH & Co. KGaA), 1–7.
Garcia, R., and Perez, R. (2002). Dynamic atomic force microscopy methods. Surf. Sci. Rep. 47, 197–301. doi:10.1016/s0167-5729(02)00077-8
Garvey, C. J., Bryant, S. J., Elbourne, A., Hunt, T., Kent, B., Kreuzer, M., et al. (2023). Phase separation in a ternary DPPC/DOPC/POPC system with reducing hydration. J. Colloid Interface Sci. 638, 719–732. doi:10.1016/j.jcis.2023.01.145
Garvey, C. J., Lenne, T., Koster, K. L., Kent, B., and Bryant, G. (2013). Phospholipid membrane protection by sugar molecules during dehydration-insights into molecular mechanisms using scattering techniques. Int. J. Mol. Sci. 14, 8148–8163. doi:10.3390/ijms14048148
Geraghty, R. J., Capes-Davis, A., Davis, J. M., Downward, J., Freshney, R. I., Knezevic, I., et al. (2014). Guidelines for the use of cell lines in biomedical research. Br. J. Cancer 111, 1021–1046. doi:10.1038/bjc.2014.166
Giugliarelli, A., Sassi, P., Urbanelli, L., Paolantoni, M., Caponi, S., Ricci, M., et al. (2016). Cryopreservation of cells: FT-IR monitoring of lipid membrane at freeze-thaw cycles. Biophys. Chem. 208, 34–39. doi:10.1016/j.bpc.2015.08.001
Gläfke, C., Akhoondi, M., Oldenhof, H., Sieme, H., and Wolkers, W. F. (2012). Cryopreservation of platelets using trehalose: The role of membrane phase behavior during freezing. Biotechnol. Prog. 28, 1347–1354. doi:10.1002/btpr.1600
Goksu, E. I., Vanegas, J. M., Blanchette, C. D., Lin, W.-C., and Longo, M. L. (2009). AFM for structure and dynamics of biomembranes. Biochimica Biophysica Acta (BBA) - Biomembr. 1788, 254–266. doi:10.1016/j.bbamem.2008.08.021
Golan, M., Přibyl, J., Pesl, M., Jelinkova, S., Acimovic, I., Jaroš, J., et al. (2018). Cryopreserved cells regeneration monitored by atomic force microscopy and correlated with state of cytoskeleton and nuclear membrane. IEEE Trans. NanoBioscience 17, 485–497. doi:10.1109/tnb.2018.2873425
Gorshkova, J. E., and Gordeliy, V. I. (2007). Investigation of the interaction of dimethyl sulfoxide with lipid membranes by small-angle neutron scattering. Crystallogr. Rep. 52, 535–539. doi:10.1134/s1063774507030364
Gosden, R. G., Yin, H., Bodine, R. J., and Morris, G. J. (2010). Character, distribution and biological implications of ice crystallization in cryopreserved rabbit ovarian tissue revealed by cryo-scanning electron microscopy. Hum. Reprod. 25, 470–478. doi:10.1093/humrep/dep395
Guo, N., Puhlev, I., Brown, D. R., Mansbridge, J., and Levine, F. (2000). Trehalose expression confers desiccation tolerance on human cells. Nat. Biotechnol. 18, 168–171. doi:10.1038/72616
Hackett, M. J., Borondics, F., Brown, D., Hirschmugl, C., Smith, S. E., Paterson, P. G., et al. (2013). Subcellular biochemical investigation of purkinje neurons using synchrotron radiation fourier transform infrared spectroscopic imaging with a focal plane array detector. ACS Chem. Neurosci. 4, 1071–1080. doi:10.1021/cn4000346
Hackett, M. J., Sylvain, N. J., Hou, H., Caine, S., Alaverdashvili, M., Pushie, M. J., et al. (2016). Concurrent glycogen and lactate imaging with FTIR spectroscopy to spatially localize metabolic parameters of the glial response following brain ischemia. Anal. Chem. 88, 10949–10956. doi:10.1021/acs.analchem.6b02588
Haines, R. B., and Barcroft, J. (1938). The effect of freezing on bacteria. Proc. R. Soc. Lond. B 124, 451–463.
Hajighasemi, F., and Tajic, S. (2017). Assessment of cytotoxicity of dimethyl sulfoxide in human hematopoietic tumor cell lines. Iran. J. Blood Cancer 9, 48–53.
Hansma, P. K., Cleveland, J. P., Radmacher, M., Walters, D. A., Hillner, P. E., Bezanilla, M., et al. (1994). Tapping mode atomic force microscopy in liquids. Appl. Phys. Lett. 64, 1738–1740. doi:10.1063/1.111795
Hartnell, D., Hollings, A., Ranieri, A. M., Lamichhane, H. B., Becker, T., Sylvain, N. J., et al. (2021). Mapping sub-cellular protein aggregates and lipid inclusions using synchrotron ATR-FTIR microspectroscopy. Analyst 146, 3516–3525. doi:10.1039/d1an00136a
Hauss, T., Dante, S., Haines, T. H., and Dencher, N. A. (2005). Localization of coenzyme Q(10) in the center of a deuterated lipid membrane by neutron diffraction. Biochimica Biophysica Acta-Bioenergetics 1710, 57–62. doi:10.1016/j.bbabio.2005.08.007
Helenius, J., Heisenberg, C.-P., Gaub, H. E., and Muller, D. J. (2008). Single-cell force spectroscopy. J. Cell Sci. 121, 1785–1791. doi:10.1242/jcs.030999
Helm, D., Labischinski, H., Schallehn, G., and Naumann, D. (1991). Classification and identification of bacteria by Fourier-transform infrared spectroscopy. Microbiology 137, 69–79. doi:10.1099/00221287-137-1-69
Henriques, J. G., Buziol, S., Stocker, E., Voogd, A., and Menezes, J. C. (2009). Monitoring mammalian cell cultivations for monoclonal antibody production using near-infrared spectroscopy. Opt. Sens. Syst. Biotechnol. 116, 73–97. doi:10.1007/10_2009_11
Heraud, P., Caine, S., Campanale, N., Karnezis, T., Mcnaughton, D., Wood, B. R., et al. (2010). Early detection of the chemical changes occurring during the induction and prevention of autoimmune-mediated demyelination detected by FT-IR imaging. Neuroimage 49, 1180–1189. doi:10.1016/j.neuroimage.2009.09.053
Higgins, A. Z., and Karlsson, J. O. M. (2008a). Coincidence error during measurement of cellular osmotic properties by the electrical sensing zone method. Cryoletters 29, 447–461.
Higgins, A. Z., and Karlsson, J. O. M. (2010). Curve fitting approach for measurement of cellular osmotic properties by the electrical sensing zone method. II. Membrane water permeability. Cryobiology 60, 117–128. doi:10.1016/j.cryobiol.2009.10.002
Higgins, A. Z., and Karlsson, J. O. M. (2008b). Curve fitting approach for measurement of cellular osmotic properties by the electrical sensing zone method. I. Osmotically inactive volume. Cryobiology 57, 223–233. doi:10.1016/j.cryobiol.2008.09.001
Higgins, M. J., Polcik, M., Fukuma, T., Sader, J. E., Nakayama, Y., and Jarvis, S. P. (2006). Structured water layers adjacent to biological membranes. Biophysical J. 91, 2532–2542. doi:10.1529/biophysj.106.085688
Hirsch, J., and Gallian, E. (1968). Methods for the determination of adipose cell size in man and animals. J. Lipid Res. 9, 110–119. doi:10.1016/s0022-2275(20)43151-7
Hoffmann, E. M., and Brogden, M. C. (1976). The use of sucrose as a cryoprotective agent for the labile sheep erythrocyte-complement component-intermediate complex, EAC. J. Immunol. Methods 10, 293–299. doi:10.1016/0022-1759(76)90180-0
Hofmann, S., Voïtchovsky, K., Spijker, P., Schmidt, M., and Stumpf, T. (2016). Visualising the molecular alteration of the calcite (104) – water interface by sodium nitrate. Sci. Rep. 6, 21576. doi:10.1038/srep21576
Hon, W. C., Griffith, M., Chong, P., and Yang, D. (1994). Extraction and isolation of antifreeze proteins from winter rye (secale cereale L) leaves. Plant Physiol. 104, 971–980. doi:10.1104/pp.104.3.971
Hossain, K. R., Holt, S. A., Le Brun, A. P., Al Khamici, H., and Valenzuela, S. M. (2017). X-Ray and neutron reflectivity study shows that CLIC1 undergoes cholesterol-dependent structural reorganization in lipid monolayers. Langmuir 33, 12497–12509. doi:10.1021/acs.langmuir.7b02872
Hsieh, M.-H., Huang, P.-T., Liou, H.-H., Liang, P.-H., Chen, P.-M., Holt, S. A., et al. (2018). The penetration depth for hanatoxin partitioning into the membrane hydrocarbon core measured with neutron reflectivity. Langmuir 34, 9036–9046. doi:10.1021/acs.langmuir.8b01076
Hubálek, Z. (2003). Protectants used in the cryopreservation of microorganisms. Cryobiology 46, 205–229. doi:10.1016/s0011-2240(03)00046-4
Hungerford, A., Bakos, H. W., and Aitken, R. J. (2023). Sperm cryopreservation: Current status and future developments. Reproduction, Fertil. Dev. 35, 265–281. doi:10.1071/rd22219
Imamura, K., Murai, K., Korehisa, T., Shimizu, N., Yamahira, R., Matsuura, T., et al. (2014). Characteristics of sugar surfactants in stabilizing proteins during freeze–thawing and freeze–drying. J. Pharm. Sci. 103, 1628–1637. doi:10.1002/jps.23988
Iwatani, M., Ikegami, K., Kremenska, Y., Hattori, N., Tanaka, S., Yagi, S., et al. (2006). Dimethyl sulfoxide has an impact on epigenetic profile in mouse embryoid body. Stem Cells 24, 2549–2556. doi:10.1634/stemcells.2005-0427
Jacobs, M. H. (1933). The simultaneous measurement of cell permeability to water and to dissolved substances. J. Cell. Comp. Physiology 2, 427–444. doi:10.1002/jcp.1030020405
Jacobsen, I. A., Pegg, D. E., Starklint, H., Chemnitz, J., Hunt, C., Barfort, P., et al. (1984). Effect of cooling and warming rate on glycerolized rabbit kidneys. Cryobiology 21, 637–653. doi:10.1016/0011-2240(84)90223-2
Jalal, N., and Zidi, M. (2018). Effect of cryopreservation at −80°C on visco-hyperelastic properties of skeletal muscle tissue. J. Mech. Behav. Biomed. Mater. 77, 572–577. doi:10.1016/j.jmbbm.2017.10.006
Jang, T. H., Park, S. C., Yang, J. H., Kim, J. Y., Seok, J. H., Park, U. S., et al. (2017). Cryopreservation and its clinical applications. Integr. Med. Res. 6, 12–18. doi:10.1016/j.imr.2016.12.001
Jesus, A. R., Meneses, L., Duarte, A. R. C., and Paiva, A. (2021). Natural deep eutectic systems, an emerging class of cryoprotectant agents. Cryobiology 101, 95–104. doi:10.1016/j.cryobiol.2021.05.002
Jin, B., Kleinhans, F. W., and Mazur, P. (2014). Survivals of mouse oocytes approach 100% after vitrification in 3-fold diluted media and ultra-rapid warming by an IR laser pulse. Cryobiology 68, 419–430. doi:10.1016/j.cryobiol.2014.03.005
Kandror, O., Deleon, A., and Goldberg, A. L. (2002). Trehalose synthesis is induced upon exposure of Escherichia coli to cold and is essential for viability at low temperatures. Proc. Natl. Acad. Sci. U. S. A. 99, 9727–9732. doi:10.1073/pnas.142314099
Kariuki, R., Penman, R., Bryant, S. J., Orrell-Trigg, R., Meftahi, N., Crawford, R. J., et al. (2022). Behavior of citrate-capped ultrasmall gold nanoparticles on a supported lipid bilayer interface at atomic resolution. ACS Nano 16, 17179–17196. doi:10.1021/acsnano.2c07751
Karow, A. M. (1969). Cryoprotectants—A new class of drugs. J. Pharm. Pharmacol. 21, 209–223. doi:10.1111/j.2042-7158.1969.tb08235.x
Kedem, O., and Katchalsky, A. (1958). Thermodynamic analysis of the permeability of biological membranes to non-electrolytes. Biochimica Biophysica Acta 27, 229–246. doi:10.1016/0006-3002(58)90330-5
Kent, B., Hauss, T., Deme, B., Cristiglio, V., Darwish, T., Hunt, T., et al. (2015). Direct comparison of disaccharide interaction with lipid membranes at reduced hydrations. Langmuir 31, 9134–9141. doi:10.1021/acs.langmuir.5b02127
Kent, B., Hunt, T., Darwish, T. A., Hauss, T., Garvey, C. J., and Bryant, G. (2014). Localization of trehalose in partially hydrated DOPC bilayers: Insights into cryoprotective mechanisms. J. R. Soc. Interface 11, 20140069. doi:10.1098/rsif.2014.0069
Keskin, N., Erdogan, C., Bucak, M. N., Ozturk, A. E., Bodu, M., Ili, P., et al. (2020). Cryopreservation effects on ram sperm ultrastructure. Biopreservation Biobanking 18, 441–448. doi:10.1089/bio.2020.0056
Khosla, K., Kangas, J., Liu, Y., Zhan, L., Daly, J., Hagedorn, M., et al. (2020). Cryopreservation and laser nanowarming of zebrafish embryos followed by hatching and spawning. Adv. Biosyst. 4, e2000138. doi:10.1002/adbi.202000138
Kiselev, M. A., Lesieur, P., Kisselev, A. M., Grabielle-Madelmond, C., and Ollivon, M. (1999). DMSO-induced dehydration of DPPC membranes studied by X-ray diffraction, small-angle neutron scattering, and calorimetry. J. Alloys Compd. 286, 195–202. doi:10.1016/s0925-8388(98)01006-8
Kitson, P. J., Rosnes, M. H., Sans, V., Dragone, V., and Cronin, L. (2012). Configurable 3D-Printed millifluidic and microfluidic ‘lab on a chip’ reactionware devices. Lab a Chip 12, 3267–3271. doi:10.1039/c2lc40761b
Kleinhans, F. W. (1998). Membrane permeability modeling: Kedem–Katchalsky vs a two-parameter formalism. Cryobiology 37, 271–289. doi:10.1006/cryo.1998.2135
Korrapati, M. C., Shaner, B. E., and Schnellmann, R. G. (2012). Recovery from glycerol-induced acute kidney injury is accelerated by suramin. J. Pharmacol. Exp. Ther. 341, 126–136. doi:10.1124/jpet.111.190249
Koster, K. L., and Bryant, G. (2006). “Dehydration in model membranes and protoplasts: Contrasting effects at low, intermediate and high hydrations,” in Cold hardiness in plants: Molecular genetics, cell biology and physiology. Editors T. H. H. Chen, M. Uemura, and S. Fujikawa (United Kingdom: CABI), 219–234.
Koster, K. L., Lei, Y. P., Anderson, M., Martin, S., and Bryant, G. (2000). Effects of vitrified and nonvitrified sugars on phosphatidylcholine fluid-to-gel phase transitions. Biophysical J. 78, 1932–1946. doi:10.1016/s0006-3495(00)76741-5
Koster, K. L., and Lynch, D. V. (1992). Solute accumulation and compartmentation during the cold-acclimation of puma rye. Plant Physiol. 98, 108–113. doi:10.1104/pp.98.1.108
Koster, K. L., Webb, M. S., Bryant, G., and Lynch, D. V. (1994). Interactions between soluble sugars and POPC (1-palmitoyl-2-oleoylphosphatidylcholine) during dehydration: Vitrification of sugars alters the phase behavior of the phospholipid. BBA - Biomembr. 1193, 143–150. doi:10.1016/0005-2736(94)90343-3
Krueger, S. (2001). Neutron reflection from interfaces with biological and biomimetic materials. Curr. Opin. Colloid and Interface Sci. 6, 111–117. doi:10.1016/s1359-0294(01)00073-5
Kuiper, M. J., Morton, C. J., Abraham, S. E., and Gray-Weale, A. (2015). The biological function of an insect antifreeze protein simulated by molecular dynamics. eLife 4, e05142. doi:10.7554/elife.05142
Kuleshova, L. L., Shaw, J. M., and Trounson, A. O. (2001). Studies on replacing most of the penetrating cryoprotectant by polymers for embryo cryopreservation. Cryobiology 43, 21–31. doi:10.1006/cryo.2001.2335
Lakey, J. H., Paracini, N., and Clifton, L. A. (2022). Exploiting neutron scattering contrast variation in biological membrane studies. Biophys. Rev. 3, 021307. doi:10.1063/5.0091372
Larson, D. J., Middle, L., Vu, H., Zhang, W., Serianni, A. S., Duman, J., et al. (2014). Wood frog adaptations to overwintering in Alaska: New limits to freezing tolerance. J. Exp. Biol. 217, 2193–2200. doi:10.1242/jeb.101931
Layman, D. L. (1987). Growth inhibitory effects of dimethyl sulfoxide and dimethyl sulfone on vascular smooth muscle and endothelial cells in vitro. Vitro Cell. Dev. Biol. 23, 422–428. doi:10.1007/bf02623858
Leclère, M., Kwok, B. K., Wu, L. K., Allan, D. S., and Ben, R. N. (2011). C-linked antifreeze glycoprotein (C-afgp) analogues as novel cryoprotectants. Bioconjugate Chem. 22, 1804–1810. doi:10.1021/bc2001837
Lenne, T., Bryant, G., Garvey, C. J., Kelderling, U., and Koster, K. L. (2006). Location of sugars in multilamellar membranes at low hydration. Phys. B-Condensed Matter 385-86, 862–864. doi:10.1016/j.physb.2006.05.127
Lenne, T., Bryant, G., Holcomb, R., and Koster, K. L. (2007). How much solute is needed to inhibit the fluid to gel membrane phase transition at low hydration? BBA-Biomembranes 1768, 1019–1022. doi:10.1016/j.bbamem.2007.01.008
Lenne, T., Garvey, C. J., Koster, K. L., and Bryant, G. (2009). Effects of sugars on lipid bilayers during dehydration - SAXS/WAXS measurements and quantitative model. J. Phys. Chem. B 113, 2486–2491. doi:10.1021/jp808670t
Lewis, J. K., Bischof, J. C., Braslavsky, I., Brockbank, K. G. M., Fahy, G. M., Fuller, B. J., et al. (2016). The Grand Challenges of Organ Banking: Proceedings from the first global summit on complex tissue cryopreservation. Cryobiology 72, 169–182. doi:10.1016/j.cryobiol.2015.12.001
Lipinski, C. A., Lombardo, F., Dominy, B. W., and Feeney, P. J. (1997). Experimental and computational approaches to estimate solubility and permeability in drug discovery and development settings. Adv. Drug Deliv. Rev. 23, 3–25. doi:10.1016/s0169-409x(96)00423-1
Liu, D., Song, K., Chen, W., Chen, J., Sun, G., and Li, L. (2022). Review: Current progresses of small-angle neutron scattering on soft-matters investigation. Nucl. Anal. 1, 100011. doi:10.1016/j.nucana.2022.100011
Liu, X. (2021). “IR spectrum and characteristic absorption bands,” in Organic chemistry I (Surrey, BC, Canada: Kwantlen Polytechnic University).
Loh, S.-H., and Jarvis, S. P. (2010). Visualization of ion distribution at the Mica−Electrolyte interface. Langmuir 26, 9176–9178. doi:10.1021/la1011378
Lovelock, J. E., and Bishop, M. W. H. (1959). Prevention of freezing damage to living cells by dimethyl sulphoxide. Nature 183, 1394–1395. doi:10.1038/1831394a0
Lovelock, J. E. (1953b). Het mechanism of the protective action of glycerol against haemolysis by freezing and thawing. Biochimica Biophysica Acta 11, 28–36. doi:10.1016/0006-3002(53)90005-5
Lovelock, J. E. (1953a). The haemolysis of human red blood-cells by freezing and thawing. Biochimica Biophysica Acta 10, 414–426. doi:10.1016/0006-3002(53)90273-x
Lynch, A. L., Chen, R., Dominowski, P. J., Shalaev, E. Y., Yancey, R. J., and Slater, N. K. H. (2010). Biopolymer mediated trehalose uptake for enhanced erythrocyte cryosurvival. Biomaterials 31, 6096–6103. doi:10.1016/j.biomaterials.2010.04.020
Lysak, D., Brychtová, M., Leba, M., Čedíková, M., Georgiev, D., Jindra, P., et al. (2021). Long-term cryopreservation does not affect quality of peripheral blood stem cell grafts: A comparative study of native, short-term and long-term cryopreserved haematopoietic stem cells. Cell Transplant. 30, 096368972110360. doi:10.1177/09636897211036004
Macaleese, L., and Maître, P. (2007). Infrared spectroscopy of organometallic ions in the gas phase: From model to real world complexes. Mass Spectrom. Rev. 26, 583–605. doi:10.1002/mas.20138
Magonov, S. N., Elings, V., and Whangbo, M. H. (1997). Phase imaging and stiffness in tapping-mode atomic force microscopy. Surf. Sci. 375, L385–L391. doi:10.1016/s0039-6028(96)01591-9
Malajczuk, C. J., Armstrong, B. I., Stachura, S. S., and Mancera, R. L. (2022a). Mechanisms of interaction of small hydroxylated cryosolvents with dehydrated model cell membranes: Stabilization vs destruction. J. Phys. Chem. B 126, 197–216. doi:10.1021/acs.jpcb.1c07769
Malajczuk, C. J., Stachura, S. S., Hendry, J. O., and Mancera, R. L. (2022b). Redefining the molecular interplay between dimethyl sulfoxide, lipid bilayers, and dehydration. J. Phys. Chem. B 126, 2513–2529. doi:10.1021/acs.jpcb.2c00353
Manuchehrabadi, N., Gao, Z., Zhang, J., Ring, H. L., Shao, Q., Liu, F., et al. (2017). Improved tissue cryopreservation using inductive heating of magnetic nanoparticles. Sci. Transl. Med. 9, eaah4586. doi:10.1126/scitranslmed.aah4586
Martinez-Madrid, B., Dolmans, M.-M., Van Langendonckt, A., Defrère, S., and Donnez, J. (2004). Freeze-thawing intact human ovary with its vascular pedicle with a passive cooling device. Fertil. Steril. 82, 1390–1394. doi:10.1016/j.fertnstert.2004.06.036
Mazur, P. (1970). Cryobiology: The freezing of biological systems. Science 168, 939–949. doi:10.1126/science.168.3934.939
Mazur, P., Farrant, J., Leibo, S. P., and Chu, E. H. Y. (1969). Survival of hamster tissue culture cells after freezing and thawing: Interactions between protective solutes and cooling and warming rates. Cryobiology 6, 1–9. doi:10.1016/s0011-2240(69)80002-7
Mazur, P. (1963). Kinetics of water loss from cells at subzero temperatures and the likelihood of intracellular freezing. J. General Physiology 47, 347–369. doi:10.1085/jgp.47.2.347
Mazur, P., Leibo, S. P., and Chu, E. H. Y. (1972). A two-factor hypothesis of freezing injury: Evidence from Chinese hamster tissue-culture cells. Exp. Cell Res. 71, 345–355. doi:10.1016/0014-4827(72)90303-5
Mazur, P., Leibo, S. P., and Miller, R. H. (1974). Permeability of the bovine red cell to glycerol in hyperosmotic solutions at various temperatures. J. Membr. Biol. 15, 107–136. doi:10.1007/bf01870084
Mazur, P., and Miller, R. H. (1976). Permeability of the human erythrocyte to glycerol in 1 and 2 m solutions at 0 or 20 °C. Cryobiology 13, 507–522. doi:10.1016/0011-2240(76)90144-9
Mazur, P. (2004). “Principles of cryobiology,” in Life in the frozen state. Editors B. J. Fuller, N. Lane, and E. E. Benson (Boca Raton: CRC Press), 3–65.
Mazur, P., and Schneider, U. (1986). Osmotic responses of preimplantation mouse and bovine embryos and their cryobiological implications. Cell Biophys. 8, 259–285. doi:10.1007/bf02788516
Mazur, P. (1977). The role of intracellular freezing in the death of cells cooled at supraoptimal rates. Cryobiology 14, 251–272. doi:10.1016/0011-2240(77)90175-4
Mcdonald, S., Elbourne, A., Warr, G. G., and Atkin, R. (2016). Metal ion adsorption at the ionic liquid-mica interface. Nanoscale 8, 906–914. doi:10.1039/c5nr05833c
Mcgrath, J. J. (1997). Quantitative measurement of cell membrane transport: Technology and applications. Cryobiology 34, 315–334. doi:10.1006/cryo.1997.2013
Mehl, P., and Boutron, P. (1988). Cryoprotection of red blood cells by 1,3-butanediol and 2,3-butanediol. Cryobiology 25, 44–54. doi:10.1016/0011-2240(88)90019-3
Mehl, P. M. (1993). Nucleation and crystal growth in a vitrification solution tested for organ cryopreservation by vitrification. Cryobiology 30, 509–518. doi:10.1006/cryo.1993.1051
Meryman, H. T. (1971). Cryoprotective agents. Cryobiology 8, 173–183. doi:10.1016/0011-2240(71)90024-1
Miyamoto, Y., Oishi, K., Yukawa, H., Noguchi, H., Sasaki, M., Iwata, H., et al. (2012). Cryopreservation of human adipose tissue-derived stem/progenitor cells using the silk protein sericin. Cell Transplant. 21, 617–622. doi:10.3727/096368911x605556
Model, M. A. (2018). Methods for cell volume measurement. Cytom. Part A 93, 281–296. doi:10.1002/cyto.a.23152
Mourant, J. R., Short, K. W., Carpenter, S., Kunapareddy, N., Coburn, L., Powers, T. M., et al. (2005). Biochemical differences in tumorigenic and nontumorigenic cells measured by Raman and infrared spectroscopy. J. Biomed. Opt. 10, 031106. doi:10.1117/1.1928050
Movasaghi, Z., Rehman, S., and Ur Rehman, D. I. (2008). Fourier transform infrared (FTIR) spectroscopy of biological tissues. Appl. Spectrosc. Rev. 43, 134–179. doi:10.1080/05704920701829043
Mukaida, T., Wada, S., Takahashi, K., Pedro, P. B., An, T. Z., and Kasai, M. (1998). Vitrification of human embryos based on the assessment of suitable conditions for 8-cell mouse embryos. Hum. Reprod. 13, 2874–2879. doi:10.1093/humrep/13.10.2874
Müller, D. J., and Dufrêne, Y. F. (2011). Atomic force microscopy: A nanoscopic window on the cell surface. Trends Cell Biol. 21, 461–469. doi:10.1016/j.tcb.2011.04.008
Nagle, J. F., and Tristram-Nagle, S. (2000). Structure of lipid bilayers. Biochimica Biophysica Acta-Reviews Biomembr. 1469, 159–195. doi:10.1016/s0304-4157(00)00016-2
Nawroth, F., Isachenko, V., Dessole, S., Rahimi, G., Farina, M., Vargiu, N., et al. (2002). Vitrification of human spermatozoa without cryoprotectants. Cryo Lett. 23, 93–102.
Nguyen, K., Kc, S., Gonzalez, T., Tapia, H., and Boothby, T. C. (2022). Trehalose and tardigrade CAHS proteins work synergistically to promote desiccation tolerance. Commun. Biol. 5, 1046. doi:10.1038/s42003-022-04015-2
Noiles, E. E., Thompson, K. A., and Storey, B. T. (1997). Water Permeability,Lp, of the mouse sperm plasma membrane and its activation energy are strongly dependent on interaction of the plasma membrane with the sperm cytoskeleton. Cryobiology 35, 79–92. doi:10.1006/cryo.1997.2033
Oliveira, O. N., Caseli, L., and Ariga, K. (2022). The past and the future of Langmuir and Langmuir–blodgett films. Chem. Rev. 122, 6459–6513. doi:10.1021/acs.chemrev.1c00754
Osei-Bempong, C., Ghareeb, A. E., Lako, M., Figueiredo, F. C., and Armitage, W. J. (2018). Defining the optimal cryoprotectant and concentration for cryopreservation of limbal stem cells. Cryobiology 84, 98–102. doi:10.1016/j.cryobiol.2018.07.008
Page, A. J., Elbourne, A., Stefanovic, R., Addicoat, M. A., Warr, G. G., Voïtchovsky, K., et al. (2014). 3-Dimensional atomic scale structure of the ionic liquid–graphite interface elucidated by AM-AFM and quantum chemical simulations. Nanoscale 6, 8100–8106. doi:10.1039/c4nr01219d
Pakhomov, O., Gurina, T., Mazaeva, V., Polyakova, A., Deng, B., Legach, E., et al. (2022). Phase transitions and mechanisms of cryoprotection of serum-/xeno-free media based on dextran and dimethyl sulfoxide. Cryobiology 107, 13–22. doi:10.1016/j.cryobiol.2022.06.004
Parker, F. (2012). Applications of infrared spectroscopy in biochemistry, biology, and medicine. Germany: Springer Science and Business Media.
Parot, P., Dufrêne, Y. F., Hinterdorfer, P., Le Grimellec, C., Navajas, D., Pellequer, J.-L., et al. (2007). Past, present and future of atomic force microscopy in life sciences and medicine. J. Mol. Recognit. 20, 418–431. doi:10.1002/jmr.857
Pasquini, C. (2018). Near infrared spectroscopy: A mature analytical technique with new perspectives – a review. Anal. Chim. Acta 1026, 8–36. doi:10.1016/j.aca.2018.04.004
Pearce, R. S. (2004). “Adaptation of higher plants to freezing,” in Life in the frozen state. Editors B. J. Fuller, N. Lane, and E. E. Benson (Boca Raton: CRC Press), 171–203.
Pegg, D. E. (2007). “Principles of cryopreservation,” in Cryopreservation and freeze-drying protocols. Editors J. G. Day, and G. N. Stacey (Totowa, NJ: Humana Press), 39–57.
Pegg, D. E. (2020). The relevance of ice crystal formation for the cryopreservation of tissues and organs. Cryobiology 93, 3–11. doi:10.1016/j.cryobiol.2020.01.005
Pegg, D. E., Wang, L., Vaughan, D., and Hunt, C. J. (2006). Cryopreservation of articular cartilage. Part 2: Mechanisms of cryoinjury. Cryobiology 52, 347–359. doi:10.1016/j.cryobiol.2006.01.007
Pellicer, A., and Del Carmen Bravo, M. (2011). “Near-infrared spectroscopy: A methodology-focused review,” in Seminars in fetal and neonatal medicine (Netherlands: Elsevier), 42–49.
Pham, D. Q., Bryant, S. J., Cheeseman, S., Huang, L. Z. Y., Bryant, G., Dupont, M. F., et al. (2020). Micro-to nano-scale chemical and mechanical mapping of antimicrobial-resistant fungal biofilms. Nanoscale 12, 19888–19904. doi:10.1039/d0nr05617k
Polge, C., Smith, A. U., and Parkes, A. S. (1949). Revival of spermatozoa after vitrification and dehydration at low temperatures. Nature 164, 666. doi:10.1038/164666a0
Pollock, G. A., Hamlyn, L., Maguire, S. H., Stewart-Richardson, P. A., and Hardie, I. R. (1991). Effects of four cryoprotectants in combination with two vehicle solutions on cultured vascular endothelial cells. Cryobiology 28, 413–421. doi:10.1016/0011-2240(91)90049-t
Preston, J., and Sandve, S. (2013). Adaptation to seasonality and the winter freeze. Front. Plant Sci. 4, 167. doi:10.3389/fpls.2013.00167
Radmacher, M. (1997). Measuring the elastic properties of biological samples with the AFM. IEEE Eng. Med. Biol. Mag. 16, 47–57. doi:10.1109/51.582176
Radmacher, M., Tillamnn, R. W., Fritz, M., and Gaub, H. E. (1992). From molecules to cells: Imaging soft samples with the atomic force microscope. Science 257, 1900–1905. doi:10.1126/science.1411505
Raju, R., Bryant, S. J., Wilkinson, B. L., and Bryant, G. (2021). The need for novel cryoprotectants and cryopreservation protocols: Insights into the importance of biophysical investigation and cell permeability. Biochimica Biophysica Acta (BBA) - General Subj. 1865, 129749. doi:10.1016/j.bbagen.2020.129749
Raju, R., Höhn, H., Karnutsch, C., Khoshmanesh, K., and Bryant, G. (2019a). Measuring volume kinetics of human monocytes in response to cryoprotectants using microfluidic technologies. Appl. Phys. Lett. 114, 223702. doi:10.1063/1.5096199
Raju, R., Merl, T., Adam, M. K., Staykov, E., Ben, R. N., Bryant, G., et al. (2019b). n-Octyl (Thio)glycosides as potential cryoprotectants: Glass transition behaviour, membrane permeability, and ice recrystallization inhibition studies. Aust. J. Chem. 72, 637–643. doi:10.1071/ch19159
Raju, R., Torrent-Burgués, J., and Bryant, G. (2020). Interactions of cryoprotective agents with phospholipid membranes - a Langmuir monolayer study. Chem. Phys. Lipids 231, 104949. doi:10.1016/j.chemphyslip.2020.104949
Rao, W., Huang, H., Wang, H., Zhao, S., Dumbleton, J., Zhao, G., et al. (2015). Nanoparticle-mediated intracellular delivery enables cryopreservation of human adipose-derived stem cells using trehalose as the sole cryoprotectant. ACS Appl. Mater. Interfaces 7, 5017–5028. doi:10.1021/acsami.5b00655
Rendal-Vázquez, M. E., Maneiro-Pampín, E., Rodríguez-Cabarcos, M., Fernández-Mallo, O., López De Ullibarri, I., Andión-Núñez, C., et al. (2001). Effect of cryopreservation on human articular chondrocyte viability, proliferation, and collagen expression. Cryobiology 42, 2–10. doi:10.1006/cryo.2001.2294
Rhys, N. H., Barlow, D. J., Lawrence, M. J., and Lorenz, C. D. (2022). On the interactions of diols and DMPC monolayers. J. Mol. Liq. 364, 119963. doi:10.1016/j.molliq.2022.119963
Ricci, M., Spijker, P., and Voïtchovsky, K. (2014). Water-induced correlation between single ions imaged at the solid–liquid interface. Nat. Commun. 5, 4400. doi:10.1038/ncomms5400
Ricci, M., Trewby, W., Cafolla, C., and Voïtchovsky, K. (2017). Direct observation of the dynamics of single metal ions at the interface with solids in aqueous solutions. Sci. Rep. 7, 43234. doi:10.1038/srep43234
Rico, E. P., Rosemberg, D. B., Senger, M. R., De Bem Arizi, M., Bernardi, G. F., Dias, R. D., et al. (2006). Methanol alters ecto-nucleotidases and acetylcholinesterase in zebrafish brain. Neurotoxicology Teratol. 28, 489–496. doi:10.1016/j.ntt.2006.05.001
Rodger, J. C. (2019). “Marsupials: Progress and prospects,” in Reproductive sciences in animal conservation. Editors P. Comizzoli, J. L. Brown, and W. V. Holt (Cham: Springer International Publishing), 309–325.
Roggo, Y., Chalus, P., Maurer, L., Lema-Martinez, C., Edmond, A., and Jent, N. (2007). A review of near infrared spectroscopy and chemometrics in pharmaceutical technologies. J. Pharm. Biomed. Analysis 44, 683–700. doi:10.1016/j.jpba.2007.03.023
Rolfe, P. (2000). In vivo near-infrared spectroscopy. Annu. Rev. Biomed. Eng. 2, 715–754. doi:10.1146/annurev.bioeng.2.1.715
Roy, S., Perez-Guaita, D., Andrew, D. W., Richards, J. S., Mcnaughton, D., Heraud, P., et al. (2017). Simultaneous ATR-FTIR based determination of malaria parasitemia, glucose and urea in whole blood dried onto a glass slide. Anal. Chem. 89, 5238–5245. doi:10.1021/acs.analchem.6b04578
Ruan, H., Wang, T., and Gao, C. (2020). Microwave-water bath hybrid warming for frozen cryoprotectant solution using a helical antenna. Cryo Lett. 41, 26–30.
Sasaki, M., Kato, Y., Yamada, H., and Terada, S. (2005). Development of a novel serum-free freezing medium for mammalian cells using the silk protein sericin. Biotechnol. Appl. Biochem. 42, 183–188. doi:10.1042/ba20050019
Scholander, P. F., Van Dam, L., Kanwisher, J. W., Hammel, H. T., and Gordon, M. S. (1957). Supercooling and osmoregulation in arctic fish. J. Cell. Comp. Physiology 49, 5–24. doi:10.1002/jcp.1030490103
Scott, K. L., Lecak, J., and Acker, J. P. (2005). Biopreservation of red blood cells: Past, present, and future. Transfus. Med. Rev. 19, 127–142. doi:10.1016/j.tmrv.2004.11.004
Segura, J. J., Elbourne, A., Wanless, E. J., Warr, G. G., Voitchovsky, K., and Atkin, R. (2013). Adsorbed and near surface structure of ionic liquids at a solid interface. Phys. Chem. Chem. Phys. 15, 3320–3328. doi:10.1039/c3cp44163f
Senden, T. J. (2001). Force microscopy and surface interactions. Curr. Opin. Colloid Interface Sci. 6, 95–101. doi:10.1016/s1359-0294(01)00067-x
Shaffner, C. S. (1942). Longevity of fowl spermatozoa in frozen condition. Science 96, 337. doi:10.1126/science.96.2493.337.a
Shan, Y., and Wang, H. (2015). The structure and function of cell membranes examined by atomic force microscopy and single-molecule force spectroscopy. Chem. Soc. Rev. 44, 3617–3638. doi:10.1039/c4cs00508b
Sharma, A., Rao, J. S., Han, Z., Gangwar, L., Namsrai, B., Gao, Z., et al. (2021). Vitrification and nanowarming of kidneys. Adv. Sci. 8, 168. doi:10.1016/j.cryobiol.2021.11.044
Sharpe, S. W., Johnson, T. J., Sams, R. L., Chu, P. M., Rhoderick, G. C., and Johnson, P. A. (2004). Gas-phase databases for quantitative infrared spectroscopy. Appl. Spectrosc. 58, 1452–1461. doi:10.1366/0003702042641281
Shashkov, S. N., Kiselev, M. A., Tioutiounnikov, S. N., Kiselev, A. M., and Lesieur, P. (1999). The study of DMSO/water and DPPC/DMSO/water system by means of the X-ray, neutron small-angle scattering, calorimetry and IR spectroscopy. Phys. B Condens. Matter 271, 184–191. doi:10.1016/s0921-4526(99)00214-8
Shaw, Z. L., Cheeseman, S., Huang, L. Z. Y., Penman, R., Ahmed, T., Bryant, S. J., et al. (2022). Illuminating the biochemical interaction of antimicrobial few-layer black phosphorus with microbial cells using synchrotron macro-ATR-FTIR. J. Mater. Chem. B 10, 7527–7539. doi:10.1039/d1tb02575a
Shettles, L. B. (1939). The respiration of human spermatozoa and their response to various gases and low temperatures. Am. J. Physiology 128, 408–415. doi:10.1152/ajplegacy.1939.128.2.408
Shu, Z., Heimfeld, S., and Gao, D. (2014). Hematopoietic SCT with cryopreserved grafts: Adverse reactions after transplantation and cryoprotectant removal before infusion. Bone marrow Transplant. 49, 469–476. doi:10.1038/bmt.2013.152
Shu, Z., Hughes, S. M., Fang, C., Huang, J., Fu, B., Zhao, G., et al. (2016). A study of the osmotic characteristics, water permeability, and cryoprotectant permeability of human vaginal immune cells. Cryobiology 72, 93–99. doi:10.1016/j.cryobiol.2016.03.003
Sieme, H., Oldenhof, H., and Wolkers, W. (2015). Sperm membrane behaviour during cooling and cryopreservation. Reproduction Domest. Animals 50, 20–26. doi:10.1111/rda.12594
Smith, A. U. (1950). PREVENTION OF HqMOLYSIS DURING FREEZING AND THAWING OF RED BLOOD-CELLS. Lancet 256, 910–911. doi:10.1016/s0140-6736(50)91861-7
Sommerfeld, V., and Niemann, H. (1999). Cryopreservation of bovine in vitro produced embryos using ethylene glycol in controlled freezing or vitrification. Cryobiology 38, 95–105. doi:10.1006/cryo.1999.2159
Sputtek, A., Kühnl, P., and Rowe, A. W. (2007). Cryopreservation of erythrocytes, thrombocytes, and lymphocytes. Transfus. Med. Hemotherapy 34, 262–267. doi:10.1159/000104136
Sputtek, A., Singbartl, G., Langer, R., Schleinzer, W., Henrich, H. A., and Kühnl, P. (1994). Cryopreservation of erythrocytes using hydroxyethyl starch: In vivo results of an autologous retransfusion model in humans. Beitr Infusionsther Transfusionsmed 32, 44–47.
Sputtek, A., and Sputtek, R. (2004). “Cryopreservation in transfusion medicine and hematology,” in life in the frozen state. Editors B. J. Fuller, N. Lane, and E. E. Benson (Boca Raton: CRC Press), 483–504.
Stacy, D. H., Miloš, F., and Jiří, Z. (2019). “Methods of thermal analysis as a tool to develop cryopreservation protocols of vegetatively propagated crops," in cryopreservation. Editors Q. Marian (Rijeka: IntechOpen).
Stefanic, M., Ward, K., Tawfik, H., Seemann, R., Baulin, V., Guo, Y., et al. (2017). Apatite nanoparticles strongly improve red blood cell cryopreservation by mediating trehalose delivery via enhanced membrane permeation. Biomaterials 140, 138–149. doi:10.1016/j.biomaterials.2017.06.018
Steif, P. S., Palastro, M. C., and Rabin, Y. (2007). The effect of temperature gradients on stress development during cryopreservation via vitrification. Cell Preserv. Technol. 5, 104–115. doi:10.1089/cpt.2007.9994
Steponkus, P. L. (1984). Role of the plasma membrane in freezing injury and cold acclimation. Annu. Rev. Plant Physiology 35, 543–584. doi:10.1146/annurev.pp.35.060184.002551
Stoecklein, K. S., Ortega, M. S., Spate, L. D., Murphy, C. N., and Prather, R. S. (2021). Improved cryopreservation of in vitro produced bovine embryos using FGF2, LIF, and IGF1. PLOS ONE 16, e0243727. doi:10.1371/journal.pone.0243727
Stolzing, A., Naaldijk, Y., Fedorova, V., and Sethe, S. (2012). Hydroxyethylstarch in cryopreservation – mechanisms, benefits and problems. Transfus. Apher. Sci. 46, 137–147. doi:10.1016/j.transci.2012.01.007
Storey, K. B. (1990). Life in a frozen state: Adaptive strategies for natural freeze tolerance in amphibians and reptiles. Am. J. Physiol. 258, R559–R568. doi:10.1152/ajpregu.1990.258.3.r559
Stuart, B. H. (2006). “Infrared spectroscopy of biological applications,” in Encyclopedia of analytical chemistry: Applications, theory and instrumentation (New Jersey: John Wiley).
Sui, X., Wen, C., Yang, J., Guo, H., Zhao, W., Li, Q., et al. (2019). Betaine combined with membrane stabilizers enables solvent-free whole blood cryopreservation and one-step cryoprotectant removal. ACS Biomaterials Sci. Eng. 5, 1083–1091. doi:10.1021/acsbiomaterials.8b01286
Takeshi, F. (2010). Water distribution at solid/liquid interfaces visualized by frequency modulation atomic force microscopy. Sci. Technol. Adv. Mater. 11, 033003. doi:10.1088/1468-6996/11/3/033003
Tan, W. H., and Takeuchi, S. (2007). A trap-and-release integrated microfluidic system for dynamic microarray applications. Proc. Natl. Acad. Sci. U. S. A. 104, 1146–1151. doi:10.1073/pnas.0606625104
Tang, J. X. (2022). Opportunities and challenges in biological soft matter research. Front. Soft Matter 2, 2022. doi:10.3389/frsfm.2022.958524
Taylor, M. J., and Pegg, D. E. (1983). The effect of ice formation on the function of smooth muscle tissue stored at −21 or −60 °C. Cryobiology 20, 36–40. doi:10.1016/0011-2240(83)90057-3
Thalhammer, A., Bryant, G., Sulpice, R., and Hincha, D. K. (2014). Disordered cold Regulated15 proteins protect chloroplast membranes during freezing through binding and folding, but do not stabilize chloroplast enzymes in vivo. Plant Physiol. 166, 190–201. doi:10.1104/pp.114.245399
Thirumala, S., Wu, X., Gimble, J. M., and Devireddy, R. V. (2010). Evaluation of polyvinylpyrrolidone as a cryoprotectant for adipose tissue-derived adult stem cells. Tissue Eng. Part C. Methods 16, 783–792. doi:10.1089/ten.tec.2009.0552
Thordarson, P., Atkin, R., Kalle, W. H. J., Warr, G. G., and Braet, F. (2006). Developments in using scanning probe microscopy to study molecules on surfaces - from thin films and single-molecule conductivity to drug–living cell interactions. Aust. J. Chem. 59, 359–375. doi:10.1071/ch06043
Tidy, R. J., Lam, V., Fimognari, N., Mamo, J. C., and Hackett, M. J. (2017). FTIR studies of the similarities between pathology induced protein aggregation in vivo and chemically induced protein aggregation ex vivo. Vib. Spectrosc. 91, 68–76. doi:10.1016/j.vibspec.2016.09.016
Touhami, A., Nysten, B., and Dufrêne, Y. F. (2003). Nanoscale mapping of the elasticity of microbial cells by atomic force microscopy. Langmuir 19, 4539–4543. doi:10.1021/la034136x
Toyosawa, T., Oumi, Y., Ogawa, A., Sasaki, M., Yamada, H., and Terada, S. (2009).“Novel serum-free cryopreservation of mammalian cells using seric,” in Animal cell technology: Basic and applied aspects. Editors S. Shirahata, K. Ikura, M. Nagao, A. Ichikawa, and K. Teruya (Netherlands: Springer), 41–45.
Trewby, W., Faraudo, J., and Voïtchovsky, K. (2019). Long-lived ionic nano-domains can modulate the stiffness of soft interfaces. Nanoscale 11, 4376–4384. doi:10.1039/c8nr06339g
Trewby, W., Livesey, D., and Voitchovsky, K. (2016). Buffering agents modify the hydration landscape at charged interfaces. Soft Matter 12, 2642–2651. doi:10.1039/c5sm02445e
Tseng, H.-Y., Chen, C.-J., Wu, Z.-L., Ye, Y.-M., and Huang, G.-Z. (2022). The non-contact-based determination of the membrane permeability to water and dimethyl sulfoxide of cells virtually trapped in a self-induced micro-vortex. Lab a Chip 22, 354–366. doi:10.1039/d1lc00846c
Tu, F., Bhat, M., and Benson, J. D. (2022). Real-time computer assisted measurement of oocyte and embryo volume for assessment of transport parameters. Cryobiology 108, 19–26. doi:10.1016/j.cryobiol.2022.08.005
Tunçer, S., Gurbanov, R., Sheraj, I., Solel, E., Esenturk, O., and Banerjee, S. (2018). Low dose dimethyl sulfoxide driven gross molecular changes have the potential to interfere with various cellular processes. Sci. Rep. 8, 14828. doi:10.1038/s41598-018-33234-z
Tyler, A. I. I., Law, R. V., and Seddon, J. M. (2015). “X-ray diffraction of lipid model membranes,” in Methods in membrane lipids. Editor D. M. Owen (Totowa: Humana Press Inc), 199–225.
Tzur, A., Moore, J. K., Jorgensen, P., Shapiro, H. M., and Kirschner, M. W. (2011). Optimizing optical flow cytometry for cell volume-based sorting and analysis. PLoS One 6, e16053. doi:10.1371/journal.pone.0016053
Uchihashi, T., Higgins, M., Nakayama, Y., Sader, J. E., and Jarvis, S. P. (2005). Quantitative measurement of solvation shells using frequency modulated atomic force microscopy. Nanotechnology 16, S49–S53. doi:10.1088/0957-4484/16/3/009
Vaknin, D., Kjaer, K., Als-Nielsen, J., and Lösche, M. (1991). Structural properties of phosphatidylcholine in a monolayer at the air/water interface: Neutron reflection study and reexamination of x-ray reflection measurements. Biophysical J. 59, 1325–1332. doi:10.1016/s0006-3495(91)82347-5
Verdanova, M., Pytlik, R., and Kalbacova, M. H. (2014). Evaluation of sericin as a fetal bovine serum-replacing cryoprotectant during freezing of human mesenchymal stromal cells and human osteoblast-like cells. Biopreservation biobanking 12, 99–105. doi:10.1089/bio.2013.0078
Von Bomhard, A., Elsässer, A., Ritschl, L. M., Schwarz, S., and Rotter, N. (2016). Cryopreservation of endothelial cells in various cryoprotective agents and media – vitrification versus slow freezing methods. PLOS ONE 11, e0149660. doi:10.1371/journal.pone.0149660
Vongsvivut, J., Pérez-Guaita, D., Wood, B. R., Heraud, P., Khambatta, K., Hartnell, D., et al. (2019). Synchrotron macro ATR-FTIR microspectroscopy for high-resolution chemical mapping of single cells. Analyst 144, 3226–3238. doi:10.1039/c8an01543k
Walters, C., Wheeler, L., and Stanwood, P. C. (2004). Longevity of cryogenically stored seeds. Cryobiology 48, 229–244. doi:10.1016/j.cryobiol.2004.01.007
Walters, K. R., Serianni, A. S., Voituron, Y., Sformo, T., Barnes, B. M., and Duman, J. G. (2011). A thermal hysteresis-producing xylomannan glycolipid antifreeze associated with cold tolerance is found in diverse taxa. J. Comp. Physiology B 181, 631–640. doi:10.1007/s00360-011-0552-8
Wang, M., Karlsson, J. O., and Aksan, A. (2018). FTIR analysis of molecular changes associated with warming injury in cryopreserved leukocytes. Langmuir 35, 7552–7559. doi:10.1021/acs.langmuir.8b02982
Wanless, E. J., and Ducker, W. A. (1996). Organization of sodium dodecyl sulfate at the graphite solution interface. J. Phys. Chem. 100, 3207–3214. doi:10.1021/jp952439x
Waters, L., Ben, R., Acker, J. P., Padula, M. P., Marks, D. C., and Johnson, L. (2020). Characterizing the ability of an ice recrystallization inhibitor to improve platelet cryopreservation. Cryobiology 96, 152–158. doi:10.1016/j.cryobiol.2020.07.003
Webber, G. B., Wanless, E. J., Armes, S. P., Baines, F. L., and Biggs, S. (2001). Adsorption of amphiphilic diblock copolymer micelles at the mica/solution interface. Langmuir 17, 5551–5561. doi:10.1021/la010335+
Wiener, M. C., and White, S. H. (1991). Fluid bilayer structure determination by the combined use of x-ray and neutron diffraction. I. Fluid bilayer models and the limits of resolution. Biophysical J. 59, 162–173. doi:10.1016/s0006-3495(91)82208-1
Wiercigroch, E., Szafraniec, E., Czamara, K., Pacia, M. Z., Majzner, K., Kochan, K., et al. (2017). Raman and infrared spectroscopy of carbohydrates: A review. Spectrochimica Acta Part A Mol. Biomol. Spectrosc. 185, 317–335. doi:10.1016/j.saa.2017.05.045
Williams, R. J., and Harris, D. (1977). The distribution of cryoprotective agents into lipid interfaces. Cryobiology 14, 670–680. doi:10.1016/0011-2240(77)90022-0
Windrum, P., and Morris, T. C. M. (2003). Severe neurotoxicity because of dimethyl sulphoxide following peripheral blood stem cell transplantation. Bone Marrow Transplant. 31, 315. doi:10.1038/sj.bmt.1703848
Wlodkowic, D., Faley, S., Zagnoni, M., Wikswo, J. P., and Cooper, J. M. (2009). Microfluidic single-cell array cytometry for the analysis of tumor apoptosis. Anal. Chem. 81, 5517–5523. doi:10.1021/ac9008463
Wolfe, J., and Bryant, G. (2001). Cellular cryobiology: Thermodynamic and mechanical effects. Int. J. Refrig. 24, 438–450. doi:10.1016/s0140-7007(00)00027-x
Wolfe, J., and Bryant, G. (1999). Freezing, drying, and/or vitrification of membrane– solute–water systems. Cryobiology 39, 103–129. doi:10.1006/cryo.1999.2195
Wolfe, J., Bryant, G., and Koster, K. L. (2002). What is 'unfreezable water', how unfreezable is it and how much is there? Cryoletters 23, 157–166.
Wolkers, W. F., and Oldenhof, H. (2016). “Use of FTIR spectroscopy to study cells and tissues during cryopreservation processing and thermal therapies,” in Multiscale technologies for cryomedicine: Implementation from nano to macroscale (Singapore: World Scientific), 353–370.
Wolkers, W. F., and Oldenhof, H. (2015). Use of in situ Fourier transform infrared spectroscopy to study freezing and drying of cells. Cryopreserv. freeze-drying Protoc. 1257, 147–161. doi:10.1007/978-1-4939-2193-5_5
Wolkers, W. F., Walker, N. J., Tablin, F., and Crowe, J. H. (2001). Human platelets loaded with trehalose survive freeze-drying. Cryobiology 42, 79–87. doi:10.1006/cryo.2001.2306
Wood, B. R., Chiriboga, L., Yee, H., Quinn, M. A., Mcnaughton, D., and Diem, M. (2004). Fourier transform infrared (FTIR) spectral mapping of the cervical transformation zone, and dysplastic squamous epithelium. Gynecol. Oncol. 93, 59–68. doi:10.1016/j.ygyno.2003.12.028
Wood, B. R., Quinn, M. A., Burden, F. R., and Mcnaughton, D. (1996). An investigation into FTIR spectroscopy as a biodiagnostic tool for cervical cancer. Biospectroscopy 2, 143–153. doi:10.1002/(sici)1520-6343(1996)2:3<143:aid-bspy1>3.0.co;2-9
Wusteman, M. C., Pegg, D. E., Robinson, M. P., Wang, L.-H., and Fitch, P. (2002). Vitrification media: Toxicity, permeability, and dielectric properties. Cryobiology 44, 24–37. doi:10.1016/s0011-2240(02)00002-0
Xie, J., Ekpo, M. D., Xiao, J., Zhao, H., Bai, X., Liang, Y., et al. (2022). Principles and protocols for post-cryopreservation quality evaluation of stem cells in novel biomedicine. Front. Pharmacol. 13, 907943. doi:10.3389/fphar.2022.907943
Yamashita, H., Taoka, A., Uchihashi, T., Asano, T., Ando, T., and Fukumori, Y. (2012). Single-molecule imaging on living bacterial cell surface by high-speed AFM. J. Mol. Biol. 422, 300–309. doi:10.1016/j.jmb.2012.05.018
Yang, N. J., and Hinner, M. J. (2015). Getting across the cell membrane: An overview for small molecules, peptides, and proteins. Methods Mol. Biol. 1266, 29–53. doi:10.1007/978-1-4939-2272-7_3
Yao, X., and Matosevic, S. (2021). Cryopreservation of NK and T cells without DMSO for adoptive cell-based immunotherapy. BioDrugs 35, 529–545. doi:10.1007/s40259-021-00494-7
Yeung, C.-H., Anapolski, M., and Cooper, T. G. (2002). Measurement of volume changes in mouse spermatozoa using an electronic sizing analyzer and a flow cytometer: Validation and application to an infertile mouse model. J. Androl. 23, 522–528. doi:10.1002/j.1939-4640.2002.tb02274.x
Yurtsever, A., Yoshida, T., Behjat, A. B., Araki, Y., Hanayama, R., and Fukuma, T. (2021). Structural and mechanical characteristics of exosomes from osteosarcoma cells explored by 3D-atomic force microscopy. Nanoscale 13, 6661–6677. doi:10.1039/d0nr09178b
Zampolla, T., Spikings, E., Zhang, T., and Rawson, D. M. (2009). Effect of methanol and Me2SO exposure on mitochondrial activity and distribution in stage III ovarian follicles of zebrafish (Danio rerio). Cryobiology 59, 188–194. doi:10.1016/j.cryobiol.2009.07.002
Zenhäusern, R., Tobler, A., Leoncini, L., Hess, O. M., and Ferrari, P. (2000). Fatal cardiac arrhythmia after infusion of dimethyl sulfoxide-cryopreserved hematopoietic stem cells in a patient with severe primary cardiac amyloidosis and end-stage renal failure. Ann. Hematol. 79, 523–526. doi:10.1007/s002770000186
Zhan, L., Han, Z., Shao, Q., Etheridge, M. L., Hays, T., and Bischof, J. C. (2022). Rapid joule heating improves vitrification based cryopreservation. Nat. Commun. 13, 44. doi:10.1016/j.cryobiol.2022.11.142
Keywords: cryopreservation, soft matter, cryoprotectant, characterization techniques, chemical interactions
Citation: Bryant SJ, Elbourne A, Greaves TL and Bryant G (2023) Applying soft matter techniques to solve challenges in cryopreservation. Front. Soft. Matter 3:1219497. doi: 10.3389/frsfm.2023.1219497
Received: 09 May 2023; Accepted: 20 June 2023;
Published: 13 July 2023.
Edited by:
Tommy Nylander, Lund University, SwedenReviewed by:
Marcus K. Dymond, University of Brighton, United KingdomCopyright © 2023 Bryant, Elbourne, Greaves and Bryant. This is an open-access article distributed under the terms of the Creative Commons Attribution License (CC BY). The use, distribution or reproduction in other forums is permitted, provided the original author(s) and the copyright owner(s) are credited and that the original publication in this journal is cited, in accordance with accepted academic practice. No use, distribution or reproduction is permitted which does not comply with these terms.
*Correspondence: Saffron J. Bryant, c2FmZnJvbi5icnlhbnRAcm1pdC5lZHUuYXU=; Gary Bryant, Z2FyeS5icnlhbnRAcm1pdC5lZHUuYXU=
Disclaimer: All claims expressed in this article are solely those of the authors and do not necessarily represent those of their affiliated organizations, or those of the publisher, the editors and the reviewers. Any product that may be evaluated in this article or claim that may be made by its manufacturer is not guaranteed or endorsed by the publisher.
Research integrity at Frontiers
Learn more about the work of our research integrity team to safeguard the quality of each article we publish.