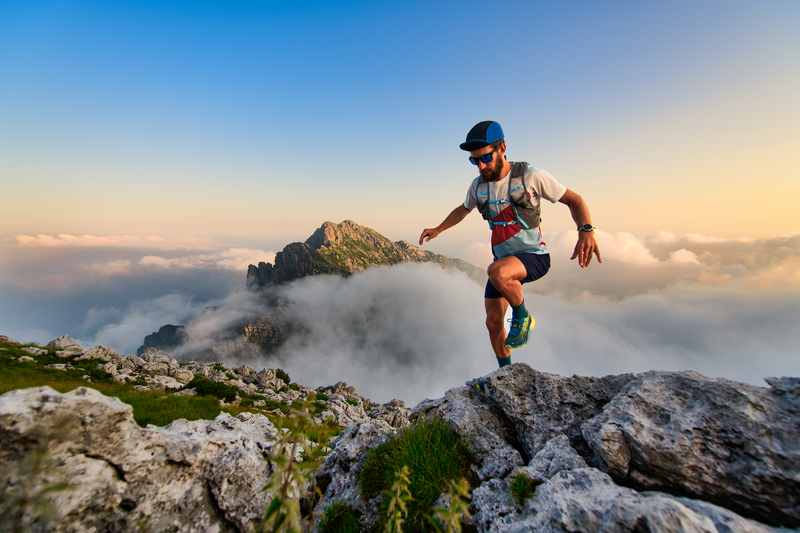
94% of researchers rate our articles as excellent or good
Learn more about the work of our research integrity team to safeguard the quality of each article we publish.
Find out more
ORIGINAL RESEARCH article
Front. Food. Sci. Technol. , 03 February 2025
Sec. Food Safety and Quality Control
Volume 4 - 2024 | https://doi.org/10.3389/frfst.2024.1490547
This article is part of the Research Topic Fermentation of Foods and Beverages: Current Insights and Perspectives View all articles
This study investigated the use of native microbiota from the sugar kelp Saccharina latissima in the form of fermented kelp fluid (FKF) from a spontaneous anaerobic fermentation process as a starter culture (SC) for the fermentation of freshly harvested biomass of the same species. Rapid (<48 h) acidification (pH < 4.3) was achieved for S. latissima inoculated with FKF, at a fermentation temperature of 21°C. Kelp inoculated with a commercial strain of Lactiplantibacillus plantarum (Lp, positive control) reached a similar pH level after 5 days, while kelp with no SC (negative control) did not reach a pH level below 4.3 within 9 days. The microbiota of the FKF-SC as well as the FKF-inoculated S. latissima samples was dominated by lactic acid bacteria (LAB) identified as L. plantarum. The SC in these samples successfully converted mannitol into lactic acid as the main fermentation product. In contrast, a higher production of acetic acid and ethanol was measured in the negative control samples than in other groups; this reflects a different microbial profile, including marine bacteria which could not be identified by MALDI-TOF biotyping. Challenge trials of S. latissima samples from experimental and commercial fermentation processes with Bacillus cereus did not result in the growth of this food pathogen, even at pH levels within a viable range for this species (pH > 4.3). These preliminary results provide a foundation for further isolation of suitable SCs for kelp fermentation in commercial production and for assessing the food safety of fermented kelp. Efficient and safe fermentation processes will increase sustainability in kelp production and enable a broader use of kelp ingredients in food applications.
Macroalgae are considered a promising alternative resource for the provision of food and feed ingredients as well as a relevant raw material in multiple industrial applications (Philis et al., 2018; Rotter et al., 2020; Blikra et al., 2021). The commercial cultivation of macroalgae, mainly the kelp species Saccharina latissima (sugar kelp) and Alaria esculenta (winged kelp), is expanding in Europe (Stévant et al., 2017). However, large-scale kelp cultivation entails technical challenges associated with the handling, processing, and storage of large volumes of biomass. Saccharina latissima spoils within 9 days post-harvest at refrigerated temperature due to a high-water content (Wirenfeldt et al., 2022). Therefore, efficient methods must be applied to stabilize the raw material. Freezing and low-temperature drying are commonly applied techniques, providing high-quality and versatile products with a long shelf-life when properly stored (Stévant and Rebours, 2021). These methods are also energy-intensive, lowering the profitability and environmental sustainability of the value-chain and limiting their applicability in the processing of large amounts of biomass (Philis et al., 2018; Thomas et al., 2020). Addressing these technical challenges with a focus on sustainability is crucial for the development of a thriving and environmentally responsible kelp cultivation industry in Europe. In this context, fermentation has been identified as a sustainable preservation method of kelp biomass (Stévant and Rebours, 2021; Thomas et al., 2020; Emblemsvåg et al., 2020; Sørensen et al., 2021).
Lactic acid bacteria (LAB)-induced fermentation is an interesting alternative for the stabilization of harvested kelp due to low requirements for energy and advanced equipment and is gaining popularity among kelp producers (Stévant and Rebours, 2021). LAB fermentation techniques under anaerobic conditions (also referred to as “ensiling”) are preservation methods commonly applied to food and forage in agriculture. Successful fermentation lowers the pH (typically below 4.0) to inhibit the growth of spoilage microorganisms (e.g., clostridia and molds). Low pH may be achieved directly by the addition of an organic acid or indirectly by inoculating the biomass with a starter culture (SC) of LAB which will then convert fermentable sugars into lactic acid. The LAB-induced fermentation of macroalgae is a recent strategy, and optimized protocols including adapted SCs for stabilizing commercially relevant species (e.g., S. latissima and A. esculenta) are not yet established. Macroalgae are rich in polysaccharides like alginate, carrageenan, and agar, which are structurally complex and not readily fermentable by common LAB. This is opposed to well-established fermentation techniques based on vegetables containing fermentable glucose, sucrose, and fructose, while dairy products contain lactose, which can be easily fermented by LAB. In addition, vegetables naturally harbor LAB, and dairy environments are conducive to LAB growth (e.g., Lactobacillus spp. and Lactococcus spp.). Native microbiota from macroalgae can adapt to marine conditions and may outcompete desirable fermentative microbes, potentially producing off-flavors and toxins.
The chemical composition of macroalgae featuring low dry-matter content and high buffering capacity related to high anion levels (e.g., chloride, sulfate) appears to be a challenge for successful fermentation, with variable results across species (Herrmann et al., 2015; Cabrita et al., 2017). The kelp S. latissima has shown better fermentation potential in anaerobic conditions compared to other species and produced lactic acid from native LAB populations (Herrmann et al., 2015). The spontaneous anaerobic fermentation of S. latissima (i.e., without the addition of SC) has also been reported in later studies (Sørensen et al., 2021; Stévant, 2019; Krook et al., 2024). However, due to the variability in inherent microbiota resulting in limited control of the fermentation process and unpredictable quality of the fermented biomass (e.g., development of spoilage-causing microorganisms and inconsistent sensory profiles), the implementation of this technique at a commercial scale remains limited. Other challenges for predictable kelp fermentation include the variability in the content of water-soluble polysaccharides and polyols (laminaran and mannitol in kelps) as substrates for LAB-induced fermentation, low initial LAB numbers, and sub-optimal SCs to the feedstock. Additional steps such as enzymatic saccharification (Uchida and Miyoshi, 2013), heat treatment (Bruhn et al., 2019), or the addition of fermentable sugars (e.g., molasses) (Larsen et al., 2021) may promote rapid acidification but also add complexity which can limit the applicability of the process to large scale commercial production.
Currently available SCs for kelp fermentation rely on commercial strains from agriculture, and knowledge about marine LAB for the fermentation of macroalgae is lacking (Uchida and Miyoshi, 2013; Uchida et al., 2004). Due to their adaptability to the kelp’s specific environment such as high salinity and mannitol as the main carbon source, native microbiota may provide a superior alternative to existing commercial LAB strains for kelp fermentation. The main objective of this study was to investigate whether native microbiota (presumably LAB) from S. latissima can be used as SC for further kelp fermentation. This native microbiota was selected from the spontaneous fermentation of cultivated S. latissima in anaerobic conditions, with the fluid resulting from this process, referred to as “fermented kelp fluid” (FKF), being used as SC. The fermentation of S. latissima using this method was compared to a similar process using the conventional SC Lactiplantibacillus plantarum (Lp, positive control) or No SC (i.e., spontaneous fermentation, negative control). The pH of the samples was monitored throughout the process, and the microbiota of fermented and fresh kelp as well as the FKF-SC was characterized. Safety aspects of the fermented S. latissima were also addressed.
Pathogenic Bacillus spp., Vibrio spp., and Aeromonas spp. have been identified as the main inherent bacteria of special concern for the food safety of macroalgae, whereas several other bacterial species, including Escherichia coli, Salmonella spp., Staphylococcus aureus, and Listeria monocytogenes, are considered potential food safety concerns by virtue of recontamination during processing. These bacteria are effectively inactivated by lowering the pH to below 4.3 upon storage at refrigerated temperatures, or to below pH 3.7 upon storage at ambient temperatures (Løvdal et al., 2021). Food pathogens from the Bacillus genus are of particular interest since they can produce spores that allow the bacteria to remain dormant in extreme environments and may germinate, develop, and produce toxins following the onset of favorable conditions. Toxin-producing Bacillus spp., including B. cereus, B. pumilus, and B. licheniformis, have been identified at low concentrations on the surface of fresh and dried edible kelp (Blikra et al., 2018; Lytou et al., 2021; Martelli et al., 2021). The microbial food safety of fermented kelp toward these pathogens is currently not established. The second objective of this study was to perform challenge trials with B. cereus on S. latissima samples from experimental and commercial fermentation processes to assess the food safety of the kelp products toward the pathogen.
Saccharina latissima was cultivated at Tango Seaweed AS (Herøy, Norway; latitude 62.1903 °N, longitude 5.4473 °E) and harvested on 26 April 2022. The biomass consisting of fronds, stipes, and holdfasts was roughly chopped then transferred to 30-L fermentation buckets equipped with a fermentation lock. Three replicate buckets received 18.2 ± 1.1 kg of fresh S. latissima which was firmly pressed to minimize entrapped air-bubbles and headspace under the lid. The buckets were transported to the laboratory and stored at 21°C in the dark. After 15 days of storage under these conditions, they were opened and the liquid fraction was collected (i.e., the FKF produced during this spontaneous fermentation). Each bucket produced approximately 7 L of FKF. The FKF from one of the replicates was characterized by an unpleasant sour odor and was therefore discarded. The FKF from the two remaining replicates were homogenized to be used as SC for the fermentation of fresh kelp biomass. FKF buckets were taken out of storage at 21°C to room ambient temperature (ca. 15°C) approximately 2 h before the experiment’s start. The FKF-SC temperature at inoculation was assumed to be near 21°C. An assessment of the LAB density of FKF-SC after 12 days of storage from serial plating (3M Petrifilm Lactic Acid Bacteria Count Plates) failed due to overgrown LAB count plates, so the LAB density was unknown at the time of the inoculation of fresh kelp with FKF-SC. Serial plating was attempted again at the experiment’s start and revealed a LAB density of 6 log (CFU mL−1). The pH of the FKF-SC was 3.6.
A commercial strain of L. plantarum (PAL LACTIC LP 2233 VEG RM D10, Laboratoire Standa, France) was used as a positive control to ferment S. latissima based on previous successful results (Stévant, 2019). Freeze-dried bacteria were rehydrated in peptone water for 30 min prior to inoculation at 6 log (CFU g−1) fresh kelp, as recommended by the ferment manufacturer.
Biomass of S. latissima was cultivated at Tango Seaweed AS and harvested on 9 May 2022. Kelp blades still attached to the substrate ropes were transported to the laboratory within 2 h then transferred to tanks supplied with ambient seawater at 7.5°C.
The fermentation experiment comprised five treatments involving the addition of different SCs (FKF, commercial strain of L. plantarum, and no added SC) and different storage temperatures of the samples during the fermentation (10°C and 21°C). These temperatures were selected according to optimal LAB growth (21°C) and realistic ambient kelp processing conditions in Norway in May (10°C). For each replicate, 3 kg of S. latissima was taken from the tank, roughly chopped, and left to drip for 5 min. The biomass was then thoroughly mixed with a SC in a large tub disinfected beforehand and split into polyethylene vacuum bags of ca. 500 g. Vacuum bags were stored in the dark either at 21°C in a controlled-temperature room or at 10°C in an incubator. The weight of each bag was registered with 1-decimal precision. The experimental protocol employed during this study and sample handling is summarized in Figure 1. The fermentation treatments were conducted in three replicates. The details of each treatment are summarized in Table 1. The samples in individual vacuum bags were opened at days 1, 2, 5, and 9 after inoculation with SC to monitor of quality indicators during the process and for further characterization.
Figure 1. Experimental design to explore the potential of fermented kelp fluid (FKF) compared to Lactiplantibacillus plantarum (Lp) as the starter culture (SC) for the fermentation of Saccharina latissima. Red stars indicate that samples were opened, drained, and reconditioned (vacuum-packed) upon arrival at Nofima’s laboratory facilities. Red circles indicate the challenge of the samples with Bacillus cereus at day 19 after inoculation of the fresh S. latissima samples.
Table 1. Fermentation treatments performed on 3-kg batches of S. latissima. Each treatment was conducted in three replicates.
After opening, the content of each sample bag was transferred to a sieve placed over a beaker and allowed to drip for 5 min. The drip losses were calculated using Equation 1, where M0 is the mass of the initial sample and Mt is the mass of the solid fraction at a sampling time t.
The dry weight (DW) of the solid fraction of the samples was determined gravimetrically as the residue remaining after drying in a laboratory oven at 105°C for 24 h. Each DW measurement was performed in three replicates using randomly picked sample pieces. The pH of the liquid fraction of the samples was measured using a pH-probe (340 pH-meter equipped with a InLab Expert Pro-C pH electrode, Mettler Toledo, OH, United States).
The WSC and organic acid content of the initial biomass prior to fermentation and fermented samples was analyzed by high-performance liquid chromatography (HPLC) as per Sørensen et al. (2021). For each sample, a few grams of wet solid material were transferred to a zip bag and then stored frozen (−20°C) until the end of the experiment. One gram of thawed samples was added to 5 mL of sulfuric acid (H2SO4, 5 mM) and then stored overnight at 4°C, and a further 5 mL of H2SO4 at the same concentration was again added. The samples were mixed by inverting the tubes five times and were centrifuged at 2,795 g for 8 min. We filtered 1 mL of supernatant through a 0.22-µm polyethersulfone (PES) filter into HPLC vials. HPLC analysis was performed with an Aminex HPX-87H (Bio-Rad, Hercules, United States) column on an Ultimate 3000 HPLC (Dionex, Thermo Fischer Scientific, United States) system equipped with a Shodex RI-101 refractive index detector (Showa Denko K.K., Tokyo, Japan) and UV Diode Array Detector (Thermo Fischer Scientific, United States). H2SO4 (5 mM) was used as the mobile phase. The column oven temperature was set to 60°C, and a flow rate of 0.5 mL min−1 was used for all samples. Standards for each HPLC-grade carbohydrate and primary organic metabolites (glucose, mannitol, fucose, lactic acid, acetic acid, and ethanol) were prepared in concentrations of 50, 100, 500, and 1,000 μg mL−1 in 5 mM H2SO4 and filtered through a 0.22 PES-filter. The results were analyzed using Chromeleon 6.0 software (Thermo Fischer Scientific, MA, United States).
The microbiota of the FKF-SC, the fresh S. latissima biomass prior to SC inoculation, and fermented samples after 9 days was investigated by Matrix-assisted laser desorption/ionization time-of-flight mass spectrometry (MALDI-TOF MS) biotyping, according to a protocol established at the Technical University of Denmark (Sedó Molina et al., 2022). To 0.5 mL glycerol (50%) in a cryovial was added 0.5 mL of liquid sample, which was then gently mixed and stored at −80°C prior to analysis. For fresh S. latissima samples prior to fermentation, a sterile swab was rolled in the chopped kelp fronds for 10 s and then transferred to 0.5 mL of 50% glycerol. The frozen glycerol stocks were streaked into the following four solid substrates: de Man, Rogosa and Sharpe (MRS) agar (Oxoid Ltd., United Kingdom) for LAB; Luria–Bertani (LB) agar (Sigma-Aldrich, MO, United States) as a non-defined nutrient substrate; yeast extract peptone dextrose (YPD) (Sigma-Aldrich, MO, United States); and marine agar (Condalab, Spain) for heterotrophic marine bacteria. Single colonies were re-streaked up to three times until purity and then fresh bacterial colonies were inoculated in the respective broth media without agar for 18 h at 30°C. For protein extraction (according to the manufacturer’s protocol), the overnight cultures were centrifuged at 3,500 × g (5 min at 4°C), washed twice with 0.9% saline water, and mixed with i) MilliQ water and ii) 96% ethanol before another centrifugation step to remove interfering substances. Following this, cell pellets were mixed with 70% formic acid and pure acetonitrile followed by vortexing, and they were centrifuged at 14,000 g for 3 min. We dispersed 1 µL of the supernatant onto the reusable steel MALDI-TOF target plate (Bruker Corp., MA, United States) in duplicates and left it to dry before adding the saturated matrix solution containing α-cyano-4-hydroxycinnamic acid (Trifluoroacetic acid:MilliQ water:Acetonitrile 1:19:20). The plate was loaded into a MALDI-TOF Biotyper Sirius (Bruker Corp., MA, United States), and the sample spectra were compared against bacterial (BDAL) and fungal (FFL) spectral libraries from Bruker Corp.
Three replicate samples from each treatment were sent overnight to Nofima’s laboratory (Stavanger, Norway) at day 12 after SC inoculation of the fresh S. latissima biomass. The samples were transported and stored under refrigeration (4°C). The vacuum pouches were opened at day 19 after SC inoculation, and the content was then drained, portioned into 25 g aliquots, repackaged under vacuum in sous vide pouches, and stored at 4°C until further analysis (Figure 1). Sampling for pH and microbial analysis was conducted at days 19, 35, 41, and 49 after SC inoculation.
Subsamples of 10 g were placed in Stomacher 400 bags with filters (Seward Ltd., United Kingdom) and diluted ten times in sterile buffered peptone water (Merck, Germany) and homogenized for 120 s in a Smasher® stomacher (bioMérieux, France). Homogenates were further diluted to appropriate concentrations in sterile water before plating. Total aerobic plate count (TAPC) was performed by plating on tryptic soy agar with 0.6% yeast extract (TSAYE) (Oxoid AS, Norway) and incubated at 25°C for 48 h prior to counting the colonies and calculating the concentrations expressed as colony-forming units (CFUs) per gram of sample. To enumerate LABs, homogenates were plated on MRS agar supplemented with amphotericin (Oxoid AS, Norway) and incubated anaerobically at 25°C for 48 h according to Nordic-Baltic Committee on Food Analysis (NMKL:140, 2023). Yeasts and molds were enumerated by plating on Dicloran Rose Bengal (DRBC) agar (Oxoid AS, Norway) and incubating at 25°C for 5–7 days according to NMKL:98 (NMKL:98, 2005). The presence of aerobe and anaerobe spore-formers was analyzed by heating aliquots of the homogenates at 80°C for 10 min prior to plating on blood agar. Duplicate plates were incubated aerobically and anaerobically, respectively, for 24 and 48 h at 37°C (NMKL:189, 2011). Coliforms were examined by plating 0.1 mL and 1 mL of sample homogenate on Neogen Petrifilm® Coliform Count Plates (Nerliens Meszansky, Norway) according to the manufacturer’s instructions (Blikra et al., 2018). Listeria monocytogenes was analyzed after enrichment in half and full Fraser broth (Oxoid AS, Norway) and confirmation by plating on Brilliance Listeria agar (Oxoid AS, Norway) according to NMKL:136 (2010). Pathogenic Vibrio species were analyzed according to NMKL:156 (1997) by plating on Thiosulfate–citrate–bile salt–sucrose (TCBS) agar (Oxoid AS, Norway) and incubating at 37°C for 24 h.
A challenge study was performed in the two samples with the highest pH at 19 days after inoculation of the kelp samples, i.e., the control with no added SC (No-SC; pH 4.38 ± 0.06) and the FKF-added samples stored at 10°C (pH 4.34 ± 0.04) (Figure 1). Bacillus cereus (strain NVH 1230-88), a cold-tolerant strain isolated from an oriental stew, involved in food poisoning (Ehling-Schulz et al., 2005); these were grown overnight in TSBYE at 37°C and shaking (225 rpm). The resulting culture was diluted in phosphate-buffered saline (PBS), and 1 mL of the diluted culture was inoculated in 10 g of S. latissima samples in plastic pouches, resulting in a final concentration of approximately 3 log (CFU g−1). The plastic pouches were vacuum sealed and stored at 4°C. Sampling for B. cereus was performed at days 0, 7, 21, and 30 post inoculation with the pathogen. The content of the pouches was transferred to a stomacher bag with a filter and diluted ten times in buffered peptone water (BPW) and homogenized for 120 s, as described above. Appropriate dilutions of the homogenates were plated on blood agar plates (Oxoid AS, Norway) and incubated aerobically at 30°C for 24 h (NMKL:67, 2021).
Saccharina latissima cultivated by Arctic Seaweed AS (Værlandet, Norway; latitude 61.3001 °N, longitude 4.7485 °E) and harvested in May 2023 was chopped into pieces approx. 2 × 2 cm, and 100 g was filled in 24 sous vide pouches. Molasses (93.8 g, Champion Melasse, Felleskjøpet, Norway) was dissolved in 375 mL of sea water previously filtered through a 47-mm polycarbonate filter (Poretics™) with a pore size of 0.45 µm (Thermo Fisher Scientific, MA, United States) and 0.94 g of a commercial freeze-dried SC of Latilactobacillus sakei was added (SafePro Flora Ctrl 01, Chr. Hansen, Denmark). To S. latissima in the sous vide pouches was added 10 mL of this solution. Overnight cultures of B. cereus NVH 1230-88 (Ehling-Schulz et al., 2005) and B. cereus ATCC 9139 (López-Pedemonte et al., 2003), which are both able to grow at temperatures <10°C, were prepared as described above. We pelleted 1 mL of each strain by centrifugation and resuspended it in 1 mL PBS and pooled it. The B. cereus suspension was further diluted in autoclaved MilliQ water, and 1 mL was inoculated in 15 of the sous vide pouches to give a final B. cereus concentration of approximately 4 log (CFU g−1). In the remaining nine sous vide pouches (controls) was added 1 mL of autoclaved MilliQ water. Three inoculated and three control pouches were sampled immediately for pH, B. cereus, LAB, and TAPC. The remaining pouches were vacuum-sealed, and the inoculated pouches were stored at 10 or 15°C, respectively. The rest of the control pouches were stored at 10°C. Each treatment condition (i.e., B. cereus inoculation and storage temperature) was replicated thrice. Samples from each treatment group were withdrawn after 48 and 72 h storage and analyzed. We measured pH in the liquid phase using a FiveEasy Plus pH-meter with a LE410 probe (Mettler Toledo, OH, United States). Subsamples of 10 g were diluted ten times in BPW, homogenized, and B. cereus, TAPC, and LAB were enumerated as described above.
The statistical analysis of the results was performed using R [version 4.2.2 (R Development Core Team, 2022)]. The results from the analysis of replicate samples were described as mean ± standard deviation. The differences in evolution of pH, WSCs, organic acids and drip loss across treatment conditions (SC and storage temperature) were analyzed by repeated measures analysis of variance [RM ANOVA, R function lmer () from the lme4 package (Bates et al., 2015)], considering samples from replicate fermentation treatments as random factor. Post-hoc pairwise comparisons of least square means [R function lsmeans () from the emmeans package (Lenth, 2016)] were performed following significant RM ANOVA results. In cases of non-normal distribution of the data and heterogeneity of variances, the results were analyzed by aligned rank transform (ART) ANOVA [R function art () from the ARTool package (Kay et al., 2021)] which is a non-parametric approach to factorial ANOVA. Post-hoc pairwise comparisons were performed using the art.con () function.
The liquid fraction resulting from the spontaneous fermentation of a previous batch of cultivated S. latissima (i.e., FKF-SC) was tested as inoculum for the fermentation of freshly harvested biomass. The efficiency of the resulting fermentation process at 21°C, characterized by pH decrease, was compared to the fermentation of S. latissima under the same conditions using Lp as SC, No-SC and the combined inoculation of kelp biomass with FKF and Lp (FKF + Lp). The efficiency of the FKF at low temperature (10°C) was also explored. The tested conditions (i.e., SC and temperature) significantly affected pH changes across the experiment (RM ANOVA, F4, 10 = 18.38, p < 0.001). The pH of all test groups was significantly lower than the negative control (No-SC) throughout the experiment, except the FKF-added group stored at 10°C (Figure 2). Both FKF- and FKF + Lp-inoculated samples stored at 21°C reached a pH below 4.3 (i.e. 4.1 ± 0.0 and 4.0 ± 0.2 respectively) within 2 days. Samples inoculated with only Lp reached a similarly low pH (3.9 ± 0.1) after 5 days, whereas the average pH of the negative control and FKF-added samples stored at 21°C were 4.4 ± 0.5 and 4.3 ± 0.2, respectively, after 10 days.
Figure 2. pH evolution during the fermentation of S. latissima samples, following the addition of different SCs. Values are given as mean ± st. dev. (n = 3). The dashed red line represents the safety pH threshold of 4.3.
The results from the sugar and organic acid profile of the samples during the experiment show higher levels of lactic acid in inoculated samples with FKF and/or Lp stored at 21°C compared to the negative control and FKF-inoculated samples stored at 10°C (LSmeans, p < 0.009) (Figure 3). The increasing levels of lactic acid in the samples mirrored the decrease in mannitol in all samples (not-significant difference between treatments, RM ANOVA F4, 40 = 2.60, p = 0.50). The initial contents of glucose and fucose were low—0.43 ± 0.06 and 0.30 ± 0.03 g (100 g)−1 DW respectively—compared to mannitol 24.1 ± 7.4 g (100 g)−1 DW. Small amounts of acetic acid and ethanol were detected in all samples. In each group, the levels of acetic acid and ethanol did not significantly differ between samples taken at 1, 2, 5, and 9 days except in No-SC samples, in which significantly higher ethanol levels were measured after 9 days of fermentation (LSmeans, p < 0.001). The relatively high standard deviations observed within samples may reflect variable bacterial equilibria among treatment replicates during experimental fermentation. Analytical sample preparation is likely an additional source of variation.
Figure 3. Evolution of water-soluble carbohydrates, organic acids, and ethanol content of S. latissima samples during fermentation, following the addition of different SCs. Values are given as mean ± st. dev. (n = 3).
The drip loss (i.e., effluent formation) measured across treatment groups after 1 day were in a range between 18% and 31%, then at 31% to 37% at 10 days (Figure 4). Despite relatively high variations measured across sample replicates, there was a trend of higher drip losses in FKF + Lp samples and lower in No-SC samples across the experiment. Personal observations while handling the samples revealed a slimier texture of FKF-inoculated samples (both 10°C- and 21°C-stored samples) compared to other sample groups.
Figure 4. Drip loss during the fermentation of S. latissima, following the addition of different SCs. Values are given as mean ± st. dev. (n = 3).
The microbiota of raw (unfermented) S. latissima, FKF-SC (i.e., the fluid used as SC), and fermented biomass was characterized by MALDI-TOF MS. The microbiota of fresh kelp was dominated by marine bacteria which could not be identified by MALDI-TOF Biotyper (Figure 5). No colonies were isolated on MRS culture medium from these samples whereas some were isolated but not identified in fermented control samples (No-SC). In many cases, the identification of marine agar isolates failed (due to low score values), with the exception of Lelliotia amnigena and Pseudomonas luteola identified in the No-SC samples. The microbiota composition of inoculated samples and FKF-SC were relatively similar to each other and dominated by lactobacilli. No marine bacteria were isolated from these samples. In the case of LB agar, which is a non-selective medium that supports the rapid growth of a high yield of bacterial cells, most colonies were not identified within an acceptable score value. The highest number of colonies from fermented kelp was obtained from FKF-inoculated samples stored at 21°C and the lowest were measured from similar treatment but stored at 10°C. Isolates from purified colonies on MRS agar were successfully identified as L. plantarum, both in FKF-SC as well as FKF- and Lp-inoculated samples. Yeasts were isolated from all samples and successfully identified in many cases.
Figure 5. Number of bacteria and yeast colonies (mean counts from replicate samples, n = 3) isolated using four different solid substrates from kelp samples: fresh S. latissima (SL) before inoculation, fermented kelp fluid starter culture (FKF-SC), and fermented S. latissima using FKF and/or Lactiplantibacillus plantarum (Lp) or no starter culture (No-SC), stored at 10 or 21°C for 9 days (top plot). These colonies underwent identification based on MALDI-TOF scores (bottom plot). Abbreviations: YPD, yeast extract peptone dextrose; LB, Luria–Bertani agar; MRS, de Man, Rogosa and Sharpe agar.
At day 12 of the fermentation process (i.e., SC inoculation of the fresh kelp), the samples were drained, then re-sealed under vacuum and transferred from their respective storage temperatures (10 or 21°C) to refrigerated temperature (4°C, Figure 1) to assess their microbial stability. The bacterial load of the samples was analyzed until day 49 of the experiment. TAPC reflects the total bacterial load including LAB (since most LABs are facultative anaerobes), yeasts, and molds. The main effect of sample treatment on TAPC was significant across the sampling period (ART ANOVA, F4, 10 = 3.54, p = 0.048), although pairwise comparisons revealed significant differences only between No-SC and FKF + Lp samples (ART contrast, p = 0.048). TAPCs were relatively high [ranging from 7.3 to 7.7 log (CFU g−1) at day 19] in fermented samples inoculated with a SC (FKF and/or Lp) and stable throughout the refrigerated storage period (Figure 6). The No-SC samples had lower initial counts [5.9 ± 1.6 log (CFU g−1)] until day 49, when counts were comparable to other treatment groups. In all samples the microbial flora was dominated by LABs which followed the same pattern as TAPCs across refrigerated storage in all sample groups. A significant effect of treatment on counts of yeasts and molds was also observed (ART ANOVA, F4, 10 = 4.78, p = 0.020). Levels of yeasts and molds varied from 2.7 ± 0.9 log (CFU g−1) measured in Lp-inoculated samples to 4.4 ± 0.2 log (CFU g−1) in FKF samples fermented at 21°C. Consistently higher yeast and mold counts were obtained from FKF-inoculated samples (fermented at 10°C and 21°C) during storage compared to the other groups.
Figure 6. Plate counts [log (CFU g−1)] of total aerobic bacteria (TAPC), lactic acid bacteria (LAB), and yeasts and molds during refrigerated (4°C) storage of fermented S. latissima samples, following the addition of different SCs. Refrigeration of the samples started at day 12 after inoculation of kelp samples with SCs. Prior to day 12, the samples were either stored at 10°C or 21°C. Values are given as mean ± st. dev. (n = 3).
Aerobe spore-formers were occasionally above the detection limit of 2 log (CFU g−1) in fermented S. latissima. On day 19, aerobe spore-formers were detected in all three replicates of No-SC samples, two of three replicates of FKF + Lp samples [2.2 ± 0.4 log (CFU g−1)], and 1 of 3 replicates in the remaining treatments (Figure 7). Aerobe spore-formers were detected at day 35 in FKF + Lp- and Lp-inoculated samples and at day 49 in No-SC and Lp samples. Anaerobe spore-formers were detected above 2 log (CFU g−1) on three occasions (data not shown): in one of three replicates of FKF + Lp samples at day 35 [plate overgrown and estimated to be above 4 log (CFU g−1)], in two of three replicates of No-SC kelp samples whereof one parallel was overgrown [>4 log (CFU g−1)], and the other was 2.6 log (CFU g−1). Listeria monocytogenes, Vibrio spp., and coliforms were not detected in any samples.
Figure 7. Microbial counts [log (CFU g−1)] of aerobe spore-formers during refrigerated (4°C) storage of fermented S. latissima samples, following the addition of different starter cultures (SCs). The dashed red line represents the detection limit 2 log (CFU g−1).
Challenge trials with B. cereus were conducted on experimentally fermented S. latissima using FKF as inoculum (stored at 10°C) and the No-SC control samples (Figure 1) at day 19. The measured pH of these samples at inoculation with the pathogen was 4.3 and 4.4 in the two sample groups, respectively. No growth of B. cereus was observed in both groups after inoculation of the pathogen and refrigerated storage at 4°C for 30 days. Another challenge trial with the same and an additional strain of B. cereus was performed on commercially fermented S. latissima at the time of inoculation with the SC (L. sakei). The inoculated B. cereus did not grow on fermented kelp stored at 10°C and 15°C despite relatively high pH levels measured in these samples compared to the threshold value of 4.3 for the growth of this foodborne pathogen (Figure 8). Only the control samples of S. latissima inoculated with L. sakei but not B. cereus reached a pH below this threshold within 3 days. In all samples, the microbial flora were dominated by LAB. The microbial load (TAPC) of control samples was superior to those of fermented S. latissima challenged with B. cereus, although only significantly higher compared to challenged samples stored at 15°C (ART contrast, p = 0.035).
Figure 8. pH (pH units) and microbial load [log (CFU g−1)] including Bacillus cereus, TAPC, and LAB of commercially fermented samples of S. latissima, following the addition of B. cereus (Bc) at inoculation with the starter culture and subsequent storage at 10°C and 15°C. Control samples were not inoculated with B. cereus. Values are given as mean ± st. dev. (n = 3). The dashed red line represents the safety pH threshold of 4.3.
The present study investigated the use of S. latissima’s native microbial flora, in the form of FKF, as a SC for the fermentation of freshly harvested biomass. A pH below 4.3 was achieved in 2 days both in kelp samples inoculated with FKF and those receiving a mixture of FKF and a commercial culture of L. plantarum stored at 21°C. A similar pH level was achieved after 5 days in S. latissima inoculated with L. plantarum only, whereas the spontaneous fermentation (No-SC) resulted in pH levels slightly above 4.3. The acidity of the FKF-SC (pH 3.6) may partly explain the rapid pH drop in this treatment group. However, a slower drop was clearly observed in S. latissima inoculated with FKF and stored at 10°C, indicating that acid production after inoculation is largely responsible for the rapid decrease in pH in FKF-added samples stored at 21°C. This was confirmed by the HPLC analysis of the samples revealing increasing levels of organic acids, mainly lactic acid and highest in kelp inoculated with FKF and/or L. plantarum stored at 21°C. The increase in lactic acid mirrored the decrease in mannitol in solid samples, suggesting that mannitol is utilized as a carbon source by the fermenting microbiota. The initial content of other WSCs, i.e., glucose and fucose, was low and did not follow a similarly decreasing trend, suggesting that mannitol is the main source of carbon for the fermentation of S. latissima, supporting similar conclusions on the anaerobic fermentation of kelp (Stévant, 2019; Krook et al., 2024; Horn and Østgaard, 2001; Hwang et al., 2011; Sandbakken et al., 2018). Minor production of acetic acid and ethanol was detected in all samples, although higher in control samples with no added SC. These fermentation products likely result from the presence of heterofermentative bacteria and yeasts in these samples, as reported earlier from the spontaneous anaerobic fermentation of S. latissima (Herrmann et al., 2015; Stévant, 2019; Sandbakken et al., 2018) and A. esculenta (Sørensen et al., 2021). The slower and lesser acidification of FKF-added kelp stored at 10°C is likely due to a sub-optimal temperature for the microbiota selected during the production of the FKF-SC at 21°C. The acidification of S. latissima to pH 4.4 upon spontaneous fermentation at 10°C based on the same protocol used in this study to prepare the FKF-SC has been observed (P. Stévant, pers. observation). This process may promote the growth of microbes that are able to ferment kelp biomass at low temperatures and should be investigated in future screening.
Results from this study and the literature (Sørensen et al., 2021; Herrmann et al., 2015; Stévant, 2019; Sandbakken et al., 2018) report a significant pH drop following the fermentation of S. latissima without the addition of SC, often stabilizing at pH levels between 4.0 and 4.5 after a relatively long time (>10 days). However, the quality of the resulting fermented product is somehow unpredictable and may allow the development of pathogenic microorganisms such as molds (Stévant, 2019). This was exemplified in this study by the occurrence of an unpleasant vomit-like odor, characteristic of butyric acid produced by Clostridium sp. in 1 of 3 replicates during the preparation of the FKF-SC (see Section 2.1.1). Although no butyric acid was detected in any of the samples (including No-SC) during experimental fermentation, suggesting that clostridial growth and activity was inhibited, spontaneous fermentation is not a reliable method for the large-scale preservation of kelp biomass to food applications. This may be due to the variability in the content of fermentable sugars across batches of S. latissima, high buffering capacity and low number of LAB initially present on the biomass. Optimal SCs will promote the rapid acidification of the kelp and outcompete the growth of pathogens potentially present. Rapid acidification of fermented foods (e.g., kimchi and sauerkraut) is crucial to inhibit the growth of pathogens early in the process and create a selective environment favoring acid-tolerant LAB, which will dominate the fermentation and prevent further spoilage by unknown microorganisms (Jung et al., 2011; Zabat et al., 2018). A rapid pH decrease may also prevent enzymatic and microbial degradations, potentially resulting in off-flavors and altered nutritional content (e.g., vitamins and antioxidants).
The preliminary characterization of the samples by MALDI-TOF MS revealed that the FKF-SC used for the fermentation of freshly harvested S. latissima was dominated by LAB identified as L. plantarum. This is opposed to the microbial flora of the fresh unfermented S. latissima (of the same origin as the biomass used to produce the FKF-SC but harvested a few weeks later) dominated by marine bacteria and some yeasts whereas no LAB were detected. LAB may be naturally present on freshly harvested S. latissima but too low in number to be detected. These bacteria may outcompete other microorganisms and grow following the onset of anaerobic conditions (as in the FKF-SC). However, L. plantarum is one of the most ubiquitous LAB species, and its dominance in FKF-SC samples may also arise from contamination upon harvest and handling of the biomass at the commercial production site. In contrast, LAB were not identified in spontaneously fermented (No-SC) control samples. Among bacterial isolates identified with secure genus identification (score value >2.0), opportunistic bacilli of the Pseudomonas and Lelliotia genera were detected from these samples. This differs from microbial flora of spontaneously fermented S. latissima reported by Sørensen et al. (2021) which consisted primarily of Psychromonas, Marinomonas, and Aliivibrio.
The protocol employed for sample preparation included the lysis and subsequent analysis of the lysates which offers a more reliable and consistent method for bacterial identification compared to the use of whole cells with direct spreading of bacterial colonies from solid substrates (Böhme et al., 2010; Emami et al., 2012). A substantial part of the microbiota of the samples, especially marine bacteria, could not be identified by MALDI-TOF MS due to missing information about marine microbiota in the database. Specifically, the low scores obtained for this fraction of isolated colonies could only give insights into the genus level. However, some genera, such as Aeromonas, exhibit high similarities in spectral patterns which limit the reliable identification among possible species candidates. Moreover, the principle of MALDI-TOF/spectral biotyping poses limitations when unsuitable reference databases are used, such as microorganisms of terrestrial origin, and this is a reported case for marine-associated bacteria (Emami et al., 2012). Although 16s rRNA amplicon sequencing was attempted for microbial characterization, the analysis could not be completed due to unforeseen sample mishandling during transport. The identification of microbiota from fermented kelp using high-throughput sequencing techniques is warranted in future studies.
Fermented foods normally contain five to seven log LAB per mL or g, whereas fermented dairy products may be as high as nine log (CFU g−1) (Rezac et al., 2018). The bacterial load of both experimentally and commercially fermented S. latissima in the present study, dominated by LAB, was within this range. The combined counts for yeasts and molds in experimentally fermented samples were below (in the case of No-SC, FKF + Lp and Lp samples) or in the lower range (FKF-inoculated samples stored at 10°C and 21°C) of yeast counts for typical fermented products [five to eight log (CFU g-1)] (Rezac et al., 2018). Neither L. monocytogenes, Vibrio spp., nor coliforms were detected in any of these samples.
Foodborne pathogens including Bacillus spp., L. monocytogenes, E. coli, and Salmonella spp. have been detected on fresh or dehydrated and ready-to-eat edible macroalgae, including kelp species (Blikra et al., 2018; Lytou et al., 2021; Martelli et al., 2021; Gupta et al., 2010; Barberi et al., 2020). These microorganisms may be present in the marine environment in which macroalgae are grown and harvested or may arise through contamination during post-harvest handling. Although in most cases these microbial pathogens were detected at low levels compared to infectious doses, measures must be taken to prevent their occurrence and growth during the harvest, processing, and storage of macroalgae to be used in food applications. Among microbial pathogens, toxin-producing spore-forming bacteria (Bacillus spp.) are of particular interest since their spores can withstand high temperatures (>100°C) and low pH (<3.0) for short periods. The spores present in the product may germinate upon viable conditions, reproduce, and eventually produce toxins, resulting in food poisoning. The concentration of B. cereus and other pathogenic Bacillus species required to produce toxins at levels capable of causing food poisoning is generally considered to exceed log 5 CFU g−1 (Kramer et al., 1989; Granum et al., 2000). When macroalgae are harvested in locations with satisfactory water quality, their concentrations of pathogenic Bacillus spp. are presumably low compared to the infectious dose threshold (Løvdal et al., 2021). However, it is essential to implement measures to limit their growth during handling and storage. In the present study, the initial inoculated B. cereus concentration of approx. log 3.5 CFU g−1 was not elevated over the course of 72 h at 10 or 15°C (Figure 8). This suggests that efficient fermentation and a sufficient pH drop can indeed be achieved at temperatures as low as 10°C and that the combination of low pH and competing microbiota effectively inhibits the growth of B. cereus under these conditions. Available guidelines and legislation concerning edible macroalgae are not specifically designed for fermented products but rather for dried and fresh products (CEVA, 2019). Based on known limits for the growth of food pathogens in relation to temperature, pH, water activity, and water-phase NaCl, Løvdal et al. (2021) recommended a pH below 4.3, where most pathogens of relevance for macroalgae, including Listeria spp, Bacillus spp., Clostridium spp., and Vibrio spp., are inactivated at refrigerated temperature (4°C). If the product is to be stored at room temperatures, the pH must be lowered to below 3.7 to prevent the growth of Salmonella spp., S. aureus, and pathogenic E. coli. At present, no guidelines have been issued on how fast the pH of fermented kelp must be lowered. A pH drop to below threshold within 48 h is currently considered an appropriate target for the successful fermentation of S. latissima by kelp producers. However, based on the present findings, there may be grounds for increasing this time limit to 72 h when fermentation is carried out at low temperatures. However, more studies and risk assessments are warranted for further recommendations.
The experimentally fermented samples of S. latissima showing the highest pH levels—FKF-inoculated samples stored at 10°C and No-SC control samples—were challenged with B. cereus at day 19 prior to storage under anaerobic conditions at 4°C. Despite pH levels near but not below the threshold in both groups (pH 4.3 and 4.4 respectively), no growth of the inoculated pathogen was observed. In this challenge study, the pathogen was inoculated late in the fermentation process when pH was significantly lower than the original levels measured in the fresh raw material (pH 7.4) simulating a cross-contamination of the fermented kelp upon processing. It should be noted that both anaerobe and aerobe heat-tolerant spore-formers were sporadically detected in all sample groups of experimentally fermented S. latissima. Although the detected colonies were not further identified, these observations warrant more occurrence data on the presence and behavior of pathogenic spore-formers in fermented kelp. Another challenge test using the same B. cereus strain and another responsible for food poisoning was conducted on commercial kelp samples at the beginning of the fermentation process, at inoculation with the SC (L. sakei). No growth of the pathogen was observed in the challenged samples both stored at 10°C and 15°C after 72 h, despite pH levels above threshold (pH 4.5 and 5.1 in the two temperature groups, respectively). This may be explained by the competition with the SC which prevented the growth of B. cereus. Moreover, challenging S. latissima with B. cereus delayed the pH reduction compared to control samples which were not exposed to the pathogen and reached pH 4.2 in less than 72 h. These results suggest that the acidification upon fermentation of S. latissima and the competition with other microorganisms (most likely L. sakei) prevented the growth of these strains of B. cereus responsible of food poisoning. Challenge tests should be repeated using a broad range of pathogenic Bacillus spp. strains along with other microbial pathogens to reach conclusions about the food safety of fermented S. latissima.
The successful fermentation of foods extends product shelf-life and improves food safety by preventing microbial spoilage. In the case of S. latissima, the process reduces iodine content (Krook et al., 2024; Bruhn et al., 2019) which may otherwise be excessive and limit the use of this species in large scale food applications. The controlled fermentation of kelp may also result in additional advantages, including increased levels of probiotic bacteria in the final product (Gupta and Abu-Ghannam, 2012), prebiotic function from the degradation of large indigestible polysaccharides (e.g., alginates) (O’Sullivan et al., 2010), and the development of desirable flavor characteristics (Bruhn et al., 2019). According to recent research, kelp fermentation has no or almost no effects on the dry matter content and content of minerals and crude proteins (Bruhn et al., 2019; Larsen et al., 2021). However protein degradation may occur (Larsen et al., 2021). Future research should investigate the impact of fermentation processes on the nutritional quality of kelp.
The rapid acidification of S. latissima to below 4.3 following inoculation with FKF-SC highlights the potential of the microbiota from spontaneously fermented kelp as SC for the low-energy preservation of cultivated biomass. The FKF-SC was dominated by LAB identified as L. plantarum, although it was unclear from this experiment whether this LAB strain was native or originated from a potential contamination following commercial harvest. Upon storage at 21°C, the microbiota of FKF-added S. latissima samples used mannitol as the main carbon source to produce lactic acid as the main fermentation product, resulting in the rapid acidification of the biomass. The screening and isolation of relevant SCs for commercial kelp fermentation should be pursued using sequencing-based methods. Successful and predictable fermentation of a biomaterial relies on microorganisms with known requirements and characteristics. One such characteristic is the utilization of carbohydrates. Although some strains can only ferment simple sugars, others may be able to metabolize more complex carbohydrates. Microbiota from spontaneously fermented kelp under various conditions in a controlled environment as well as other marine sources, such as the digestive tract of kelp grazers and naturally decaying kelp, may be explored as sources of SCs for kelp fermentation in future studies. Future research should not only focus on searching for and improving fermentation with natural isolates of L. plantarum but also on using adaptive laboratory evolution (Mavrommati et al., 2022) to further improve L. plantarum isolates from FKF for kelp fermentation.
Preliminary results from challenge studies of fermenting S. latissima with the toxin-producing spore-forming bacteria B. cereus showed no growth of the inoculated pathogen. This was observed even at pH levels above the threshold of 4.3, known to inhibit the growth and development of this species. Repeated challenge tests using multiple strains and microbial species are warranted in future studies. Adequate SCs which will outcompete any pathogenic microorganisms potentially present in low numbers for further development, combined with strict hygiene measures throughout harvest and processing steps will ensure the food safety of fermented kelp. In addition to food safety, future research should investigate the nutritional and sensory profile, as well as bioactive (e.g., prebiotic) potential, and evaluate the digestibility of fermented kelp products. LAB-induced fermentation is an energy-efficient alternative to conventional stabilization methods of kelps (e.g., air-drying, freezing) (Thomas et al., 2020) in line with current goals to improve the sustainability of food production systems. The demand for sustainable and healthy food products continues to grow, and kelp fermentation offers a promising avenue for low-energy stabilization of kelp biomass.
The datasets from this study, including raw data from pH measurements, carbohydrate and acid analyses, microbial counts, and the R script used for data analysis, are available in a public data repository: https://doi.org/10.5061/dryad.47d7wm3pk.
PS: conceptualization, data curation, formal analysis, funding acquisition, investigation, methodology, project administration, visualization, and writing–review and editing. EZ: investigation and writing–review and editing. JSS: investigation and writing–review and editing. CHB-B: investigation, writing–review and editing, and methodology. THD: investigation and writing–review and editing. TB: investigation and writing–review and editing. MK: investigation, methodology, and writing–review and editing. TL: investigation, methodology, writing–review and editing, and formal analysis.
The author(s) declare that financial support was received for the research, authorship, and/or publication of this article. This work was financed by the Research Council of Norway (SusKelpFood project, RCN grant number 326803), the Regional Research Fund (FermenTare project, RFF grant number 350640), and the Møre and Romsdal County Council (grant number 2021–0164). EZ received funding from a joint strategic alliance PhD grant from KTH and DTU.
The authors thank Annelise Chapman (Tango Seaweed AS) for providing kelp biomass and for her assistance in the preparation of the FKF-SC, as well as the production team at Ocean Forest AS for kindly providing kelp to the challenge trials. Leena Prabhu (Nofima) is thanked for technical assistance on microbiological analyses. They also thank the research infrastructure Open Innovation FOOD and Health Laboratory (FOODHAY) for contributing to the MALDI-TOF Biotyping equipment.
Author MK was employed by Lerøy Seafood Group ASA.
The remaining authors declare that the research was conducted in the absence of any commercial or financial relationships that could be construed as a potential conflict of interest.
All claims expressed in this article are solely those of the authors and do not necessarily represent those of their affiliated organizations, or those of the publisher, the editors and the reviewers. Any product that may be evaluated in this article, or claim that may be made by its manufacturer, is not guaranteed or endorsed by the publisher.
Barberi, O. N., Byron, C. J., Burkholder, K. M., Gelais, A. T. St, and Williams, A. K. (2020). Assessment of bacterial pathogens on edible macroalgae in coastal waters. J. Appl. Phycol. 32 (1), 683–696. doi:10.1007/s10811-019-01993-5
Bates, D., Mächler, M., Bolker, B., and Walker, S. (2015). Fitting linear mixed-effects models using lme4. J. Stat. Softw. 67 (1), 1–48. doi:10.18637/jss.v067.i01
Blikra, M. J., Altintzoglou, T., Løvdal, T., Rognså, G., Skipnes, D., Skåra, T., et al. (2021). Seaweed products for the future: using current tools to develop a sustainable food industry. Trends Food Sci. Technol. 118, 765–776. doi:10.1016/j.tifs.2021.11.002
Blikra, M. J., Løvdal, T., Vaka, M. R., Roiha, I. S., Lunestad, B. T., Lindseth, C., et al. (2018). Assessment of food quality and microbial safety of brown macroalgae (Alaria esculenta and Saccharina latissima). J. Sci. Food Agric. 99 (3), 1198–1206. doi:10.1002/jsfa.9289
Böhme, K., Fernández-No, I. C., Barros-Velázquez, J., Gallardo, J. M., Cañas, B., and Calo-Mata, P. (2010). Comparative analysis of protein extraction methods for the identification of seafood-borne pathogenic and spoilage bacteria by MALDI-TOF mass spectrometry. Anal. Methods-UK 2 (12), 1941–1947. doi:10.1039/c0ay00457j
Bruhn, A., Brynning, G., Johansen, A., Lindegaard, M. S., Sveigaard, H. H., Aarup, B., et al. (2019). Fermentation of sugar kelp (Saccharina latissima)—effects on sensory properties, and content of minerals and metals. J. Appl. Phycol. 31, 3175–3187. doi:10.1007/s10811-019-01827-4
Cabrita, A. R. J., Maia, M. R. G., Sousa-Pinto, I., and Fonseca, A. J. M. (2017). Ensilage of seaweeds from an integrated multi-trophic aquaculture system. Algal Res. 24 (Part A), 290–298. doi:10.1016/j.algal.2017.04.024
CEVA (2019). Réglementation algues alimentaires. Pleubian, France: Centre d'Étude et de Valorisation des Algues.
Ehling-Schulz, M., Svensson, B., Guinebretiere, M.-H., Lindbäck, T., Andersson, M., Schulz, A., et al. (2005). Emetic toxin formation of Bacillus cereus is restricted to a single evolutionary lineage of closely related strains. Microbiology 151 (1), 183–197. doi:10.1099/mic.0.27607-0
Emami, K., Askari, V., Ullrich, M., Mohinudeen, K., Anil, A. C., Khandeparker, L., et al. (2012). Characterization of bacteria in ballast water using MALDI-TOF mass spectrometry. Plos One 7 (6), e38515. doi:10.1371/journal.pone.0038515
Emblemsvåg, J., Kvadsheim, N. P., Halfdanarson, J., Koesling, M., Nystrand, B. T., Sunde, J., et al. (2020). Strategic considerations for establishing a large-scale seaweed industry based on fish feed application: a Norwegian case study. J. Appl. Phycol. 32 (6), 4159–4169. doi:10.1007/s10811-020-02234-w
Granum, P. E., and Braid-Parker, T. (2000). “Bacillus species,” in The microbiological safety and quality of food. 2. Editors B. Lund, T. Braid-Parker, and W. Gould (Gaithersburg, MD, USA: Aspen Publishers), 1029–1039.
Gupta, S., and Abu-Ghannam, N. (2012). Probiotic fermentation of plant based products: possibilities and opportunities. Crit. Rev. Food Sci. Nutr. 52 (2), 183–199. doi:10.1080/10408398.2010.499779
Gupta, S., Rajauria, G., and Abu-Ghannam, N. (2010). Study of the microbial diversity and antimicrobial properties of Irish edible brown seaweeds. Int. J. Food Sci. Tech. 45 (3), 482–489. doi:10.1111/j.1365-2621.2009.02149.x
Herrmann, C., FitzGerald, J., O’Shea, R., Xia, A., O’Kiely, P., and Murphy, J. D. (2015). Ensiling of seaweed for a seaweed biofuel industry. Bioresour. Technol. 196, 301–313. doi:10.1016/j.biortech.2015.07.098
Horn, S. J., and Østgaard, K. (2001). Alginate lyase activity and acidogenesis during fermentation of Laminaria hyperborea. J. Appl. Phycol. 13 (2), 143–152. doi:10.1023/A:1011187526918
Hwang, H., Lee, S., Kim, S., and Lee, S. (2011). Fermentation of seaweed sugars by Lactobacillus species and the potential of seaweed as a biomass feedstock. Bioprocess Eng. 16 (6), 1231–1239. doi:10.1007/s12257-011-0278-1
Jung, J. Y., Lee, S. H., Kim, J. M., Park, M. S., Bae, J. W., Hahn, Y., et al. (2011). Metagenomic analysis of kimchi, a traditional Korean fermented food. Appl. Environ. Microbiol. 77 (7), 2264–2274. doi:10.1128/AEM.02157-10
Kay, M., Elkin, L., Higgins, J., and Wobbrock, J. (2021). ARTool: aligned rank transform for nonparametric factorial ANOVAs. Available at: https://github.com/mjskay/ARTool.
Kramer, J., and Gilbert, R. (1989). “Bacillus cereus and other Bacillus species,” in Food borne bacterial pathogens. Editor M. Doyle (New York: Marcel Dekker Inc), 21–70.
Krook, J. L., Riboldi, L., Birkeland, I. M., Stévant, P., Larsen, W. E., Rhein-Knudsen, N., et al. (2024). Acid preservation of the brown seaweed Saccharina latissima for food applications. Algal Res. 80, 103524. doi:10.1016/j.algal.2024.103524
Larsen, S. U., Ma, N., Hou, X., Bruhn, A., Boderskov, T., MacLeod, A., et al. (2021). Ensiling of sugar kelp biomass for biorefining. Biomass Bioenergy 151, 106134. doi:10.1016/j.biombioe.2021.106134
Lenth, R. V. (2016). Least-squares means: the R package lsmeans. J. Stat. Softw. 69 (1), 1–33. doi:10.18637/jss.v069.i01
López-Pedemonte, T. J., Roig-Sagués, A. X., Trujillo, A. J., Capellas, M., and Guamis, B. (2003). Inactivation of spores of Bacillus cereus in cheese by high hydrostatic pressure with the addition of nisin or lysozyme. J. Dairy Sci. 86 (10), 3075–3081. doi:10.3168/jds.S0022-0302(03)73907-1
Løvdal, T., Lunestad, B. T., Myrmel, M., Rosnes, J. T., and Skipnes, D. (2021). Microbiological food safety of seaweeds. Foods 10 (11), 2719. doi:10.3390/foods10112719
Lytou, A. E., Schoina, E., Liu, Y., Michalek, K., Stanley, M. S., Panagou, E. Z., et al. (2021). Quality and safety assessment of edible seaweeds Alaria esculenta and Saccharina latissima cultivated in Scotland. Foods 10 (9), 2210. doi:10.3390/foods10092210
Martelli, F., Marrella, M., Lazzi, C., Neviani, E., and Bernini, V. (2021). Microbiological contamination of ready-to-eat algae and evaluation of Bacillus cereus behavior by microbiological challenge test. J. Food Prot. 84 (7), 1275–1280. doi:10.4315/JFP-20-407
Mavrommati, M., Daskalaki, A., Papanikolaou, S., and Aggelis, G. (2022). Adaptive laboratory evolution principles and applications in industrial biotechnology. Biotechnol. Adv. 54, 107795. doi:10.1016/j.biotechadv.2021.107795
NMKL:136 (2010). Listeria monocytogenes. Detection in foods and feeding stuffs and enumeration in foods. Bergen, Norway: Nordisk Metodikkomité for Næringsmidler.
NMKL:140 (2023). Lactic acid bacteria. Determination in food in association with food spoilage. Bergen, Norway: Nordisk Metodikkomité for Næringsmidler.
NMKL:156 (1997). Pathogenic Vibrio species. Detection and enumeration in foods. Bergen, Norway: Nordisk Metodikkomité for Næringsmidler.
NMKL:189 (2011). Aerobic or anaerobic microorganisms or bacterial spores. Enumeration on Blood Agar. Bergen, Norway: Nordisk Metodikkomité for Næringsmidler.
NMKL:67 (2021). Presumptive Bacillus cereus. Determination in foods. Bergen, Norway: Nordisk Metodikkomité for Næringsmidler.
NMKL:98 (2005). Mould and yeasts. Determination in foods and feed. Bergen, Norway: Nordisk Metodikkomité for Næringsmidler.
O'Sullivan, L., Murphy, B., McLoughlin, P., Duggan, P., Lawlor, P. G., Hughes, H., et al. (2010). Prebiotics from marine macroalgae for human and animal health applications. Mar. Drugs 8 (7), 2038–2064. doi:10.3390/md8072038
Philis, G., Gracey, E. O., Gansel, L. C., Fet, A. M., and Rebours, C. (2018). Comparing the primary energy and phosphorus consumption of soybean and seaweed-based aquafeed proteins – a material and substance flow analysis. J. Clean. Prod. 200, 1142–1153. doi:10.1016/j.jclepro.2018.07.247
R Development Core Team (2022). R: a language and environment for statistical computing. Vienna, Austria: R Foundation for Statistical Computing. Available at: https://www.R-project.org/.
Rezac, S., Kok, C. R., Heermann, M., and Hutkins, R. (2018). Fermented foods as a dietary source of live organisms. Front. Microbiol. 9, 1785. doi:10.3389/fmicb.2018.01785
Rotter, A., Bacu, A., Barbier, M., Bertoni, F., Bones, A. M., Cancela, M. L., et al. (2020). A new network for the advancement of marine biotechnology in Europe and beyond. Front. Mar. Sci. 7 (278). doi:10.3389/fmars.2020.00278
Sandbakken, I. S., Sæther, M., Funderud, J., and Aasen, I. M. (2018). Acid preservation of Saccharina latissima for application as a carbon source for fermentation to biofuels and chemicals. J. Appl. Phycol. 30 (6), 3581–3588. doi:10.1007/s10811-018-1489-z
Sedó Molina, G. E., Shetty, R., Xiao, H., Wätjen, A. P., Tovar, M., and Bang-Berthelsen, C. H. (2022). Development of a novel lactic acid bacteria starter culture approach: from insect microbiome to plant-based fermentations. LWT-Food Sci. Technol. 167, 113797. doi:10.1016/j.lwt.2022.113797
Sørensen, J. S., Madsen, S. K., Bang-Berthelsen, C. H., and Hansen, L. T. (2021). Quality and safety aspects in fermentation of winged kelp (Alaria esculenta) and sugar kelp (Saccharina latissima) by the natural microbiota with or without addition of a Lactiplantibacillus plantarum starter culture. Food Res. Int. 150, 110800. doi:10.1016/j.foodres.2021.110800
Stévant, P. (2019). Seaweeds in food applications: effects of processing on product quality. Ph.D thesis. Trondheim, Norway: Norwegian University of Science and Technology NTNU. Available at: https://ntnuopen.ntnu.no/ntnu-xmlui/handle/11250/2606704.
Stévant, P., and Rebours, C. (2021). Landing facilities for processing of cultivated seaweed biomass: a Norwegian perspective with strategic considerations for the European seaweed industry. J. Appl. Phycol. 33, 3199–3214. doi:10.1007/s10811-021-02525-w
Stévant, P., Rebours, C., and Chapman, A. (2017). Seaweed aquaculture in Norway: recent industrial developments and future perspectives. Aquacult Int. 25 (4), 1373–1390. doi:10.1007/s10499-017-0120-7
Thomas, J.-B. E., Sodré Ribeiro, M., Potting, J., Cervin, G., Nylund, G. M., Olsson, J., et al. (2020). A comparative environmental life cycle assessment of hatchery, cultivation, and preservation of the kelp Saccharina latissima. ICES J. Mar. Sci. 78 (1), 451–467. doi:10.1093/icesjms/fsaa112
Uchida, M., Amakasu, H., Satoh, Y., and Murata, M. (2004). Combinations of lactic acid bacteria and yeast suitable for preparation of marine silage. Fish. Sci. 70, 507–517. doi:10.1111/j.1444-2906.2004.00832.x
Uchida, M., and Miyoshi, T. (2013). Algal Fermentation^|^mdash;The seed for a new fermentation industry of foods and related products. JARQ-Jpn Agr Res. 47 (1), 53–63. doi:10.6090/jarq.47.53
Wirenfeldt, C. B., Sørensen, J. S., Kreissig, K. J., Hyldig, G., Holdt, S. L., and Hansen, L. T. (2022). Post-harvest quality changes and shelf-life determination of washed and blanched sugar kelp (Saccharina latissima). Front. Food Sci. Technol. 2, 1030229. doi:10.3389/frfst.2022.1030229
Keywords: processing, preservation, seaweed, macroalgae, lactic acid bacteria, challenge test
Citation: Stévant P, Zioga E, Steenholdt Sørensen J, Heiner Bang-Berthelsen C, Dahl TH, Barnung T, Kleppe M and Løvdal T (2025) Fermentation of sugar kelp (Saccharina latissima): exploring the potential of the kelp’s native microbiota as starter culture and the microbiological food safety of fermented products. Front. Food. Sci. Technol. 4:1490547. doi: 10.3389/frfst.2024.1490547
Received: 03 September 2024; Accepted: 22 November 2024;
Published: 03 February 2025.
Edited by:
Milena Ramirez-Rodrigues, California Polytechnic State University, United StatesReviewed by:
Giustino Tribuzi, Federal University of Santa Catarina, BrazilCopyright © 2025 Stévant, Zioga, Steenholdt Sørensen, Heiner Bang-Berthelsen, Dahl, Barnung, Kleppe and Løvdal. This is an open-access article distributed under the terms of the Creative Commons Attribution License (CC BY). The use, distribution or reproduction in other forums is permitted, provided the original author(s) and the copyright owner(s) are credited and that the original publication in this journal is cited, in accordance with accepted academic practice. No use, distribution or reproduction is permitted which does not comply with these terms.
*Correspondence: Pierrick Stévant, cGllcnJpY2suc3RldmFudEBtb3JlZm9yc2tpbmcubm8=
Disclaimer: All claims expressed in this article are solely those of the authors and do not necessarily represent those of their affiliated organizations, or those of the publisher, the editors and the reviewers. Any product that may be evaluated in this article or claim that may be made by its manufacturer is not guaranteed or endorsed by the publisher.
Research integrity at Frontiers
Learn more about the work of our research integrity team to safeguard the quality of each article we publish.