- 1Department of Food Science and Technology, Biological and Agricultural Engineering, University of California, Davis, Davis, CA, United States
- 2Food Nutrition and Health Team, AgResearch, Palmerston North, New Zealand
- 3Riddet Institute, Massey University, Palmerston North, New Zealand
- 4Faculty of Animal Science and Food Engineering, University of Sao Paulo, Pirassununga, SP, United States
- 5Food and Biodynamic Chemistry Laboratory, Graduate School of Agricultural Science, Tohoku University, Sendai, Miyagi, Japan
- 6High-Value Nutrition National Science Challenge, Auckland, New Zealand
- 7Department of Human Nutrition, University of Otago, Dunedin, New Zealand
Heat treatment and homogenization of milk are common processing steps intended to reduce microbial load for safe human consumption, and to avoid creaming, respectively. Although the effects of combined pasteurization and homogenization on free fatty acids (FFA) and lipid oxidation markers such as hydroperoxides, and thiobarbituric acid reactive species (TBARS) have been well characterized, their effects on primary oxidized fatty acids known as oxylipins have not yet been determined. This study aimed to determine the effects of two heat treatments: milk pasteurization [high-temperature short time, 72°C for 15 s (HTST)] and sterilization [ultra-high temperature, 135°C for 3 s (UHT)] with or without homogenization (10, 17 or 24 MPa) on FFA (%), primary (hydroperoxides and oxylipins) and secondary oxidation compounds (TBARS). Heat treatments alone significantly reduced most oxylipins compared with raw milk, but did not alter % FFA, hydroperoxide, and TBARS levels. The combination of UHT and homogenization at 24 MPa increased % FFA, hydroperoxide value, and some oxylipins, compared to raw milk and other treatments. Overall, the combination of heat treatment and homogenization significantly increased oxylipin formation compared with heat treatment alone.
1 Introduction
Thermal treatment and homogenization of milk are standard industrial processes applied to inactivate pathogens and spoilage microorganisms and to stabilize the fat emulsion against gravity separation. However, heat and mechanical treatments can adversely affect the nutritional and sensorial characteristics of milk and milk products (van Lieshout et al., 2019; Zhao et al., 2019).
Milk contains polyunsaturated fatty acids (PUFAs) which are susceptible to oxidation, giving rise to unstable hydroperoxides, as well as hydroxy, epoxy, diol, trihydroxy, and ketone fatty acid metabolites known as oxylipins (Dias et al., 2020). Oxylipins can break down into secondary oxidation products, mostly volatiles, including hexanal, pentanal, and malonaldehyde (MDA) (Valero et al., 2001) that can affect milk’s sensory properties, reduce milk quality, and impact shelf-life. Milk heat treatment can affect lipid oxidation (Allen and Hamilton, 1994; Meshref, 2008; Cappozzo et al., 2015; Ajmal et al., 2018), forming antioxidants derived from the Maillard reaction during Ultra High Temperature (UHT) treatment (Van Boekel and Walstra, 1995; Calligaris et al., 2004), inactivating natural milk antioxidants (e.g., vitamins) (Li et al., 2019) or increasing the migration of pro-oxidants such as copper to the milk fat globule membrane (Mulder and Walstra, 1974). Standard methods of pasteurization, such as batch pasteurization (63°C for at least 30 min), High-Temperature Short Time (HTST; i.e. 72°C for at least 15 s) and Ultra High Temperature (UHT; 135°C–150°C for 2–6 s) processes, do not significantly increase milk lipolysis (Shipe and Senyk, 1981; Tallini et al., 2015), but reduce to varying degrees oxylipin concentrations (Dias et al., 2020) compared with raw milk. The cause of oxylipin reduction observed after HTST and UHT is not fully understood, and it may be related to the rapid turnover of oxylipins into secondary products, which were not previously captured.
Our group has recently demonstrated that oxylipin measurements can be used as a sensitive tool to capture early lipid oxidation in HTST and UHT bovine milk (Dias et al., 2020) compared to conventional oxidation markers such as hydroperoxide and TBARS. However, the integrated impact of industrial heat treatments and homogenization pressures on these oxidation markers remains to be investigated. The goal of this exploratory study was to assess the combined effects of industrial heat treatments (HTST and UHT) and homogenization pressures (10, 17 or 24 MPa) on oxylipin concentrations, free fatty acids (% as oleic acid), hydroperoxide content, and TBARS values. Our secondary objective was to identify which of these oxidation markers would be more sensitive for detecting lipid oxidation attributed to heat and/or pressure. We hypothesized that oxylipins, measured by mass-spectrometry, would be more sensitive than conventional methods in addressing the effects of industrial heat treatments and homogenization on bovine milk lipid oxidation. Detecting lipid oxidation with a sensitive method could guide the selection of optimal processing conditions for minimizing bovine milk lipid oxidation.
2 Material and methods
2.1 Milk source and pilot-scale treatments (pasteurization, sterilization, and homogenization)
Fresh raw bovine milk was obtained from the UC Davis local dairy farm (Davis, California, United States). Raw milk was heat-treated and homogenized at the Advanced Milk Processing Laboratory (Davis, California, United States) using an indirect tubular continuous pasteurizer (UHT/HTST Lab 25 EHV Hybrid w/PLC Touchscreen Control, MicroThermics, Raleigh, NC, United States) and an in-line two-stage homogenizer (GEA Niro Soavi, NS 2006, Colombia, MD, United States) with maximum working pressure of 40–50 MPa. Raw milk was subjected to either heat treatments only (UHT or HTST) or heat treatment (UHT or HTST) followed by homogenization at different pressures.
Milk samples were preheated at 65°C and subsequently heated to 72°C for 15 s (High-Temperature Short Time, HTST) or to 135°C for 3 s (Ultra High Temperature, UHT). For the evaluation of the effects of heat treatments and homogenization on lipid oxidation, heat-treated samples were subjected to a two-stage homogenization, operated at 0.4 MPa and at 10, 17, or 24 MPa, at 55°C. Each heat treatment or heat treatment + homogenization was performed in triplicate, with a fresh batch of raw milk being used for each replicate. Raw and heat-treated samples were stored at −80°C for 4 weeks until analysis.
2.2 Hydroperoxide value determination
Lipid hydroperoxides were determined using a spectrophotometric method according to Shanta and Decker (Shantha and Decker, 1994), with the following modifications. Milk (300 μL) was mixed with a 1.5 mL isooctane/isopropanol solution (3:1, v/v) and vortexed. The mixture was centrifuged at 14,600 × g for 10 s, and 200 µL of the top layer was mixed with 2.8 mL of a methanol/n-butanol solution (2:1, v/v) and 30 µL of the NH4SCN: Fe2+ (1:1, v/v) obtained from the mixture of 3.94 M NH4SCN and 0.072 M Fe+2. The tubes were vortexed and incubated at room temperature for 20 min. Absorbance was measured at 510 nm by spectrophotometry (Thermo Scientific, GENESYS 10S UV-Vis, United States). The hydroperoxide content was calculated using a standard curve of cumene hydroperoxide (CHP) (1–6 mmol/L x10−4) and expressed as mmol of CHP/L x10−4 of milk. Hydroperoxide quantification was performed in triplicate.
2.3 Free fatty acid (FFA) content determination by titration
To measure FFA content, milk fat was extracted according to the Bligh and Dyer (1959) method, with some modifications. Approximately 100 g of milk was accurately weighed and transferred to a separatory funnel. One hundred mL of chloroform and 200 mL of methanol were added, and the mixture (milk + solvent) was manually shaken for 1 min. Subsequently, 100 mL of chloroform was added, and the mixture was shaken for 1 min. The mixture resulted in two layers, the upper layer being composed of methanol and water and the bottom layer being composed of chloroform and milk lipids. The bottom layer was separated and filtered in a filter paper with 10 mg of sodium sulfate. After filtration, the chloroform present in the filtrate was removed in a rotary evaporator at 2,991 × g for 18 h, at room temperature. The lipid fraction was collected, and the FFA content was analyzed according to the method described by Rukunudin et al. (1998), with some modifications. Approximately 3 g of lipids were weighed into a 150 mL Erlenmeyer flask, and 5.0 mL of ethanol (95%) was added. Subsequently, 2 mL of phenolphthalein (1% in 95% ethanol) was added as an indicator. The mixture was titrated with 0.013 N sodium hydroxide while shaking vigorously. Titration was stopped immediately after the first appearance of a pink color which persisted for at least 30 s. FFA quantification was performed in triplicate. The FFA content (FFA%) was calculated as percentage of oleic acid as previously described (Rukunudin et al., 1998).
2.4 Oxylipins profiling by liquid chromatography tandem mass spectrometry
A targeted lipidomics platform was used to quantify total (free + esterified) oxylipins derived from linoleic acid (LA), dihomo-gamma-linolenic acid (DGLA), arachidonic acid (ARA), α-linolenic acid (ALA), eicosapentaenoic acid (EPA) and docosahexaenoic acid (DHA) (compounds profiled are listed in the Supplementary Table S1). Oxylipins were analyzed, according to Dias et al. (2020). 600 µL of milk was weighed and spiked with 10 µL of an antioxidant solution containing 0.2 mg/mL butylated hydroxytoluene (BHT), EDTA, and triphenylphosphine (TPP) in water:methanol (1:1), and 10 µL of a surrogate standard solution containing 2 µM d11-11 (12) epoxyeicosatrienoic acid (EpETrE), d11-11,14- dihydroxyeicosatrienoic acid (DiHETrE), d4-6-keto- prostaglandin F1 α (PGF1α), d4-9-hydroxyoctadecadienoic acid (HODE), d4-leukotriene B4 (LTB4), d4-prostaglandin E 2 (PGE2), d4-thromboxane B2 (TXB2), d6-20- hydroxy arachidonic acid (HETE) and d8-5-HETE (Cayman Chemical, Ann Arbor, MI, United States).
Samples were hydrolyzed by adding 600 µL of 0.25 M sodium carbonate solution in methanol/water (1:1 v/v) and heating for 30 min at 60°C. Subsequently, samples were cooled at room temperature and acidified with 75 µL of acetic acid. The pH was confirmed in a representative sample to be between 4 and 6 using Litmus paper. Ultrapure water (4,725 µL) was added and the samples were centrifuged for 10 min at 388 × g. The supernatant was loaded onto Oasis HLB solid phase extraction (SPE) columns (60 mg, 3 cm cartridges; Waters, Milford, MA, United States), pre-washed with one volume of ethyl acetate and two volumes of methanol and conditioned with two volumes of SPE buffer containing 0.1% acetic acid and 5% methanol in ultrapure water (Wu et al., 2016; Richardson et al., 2017). The columns were washed with two volumes of SPE buffer and dried under vacuum for 20 min. Oxylipins were eluted from the column with 0.5 mL methanol followed by 1.5 mL of ethyl acetate. Samples were dried under nitrogen and reconstituted in 100 µL methanol. Reconstituted samples were filtered using centrifugal filter tubes (UltraFree-MC VV Centrifugal Filter; 0.1 µm; EMD Millipore, Bedford, MA, United States) and transferred to LC-MS/MS vials.
Oxylipins were analyzed on a 1290 Infinity ultra-high-pressure-liquid chromatography (UHPLC) coupled to a 6460 QqQ MS/MS with electrospray ionization (ESI) via Jet Stream Technology (Agilent Technologies, Santa Clara, CA, United States). Oxylipins were separated using a ZORBAX Eclipse Plus C18 column (ID: 2.1 mm; length: 150 mm; particle size; 1.8 µm; Agilent Technologies, Santa Clara, CA, United States). The mobile phase A consisted of 0.1% acetic acid in ultrapure water and the mobile phase B of acetonitrile: methanol (80: 15) with 0.1% acetic acid. The following gradient program was used: Mobile phase B was increased from 35% to 40% between 0 and 3 min, to 48% from 3 to 4 min, to 60% from 4 to 10 min, to 70% from 10 to 20 min, and to 85% from 20 to 24 min. Mobile phase B was held at 85% from 24 to 24.5 min, increased to 99% from 24.5 to 24.6 min, held at 99% for 1.4 min, decreased to 35% from 26 to 26.1 min and held at 35% for 28 min. The mobile phase flow was maintained at 0.3 mL/min from 0 to 3 min, reduced to 0.25 mL/min between 3 and 24.6 min, increased to 0.35 mL/min from 24.6 to 27.3 min and then reduced to 0.3 mL/min at 28 min. The injection volume was 10 µL per sample.
Oxylipins were analyzed in negative electrospray ionization (ESI) mode. The drying gas temperature and flow rates were 300°C and 10 L/min, respectively. The sheath gas temperature and flow rates were 350°C and 11 L/min, respectively. The nebulizer pressure was 35 psi. Dynamic multiple reaction monitoring (MRM) was used to capture oxylipins using the precursor and product ions masses and fragmentor and collision energy values summarized in Supplementary Table S2.
2.5 Thiobarbituric acid reactive substance analysis
Thiobarbituric acid reactive substances (TBARS) were used to test the formation of malondialdehyde and other aldehydes, which are secondary products of fatty acid oxidation. This oxidative product reacts with thiobarbituric acid and creates a reddish/pink color, which can be read spectrophotometrically as described by Vyncke (1970) and Sørensen and Jørgensen (1996), with the following modifications. Milk (10 g) was homogenized with 15 mL of a homogenizing solution (7.5% trichloroacetic acid, 0.1% Propyl 3,4,5-Trihydroxy-benzoate, and 0.1% Ethylenediaminetetraacetic acid disodium salt) in a vortex for 30 s. The mixture was filtered in a 12.5-mm qualitative filter paper, and 5 mL of the filtrate was mixed with 5 mL of a 0.02 M 2-thiobarbituric acid (TBA) aqueous solution. Samples were incubated in a water bath at 95°C for 40 min and then cooled down in cold water. The absorbance was measured at 532 and 600 nm by spectrophotometry (Thermo Scientific, GENESYS 10S UV-Vis, United States). A blank consisting of 5 mL of the same homogenizing solution plus 5 mL of TBA solution was used as the reference control. The results were calculated based on the standard curve of 1,1,3,3-tetraethoxypropane (TEP; approximately 97%) and expressed as mg of malonaldehyde (MDA)/kg milk. TBARS measurements were performed in triplicate.
2.6 Statistical analysis
Statistical analyses were performed using GENSTAT 18th (International, 2018). A two-way ANOVA was used to determine the effects of heat treatments (HTST, UHT) and homogenization pressures (10, 17, or 24 MPa) on FFA, hydroperoxide, oxylipins and TBARS values in bovine milk. Post-hoc multiple comparison adjusted p-values were performed using the TUKEY method (Haynes, 2013). A significance level of p < 0.05 was used. Transformation to a natural logarithm was applied to oxylipins data to reduce skewness.
3 Results
3.1 Effects of heat treatment and homogenization pressure on free fatty acid content (% FFA)
The analysis of FFA content, using acid titration, showed that pasteurization and sterilization alone did not alter % FFA at 10, 17, or 24 MPa (p-value>0.1) (Table 1). Homogenization at 24 MPa and at 55°C temperature significantly increased % FFA in HTST (0.67% ± 0.1%) and UHT (0.65% ± 0.1%) milk compared to raw milk (0.39% ± 0.03%) (p-value<0.001) (Table 1).
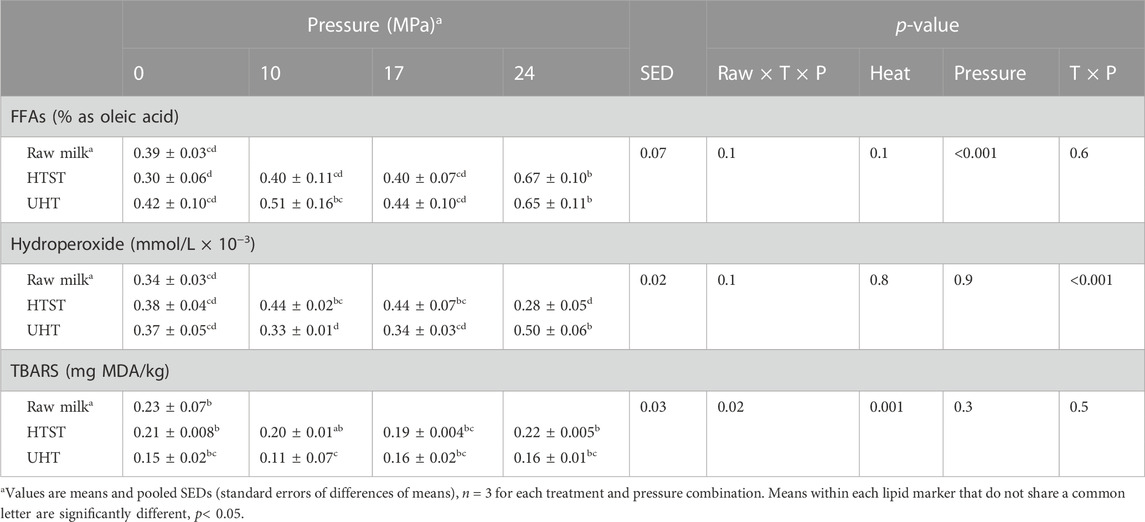
TABLE 1. Effect of heat treatments and homogenization pressures on bovine milk free fatty acid content (%FFA as oleic acid), hydroperoxide content (mmol/L × 10−3) and TBARS concentration (mg MDA/kg), mean ± standard deviation.
3.2 Effects of heat treatment and homogenization pressure on hydroperoxide and thiobarbituric acid reactive substances (TBARs)
After the UHT treatment, the hydroperoxide content increased with increasing homogenization pressure (24 MPa, 0.50 ± 0.06 mmol/L × 10−3), while in the HTST treatment, a variable behavior was observed, remaining constant at 10 and 17 MPa and decreasing at 24 MPa (0.28 ± 0.05 mmol/L × 10−3), compared to raw milk (0.34 ± 0.03 mmol/L × 10−3) (Table 1).
There were no significant changes in TBARS values following heat treatment.
3.3 Oxylipins
Significant effects of heat and homogenization pressures on log-transformed oxylipin concentrations are reported in Table 2. Corresponding means and standard deviations as well as raw data of all oxylipins detected are reported in Supplementary Tables S2, S3, respectively. Statistical analysis of the log-transformed data showed that HTST and UHT resulted in a significant reduction of nine [9 HODE, 13-HODE, 15(S)-HETrE, 11 (12)-EpETrE, 8 (9)-EpETrE, 11,12-DiHETrE, 8,9-DiHETrE, 5-HEPE, 17-HDoHE] out of seventeen oxylipins detected, compared with raw milk. However, the homogenization pressures evaluated did not alter the majority of detected oxylipins, except for ARA-derived [11 (12)-EpETrE, 8 (9)-EpETrE, 11,12-DiHETrE, 8,9-DiHETrE, 6-keto-PGF1α], LA-derived [9-HODE, 13-HODE) and DHA-derived (17-hydroxydocosahexaenoic acid (HDoHE)] oxylipins, which significantly increased at higher homogenization pressures, irrespective of the upstream heat treatment employed (Table 2).
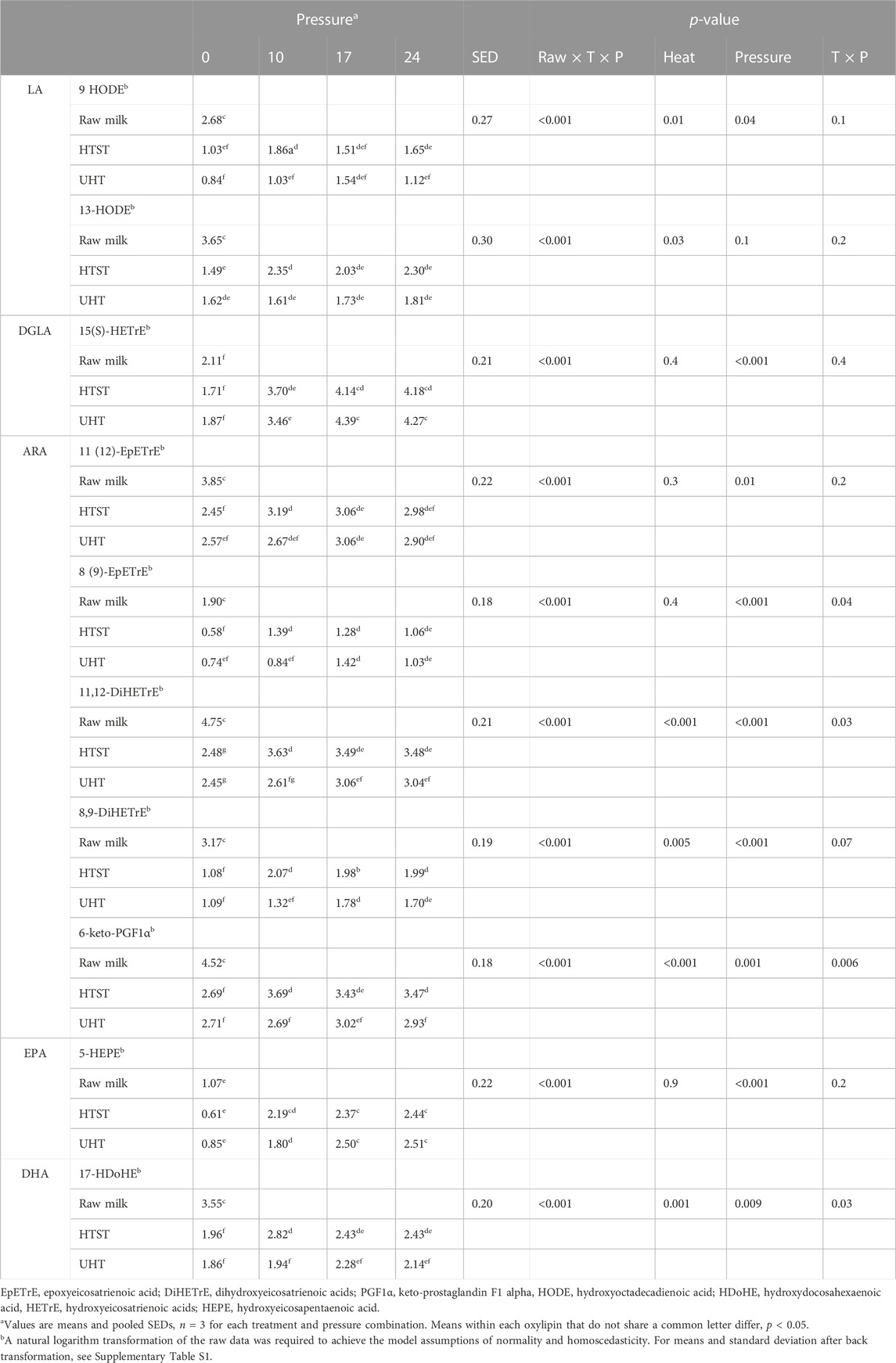
TABLE 2. Significant effects of heat treatments (HTST, and UHT) and homogenization pressures (10, 17 or 24 MPa) on the log-transformed concentration of metabolites derived from arachidonic acid (C20:4; ARA), linoleic acid (C18:2; LA), docosahexanoic acid (C22:6; DHA), dihomo-γ-linoleic acid (C20:3; DGLA) and eicosapentaenoic acid (C20:5; EPA) in milk.
4 Discussion
The main finding of this preliminary study is that compared to raw milk, HTST and UHT, alone, significantly decreased the concentration of oxylipins derived from LA, ARA, and DHA, whereas the combined impact of UHT and homogenization at 24 mPa significantly increased the concentration of FFAs, hydroperoxide, and the oxylipins 15(S) HETrE and 5-HEPE, compared to non-homogenized milk. These findings suggest that oxylipins, especially, 15(S) HETrE and 5-HEPE, can be used, in combination with % markers, to elucidate the effects of industrial heat treatments and homogenization pressures on bovine milk lipid oxidation.
In agreement with previous studies (Allen and Hamilton, 1994; Meshref, 2008; Cappozzo et al., 2015; Ajmal et al., 2018; Dias et al., 2020), pasteurization and thermal treatments had no significant effects on % FFAs and hydroperoxide compared to raw milk. FFAs are a product of lipolysis, which can be catalyzed by milk natural lipases or by thermoresistant microbial lipases when milk is stored (Deeth and Fitz-Gerald, 2006). Heat treatment can inactivate the milk native lipoprotein lipase (LPL; EC 3.1.1.34) (Deeth and Fitz-Gerald, 2006) and cause whey proteins to denature and interact with the milk fat globule membrane components, which may protect the triacylglycerol core from lipolysis and oxidation (Ye et al., 2004). On note, the initial FFA content in raw milk is higher than generally described in the literature (National Food Safety Standard Infant Formula, 2018) and may have affected the concentration of some oxidation products. High levels of %FFA at baseline compared to other studies may be due to milk handling and herd genetic background (Wiking et al., 2019).
An increase in % FFAs, was observed in samples that were heat-treated and homogenized at 24 MPa. Homogenization causes significant compositional changes by disrupting milk fat globules and causing losses of original membrane components (such as phospholipids, and proteins) (Kim and Jimenez-Flores, 1995; Corredig and Dalgleish, 1996; Corredig and Dalgleish, 1997). These modifications result in reduced fat globule size and increased surface area, which might increase their susceptibility to lipolysis and oxidation (Kim et al., 2020). Some studies reported that homogenized milk is less susceptible to oxidation than raw milk because, after homogenization, casein micelles adsorb onto the new milk fat droplets where they act as antioxidants (Dunkley et al., 1962; Lee et al., 2015). This is consistent with studies showing that increased milk homogenization pressures (30–90 MPa) increased protein load on the milk fat globule surface (Cano-Ruiz and Richter, 1997). On the other hand, it has been reported that most of the intrinsic milk lipoprotein lipase is associated with micellar casein (Anderson, 1982), which may promote lipolysis in milk fat globules containing adsorbed caseins in non-heat treated milk (Sundheim and Bengtsson-Olivecrona, 1987). Here we showed that UHT-treated samples homogenized at 24 MPa had an increase in the hydroperoxide value and in 15(S)- hydroxyeicosatrienoic acids (HETrE) and 5-hydroxyeicosapentaenoic acid (HEPE) oxylipins compared to raw milk and other treatments. The higher concentration of % FFAs, hydroperoxides, and some oxylipins, but not TBARS, in UHT samples homogenized at 24 MPa reflect lipolysis (Shipe and Senyk, 1981) and lipid oxidation at these temperature and pressure conditions.
To the best of our knowledge, this is the first study to demonstrate the integrated effect of heat treatments and homogenization pressures on oxylipin concentrations in bovine milk. We showed that the oxylipins derived from ARA, LA, and DHA, were lower in heat-treated samples compared to raw milk, which supports our previous study showing that heat treatments (HTST and UHT) reduced oxylipins compared to raw milk (Dias et al., 2020). The cause of reduced oxylipin concentrations for HTST and UHT milk remains unclear and further mechanistic studies are needed to identify the factors (enzymatic activity, auto-oxidation, and or heat) that mediate the production and degradation of oxylipins. It is possible that the high temperatures involved in UHT and HTST results in rapid oxylipin degradation into secondary volatiles not measured in this study.
Homogenizing HTST and UHT milk increased the concentration of several oxylipins [11,12-DiHETrE, 8,9-DiHETrE, 15(S)-HETrE, 5-HEPE] compared to heated and non-homogenized samples. Other oxylipins [8 (9)-EpETrE, 6-keto-PGF1α, 9-HODE, 13-HODE, 17-HDoHE] only increased in homogenized HTST samples compared to non-homogenized samples. These results support our observations that homogenization, in combination with HTST and UHT heat treatments, may expose milk fat globules to pro-oxidant compounds such as copper (Foley and King, 1977) and increase exposure of milk PUFAs to oxygen or decrease the concentration of heat-labile anti-oxidant compounds (e.g., vitamins) (Li et al., 2019). It should be pointed out that many of the oxylipins that increased after homogenization are enzymatically made, suggesting enhanced accessibility of oxygenase enzymes to their fatty acid substrates following homogenization.
In conclusion, UHT followed by homogenization increased %FFA, hydroperoxide content, and oxylipins, whereas temperature alone reduced oxylipins without altering other markers of oxidation. The combination of heat treatments (HTST and UHT) and homogenization at 24 MPa increased % FFAs, whereas both heat treatment and homogenization at all pressures (10–24 MPa) increased oxylipin concentrations compared to raw milk. Oxylipin measurements seem to be more sensitive to temperature and pressure changes than other classic markers of lipid oxidation. Future studies investigating the production of secondary volatile products of oxylipin degradation and the progression of these changes during storage in relation to microbial stability and sensory attributes may better inform on the long-term impact of industrial heat treatment and homogenization on milk shelf-life.
Data availability statement
The original contributions presented in the study are included in the article/Supplementary Material, further inquiries can be directed to the corresponding authors.
Author contributions
CT and JB designed the study. CT and WM, performed the experiment, analyzed the data, and wrote the paper. AC and GO performed pilot-plant experiments and YO performed the oxylipin analysis. JB and AT overlooked the experimental design, and revised and edited the manuscript. All authors listed have made a substantial, direct, and intellectual contribution to the work and approved it for publication.
Funding
This research was supported by the UC-Davis-FAPESP SPRINT grant, USDA National Institute of Food and Agriculture, Hatch/Multi-State project [1023517; de Moura Bell] and start-up funds from the Food Science and Technology and Biological and Agricultural Engineering Departments at the University of California at Davis, and the National Institutes of Health awards. This research was also supported by the USDA National Institute of Food and Agriculture, Hatch/Taha (project #1008787), the Center for Advanced Processing and Packaging Studies (CAPPS Award # 735818-0), the Riddet Institute, a New Zealand Centre of Research Excellence, and the Strategic Science Investment Fund (SSIF) from the New Zealand Ministry of Business, Innovation and Employment. YO is a recipient of a fellowship from the Japan Society for the Promotion of Science Core-to-Core Program, A. (Advanced Research Networks entitled “Establishment of international agricultural immunology research-core for a quantum improvement in food safety”).
Conflict of interest
The authors declare that the research was conducted in the absence of any commercial or financial relationships that could be construed as a potential conflict of interest.
Publisher’s note
All claims expressed in this article are solely those of the authors and do not necessarily represent those of their affiliated organizations, or those of the publisher, the editors and the reviewers. Any product that may be evaluated in this article, or claim that may be made by its manufacturer, is not guaranteed or endorsed by the publisher.
Supplementary material
The Supplementary Material for this article can be found online at: https://www.frontiersin.org/articles/10.3389/frfst.2023.1027418/full#supplementary-material
References
Ajmal, M., Nadeem, M., Imran, M., and Junaid, M. (2018). Lipid compositional changes and oxidation status of ultra-high temperature treated Milk. Lipids health Dis. 17, 227. doi:10.1186/s12944-018-0869-3
Allen, J. C., and Hamilton, R. J. (Editors) (1994). Rancidity in foods. 2nd. Edn. London: Chapman & Hall, 1–22.
Anderson, M. (1982). Factors affecting the distribution of lipoprotein lipase activity between serum and casein micelles in bovine milk. J. Dairy Res. 49, 51–59. doi:10.1017/s0022029900022123
Calligaris, S., Manzocco, L., Anese, M., and Nicoli, M. C. (2004). Effect of heat-treatment on the antioxidant and pro-oxidant activity of milk. Int. Dairy J. 14, 421–427. doi:10.1016/j.idairyj.2003.10.001
Cano-Ruiz, M., and Richter, R. (1997). Effect of homogenization pressure on the milk fat globule membrane proteins. J. Dairy Sci. 80, 2732–2739. doi:10.3168/jds.s0022-0302(97)76235-0
Cappozzo, J. C., Koutchma, T., and Barnes, G. (2015). Chemical characterization of milk after treatment with thermal (HTST and UHT) and nonthermal (turbulent flow ultraviolet) processing technologies. J. Dairy Sci. 98, 5068–5079. doi:10.3168/jds.2014-9190
Corredig, M., and Dalgleish, D. G. (1996). Effect of different heat treatments on the strong binding interactions between whey proteins and milk fat globules in whole milk. J. Dairy Res. 63, 441–449. doi:10.1017/s0022029900031940
Corredig, M., and Dalgleish, D. G. (1997). Studies on the susceptibility of membrane-derived proteins to proteolysis as related to changes in their emulsifying properties. Food Res. Int. 30, 689–697. doi:10.1016/s0963-9969(98)00018-0
Deeth, H. C., and Fitz-Gerald, C. (2006). Lipolytic Enzymes and Hydrolytic Rancidity in Advanced Dairy Chemistry Volume 2 Lipids. Editors P. F. Fox and P. L. H. McSweeney (Springer, Boston, MA). doi:10.1007/0-387-28813-9_15
Dias, F. F. G., Augusto-Obara, T. R., Hennebelle, M., Chantieng, S., Ozturk, G., Taha, A. Y., et al. (2020). Effects of industrial heat treatments on bovine milk oxylipins and conventional markers of lipid oxidation. Prostagl. Leukot. Essent. Fat. Acids 152, 102040. doi:10.1016/j.plefa.2019.102040
Dunkley, W., Franklin, J., and Pangborn, R. (1962). Influence of homogenization, copper, and ascorbic acid on light-activated flavor in milk. J. Dairy Sci. 45, 1040–1044. doi:10.3168/jds.s0022-0302(62)89557-5
Foley, J., and King, J. (1977). Influence of pasteurization before and after separation of cream on the oxidative stability of ripened cream butter. J. Food Prot. 40, 480–483. doi:10.4315/0362-028X-40.7.480
Kim, H.-H., and Jimenez-Flores, R. (1995). Heat-induced interactions between the proteins of milk fat globule membrane and skim milk. J. Dairy Sci. 78, 24–35. doi:10.3168/jds.S0022-0302(95)76612-7
Kim, S. C., Yun, S. Y., Ahn, N. H., Kim, S. M., and Imm, J. Y. (2020). Effect of homogenization pressure on plasmin activity and mechanical stress-induced fat aggregation of commercially sterilized ultra high temperature milk during storage. Food Sci. Anim. Resour. 40, 734–745. doi:10.5851/kosfa.2020.e48
Lee, D., Albenberg, L., Compher, C., Baldassano, R., Piccoli, D., Lewis, J. D., et al. (2015). Diet in the pathogenesis and treatment of inflammatory bowel diseases. Gastroenterology 148, 1087–1106. doi:10.1053/j.gastro.2015.01.007
Li, Y. H., Wang, W. J., Zhang, F., Shao, Z. P., and Guo, L. (2019). Formation of the oxidized flavor compounds at different heat treatment and changes in the oxidation stability of milk. Food Sci. Nutr. 7, 238–246. doi:10.1002/fsn3.874
Meshref, A. (2008). Effect of heating treatments, processing methods and refrigerated storage of milk and some dairy products on lipids oxidation. Pak. J. Nutr. 7, 118–125.
Mulder, H., and Walstra, P. (1974). The milk fat globule. Wageningen, Netherlands: Centre for Agricultural Publishing and Documentation.
National Food Safety Standard Infant Formula (2018). National Health commission of the people’s Republic of China. Available at: https://members.wto.org/crnattachments/2018/SPS/CHN/18_4984_00_x.pdf.
Richardson, C. E., Hennebelle, M., Otoki, Y., Zamora, D., Yang, J., Hammock, B. D., et al. (2017). Lipidomic analysis of oxidized fatty acids in plant and algae oils. J. Agric. food Chem. 65, 1941–1951. doi:10.1021/acs.jafc.6b05559
Rukunudin, I., White, P., Bern, C., and Bailey, T. (1998). A modified method for determining free fatty acids from small soybean oil sample sizes. J. Am. Oil Chemists' Soc. 75, 563–568. doi:10.1007/s11746-998-0066-z
Shantha, N. C., and Decker, E. A. (1994). Rapid, sensitive, iron-based spectrophotometric methods for determination of peroxide values of food lipids. J. AOAC Int. 77, 421–424. doi:10.1093/jaoac/77.2.421
Shipe, W., and Senyk, G. (1981). Effects of processing conditions on lipolysis in milk. J. Dairy Sci. 64, 2146–2149. doi:10.3168/jds.s0022-0302(81)82821-4
Sørensen, G., and Jørgensen, S. S. (1996). A critical examination of some experimental variables in the 2-thiobarbituric acid (TBA) test for lipid oxidation in meat products. Z. für Lebensm. Forsch. 202, 205–210. doi:10.1007/bf01263541
Sundheim, G., and Bengtsson-Olivecrona, G. (1987). Hydrolysis of bovine and caprine milk fat globules by lipoprotein lipase. Effects of heparin and of skim milk on lipase distribution and on lipolysis. J. Dairy Sci. 70, 2467–2475. doi:10.3168/jds.S0022-0302(87)80313-2
Tallini, R. A., Gennari, A., Monteiro, B. W., Lehn, D. N., and Souza, C. F. V. (2015). Effects of pasteurization and ultra-high temperature processes on proximate composition and fatty acid profile in bovine milk. Am. J. Food Technol. 10, 265–272. doi:10.3923/ajft.2015.265.272
Valero, E., Villamiel, M., Miralles, B., Sanz, J., and Martınez-Castro, I. (2001). Changes in flavour and volatile components during storage of whole and skimmed UHT milk. Food Chem. 72, 51–58. doi:10.1016/s0308-8146(00)00203-x
Van Boekel, M., and Walstra, P. (1995). Effect of heat treatment on chemical and physical changes to milkfat globules. Brussels, Belgium: International Dairy Federation.
van Lieshout, G. A., Lambers, T. T., Bragt, M. C., and Hettinga, K. A. (2019). How processing may affect milk protein digestion and overall physiological outcomes: A systematic review. Crit. Rev. Food Sci. Nutr. 60, 2422–2445. doi:10.1080/10408398.2019.1646703
Vyncke, W. (1970). Direct determination of the thiobarbituric acid value in trichloracetic acid extracts of fish as a measure of oxidative rancidity. Fette, Seifen, Anstrichm. 72, 1084–1087. doi:10.1002/lipi.19700721218
Wiking, L., Bjerring, M., Løkke, M. M., Løvendahl, P., and Kristensen, T. (2019). Herd factors influencing free fatty acid concentrations in bulk tank milk. J. Dairy Res. 86, 226–232. doi:10.1017/S0022029919000190
Wu, J., Gouveia-Figueira, S., Domellöf, M., Zivkovic, A. M., and Nording, M. L. (2016). Oxylipins, endocannabinoids, and related compounds in human milk: Levels and effects of storage conditions. Prostagl. Other Lipid Mediat 122, 28–36. doi:10.1016/j.prostaglandins.2015.11.002
Ye, A., Singh, H., Taylor, M. W., and Anema, S. (2004). Interactions of whey proteins with milk fat globule membrane proteins during heat treatment of whole milk. Le. Lait. 84, 269–283. doi:10.1051/lait:2004004
Keywords: milk pasteurization, homogenization, oxylipins, hydroperoxide values, TBARS
Citation: Thum C, Cirelli A, Otoki Y, Ozturk G, Taha AY, McNabb WC, Roy NC and Leite Nobrega de Moura Bell JM (2023) Concentration of milk oxylipins after heat and homogenization treatments. Front. Food. Sci. Technol. 3:1027418. doi: 10.3389/frfst.2023.1027418
Received: 25 August 2022; Accepted: 08 May 2023;
Published: 26 May 2023.
Edited by:
Essam Hebishy, University of Lincoln, United KingdomReviewed by:
Manuela Hernandez-Herrero, Autonomous University of Barcelona, SpainMichael Lewis, University of Reading, United Kingdom
Copyright © 2023 Thum, Cirelli, Otoki, Ozturk, Taha, McNabb, Roy and Leite Nobrega de Moura Bell. This is an open-access article distributed under the terms of the Creative Commons Attribution License (CC BY). The use, distribution or reproduction in other forums is permitted, provided the original author(s) and the copyright owner(s) are credited and that the original publication in this journal is cited, in accordance with accepted academic practice. No use, distribution or reproduction is permitted which does not comply with these terms.
*Correspondence: Juliana Maria Leite Nobrega de Moura Bell, amRlbW91cmFiZWxsQHVjZGF2aXMuZWR1