- 1Agricultural and Food Engineering Department, Indian Institute of Technology Kharagpur, Kharagpur, India
- 2Max Planck Institute for Polymer Research, Mainz, Germany
Introduction: Elephant foot yam starch is highly susceptible to enzymatic digestion. To address this, heat-moisture treatment (HMT) has been used to modify its digestive properties and functionalities. However, when using modified starch in various food compositions, it is ultimately necessary to cook it in water-rich environments. The present work thus offers a systematic understanding of the heating and cooking procedures for modified starch, with a view to maintaining its structural and digestive properties.
Methods: Experimental investigations employing X-ray diffraction, scanning electron microscopy, and enzymatic digestion have been utilized to gain insights into the characteristics of cooked starches. The interactions between amylose and amylopectin in native starch are more susceptible to disruption during cooking, which results in changes in texture and a reduction in resistant starch (RS) retention. Additionally, these preparations have been investigated by tribology to test structure and and friction.
Results and discussion: The application of HMT has been observed to induce changes that stabilize the interactions between amylose and amylopectin, thereby ensuring better maintenance of granular integrity and higher RS retention under specific cooking conditions. The findings indicate that following a 2-min cooking period at 100°C, the modified elephant foot yam starch (EFYS) demonstrated enhanced granular stability, which was subsequently compromised after 10 min of cooking. Additionally, when modified elephant foot yam starch (EFYS) was heated at a temperature of 86°C for 2 and 6 min, it demonstrated superior preservation of resistant starch (RS) in comparison to a cooking time of 10 min. Moreover, the HMT starch exhibited the highest RS retention when cooked at 100°C for 2 and 6 min at approximately 39.12% and 20.23%, respectively. However, this retention decreased significantly to 1.08% after 10 min of cooking. Furthermore, the insights from tribology, when combined with the proposed naive models, enhance the understanding of how preparation conditions affect the functionalities of starches, which is crucial for designing food products with desired textural and nutritional qualities, especially in water-rich environments, where maintaining structural integrity and RS content is important.
1 Introduction
Starch serves as a substantial source of carbohydrate that in addition to being a staple food, is also utilized as a functional ingredient. The physicochemical properties of starch directly result in its utilization in a diverse range of food and non-food applications. Starch contributes significant features such as thickening, homogenization, gelatinization, and shelf stability in a variety of food applications. Despite these technologically convincing advantages, starch has been shown to have unfavorable negative health effects on consumers. Due to the enhanced glycemic index of starch, it can lead to pancreas-related health problems when consumed in high quantities (Lal et al., 2021). In consequence, there is a huge demand for producing functional carbohydrate with a lower glycemic index, especially in view of the required food transformation toward plant-based foods. It is, therefore, considered that resistant starch represents a promising solution. Resistant starch (RS) refers to starches that are less digestible and, therefore, do not release all glucose building blocks during the gastrointestinal passage. Instead, they are only fermented in the large intestine, offering numerous and diverse advantages. Starches, which consist of both linear amylose and branched amylopectin, need to be modified in such a way that they are not broken down by amylases in the oral cavity and small intestine (Bojarczuk et al., 2022). Conversely, consumers are more inclined to accept modifications to starch that are solely physical in nature than those that involve chemical changes. This preference is exemplified by the use of different heat and moisture treatments, which will be discussed in the following sections.
Elephant foot yam (EFY) roots are an under-utilized tropical tuber crop mainly cultivated in humid regions in India, South-East Asia, and Africa and referred to as the ‘king of tuber crops’ because of its higher starch yield (Kumar et al., 2016). Therefore, the starch derived from EFY can be considered an exceptional alternative to commonly available sources of starch such as rice, potato, maize, corn, and taro (Barua et al., 2021a). To improve functionality and reduce negative health effects, starch extracted from the EFY root was subjected to heat-moisture treatment (HMT). HMT is a well-chosen physical modification method where starch is exposed to moisture at ≤ 35% (w/w) and treated at a definite temperature range between 80°C and 140°C for a defined period of 0.25 to 16 h (Sui et al., 2015). Moisture and temperature need to be adjusted between the moisture-dependent glass transition and melting temperature. However, previous studies have already addressed the optimization of HMT processes and the impact of modifications on the structural length scales of elephant foot yam starch (EFYS) (Barua et al., 2021b; Barua et al., 2022; Barua et al., 2024). Moreover, the current study emphasizes the understanding of the cooking behavior on the alteration of lamellar structure and the degree of branching (DB) of amylopectin resulting from the localized debranching of α-(1,6)-glycosidic bonds during various forms of hydrothermal modification treatments, such as hot air oven (HAO), autoclave (AL), and microwave treatment (MW). This is exemplified by the X-ray experiments conducted in the study. These structural changes have important impacts on molecular and macroscopic levels (Wang et al., 2016; Chen et al., 2017; Barua et al., 2022). For convenience, the main physical changes during the different HMT methods are summarized in Figure 1.
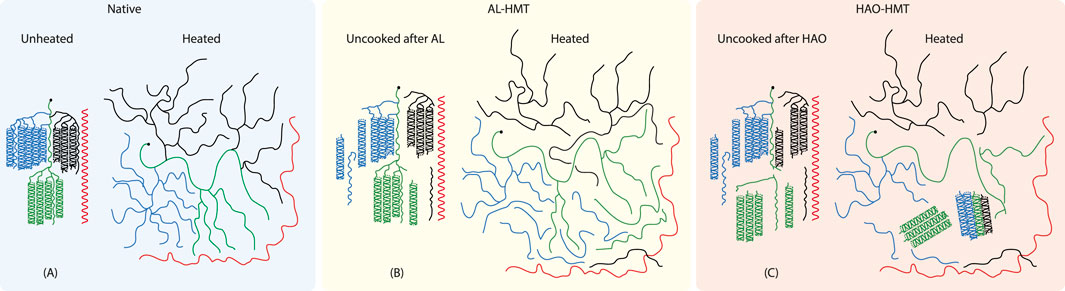
Figure 1. Illustration of the main changes of native starch (A) by autoclave (AL) (B) and hot air oven (HAO) treatment (C) (strongly exaggerated drawing).
Experiments (Barua et al., 2022; Barua et al., 2024) and literature data (Fonseca et al., 2021; Li et al., 2023) suggested that heat-moisture treatment changes the starch on molecular levels. These experiments indicate that HAO treatments showed the most changes regarding amylopectin. Some blocklets are cleaved from amylopectin branches and form nano-crystals (shown in green color in Figure 1), as well as some helical parts, which get rearranged during cooking. Although the remnants of amylopectin swell, the nano-crystals are stable, partly defining the indigestible resistant part of the starch. AL treatment had the least effect on the structure. According to gel permeation chromatography (GPC) results, it can be concluded that far fewer branches of amylopectin are cleaved off (Barua et al., 2022, see Supplementary Figure S1; Supplementary Table S1 in Supplementary Material). Thus, the behavior is closer to that of native starch compared to that of HAO-treated starch. The microwave (MV) treatment is not shown explicitly since the changes are somewhere in between native and HAO-treated starch.
As can be seen, the utilization of any modified starch in different food applications requires cooking in water-rich environments, which changes the structure of the modified starch accordingly. It has been reported that modified starch contains still higher concentrations of digestion-resistant components (Cheng et al., 2022). As the purpose of the production of modified starch is to enhance the functionalities and reduce the digestibility for diabetes and obese patients, hence the systematic study of the alterations in the structure and digestion is important after cooking. Thus, the present work demands an intensive deeper understanding of the structure–property–digestion relationship of the cooked starch. To investigate the effects of the structural changes induced by HMT, several new experiments have been performed in the present work. As suggested earlier, different HMT methods change structures of native starch, which leads to the alterations in functional properties, pasting behavior, and the physical properties of the modified starch (Liu et al., 2020). According to Barua et al. (2024), detailed rheology experiments were carried out, yielding valuable insights into the flow and pasting properties of the samples. These findings, combined with the results obtained from GPC investigations, facilitated the development of straightforward structural models. The application of HMT resulted in higher levels of short-chain amylose (SCA), leading to significant alterations in the physical properties. However, to understand the structure–property relationships and their implications on digestion better, further confirming experiments are needed. Therefore, to control the crystalline and indigestible parts before and after cooking and alternative heat processing, wide-angle X-ray scattering experiments will be discussed in the present work. Finally, tribology offers further information about the conformation of the modified starches’ paste since friction coefficients are sensitive to the molecular structure and show important differences between native and distinguishably HMT starches. In addition, it relates the structural changes to mouthfeel parameters.
The current study aims to identify the optimal cooking time–temperature parameters for HMT EFYS with the goal of maintaining the existing structural modifications and resistant starch fractions while enhancing their suitability for various applications. These findings will be further expanded upon and complement previous investigations, focusing on the optimization of HMT methods and the resulting modifications in the functional properties of EFYS (Barua et al., 2021; Barua et al., 2022). As discussed earlier, it was clearly shown that HAO treatment imposes the most substantial changes in the functional and digestive properties of EFYS compared to the other HMT methods. Therefore, the properties of HAO-treated EFYS are considered the main objective, although all results from the other methods will be presented for comparison. Hence, three cooking temperatures were selected based on the gelatinization temperatures of native and HAO-modified EFYS. The gelatinization temperature of native and HAO-modified EFYS is 86°C and 91 °C, respectively (Barua et al., 2022), and in addition, a cooking temperature of 100°C was also selected as a reference. Furthermore, scanning electron microscopy was also performed to gather additional structural information on cooked starch, and X-ray diffraction analysis was accomplished to measure the crystallinity of cooked starches. Thus, with the aforementioned analysis, we offered a systematic understanding that describes the modification effect on EFYS on different length scales and cooking properties.
2 Materials and methods
2.1 Materials
Bidhan Chandra Krishi Vishwavidyalaya, West Bengal, India, provided the elephant foot yam (EFY) (cultivar: Bidhan Kusum). The chemicals and solvents used were of the highest quality according to the American Chemical Society (ACS).
2.2 Starch extraction
Sukhija et al. (2016) described a method for extracting starch from EFY. The tuber crops were subjected to peeling and shredding, followed by cleaning with potassium metabisulphite solution (0.25% w/v) containing 0.12% citric acid (0.12% w/v) for an hour. The cleaned, peeled, and cut tuber crops were exposed to grinding in a laboratory grinder with the addition of water and then filtered through a 300 BSS filter mesh. The filtrate was stored at 4°C for 90 min for sedimentation. The starch slurry was washed many times until the pH reached neutral, and to ensure maximal starch isolation, the starch slurry was centrifuged at 3,500 g for 10 min. Finally, the layer from the top was decanted, and the residue starch was subjected to drying in a hot air oven at 40°C overnight. The extracted starch had a moisture content of 6% (w/w) and a purity of 99.09%.
2.3 Starch modification using various heat-moisture treatment methods
The methodology described by Barua et al. (2021b) was used to modify the optimal condition. The starch had the initial moisture content of 6% (w/w), and with frequent stirring, a precise amount of distilled water was added to obtain the targeted moisture contents for each treatment. Using the various heating procedures, the following methods can be used to produce the optimized modified EFYS for minimizing digestibility:
• EFYS of 100 g (35% w/w, dry basis) was transferred into a screw-capped glass container, which was placed in an oven. Hot-air oven (HAO) treatment was performed in a Heraeus oven (Thermo Fisher Scientific, United States) at 120°C for 4 h.
• EFYS of 100 g (15% w/w, dry basis) was subjected to an airtight autoclavable polypropylene container for treating in an autoclave (Model RA-PC, manufactured by Reico Equipment and Instrument Pvt. Ltd.) at 128°C (pressure 25 PSI) for 30 min.
• EFYS of 100 g (35% w/w moisture content, dry basis) was treated in an airtight microwavable glass container in a microwave (Model UWave-1000, produced by Shanghai Sineo Microwave Chemistry Technology Co., Ltd.) for 120 s at 520 W.
All the treated starch samples were dried in a hot air oven at 40°C overnight. To obtain homogeneous particle sizes, the dried starch samples were ground with a mortar–pestle and passed through a 150-µm sieve mesh.
2.4 Cooking of native and modified starches
All the starch slurries were prepared by mixing 1 g of the sample with 4 mL of distilled water (25% w/w) in a vortex shaker (Ika Vortex Genius 2, Sigma-Aldrich, Germany) at 500 rpm for 5 min. Then, the starch slurries were subjected to heating at 81°C, 95°C, and 100°C for 2, 6, and 10 min, respectively, using the Heidolph MR Hei-Standard thermal shaker (Hans Heidolph GmbH and Co. KG, Germany) at 500 rpm.
2.5 Wide angle X-ray diffraction of cooked native and modified starch pastes
Uncooked and cooked native and modified EFYS was measured using a Rigaku SmartLab X-ray diffractometer (9 kW, fine focus, CuK α radiation) and parallel Cross Beam Optics HyPix-3000 semiconductor 2D detector. At 25°C, data were gathered in 0 to 2θ scans between 5° and 80° with an increment speed of 0.5°/min. The relative crystallinity (RC) percent of the samples was calculated using the following equation:
where Aa denoted the amorphous phase region and Ac denoted the crystalline peak, respectively.
2.6 Morphology of cooked native and modified starch pastes
A scanning electron microscope (LEO 1530 Gemini, Germany) was applied to analyze the morphology of native and modified cooked starch pastes at a low voltage of 0.100 kV, indicating that no metal sputtering or carbon coating was applied to the surface previous to the observation. The operating distance of the electron probe was kept constant at 1.9 mm.
2.7 Resistant starch fractions of cooked native and modified starch pastes
The method reported by Ye et al. (2016) was used to measure the resistant starch (RS) fractions in cooked native and HAO starch. After heating the starch suspensions as stated in the preceding section, the samples were cooled at room temperature before adding 10 mL (0.5 M) sodium acetate buffer with a pH of 5.2. The starch suspension was vigorously mixed with a vortex mixer (Ika Vortex Genius 3), and the enzyme suspension (amyloglucosidase, 35 U/mg, 1.1 mg/mL and pancreatic α -amylase, 50 U/mg, 5 mg/mL) was added for each sample. All the samples with enzyme solutions were incubated into a shaking water bath (RWBS, Reico Equipment and Instrument Pvt. Ltd.) of 220 strokes/min at 37°C for 120 min. After 120 min, aliquots (0.2 mL) were removed and boiled in a hot-water bath at 100°C to terminate the hydrolysis reactions. The GOD-POD kit-released glucose was measured using a spectrometer (Varian 50 Bio UV-visible spectrometer, Agilent Technologies, United States) at 510 nm using aliquots (0.2 mL) of the supernatant. By a factor of 0.9, the amount of released glucose was transformed into a starch component. The resistant starch fraction (RS) was determined using the following equation:
where DS120 (%) represents the amount of starch content at 120 min in the starch. The purity of starch was 99.09 g/g after the extraction from EFY.
2.8 Tribology
The friction of native and various modified EFYS was identified with a Tribo-Rheometry Accessory for Discover HR-3 Rheometers (TA Instruments New Castle, England). Plate geometry with three balls was utilized in the tribology test, with three spheres positioned on a circular plate with a radius of 15 mm for the upper portion, and a silicon substrate was attached on the lower plate. A stainless-steel ball and a silicon rubber with a thickness of 0.5 mm and diameter of 40 mm formed the contact surface of tribopairs. The DHR-3 Rheometer supplied the stainless-steel balls, which are hemispheres with a diameter of 7.9375 mm (5/16′′) and ensure point contact with the silicon surface. The stainless-steel hemispheres are screwed onto the upper plate that is connected to a helical structure spring-like aluminum beam coupling that can withstand loads of up to 20 N. This self-aligned construction enables homogeneous contact (solid–solid) and controls the distribution of axial force. This allows axial flexibility to provide uniform axial force adjustment for appropriate surface alignment and homogeneous normal force distribution throughout rotation (specified by the Trios manual 2019). The Peltier plate was coupled with the lower plate geometry for stable temperature regulation. All the starch slurries were prepared by mixing 1 g of the sample with 4 mL of distilled water (25% w/w) and mixed using a vortex shaker (Ika Vortex Genius 3, Sigma-Aldrich, Germany) for 5 min. Then, the starch slurries were heated at 95°C for 5 min using Heidolph MR Hei-Standard (Hans Heidolph GmbH and Co. KG, Germany) at 500 rpm. The gelatinized samples were cautiously transferred onto the silicon surface using a spatula during the tribology tests, which were carried out at 25°C. The typical forces employed were 3 N, which were similar to the normal forces used during oral processing (Ghebremedhin et al., 2021), and the upper three-ball geometry was rotated ranging from 100 to 1,000,000 micron/s to adjust the sliding speed, while the lower plate geometry remained static. The friction force FF and friction coefficient μ were estimated using Trios software.
2.9 Statistical analysis
All the experiments were conducted in triplicates, and the results were provided as mean ± standard deviation. Means were compared using one-way analysis of variance (ANOVA) with Duncan’s multiple comparison test (p < 0.05) with a significance of 95% using SPSS statistical software (22 version, IBM).
3 Results and discussions
3.1 Effect of cooking on the crystalline structure of native and modified starches
The changes in the orthorhombic (black dashed lines) and hexagonal (red dashed lines) structural indexing for the starches during X-ray diffraction analysis after cooking are shown in Figures 2A–C. The reduction in relative crystallinity was clearly visible by increasing the cooking time for native and HAO-treated EFYS. Rodriguez-Garcia et al. (2021) recently published a full indexation for the orthorhombic and hexagonal crystalline formations that have been adapted in our present study. The alternation in crystalline structures can be due to the uncoiling of amylopectin double helices and restructuring of amylopectin side chains during cooking. However, the changes from orthorhombic to hexagonal structures were prominent in all cooked starches. The samples of native starch cooked at 86°C for 2 min showed the retention of crystalline fractions (42%), and upon increasing the heating time up to 6 min, the relative crystallinity was reduced to 19%. However, native EFYS cooked at 86°C for 10 min turned into completely amorphous state. On the other hand, the retention of orthorhombic and hexagonal crystalline peaks was noted in HAO-treated EFYS after cooking at 86°C for 2, 6, and 10 min (Figure 2A). However, the slight retention of the peak at 23° was observed in HAO-treated EFYS compared to its native counterpart. The retention of certain crystalline peaks of HAO-treated EFYS can be attributed to the presence of stronger structural arrangement that can show resistance against heating at various cooking times, as described in the Introduction.
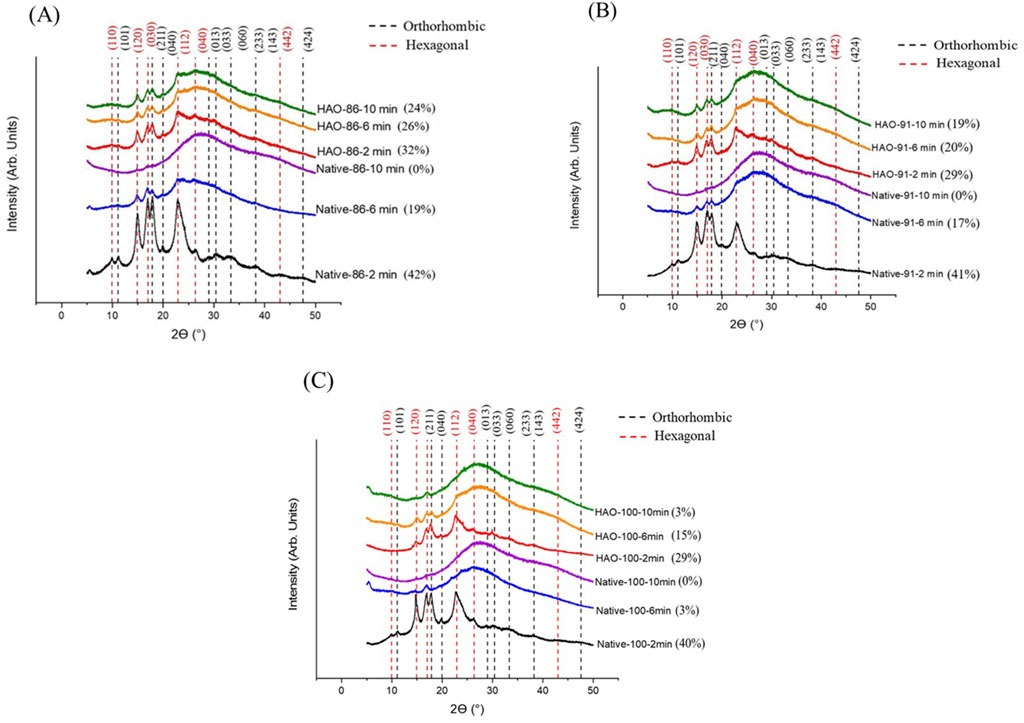
Figure 2. (A) X-ray diffraction patterns for native and HAO-treated EFYS cooked at 86°C. (B) X-ray diffraction patterns for native and HAO-treated EFYS cooked at 91°C. (C) X-ray diffraction patterns for native and HAO-treated EFYS cooked at 100°C.
The native and HAO-treated EFYS showed reduction in RC while cooking at 91°C for 2, 6, and 10 min. However, HAO-treated EFYS showed persistence of crystalline peaks upon increasing the heating time, which led to 29, 20, and 19% retention of RC for 2, 6, and 10 min, respectively (Figure 2B). In the native EFYS samples, all crystallinity disappeared after 10 min of cooking and turned amorphous. HAO-treated EFYS preserved the crystalline structures and showed crystalline peaks at 17°. The retention of crystalline peaks by HAO-treated EFYS when heated at 91°C, in comparison to its native counterpart, also corroborates the strengthening of the structure, following HMT modification, which permits the retention of the partial crystalline structure upon heating. At a further increase of the cooking temperature, i.e., 100°C for 10 min, destruction of the crystalline structure for both native and HAO-treated EFYS is visible. The relative crystallinity of the HAO-treated EFYS was observed to be 29% when cooked for the duration of 2 min, which subsequently reduced to 15% when the cooking time was extended to 6 min. Furthermore, an increase in the heating time of up to 10 min resulted in the destruction of starch crystallites, leading to a reduction in the RC to 3%.
However, native EFYS showed reduction to 3% RC when cooked for 6 min, and further 10 min of cooking led to the generation of only the amorphous state (Figure 2C). The Miller indices and crystalline phase according to the peak positions of the cooked starches are shown in Supplementary Table S1 in Supplementary Material. The current study confirms the retention of crystalline structures and crystallinity of HAO-treated EFYS. As expected, native EFYS showed a strong reduction of crystallinity during heating at 86, 91, and 100°C compared to HAO-treated EFYS.
3.2 Effect of cooking on the morphology of native and HAO-treated EFYS
The effect of cooking time and temperature combination on the morphology of native and HAO-treated EFYS was studied and is shown in Figures 3A–C. Native EFYS, when cooked at 86°C for 2 min, showed the presence of granular interspace with no aggregations or cluster formations. This structural occurrence can lead to higher enzyme susceptibility. However, upon increasing the heating time to up to 10 min, native starch showed formation of particle clusters with more disrupted surface, accelerating a higher enzyme attack. Contradictorily, HAO-treated EFYS, when cooked at 86°C for 2 and 10 min, showcased granular integrity with less surface distortion. This can be due to the structural changes that occurred after modification that led to increased granular stability after cooking.
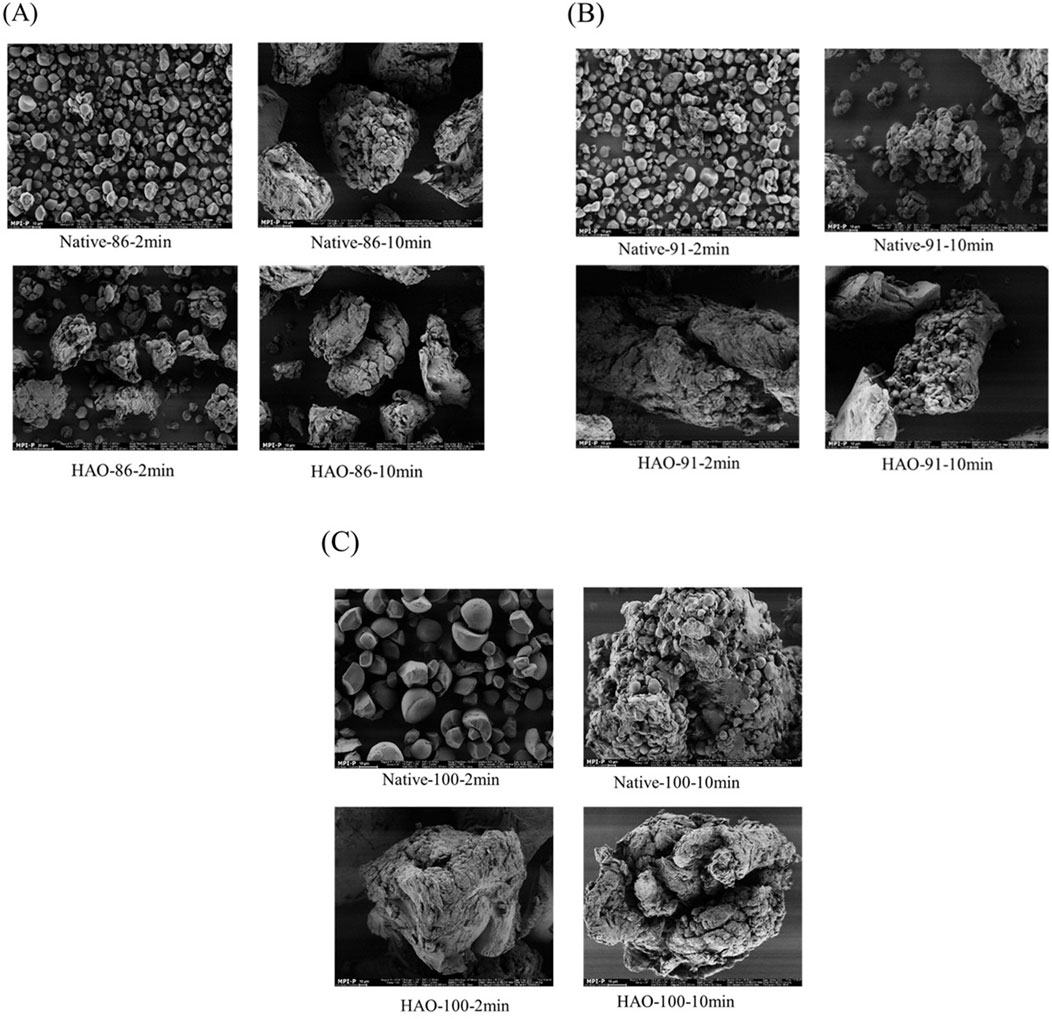
Figure 3. (A) Granular morphology of native and HAO-treated EFYS when cooked at 86°C for 2 and 10 min. (B) Granular morphology of native and HAO-treated EFYS when cooked at 91°C for 2 and 10 min. (C) Granular morphology of native and HAO-treated EFYS when cooked at 100°C for 2 and 10 min.
Morphology of native EFYS showed higher granular disorder compared to that of HAO-treated EFYS when cooked at 91°C (Figure 3B). The disrupted native EFYS granules contribute to a higher surface area for enzyme attack compared to its HAO-treated counterpart. Meanwhile, HAO-treated samples cooked at 91°C for 2 min retained their granular integrity with a less available path for enzyme hydrolysis. However, higher surface defect was observed when cooked for 10 min in HAO-cooked EFYS. This indicates that the retention of structural integrity is highly visible in HAO EFYS compared to its native counterpart when heated at 91°C for 2 and 10 min.
In addition, a similar understanding was identified when native and HAO-treated EFYS were cooked at a classical cooking temperature of 100°C for 2 and 10 min (Figure 3C). Native EFYS granules were evidently present upon 100°C cooking for 2 min, whereas increasing cooking time up to 10 min showed disruption of starch granules with more available space for enzymatic hydrolysis. However, generation of a compact and resistant structure against enzymes has been observed in HAO EFYS when treated at 100°C for 2 min. Increasing the treatment time until 10 min leads to the increase of surface disorder, leading to the structure being highly accessible to enzymes for hydrolysis. The current results are in line with previous publications, supporting the hypothesis that HAO modification results in the creation of a more stable and compact structure that is not degraded further upon enzyme hydrolysis after cooking, which also aligns with RC calculated from XRD analysis in the previous section.
3.3 Effect of cooking on resistant starch (RS) fractions
The effect of cooking at 86, 91, and 100°C on resistant starch fractions in native and HMT-modified EFYS is described in Figures 4A–C. Native EFYS starch cooked at 86°C for 2 min showed RS of approximately 29.59%, which further reduced to 20.08% upon increasing the cooking time to 6 min and exhibited only 1% RS upon 10 min of cooking. Meanwhile, HAO EFYS cooked at 86°C for 2 min showed 45.12% of RS which reduced to 31.23% upon 6 min cooking time (Figure 4A). However, HAO EFYS cooked at 86°C for 10 min EFYS showed higher retention RS fraction to 21.08% compared to the native counterpart. Considering 91°C, native EFYS cooked for 2 min showed an RS fraction of 24.59%, which reduced to 19.08% upon 6 min cooking time and further drastically reduced to 1% at 10 min. At the same temperature, HAO EFYS cooked for 2 min showed a comparatively higher RS fraction of 42.12%, which also similarly reduced to 20.23% upon 6 min of cooking and finally showed 10.08% RS fraction after 10 min cooking (Figure 4B). Furthermore, upon increasing the cooking temperature to 100°C, native EFYS cooked for 2 min exhibited 22.59% RS fraction, which was reduced drastically to 1.08% and 1% for 6 and 10 min of cooking. However, HMT-modified EFYS shows considerably higher RS fractions after cooking at the same temperature for 2, 6, and 10 min compared to native starch (Figure 3C). Hence, HMT modification confirms the structural rearrangements inside starch granules, resulting in resistance to enzyme attack, and increased RS fractions after cooking.
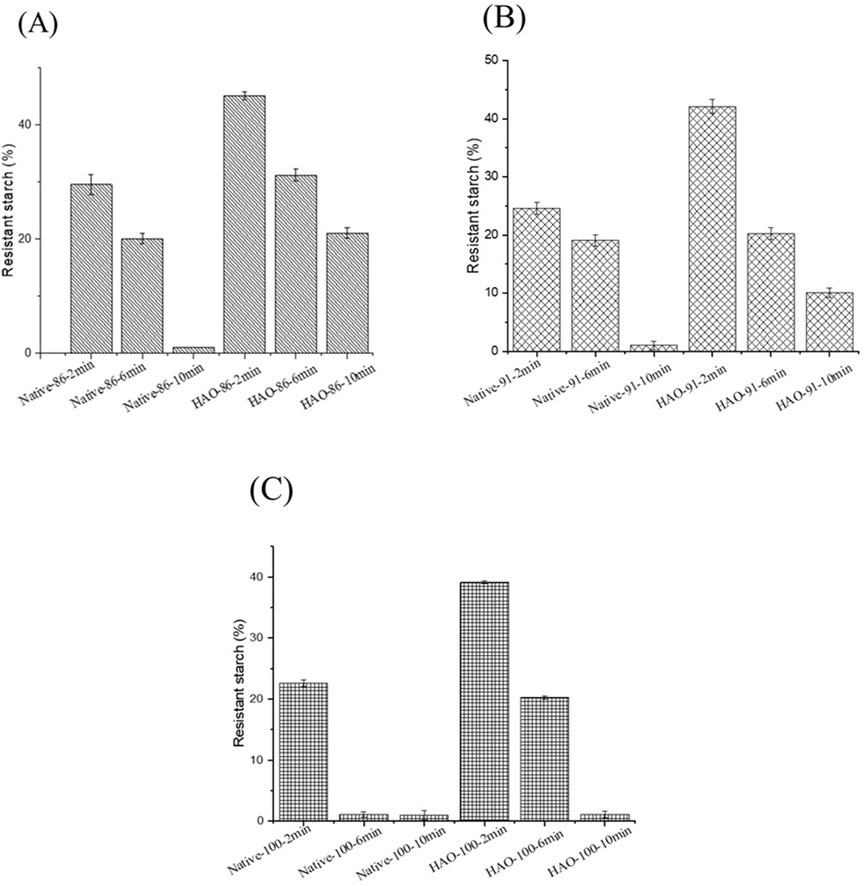
Figure 4. (A) Resistant starch fraction of native and HAO-treated EFYS when cooked at 86°C. (B) Resistant starch fraction of native and HAO-treated EFYS when cooked at 91°C. (C) Resistant starch fraction of native and HAO-treated EFYS when cooked at 100°C.
3.4 Tribology
Figure 5 displays the friction coefficient as a function of the sliding speed (see e.g., Stribeck curve, Wen et al., 2018), increasing from 100 to 1,000,000 μm/s. First, it can be seen that native starch, AL, and MW-treated starches show an overall higher friction coefficient than HAO-treated samples, except for very high speeds toward the hydrodynamic regime. The initial increase in friction at a low sliding speed, up to ∼237 μm/s (see dashed vertical line in Figure 5), is visible only in MW- and HAO-treated EFYS. For all four samples, a further increase in sliding speed of more than 1,000,000 μm/s near the hydrodynamic regime resulted in decrease in friction. Commonly, it can be observed and explained that in the hydrodynamic regime, the speed of lubricant entrainment increases and separates the distance between the two surfaces, which leads to the decrement of the coefficient of friction (Ghebremedhin, et al., 2021; Shewan et al., 2020). Interestingly, as depicted in Figure 5, all the modified starches exhibited reduced coefficients of friction, resulting in minimal alterations in the case of AL starch when compared to native starch. To better understand and interpret the experimental data, it is useful to recall the effects of the HMT on starch. The HAO modification exhibits a significant impact on EFYS compared to other modifications, particularly in the localized breaking of amylopectin side chains (α-1, 6 glycosidic bonds). This alteration results in the formation of flexible linear short chains that, upon cooling, connect with neighboring chains to generate double helices. These double helices are stabilized by hydrogen bonds and contribute to the development of a well-ordered short crystalline structure.
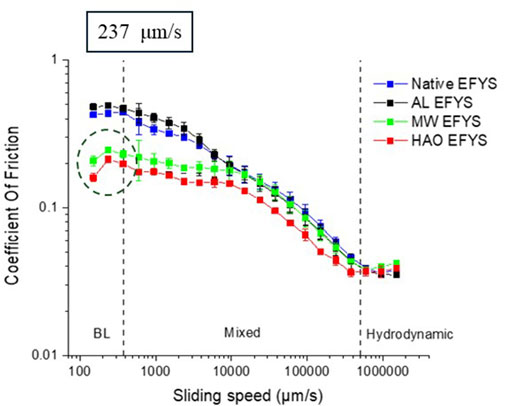
Figure 5. Stribeck curves with 3N applied normal load on native and modified starch pastes. The dashed lines (vertically) represent the various lubrication regimes: boundary lubrication (BL), mixed lubrication, and hydrodynamic lubrication.
During higher sliding speed, amylopectin requires higher conformational changes to pass through the gap between the balls and the silicon surface, which increases the friction as well. These assumptions are in line with the observation: the friction of native and AL, which shows the least effect of change, showcases the highest friction confident for all sliding speeds compared to HAO and MW starches. Furthermore, higher sliding speeds dynamically separate linear and branched chains, increasing alignment and reducing stickiness. These factors collectively contribute to a gradual reduction in friction across all starch samples. Interestingly the friction of HAO- and MW-treated starches is significantly lower compared to native and AL-treated starches, which originates from the treatment-induced conformational changes. Initially, at lower sliding speeds, the increase in friction for HAO suggests a response from higher degrees of degraded amylopectin. As shown by Barua et al. (2024) by GPC experiments, these methods have a higher impact on the crystalline part of the starch, and the amylose content is higher in HAO- and MW-treated EFYS. Shorter linear chains show a similar impact in concentrated systems on friction coefficients (Osaheni et al., 2020; Zhang et al., 2017; Liu et al., 2016). Barua et al. (2024) suggested that HAO and MW induced cleaved blocklets from the amylopectin backbone and branches from shorter amylose molecules, forming nano-crystals. These nano-crystals contribute significantly to the resistant nature of the resulting starch. These form anisotropic nano-crystals, which show higher stability and higher melting temperatures (Niu et al., 2020; Fonseca et al., 2021), ranging in size between 40 nm and 130 nm and 7 nm–40 nm in thickness depending on the origin of the starch and the experimental conditions (Li et al., 2023). The gaps in soft tribology are reported to be approximately 100 nm (Xu et al., 2021) and are able to pass through having less direct influence on the friction, and the overall friction is lower. However, since HAO- and MW-treated starch produces more amylose and more nano-sized blocklets, the friction is indirectly reduced. The remnant amylose and the transient network of amorphous long-chain amylose require strong conformational changes that contribute to the enhancement of the friction during the boundary lubrication (BL) regime during low sliding speeds. However, at initial sliding speed until 6000 μm/s, HAO modified EFYS showed the highest reduction in friction as compared to the other treated and native EFYS. During gelatinization the amylose leaching occurs from the granules and randomly forms transient network structures on a short time scale (Barua et al., 2024). Since linear and branched chains do not mix on local scales (Vilgis, 1988; Vilgis et al., 1994), the increase amount of amylose may act as lubricant and the overall friction reduces in modified EFYS as compared to native EFYS.
Furthermore, the results obtained in the friction experiments for HAO- and MW-treated EFYS are in accordance with the observations in rheology experiments published earlier (Barua et al., 2024). A rise in viscosity with increasing shear rate was observed, which imparts lubrication on surfaces, hence reducing the friction (Barua et al., 2024). At the hydrodynamic region, during high sliding speeds, the amylose and amylopectin branches could cause jamming in front of the stainless-steel rotating ball before their entrainment. They are then compressed and distorted before fitting between the surface gap. Therefore, upon further increasing the sliding speed, the friction increases simultaneously for all the samples. There are several important phenomena that can affect the tribology measurements simultaneously. To have a brief understanding, the effect of gelatinized starch on friction is a result of a combination of following factors: 1) surface contacts are separated by an increase in amylose transient network structures (lubrication effect for HAO- and MW-treated EFYS); 2) increase in stickiness due to a higher amount of amylopectin (native and AL treated EFYS) that prevent the surface from moving. As illustrated in Figure 6, the mechanisms for friction undergo alteration as a consequence of heat-moisture treatment. During the heating of native starch in water, gelatinization occurs, resulting in significant structural changes. The friction is primarily influenced by the rearrangement of the highly branched amylopectin and the transient network of linear amylose, which are constrained by the limited space within the gap. High excluded volume forces further separate the branched chains, while the linear chains are compelled to form an oriented transient network. Additionally, the entropic component of friction is augmented by the introduction of increased sticking interactions. In the case of HAO starch, the remnants of amylopectin are characterized by a reduction in branching, which consequently results in a decrease in entropic restrictions. Consequently, the adhesive interactions are also reduced. Furthermore, cleaved blocklets and reformed nano-crystals diminish friction, and they do not induce exhaustive adhesive interactions.
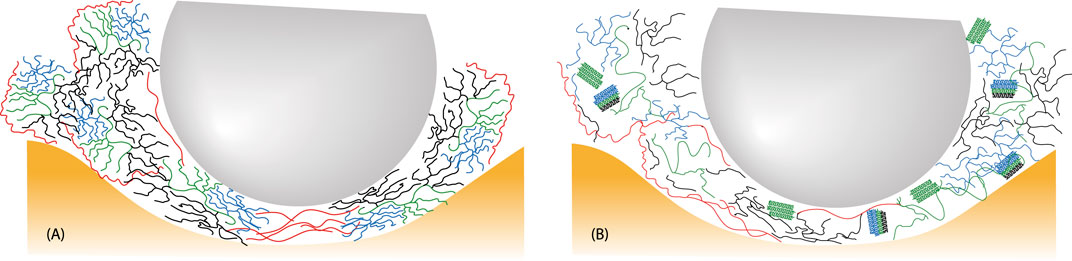
Figure 6. Oversimplified picture of native (A) and HAO starch (B) between one tribo-ball and the silicon surface. The colors of the amylopectin and amylose correspond to those as shown in Figure 1.
4 Summary and conclusion
The present study investigates the significance of the cooking time and temperature on the digestibility and structural properties of modified EFYS. Cooking at three different temperatures based on the gelatinization temperature of native and HAO-modified EFYS i.e., 86 and 91°C, showed changes in the hexagonal and orthorhombic crystalline structures of native and HAO-treated EFYS. At the classical cooking temperature of 100°C, it was observed that native starch underwent complete melting after 2 min of cooking. In contrast, HAO-modified starch demonstrated the retention of a crystalline phase in its structure even after the same cooking duration. Moreover, the notable preservation of the crystalline phase in HAO-modified EFYS, even after being cooked at 100°C for 6 min, indicates the presence of a robust structural arrangement that resist against melting at this elevated temperature. Nevertheless, the present study strongly suggests that when cooked under these conditions, the modified starch (HAO EFYS) exhibits superior structural preservation and is less susceptible to enzymatic digestion compared to its native counterpart. The retention of crystalline peaks of HAO-treated EFYS after cooking can be attributed to the presence of stronger structural arrangement that can show resistance against heating at various cooking times.
When native EFYS was cooked at an even lower temperature at 86°C for the duration of 10 min, the formation of particle clusters or aggregations with surface disorder was observed. This structural alteration makes the starch more susceptible to enzymatic digestion, resulting in a lower retention of the resistant starch (RS) fraction. However, scanning electron microscopic images from cooked HAO EFYS strongly suggest the presence of particle agglomerations with a denser and compact structure when cooked in similar cooking conditions. The preservation of the crystalline structure in cooked HAO-modified EFYS additionally aids in maintaining a higher retention of the resistant starch (RS) fraction during enzymatic digestion. The cooked native EFYS exhibited signs of having an increased surface area that is available for enzyme attack, which makes the structure more amorphous. Furthermore, the present investigation on the cooking conditions for modified EFYS is beneficial for further application of it into several food applications, considering its structural and digestive properties.
The optimization of preparation and cooking conditions for modified EFYS is crucial to retain its modification effect as it can yield better, healthier, and functional benefits in food application. In particular, the reduction of the cooking temperature below 90°C but higher than 80°C turns out to be a key issue for keeping sufficient non-digestible starch crystals in nutritional valuable concentrations, as confirmed by XRD studies. Despite the relatively low preparation temperatures, the friction in the corresponding physical heat-moisture treatments remains low, resulting in minimal perceptible differences in mouthfeel. The health benefits are clear: the proportion of resistant starch remains high, the glycemic index is lower, and the effective fiber content is higher. It is also important to note that not all HMT treatments result in a high yield of resistant starch. For example, the autoclave method (AL) has been shown to produce lower yields than other methods. Yields of resistant starch remain low in these cases. Going forward, it will be crucial for application-oriented research and product development to first fully characterize the HMT process using appropriate physicochemical methods based on basic research and model experiments. Additionally, it is important to clearly define the corresponding preparation methods, such as those applicable for pasta or prefabricated bakery products.
Data availability statement
The original contributions presented in the study are included in the article/Supplementary Material; further inquiries can be directed to the corresponding author.
Author contributions
SB: conceptualization, data curation, formal analysis, funding acquisition, investigation, methodology, project administration, resources, software, supervision, validation, visualization, writing–original draft, and writing–review and editing. PS: supervision and writing–review and editing. TV: conceptualization, data curation, funding acquisition, methodology, project administration, resources, supervision, validation, visualization, and writing–review and editing.
Funding
The author(s) declare that financial support was received for the research, authorship, and/or publication of this article. This project received support through the DAAD German Academic Exchange Service, Research Grants Bi-nationally Supervised Doctoral Degrees, 2019/20 (57440919).
Acknowledgments
The authors acknowledge valuable help by Stefan Geiter and Markus Mezger for the X-ray diffraction experiments, Gunnar Glaßer for scanning electron microscopy analysis, and Andreas Hanewald for tribology measurements. The authors are thankful to the authorities of the Institute, German Academic Exchange Service (DAAD), for providing the services and financial assistance to conduct this study.
Conflict of interest
The authors declare that the research was conducted in the absence of any commercial or financial relationships that could be construed as a potential conflict of interest.
The author(s) declared that they were an editorial board member of Frontiers, at the time of submission. This had no impact on the peer review process and the final decision.
Publisher’s note
All claims expressed in this article are solely those of the authors and do not necessarily represent those of their affiliated organizations, or those of the publisher, the editors, and the reviewers. Any product that may be evaluated in this article, or claim that may be made by its manufacturer, is not guaranteed or endorsed by the publisher.
Supplementary material
The Supplementary Material for this article can be found online at: https://www.frontiersin.org/articles/10.3389/frfst.2024.1466604/full#supplementary-material
References
Barua, S., Hanewald, A., Bächle, M., Mezger, M., Srivastav, P. P., and Vilgis, T. A. (2022). Insights into the structural, thermal, crystalline and rheological behavior of various hydrothermally modified elephant foot yam (Amorphophallus paeoniifolius) starch. Food Hydrocoll. 129, 107672. doi:10.1016/j.foodhyd.2022.107672
Barua, S., Khuntia, A., Srivastav, P. P., and Vilgis, T. A. (2021a). Understanding the native and hydrothermally modified elephant foot yam (Amorphophallus paeoniifolius) starch system: a multivariate approach. Lwt 150, 111958. doi:10.1016/j.lwt.2021.111958
Barua, S., Luciano, G., Ahmed, J., Srivastav, P. P., and Vilgis, T. A. (2024). Physics of starch system: rheological and mechanical properties of hydrothermally modified elephant foot yam starch. Food Biophys. 19 (1), 71–84. doi:10.1007/s11483-023-09803-9
Barua, S., Rakshit, M., and Srivastav, P. P. (2021b). Optimization and digestogram modeling of hydrothermally modified elephant foot yam (Amorphophallus paeoniifolius) starch using hot air oven, autoclave, and microwave treatments. LWT 145, 111283. doi:10.1016/j.lwt.2021.111283
Barua, S., Tudu, K., Rakshit, M., and Srivastav, P. P. (2021c). Characterization and digestogram modeling of modified elephant foot yam (Amorphophallus paeoniifolius) starch using ultrasonic pretreated autoclaving. J. Food Process Eng. 44 (11), e13841. doi:10.1111/jfpe.13841
Bojarczuk, A., Skąpska, S., Khaneghah, A. M., and Marszałek, K. (2022). Health benefits of resistant starch: a review of the literature. J. Funct. foods 93, 105094. doi:10.1016/j.jff.2022.105094
Chen, Y., Yang, Q., Xu, X., Qi, L., Dong, Z., Luo, Z., et al. (2017). Structural changes of waxy and normal maize starches modified by heat moisture treatment and their relationship with starch digestibility. Carbohydr. Polym. 177, 232–240. doi:10.1016/j.carbpol.2017.08.121
Cheng, Z., Li, J., Qiao, D., Wang, L., Zhao, S., and Zhang, B. (2022). Microwave reheating enriches resistant starch in cold-chain cooked rice: a view of structural alterations during digestion. Int. J. Biol. Macromol. 208, 80–87. doi:10.1016/j.ijbiomac.2022.03.034
Fonseca, L. M., El Halal, S. L. M., Dias, A. R. G., and da Rosa Zavareze, E. (2021). Physical modification of starch by heat-moisture treatment and annealing and their applications: a review. Carbohydr. Polym. 274, 118665. doi:10.1016/j.carbpol.2021.118665
Ghebremedhin, M., Seiffert, S., and Vilgis, T. A. (2021). Physics of agarose fluid gels: rheological properties and microstructure. Curr. Res. Food Sci. 4, 436–448. doi:10.1016/j.crfs.2021.06.003
Kumar, A., Ramakumar, P., Patel, A. A., Gupta, V. K., and Singh, A. K. (2016). Influence of drying temperature on physico-chemical and techno-functional attributes of elephant foot yam (Amorphophallus paeoniifolius) var. Gajendra. Food Biosci. 16, 11–16. doi:10.1016/j.fbio.2016.07.004
Lal, M. K., Singh, B., Sharma, S., Singh, M. P., and Kumar, A. (2021). Glycemic index of starchy crops and factors affecting its digestibility: a review. Trends Food Sci. and Technol. 111, 741–755. doi:10.1016/j.tifs.2021.02.067
Li, C., Guo, Y., Chen, M., Wang, S., Gong, H., Zuo, J., et al. (2023). Recent preparation, modification and application progress of starch nanocrystals: a review. Int. J. Biol. Macromol. 250, 126122. doi:10.1016/j.ijbiomac.2023.126122
Liu, G., Gu, Z., Hong, Y., Wei, H., Zhang, C., Huang, S., et al. (2020). Effects of molecular interactions in debranched high amylose starch on digestibility and hydrogel properties. Food Hydrocoll. 101, 105498. doi:10.1016/j.foodhyd.2019.105498
Liu, K., Stieger, M., van der Linden, E., and van de Velde, F. (2016). Tribological properties of rice starch in liquid and semi-solid food model systems. Food Hydrocoll. 58, 184–193. doi:10.1016/j.foodhyd.2016.02.026
Niu, W., Pu, H., Liu, G., Fang, C., Yang, Q., Chen, Z., et al. (2020). Effect of repeated heat-moisture treatments on the structural characteristics of nanocrystals from waxy maize starch. Int. J. Biol. Macromol. 158, 732–739. doi:10.1016/j.ijbiomac.2020.04.236
Osaheni, A. O., Mather, P. T., and Blum, M. M. (2020). Mechanics and tribology of a zwitterionic polymer blend: impact of molecular weight. Mater. Sci. Eng. C 111, 110736. doi:10.1016/j.msec.2020.110736
Rodriguez-Garcia, M. E., Hernandez-Landaverde, M. A., Delgado, J. M., Ramirez-Gutierrez, C. F., Ramirez-Cardona, M., Millan-Malo, B. M., et al. (2021). Crystalline structures of the main components of starch. Curr. Opin. Food Sci. 37, 107–111. doi:10.1016/j.cofs.2020.10.002
Shewan, H. M., Pradal, C., and Stokes, J. R. (2020). Tribology and its growing use toward the study of food oral processing and sensory perception. J. texture Stud. 51 (1), 7–22. doi:10.1111/jtxs.12452
Sui, Z., Yao, T., Zhao, Y., Ye, X., Kong, X., and Ai, L. (2015). Effects of heat–moisture treatment reaction conditions on the physicochemical and structural properties of maize starch: moisture and length of heating. Food Chem. 173, 1125–1132. doi:10.1016/j.foodchem.2014.11.021
Sukhija, S., Singh, S., and Riar, C. S. (2016). Effect of oxidation, cross-linking and dual modification on physicochemical, crystallinity, morphological, pasting and thermal characteristics of elephant foot yam (Amorphophallus paeoniifolius) starch. Food Hydrocoll. 55, 56–64. doi:10.1016/j.foodhyd.2015.11.003
Vilgis, T. A. (1988). Flory theory of polymeric fractals-intersection, saturation and condensation. Phys. A Stat. Mech. its Appl. 153 (3), 341–354. doi:10.1016/0378-4371(88)90228-2
Vilgis, T. A., Haronska, P., and Benhamou, M. (1994). Branched polymers in restricted geometry: flory theory, scaling and blobs. J. de Physique II 4 (12), 2187–2196. doi:10.1051/jp2:1994255
Wang, H., Zhang, B., Chen, L., and Li, X. (2016). Understanding the structure and digestibility of heat-moisture treated starch. Int. J. Biol. Macromol. 88, 1–8. doi:10.1016/j.ijbiomac.2016.03.046
Xu, Y., Cartwright, B., Advincula, L., Myant, C., and Stokes, J. R. (2021). Generalised scaling law for soft contact tribology: influence of load and asymmetric surface deformation. Tribol. Int. 163, 107192. doi:10.1016/j.triboint.2021.107192
Ye, X., Lu, F., Yao, T., Gan, R., and Sui, Z. (2016). Optimization of reaction conditions for improving nutritional properties in heat moisture treated maize starch. Int. J. Biol. Macromol. 93, 34–40. doi:10.1016/j.ijbiomac.2016.08.057
Zhang, B., Selway, N., Shelat, K. J., Dhital, S., Stokes, J. R., and Gidley, M. J. (2017). Tribology of swollen starch granule suspensions from maize and potato. Carbohydr. Polym. 155, 128–135. doi:10.1016/j.carbpol.2016.08.064
Keywords: elephant foot yam starch, modification, tribology, resistant starch, X-ray diffraction, scanning electron microscopy
Citation: Barua S, Srivastav PP and Vilgis TA (2024) Enhancing resistant starch fraction in modified elephant foot yam starch: optimizing preparation conditions and exploring tribological properties. Front. Food. Sci. Technol. 4:1466604. doi: 10.3389/frfst.2024.1466604
Received: 18 July 2024; Accepted: 05 September 2024;
Published: 30 September 2024.
Edited by:
Marcello Alinovi, University of Parma, ItalyReviewed by:
Kaiser Mahmood, Putra Malaysia University, MalaysiaEvžen Šárka, University of Chemistry and Technology in Prague, Czechia
Copyright © 2024 Barua, Srivastav and Vilgis. This is an open-access article distributed under the terms of the Creative Commons Attribution License (CC BY). The use, distribution or reproduction in other forums is permitted, provided the original author(s) and the copyright owner(s) are credited and that the original publication in this journal is cited, in accordance with accepted academic practice. No use, distribution or reproduction is permitted which does not comply with these terms.
*Correspondence: Sreejani Barua, c3JlZWphbmkzMDFAZ21haWwuY29t; Thomas A. Vilgis, dmlsZ2lzQG1waXAtbWFpbnoubXBnLmRl
†ORCID: Sreejani Barua, orcid.org/0000-0002-9681-4898, Thomas A. Vilgis, orcid.org/0000-0003-2101-7410