- 1College of Art, Beijing Sport University, Beijing, China
- 2College of Sports Science, Tianjin Normal University, Tianjin, China
- 3Department of Kinesiology, College for Health, Community, and Policy, The University of Texas at San Antonio, San Antonio, TX, United States
- 4School of Public Health, Jilin Medical University, Jilin, China
- 5Center for Mobility and Rehabilitation Engineering Research, Kessler Foundation, West Orange, NJ, United States
- 6Rutgers New Jersey Medical School, Rutgers, The State University of New Jersey, New Brunswick, NJ, United States
Objective: The current review was aimed to determine the effectiveness of mental imagery training (MIT) on the enhancement of maximum voluntary muscle contraction (MVC) force for healthy young and old adults.
Data sources: Six electronic databases were searched from July 2021 to March 2022. Search terms included: “motor imagery training,” “motor imagery practice,” “mental practice,” “mental training,” “movement imagery,” “cognitive training,” “strength,” “force,” “muscle strength,” “performance,” “enhancement,” “improvement,” “development,” and “healthy adults.”
Study selection and data extraction: Randomized controlled trials of MIT in enhancing muscle strength with healthy adults were selected. The decision on whether a study met the inclusion criteria of the review was made by two reviewers independently. Any disagreements between the two reviewers were first resolved by discussion between the two reviewers. If consensus could not be reached, then it would be arbitrated by a third reviewer.
Data synthesis: Twenty-five studies including both internal MIT and external MIT were included in meta-analysis for determining the efficacy of MIT on enhancing muscle strength and 22 internal MIT were used for subgroup analysis for examining dose-response relationship of MIT on MVC.
Results: MIT demonstrated significant benefit on enhancing muscle strength when compared with no exercise, Effect Size (ES), 1.10, 95% confidence interval (CI), 0.89–1.30, favoring MIT, but was inferior to physical training (PT), ES, 0.38, 95% CI, 0.15–0.62, favoring PT. Subgroup analysis demonstrated that MIT was more effective for older adults (ES, 2.17, 95% CI, 1.57–2.76) than young adults (ES, 0.95, 95% CI, 0.74–1.17), p = 0.0002, and for small finger muscles (ES, 1.64, 95% CI, 1.06–2.22) than large upper extremity muscles (ES, 0.86, 95% CI, 0.56–1.16), p = 0.02. No significant difference was found in the comparison of small finger muscles and large lower extremity muscles, p = 0.19 although the ES of the former (ES, 1.64, 95% CI, 1.06–2.22) was greater than that of the later (ES, 1.20, 95%, 0.88–1.52).
Conclusion: This review demonstrates that MIT has better estimated effects on enhancing MVC force compared to no exercise, but is inferior to PT. The combination of MIT and PT is equivalent to PT alone in enhancing muscle strength. The subgroup group analysis further suggests that older adults and small finger muscles may benefit more from MIT than young adults and larger muscles.
Introduction
Motor imagery (MI) is a phenomenon of internally perceiving or simulating an actual motor action without physically executing it by using motor-related parts of the brain as its substrate (Decety, 1996; Krüger et al., 2020). That is, MI is an active cognitive process during which the representation of a specific action is internally reproduced within working memory without any overt motor activities. Imaging studies show that MI increases activity in cortical areas that overlap with cortical areas activated during the actual performance of motor acts, providing evidence for the common coding between perception and action (Hinshaw, 1991; Decety, 1996; Decety and Grezes, 1999; Hétu et al., 2013). Repetitive application of MI in the acquisition of motor skills is MI training (MIT), which is also known as mental practice or action-imagery practice (Driskell et al., 1994; Simonsmeier et al., 2021; Dahm et al., 2022). MIT has shown to be effective in motor skill acquisition (Feltz and Landers, 1983; Driskell et al., 1994; Land et al., 2016; Lindsay et al., 2021). Additionally, MIT has also been reported to be beneficial to the elderly population, not only in motor performance but cognitive function as well (Altermann et al., 2014). Furthermore, studies (Shelton and Mahoney, 1978; Gould et al., 1980; Tod et al., 2015) also demonstrated that applying MI to or “Psyching-Up” during muscle contractions could improve muscle force outcome.
To examine the effect of MIT on muscle strength, Yue and Cole (1992) conducted one of the first investigations showing the efficacy of MIT enhancing maximal voluntary muscle force. The study by Yue and Cole (1992) included three groups (MIT, physical training, and control groups) and examined not only the MIT effect on improving muscle strength but also neuromuscular mechanisms underlying the MIT-induced strength increase. The young-healthy subjects trained their left hypothenar (little finger abductor) muscles for 4 weeks by either producing real maximal isometric contractions of the muscle (physical training group, PT), imagining producing these same isometric contractions (motor imaging training group, MIT), or having no training of the muscle at all (no-exercise control group, CTRL). After the 4-weeks training, the maximal little finger abduction force increased significantly for the MIT group and the PT group (22 and 30%, respectively), but not for the CTRL group (3.7%). Since MI is a process to internally simulate an actual motor action without physically executing it, it is not expected to have any changes in muscle fiber hypertrophy through repetitive rehearsal of MI of the maximum voluntary muscle contraction (MVC) of the muscle. Muscle fiber hypertrophy has been shown to be one of the potential factors accounting for the muscle strength increases after physical resistance training (Jones et al., 2008; Krzysztofik et al., 2019). Alternatively, the authors (Yue and Cole, 1992) attributed the increase of muscle strength after the MIT to the increase of brain-to-muscle signal, that might lead to the recruitment of large motor units and an increase in discharge rate of originally recruited motor units in an MVC. Muscle force is controlled by the recruitment and discharge rate of motor units. Moreover, the central nervous system can increase muscle force by increasing the number of active motor units and the firing rate of the originally recruited motor units (Duchateau and Enoka, 2011; Enoka and Pearson, 2014). It has been shown that large-sized motor units cannot be voluntarily activated by the untrained young (Yue et al., 2000) and old adults (Yue et al., 1999; Rozand et al., 2020). However, as Yue and Cole (1992) suggested, MIT could significantly enhance brain-to-muscle drive that in turn, might help recruit the larger motor units, which were otherwise inactive in an untrained state, and drive the originally activated motor units to higher discharge rate, therefore, leading to greater muscle force. This finding has significant application in rehabilitation medicine (Jackson et al., 2001) since weak patients or frail older adults, who find it difficult or unsafe to participate in conventional strength training programs, may be able to enhance their muscle strength via their mind.
Since the seminal work by Yue and Cole (1992), the efficacy of MIT on MVC muscle force has been tested with different muscles and types of muscle contractions (Ranganathan et al., 2004; Sidaway and Trzaska, 2005; Wright and Smith, 2009; de Ruiter et al., 2012), in exploring the functional role of internal and external MIT in muscle strength enhancement (Yao et al., 2013; Montuori et al., 2018), on determining its effect on muscle strength in aging population (Darvishi et al., 2013; Mamone, 2013; Jiang et al., 2016; Goudarzian et al., 2017) and on its role in maintaining or enhancing the MVC force for the individuals with temporary immobilization of the upper extremity or anterior cruciate ligament (Yue et al., 1996; Slimani et al., 2016). There are two common types of mental imagery—internal and external imagery. In internal imagery (IMI; also known as kinesthetic or first-person imagery), a person imagines or mentally creates the feeling of performing the exercise from within the body (i.e., from a first-person perspective). For example, mental strength training using internal imagery emphasizes that the subject generates a similar feeling as he/she felt during a physical MVC (Ranganathan et al., 2004; Sidaway and Trzaska, 2005; Yao et al., 2013). In external imagery (EMI; also known as third-person visual imagery), the person sees or visualizes performing the task from outside the body—similar to watching oneself in a mirror performing an exercise (Herbert et al., 1998). Performing IMI generates significantly more physiological responses (i.e., heart rate, blood pressure, and respiration rate) compared to doing EMI (Ranganathan et al., 2004) and is superior to EMI for elevating MVC-related cortical potentials on scalp locations over primary motor (M1) and supplementary motor cortices and for enhancing muscle strength (Yao et al., 2013).
Overall, accumulating evidence suggests that MIT is effective in enhancing muscle strength. While the majority of studies in the area presented the beneficial effect of MIT in enhancing muscle strength, contradictory findings were also demonstrated from a few studies, including a recent meta-analysis study (Manochio et al., 2015). In their study, Manochio et al. (2015) revealed an non-beneficial effect of MIT over no-exercise CTRL [ES (effect size) = −0.10, 95% CI (confidential interval) = −1.46 to 1.24]. However, as Paravlic et al. (2018) argued, the contradictory finding from study Manochio et al.’s (2015) could be due to its lack of statistical power since only four studies were included in the meta-analysis while many other relevant studies were available. It is possible that Manochio et al. (2015) only included studies with post-innervation values (PIV) and excluded studies with change-from-baseline values (CBV) due to the concerns about biased outcome (Cuijpers et al., 2017). However, it has been suggested that an analysis based on CBV could be more efficient and powerful than comparison of PIV, as it removes a component of between-person variability from the analysis (Durg et al., 2015; Deeks et al., 2022). It is further stated that it is statistically legitimate for a meta-analysis to include studies with both CBV and PIV (da Costa et al., 2013; Deeks et al., 2022; Higgins et al., 2022).
A more recent and outstanding systematic review and meta-analysis study by Paravlic et al. (2018), which included more relevant articles (13 articles published either in English or German by April 2017), demonstrated medium effect size (ES = 0.72, 95% CI = 0.45–0.97), based on the revised Cohen’s d effect size chart (Sawilowsky, 2009), in favor of the MIT groups over the no-exercise CTRL groups. The meta-analysis in study Paravlic et al.’s (2018) generated four forest plots, MIT vs. no-exercise CTRL, PT vs. no-exercise CTRL, MIT vs. PT, and MIT + PT vs. PT. In addition, the Meta-regression analysis showed that two out of six training volume variables, the number of repetitions per training session (p = 0.01) and per study (p = 0.05), predicted the effects of MIT on muscle strength enhancement and additional dose–response analysis further showed that the largest effects were found after the use of the greatest number of repetitions (Paravlic et al., 2018). Due to the limited number of available studies in old adults at the time, age was not included as a moderator variable in their meta-analysis (Paravlic et al., 2018). In an attempt to overcome this limitation, Meier (2021) conducted a systematic review and meta-analysis including both young and old adults. Consistent with the study of Paravlic et al. (2018), the meta-analysis by Meier (2021) also revealed a medium effect size (ES = 0.76, 95% CI = 0.53–0.99) for the young adults when the MIT groups were compared with the no-exercise CTRL groups. However, the MIT did not show clear beneficial effect for the old adults in study Meier’s (2021). A qualitative visual analysis of the studies’ results for the elderly group suggests a non-significant effect since the “gray rhombus,” reflecting the elderly group’s point estimate of the treatment effect, touched the line of no effect (ES = 0.53, 95% CI = −0.03–1.10) (Meier, 2021). The results of study Meier’s (2021) on older adults was surprising since previous studies (Sale, 1986, 1987; Semmler and Enoka, 2000; Simoneau et al., 2006; Mamone, 2013) suggest that elderly should be able to benefit from MIT as much as, if not more than, younger adults due to the greater neural deficit for the elderly that gives a larger potential for mental training-related strength improvements. The results of Meier’s study on older adults could be due to its use of PIV only and lack of the number of studies included. Indeed, all the three studies with older adults included in Meier’s study reported positive increase from the baseline in MVC force after the MIT, ranging from 8.7 to 18.6% (Table 2 in Meier’s study). Thus, the main objective of the current study was to undertake a systematic review and a meta-analysis of randomized controlled studies with either PIV or CBV available, published in peer-reviewed journals or unpublished dissertation or thesis, investigating the beneficial and adverse effects of motor imagery training (MIT) on enhancing muscle strength for both healthy young and older adults. It was hypothesized that both young and older adults should benefit from MIT to enhance their muscle strength. The hypothesis was developed based on the facts that imagery abilities are sustained with aging (Saimpont et al., 2013) and sarcopenia, the age-related decrease in lean muscle mass (Siparsky et al., 2014), makes it feasible to augment old adults’ muscle strength by increasing net excitation of the motoneurons, and increasing activation of prime movers and inhibition of antagonists (Sale, 1987) as the results of MIT.
Research methods
The current review followed the checklists and guidance on systematic reviews described in PRISMA Statement 2020 (Page et al., 2021).
Eligibility criteria
The PICOS’s (population, intervention, comparison, outcome, and study design) recommendation was adopted to determine the eligibility (Amir-Behghadami and Janati, 2020).
Inclusion criteria
(1) Population: Healthy male and female adults aged at or above 18 years; (2) Intervention: The MIT could be given as an independent intervention or combined with PP. The MIT had to be given at least three training sessions in three separate days and included at least one CTRL group (no-excise group) and/or one physical training (PT) group; (3) Comparison: maximal voluntary contraction (MVC) force was compared between (a) the intervention type (i.e., MIT vs. no-exercise CTRL, MIT vs. PT, and MI + PT vs. PT alone), (b) the age groups (young and old adults), and (c) the muscle groups trained (larger muscle groups vs. smaller muscle groups); (4) Outcome: The outcome measures could be either PIV (the post-innervation values) or CBV (the change-from-baseline values) of MVC force. If both PIV and CBV are available, CBV would be selected for further analysis [see Higgins et al. (2022) for the rationals behind the decision, Deeks et al. (2022)]; and (5) Study design: randomized controlled trials published in peer-reviewed journals or non-published dissertation/thesis before March 30, 2022 that tested efficacy of MIT on muscle strength improvement.
Exclusion criteria
(1) studies written in languages other than English; (2) non-randomized studies or studies with unhealthy populations; and (3) studies without enough information for obtaining means and standard deviations of the two comparison groups (e.g., MIT and CTRL) to calculate ESs and/or determine dose–response relationship.
Search sources and screening strategy
Computer-aided search was performed by the two researchers (XL and WY) using PubMed/Medline, ERIC, Web of Science, Google Scholar, ScienceDirect, and ProQuest. Key words used were: “motor imagery training,” “motor imagery practice,” “mental practice,” “mental training,” “movement imagery,” “strength,” “force,” “muscle strength,” “performance,” “enhancement,” “improvement,” and “healthy adults.” To find additional articles, the authors hand-searched reference lists of obtained articles (reference and author tracking). The search was terminated on March 15, 2022.
To insure effective and accurate search, the titles and abstracts were first analyzed by the two reviewers based on the pre-determined inclusion and exclusion criteria. Then, the full texts of the remaining papers that met the inclusion criteria were retrieved and thoroughly reviewed by the two reviewers to determine which articles to include in the meta-analysis. Any studies for which suitability was unclear were then reviewed by the two reviewers (XL and WY) again and a decision was made through discussion between the two reviewers. If consensus could not be reached, then it would be arbitrated by a third reviewer (GY).
Selection process
As described above, the decision on whether a study met the inclusion criteria of the review was made by two reviewers independently (XL and WY). Any disagreements between the two reviewers were first resolved by discussion between the two reviewers. If consensus could not be reached, then it would be arbitrated by a third reviewer (GY). See Figure 1 for a more visible flow of selection process.
Data extraction
Data were extracted by one reviewer (XL) into a data extraction spreadsheet that was developed based on the Template for Intervention Description and Replication checklist (Hoffmann et al., 2014). Data extraction was checked by a second reviewer (WY). The following data were extracted: study design; number of groups; sample size in each group; description of the intervention; randomization; frequency of MIT sessions; duration or number of trials of each MIT session; the length of the entire MIT intervention; and baseline and post-intervention values. When the manuscript did not present data needed for further analysis, the authors were contacted for this information. For the studies that data were shown in figures, the WebPlotDigitizer software (version 4.5, August, 2021; Ankit Rohatgi; Pacifica, CA, USA) was used to extract the necessary data.
Risk of bias (methodological quality) assessment
Two reviewers (XL and WY) independently assessed the quality of all included studies using the PEDro criteria scores (de Morton, 2009). Three ratings were determined based on the scores: poor (< 2), moderate (3–5), or high (6–10) quality trials (de Morton, 2009). Any disagreements between the two reviewers were first resolved by discussion between the two reviewers. If consensus could not be reached, then it would be arbitrated by a third reviewer (GY).
Reporting bias
Funnel plot was used to visualize evidence of publication bias and Egger’s regression test was performed to provide further statistical evidence of publication bias if any. The authors gave their views on the potential sources of the asymmetry and on the implications of the missing results (Page et al., 2022).
Synthesis
Studies for the synthesis were selected based on the pre-determined inclusion and exclusion criteria and the outcomes of the selected studies were grouped based on their innervation types (i.e., MIT, PT, MIT + PT, and CTRL). The meta-analyses were performed using R-Studio (1.4.1717-3, “Juliet Rose” for macOS), a language and environment for statistical computing and graphics. Main packages used in the study were “meta,” “rmeta,” and “metafor.” Meta-analysis of the different continuous measures of MVC force, presenting results as point estimates and 95% confidence intervals (CI), was undertaken by one reviewer (WY). SMD (standardized mean differences) was used in the study since the outcomes in the study included both PIV (post-innervation values) and CBV (change-from-baseline values). Heterogeneity was assessed through visual inspection of forest plots and the calculation of the chi-square and I2 statistics. Subgroup analysis was conducted to examine the similarities and/or differences in MIT effect on MVC force between the two age groups (i.e., young vs. old adults) and three muscle groups (i.e., lower extremity, upper extremity, and finger muscles). In addition, the dose-response relationship of MIT effect on MVC force was also examined. Due to the concern of multi-collinearity among training variables such as training periods and total training trials and non-linear-relationship between estimated effects (dependent variable) and training variables (independent variables) that might pose a big threat to the validity of applying multiple meta-regression (Harrer et al., 2022), the dose-response relationship was examined by detecting the estimated effects of each training volume variable on the MVC force, instead of applying multiple meta-regression. The moderators in the dose-response analysis were the four training variables (training periods in weeks, training sessions per week, training trials per session, and total training trials per study). The Q-test based on the overall subgroup results was used to determine if the subgroup differed significantly (Harrer et al., 2022).
Effect measures
Once again, meta-analysis of the different continuous measures of MVC force, presenting results as point estimates and 95% confidence intervals (CI), was undertaken by one reviewer (WY) and SMD (standardized mean differences) was used in the study since the outcomes in the study included both PIV (post-innervation values) and CBV (change-from-baseline values).
Results
Study selection
A total of 1,025 articles were identified by the online database literature search (Figure 1). Following the removal of duplicates and the elimination of articles based on title and abstract screening, 65 studies remained. An evaluation of the remaining 65 studies was conducted independently by the two reviewers (XL and WY). Following the final screening process, 24 articles involving 25 studies were included in the systematic review and meta-analysis.
Risk of bias (methodological quality) assessment
PEDro scores are displayed in Table 1. Overall, all the included studies were rated with high quality (PEDro scores of 6.00). All the included studies received points for the following items: baseline indicators, obtaining measures of at least one key outcome from more than 85% of the subjects, allocation of all subjects in either a treatment or control condition, and reporting the results of statistical comparison between groups and measures of variability. On the other hand, all the included studies failed to satisfy the following items: concealed allocation, and blinded assessors/experimenters. All but one (Zijdewind et al., 2003) failed to report blinding of subjects.
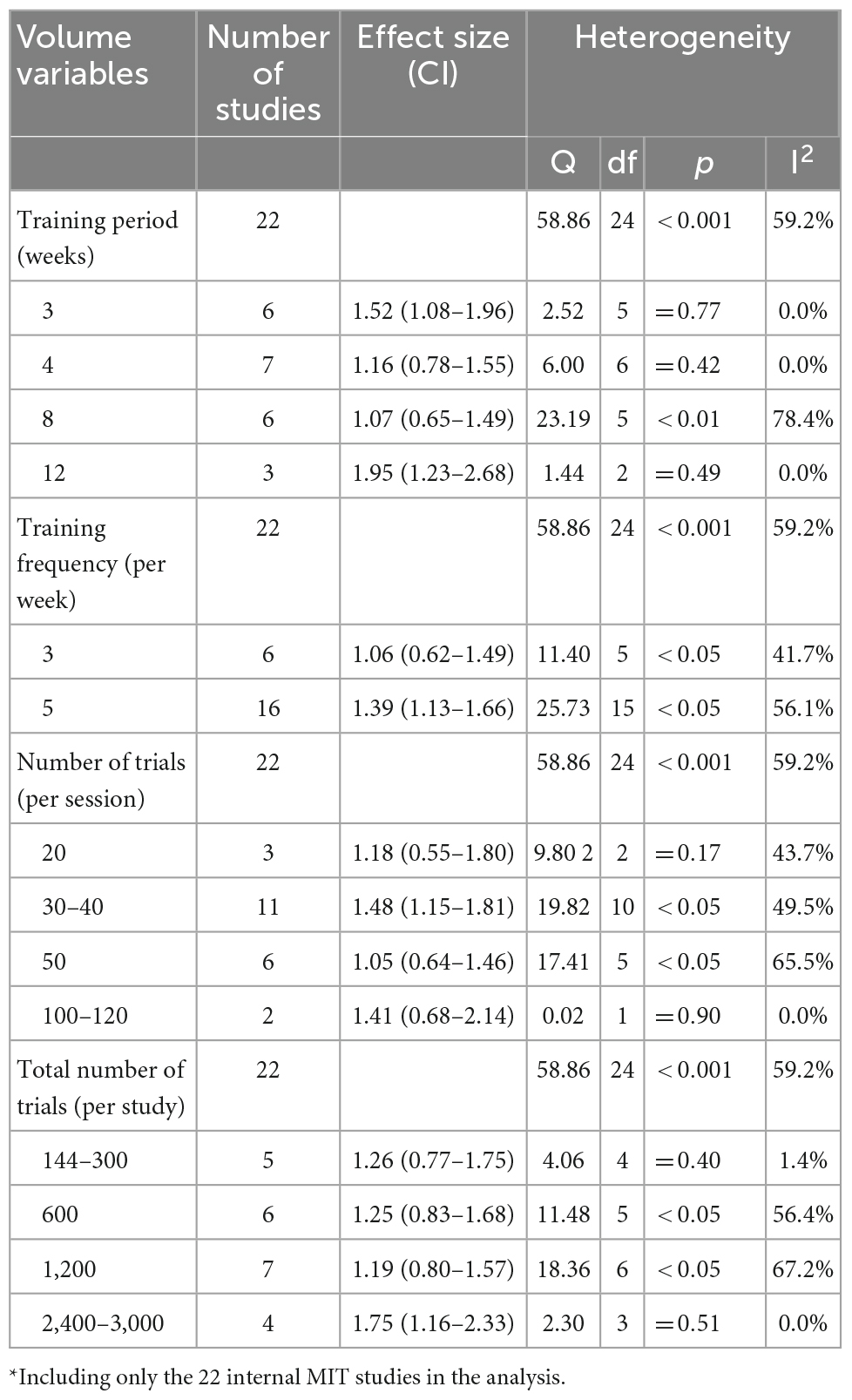
Table 2. Training volume variables with the effect size (ES): Motor imagery training* vs. no-exercise control.
Reporting bias
The funnel plot (Figure 2) suggests asymmetry for the publications. The Egger’s regression test provided further statistical evidence to show bias for the publications, p < 0.001.
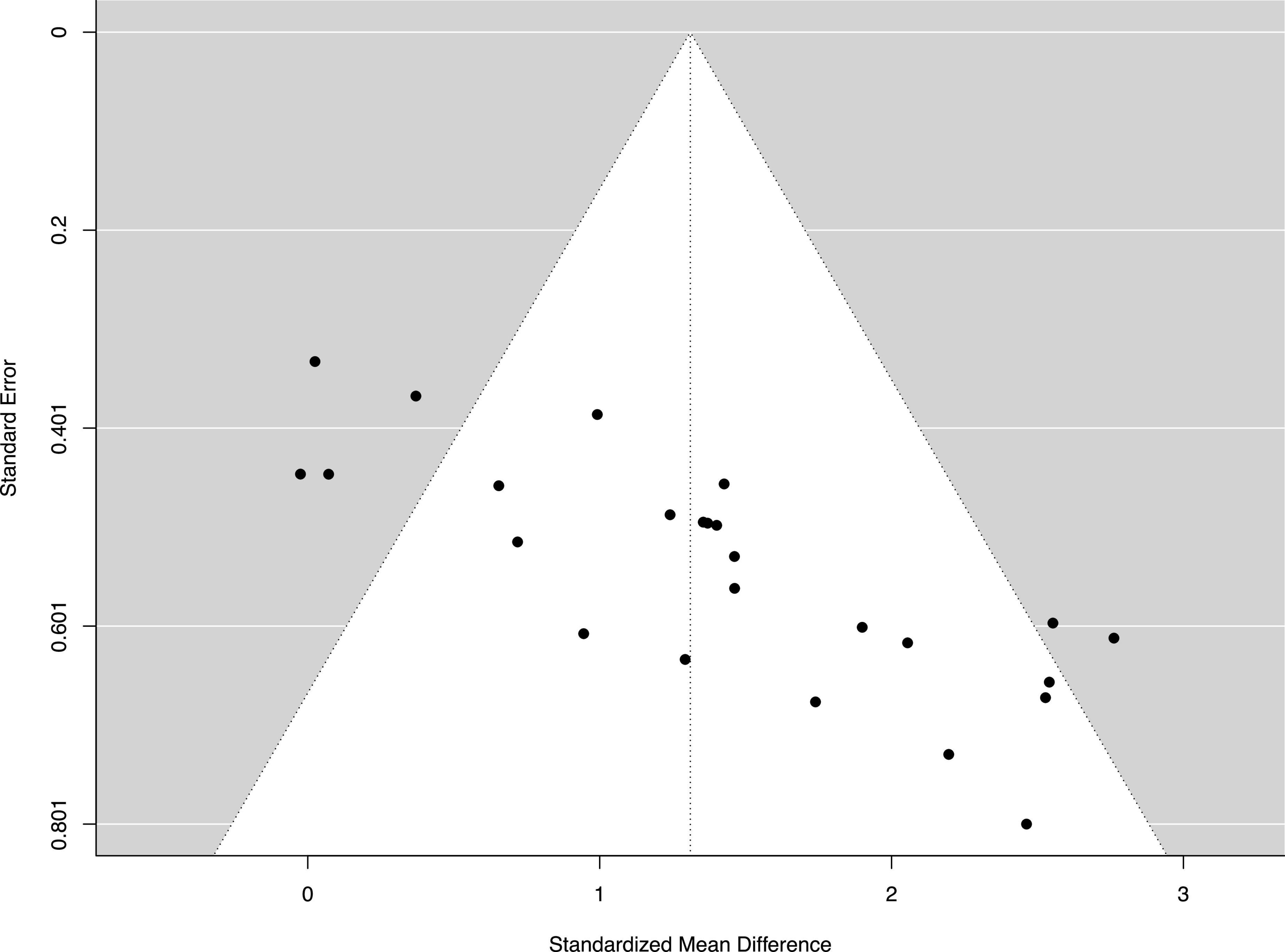
Figure 2. Funnel plot of the standard differences in means vs. standard errors. The aggregated standard difference in means is the random effects mean effect size weighted by the degrees of freedom study characteristics.
After the online database literature search, 23 eligible articles with 25 MIT groups were selected (Table 1). Table 1 presents details of each included article regarding sample, measures, and key results. The sample size of the MIT groups in the included studies ranged from 6 to 18, three studies with a sample size of 6 (Leung et al., 2013; Yao et al., 2013; Jiang et al., 2017), two studies with a sample size of 8 (Ranganathan et al., 2004; Bahari et al., 2011), two studies with a sample size of 9 (Lebon et al., 2010; Grôspretre et al., 2017), nine studies with a sample size of 10 (Yue and Cole, 1992; Sidaway and Trzaska, 2005; Fontani et al., 2007; Shackel and Standing, 2007; Wright and Smith, 2009; de Ruiter et al., 2012; Darvishi et al., 2013; Jiang et al., 2016; Bouguetoch et al., 2021), three studies with a sample size of 12 (Cornwall et al., 1991; Reiser and Munzert, 2011; Goudarzian et al., 2017), one study with a sample size of 15 (Mamone, 2013), three studies with a sample size of 16 (Smith et al., 2003; Niazi et al., 2014; Alenezi, 2018), and one study with a sample size of 18 (Herbert et al., 1998). All the selected studies included a non-exercise and/or a non-imagery control group.
Fourteen selected studies included an additional PT group (Yue and Cole, 1992; Herbert et al., 1998; Smith et al., 2003; Ranganathan et al., 2004; Sidaway and Trzaska, 2005; Fontani et al., 2007; Shackel and Standing, 2007; Wright and Smith, 2009; de Ruiter et al., 2012; Darvishi et al., 2013; Leung et al., 2013; Mamone, 2013; Niazi et al., 2014; Jiang et al., 2016; Goudarzian et al., 2017). Four studies examined the effect of MIT on older subjects (Darvishi et al., 2013; Mamone, 2013; Jiang et al., 2016; Goudarzian et al., 2017). Most of the included studies investigated the effects of internal MIT, but three of the studies either examined both internal MIT and external MIT (EMIT) (Wright and Smith, 2009; Yao et al., 2013) or examined the effect of EMIT only (Herbert et al., 1998). The selected studies varied in training volume, ranging from 1 to 12 weeks, 3 to 7 sessions per week, and 6 to 120 trials per session (Table 1). In addition, the selected studies varied also in muscles examined, nine studies trained lower extremity (LE) muscles (Cornwall et al., 1991; Sidaway and Trzaska, 2005; Shackel and Standing, 2007; de Ruiter et al., 2012; Niazi et al., 2014; Goudarzian et al., 2017; Grôspretre et al., 2017; Alenezi, 2018; Bouguetoch et al., 2021), ten studies trained upper extremity (UE) muscles (Herbert et al., 1998; Ranganathan et al., 2004; Fontani et al., 2007; Wright and Smith, 2009; Bahari et al., 2011; Leung et al., 2013; Mamone, 2013; Yao et al., 2013; Jiang et al., 2016, 2017), and four studies trained finger muscles (Yue and Cole, 1992; Smith et al., 2003; Ranganathan et al., 2004; Darvishi et al., 2013).
Overall findings of effects of motor imagery training on maximal voluntary contraction
MIT effects compared with no training (CTRL)
Overall estimated effects
Compared to the CTRL, the estimated effect of MIT was moderately beneficial for enhancing MVC force (ES = 1.10, 95% CI 0.89–1.30) (Figure 3).
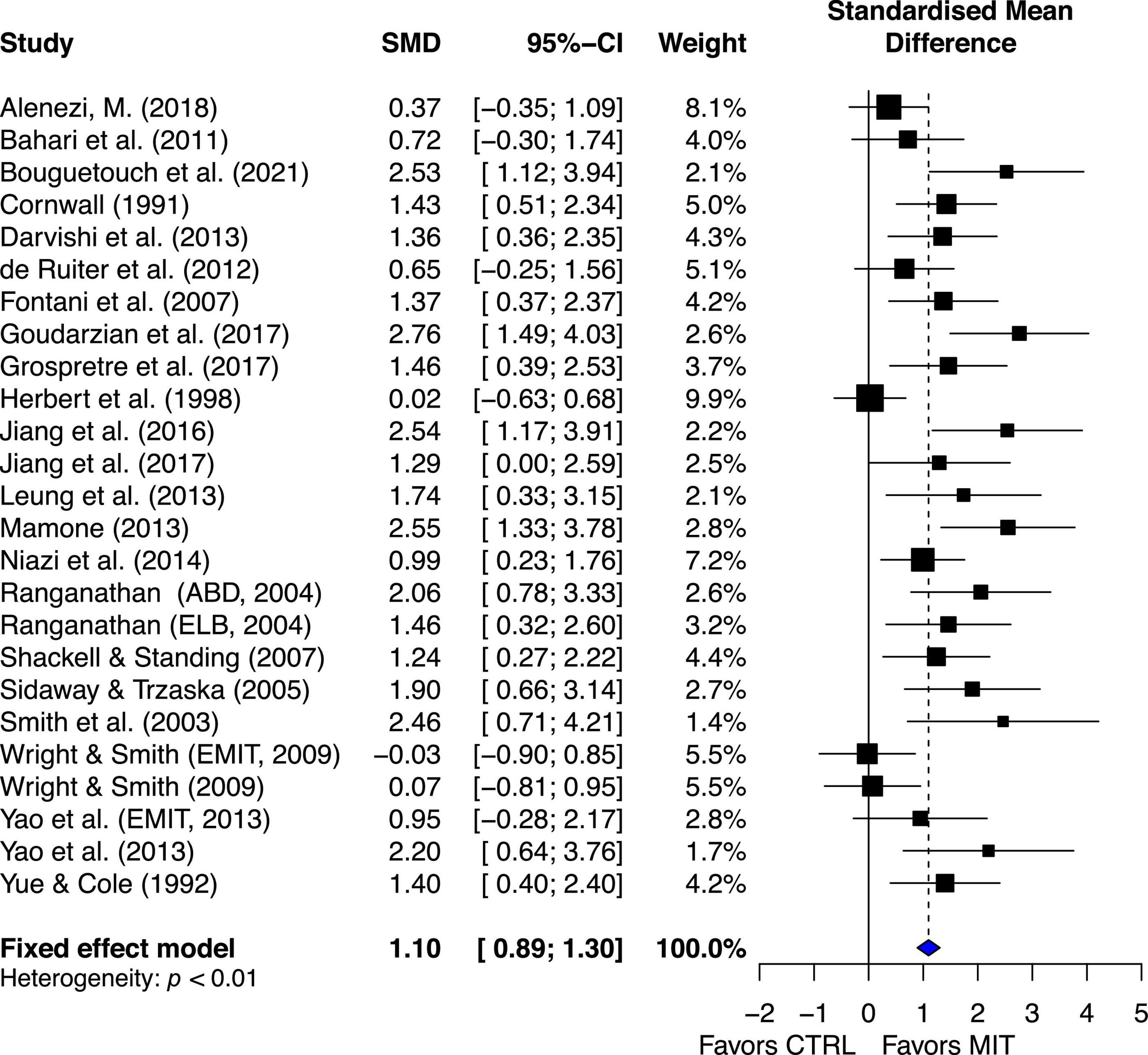
Figure 3. Effects on maximal muscle strength: Mental imagery training (MIT) vs. control group (CTRL).
The chi-square test for heterogeneity was significant (Q24 = 58.86, p < 0.01). The I2 value was 59%, indicating medium heterogeneity (Higgins et al., 2003). The results suggest there was study variability/heterogeneity among all the included studies.
Aging subgroup estimated effects
The estimated effect of MIT was more beneficial for the elder group than for the younger group in enhancing muscle strength, ES = 2.17, 95% CI = 1.57–2.76, and ES = 0.95, 95% CI = 0.74–1.17, respectively. Quantitative analysis provided further evidence to show significant statistical difference between the two groups, Q1, 23 = 14.15, p = 0.0002. A qualitative analysis of the younger-group studies’ results suggests between-study variability (Figure 4).
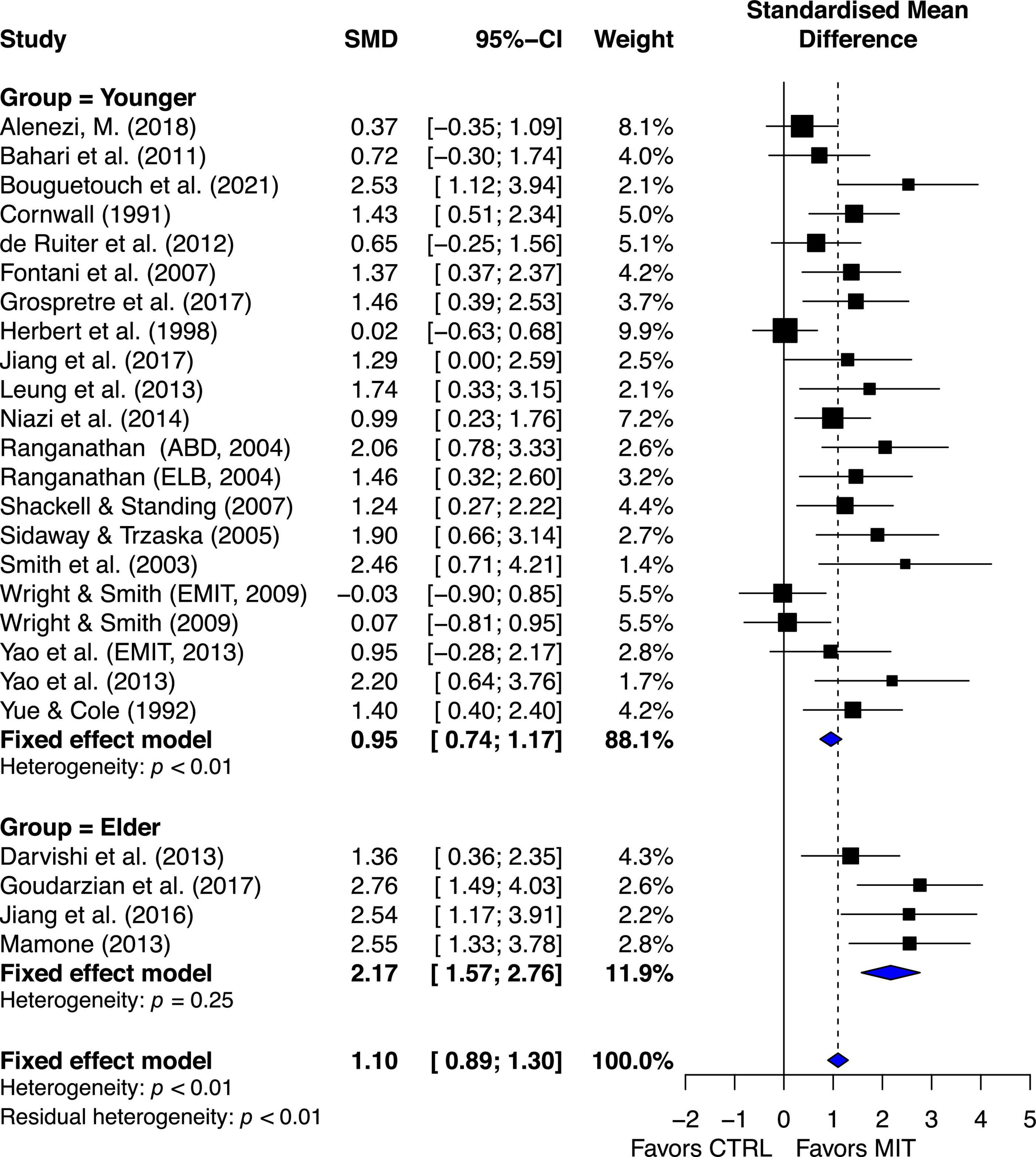
Figure 4. Aging subgroup’s effects on maximal muscle strength: Mental imagery training (MIT) vs. control group.
The chi-square test for heterogeneity was significant at a level of < 0.01. The I2 value was 50.8%, indicating medium heterogeneity (Higgins et al., 2003). These quantitative results suggest there was study variability/heterogeneity among the younger-group studies. In contrast, the chi-square test for heterogeneity in the elder-group studies was non-significant, p = 0.25. The I2 value was 26%, indicating small heterogeneity (Higgins et al., 2003). The results suggest that there was little between-study variability or heterogeneity in the aging studies.
Muscle subgroup estimated effects
The estimated effects of MIT for lower extremity (LE), upper extremity (UE), and finger muscles are ES = 1.20, 95% CI = 0.88–1.52; ES = 0.86, 95% CI = 0.56–1.16; and ES = 1.64, 95% CI = 1.06–2.22, respectively (Figure 5).
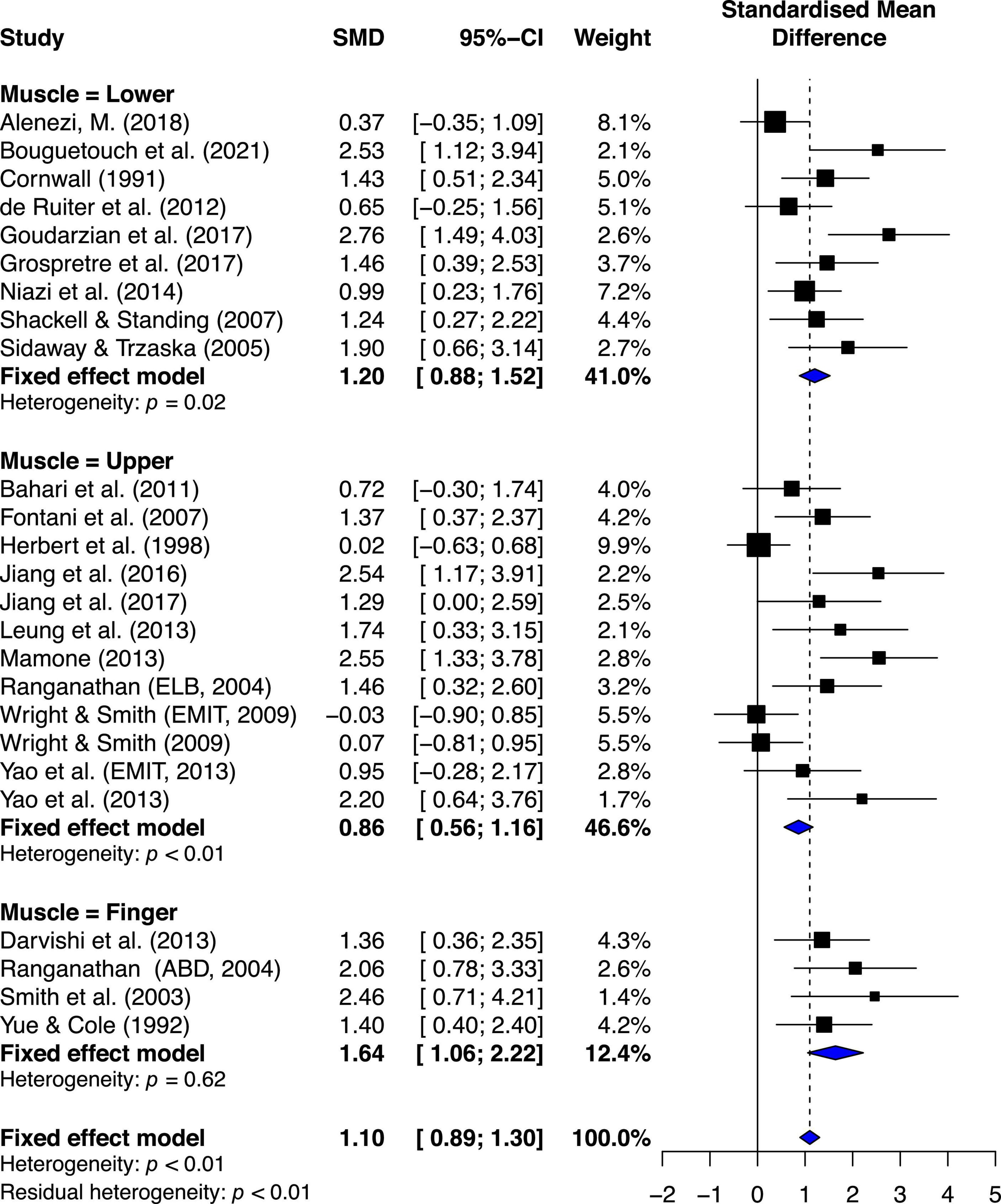
Figure 5. Muscle subgroup’s effects on maximal muscle strength: Mental imagery training (MIT) vs. control group.
Quantitative analysis indicated significant statistical difference between the groups, Q2, 22 = 6.07, p = 0.0481. Further quantitative analyses of two-group comparisons on the effects of MIT indicated the only significant comparison between finger muscle and UE muscle, Q1,14 = 5.38, p = 0.02, and no other significant differences between finger muscle and LE muscle (Q1,11 = 1.65, p = 0.19), and between LE muscle and UE muscle (Q1,19 = 2.30, p = 0.12). A qualitative analysis of the younger-group studies’ results suggests between-study variability (see Figure 3). The chi-square test for heterogeneity was significant at a level of < 0.01. The I2 value was 50.8%, indicating medium heterogeneity (Higgins et al., 2003).
Motor imagery training effects compared with physical practice
The estimated effect for the 15 studies demonstrated small beneficial effect (ES = 0.38, 95% CI 0.15–0.62), favoring PT. Although the qualitative visual analysis of the overall estimated effect for the 15 studies suggests possible heterogeneity (Figure 6), the chi-square test for heterogeneity was not significant, Q14 = 13.85, P = 0.46. The I2 value was 0%, indicating little heterogeneity (Higgins et al., 2003). These quantitative results suggest there was no significant study variability/heterogeneity among the studies that included both PT and MIT groups.
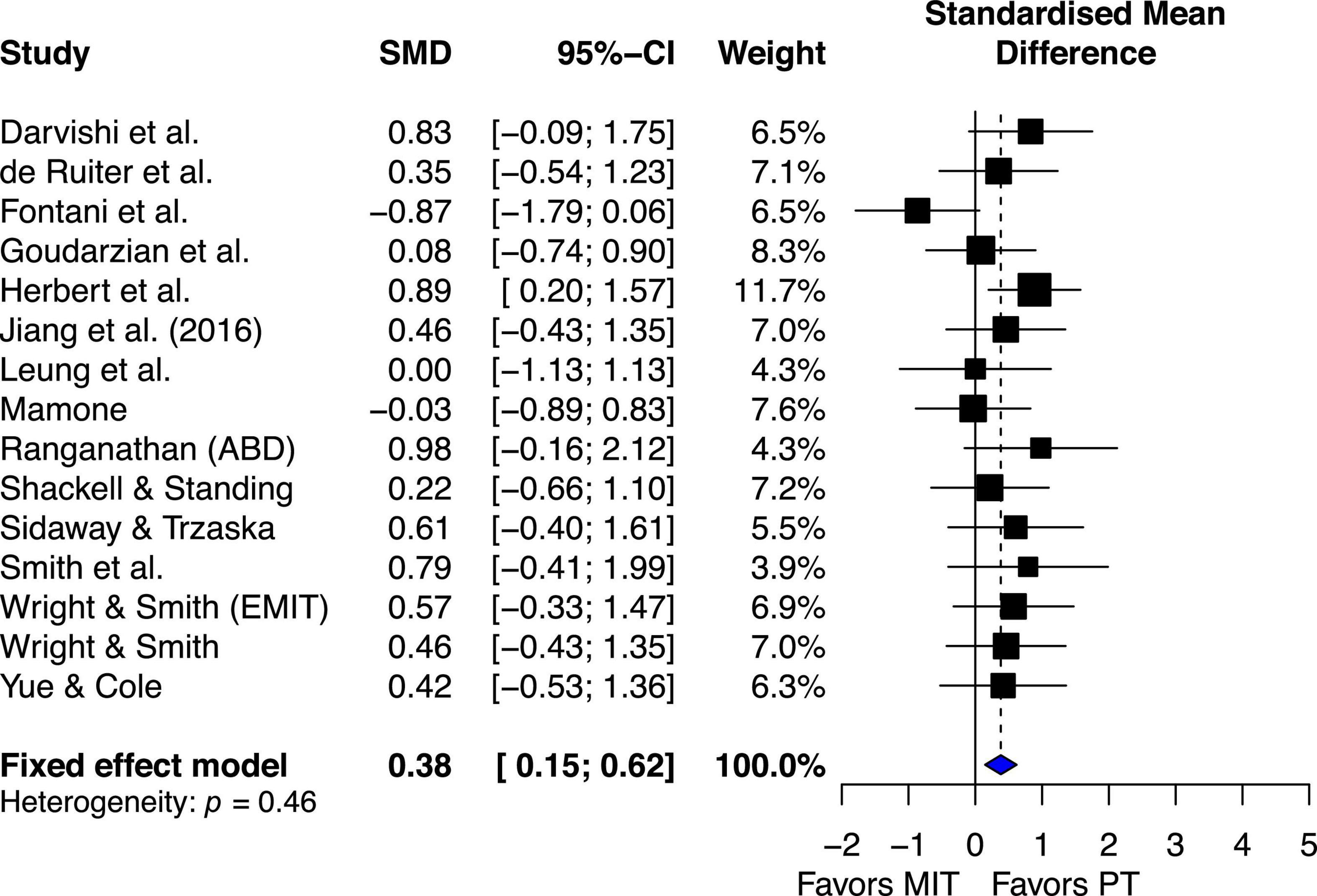
Figure 6. Effects on maximal muscle strength: Mental imagery training (MIT) vs. physical training (PT).
MIT combined with PT (MITPT) vs. PT only
Overall, the estimated effect of MITPT in enhancing MVC force was almost same as PT’s estimated effect (ES = 0.04, 95% CI −0.32–0.39) (Figure 7).
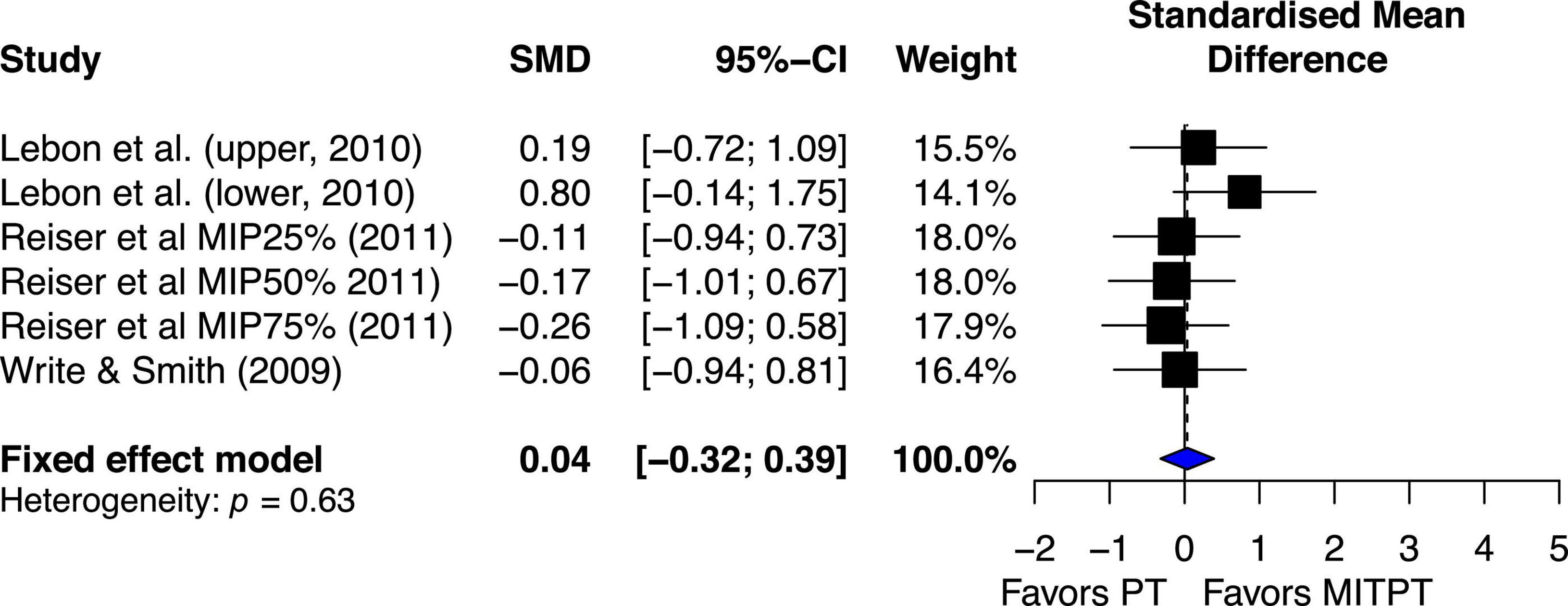
Figure 7. Effects on maximal muscle strength: Mental imagery training combined with physical training (MITPT) vs. physical training (PT).
The chi-square test for heterogeneity was significant (Q5 = 3.48, p = 0.62). The I2 value was 0%, indicating no heterogeneity (Higgins et al., 2003).
Dose-response relationship of MIT effects on MVC force
The dose-response relationship was examined by detecting the estimated effects of each training volume variables on the MVC force (Table 2). The training period for the included studies ranged from 1 to 12 weeks, median = 4. The largest mean effect (ES = 1.95, 95% CI 1.23–2.68) was associated with a period of 12-weeks training (three studies only). The training sessions per week ranged from 2 to 7 (only one study with seven sessions per week), median = 5. The largest effect (ES = 1.39, 95% CI 1.13–1.66) was associated with the five sessions per week training frequency (16 studies). The number of trials per session ranged from 6 to 120, median = 32. The largest mean effect (ES = 1.48, 95% 1.15–1.81) was observed at the 30–40 trials per session (11 studies). The total trials per study ranged from 144 to 3,000, median = 700. The largest mean effect (ES = 1.75, 95% 1.16–2.33) was associated with the 3,000-trials per study (four studies). Although the differences in the values among the groups in each training variable were noticeable, they were not statistically significant, Q(3) = 5.66, p = 0.13; Q(1) = 1.68, p = 0.19; Q(3) = 2.80, p = 0.42; and Q(3) = 2.65, p = 45, for Training period, Training sessions per week, number of trials per session, and total number of trials per study, respectively.
Discussion
The main purpose of this study was to conduct a systematic review and a meta-analysis of randomized controlled studies to examine the beneficial and adverse effects of motor imagery training (MIT) on enhancing muscle strength for both healthy young and older adults. The results of current study showed that MIT generates moderate improvements in muscle strength for both young and older adults (Figure 4). While the current study clearly presents beneficial effect of MIT, PT overperformed MIT in enhancing muscle strength (Figure 6) which is consistent with the findings of the previous studies (Paravlic et al., 2018; Meier, 2021). When the estimated effect of MIT was analyzed in subgroups based on the trained muscle, the results (Figure 5) indicated that finger muscles (ES = 1.64) benefited significantly more from MIT than the upper extremity muscles (ES = 0.86) but had no significant difference compared to the lower extremity muscles (ES = 1.20). In addition, a meta-regression analysis with the training volumes showed that only the number of sessions per week significantly predicted the effects of MIT on strength gain. However, it should be noted that there was moderate heterogeneity of the effects within each meta-analysis with comparisons of MIT and no exercise, suggesting that possible varied population effects were examined (Fletcher, 2007; Borenstein et al., 2009). However, it should be noted that the interpretation of the results of this study might be overestimated due to the publication bias (Figure 2).
Overall effects of MIT on maximal voluntary contraction (MVC) force or muscle strength
Consistent with the previous reviews (Paravlic et al., 2018; Meier, 2021), the current review showed moderate overall estimated effect of MIT (ES = 1.10, 95% CI = 0.89–1.3) on enhancing muscle strength compared to no exercise groups (Figure 3). However, the results of both qualitative (Figure 3) and quantitative (Q24 = 58.86, p < 0.001, I2 = 59%) analysis of heterogeneity suggested the existence of study variability/heterogeneity among all the included studies. The estimated effect of variability among all the included studies could be caused by quality of MIT performed by subjects of different studies and/or different forms of MIT (i.e., internal MIT and external MIT) adopted by the analyzed studies. The Figure 3 shows that the estimated effects of two of the three studies (Herbert et al., 1998; Wright and Smith, 2009; Yao et al., 2013) falling on the no-effect line used external MIT (EMIT) while those of all other twenty-two studies falling on the right side of the no-effect line favoring MIT adopted internal MIT. This result was not surprising since studies (Ranganathan et al., 2004; Yao et al., 2013) have demonstrated that the effects of internal MIT were more effective than those of EMIT on improving the power of central drive [defined by motor-related cortical potential (MRCP) derived from scalp EEG data] that led to the enhancement of MVC force. Literature suggests that early strength gains are mainly due to neural adaptations including increases in the neural (descending) drive that leads to motor-unit recruitment and/or increases in firing rate of the activated motor unites (Semmler and Enoka, 2000), and/or changed input signals to the motoneuron pools that improve muscle coordination (e.g., reduction of antagonist muscle activation level during MVC, Mamone, 2013). Additional support for the effect of neural adaptations on muscle force is from the observation of the influence of motor-unit synchronization on muscle force. Previous studies (Milner-Brown et al., 1975; Semmler and Nordstrom, 1998) found that the level of motor-unit synchronization appears to be greater with hand muscles of individuals who consistently engage themselves in hand-muscle strength training, and one explanation of this finding is that an enhancement of motor-unit synchronization contributes to training-induced increases in muscle strength see Yao et al. (2000); Yao (2004), for different view on the effect of motor unit synchronization on muscle force.
While the current review demonstrated strong evidence for MIT effects on the enhancement of muscle strength when compared to no exercise, physical training still resulted in better mean effect (ES = 0.38, 95% CI 0.15–0.62) than MIT (Figure 6), that are consistent with the comparisons of MIT and PT in motor skill acquisition (Feltz and Landers, 1983). Favorable PT effect over MIT is not surprising since PT works on both muscle and central nervous system (CNS) (Sale, 1986; Enoka, 1997; Gandevia, 1999) but MIT works on CNS only (Ranganathan et al., 2004; Grôspretre et al., 2017). In general, the current review shows that PT is better than MIT. However, MIT is better than no exercise. This finding alone is important because it shows the efficacy of MIT in enhancing muscle strength which has great application in rehabilitation settings. An even more impressive finding from the current review is the effect of using a combination of PT and MIT. The combination of PT and MIT shows no noticeable difference in mean effect on enhancing MVC compared PT (Figure 7), ES = 0.04 favoring PT. One of the more extensive comparisons of combinations of MIT and PT was made in a study by Reiser and Munzert (2011). They compared five different PT and MIT conditions. One was 100% PT, and the three combinations were training routines requiring 75% PT and 25% MIT, 50% PT and 50% MIT, and 25% PT and 75% MIT. The control group required neither PT nor MIT. The results showed that the three combination groups demonstrated the increase of MVC force (3.0 to 4.2%) similar to the PT group (5.1%) but significantly greater than the control group (−0.2%). A noteworthy and interesting finding is that all three rates of MIT (25, 50, and 75%) yield nearly the same improvement of muscle strength as PT alone. This is inconsistent with a similar study by Hird et al. (1991), in which they compared the effect of PT alone with the effects of different levels of combination of PT and MIT on learning two motor skills. One was to learn a pegboard task and the other was to learn a rotary pursuit task. Regarding the effect of rates of MIT on learning the two motor skills, they (Hird et al., 1991) found that as the proportion of PT increased, the better learning would be. The discrepancy between the two studies could be explained by the difference in the tasks used in the two studies. The tasks examined in the current review were simple performances of muscle contractions but the tasks in the study by Yue and Cole (1992) were more complicated performances of motor skills. The development of performance of motor skills needs not only understanding of the movement but also visual, tactile, and proprioceptive feedback. It has been well-documented that MIT has an advantage in improving cognitive understanding of movement, and thus could facilitate the formation of the fundamental movement patterns, which is important during the early stages of learning (Driskell et al., 1994). However, imagery is not able to provide direct knowledge of results or visual and tactile feedback, which is critical for the improvement of performance during the later stages of motor skill learning. Thus, MIT might be as effective as PT during the early stage of learning when the understanding of movement sequences is a major concern but overall, PT might be superior to MIT due to its role in both obtaining knowledge of fundamental movement patterns and using visual, tactile, and proprioceptive feedback for refining motor skills (Driskell et al., 1994; Land et al., 2016).
Aging subgroup effects of MIT based on MVC force
One of the objectives of the study was to compare the effect MIT on enhancing muscle strength for young adults and older adults. Previous review by Meier (2021) showed moderate estimated effects of MIT on strength for both older (ES = 0.53, 95% CI = −0.03–1.10) and younger (ES = 0.76, 95% CI = 0.53–0.99) adult. Since the “gray rhombus” reflecting the elderly group’s point estimate of the treatment effect touched the line of no effect, it was suggested that little or unclear beneficial effect of MIT on muscle strength for the older adults. However, as argued earlier, the results of Meier’s study on older adults could be due to its use of PIV only and a small number of studies included. When CBV was also used and one more study added, the current review demonstrated clear beneficial effect of MIT on strength for older adults (Figure 4). Indeed, the estimated effect of MIT was more beneficial for the elder group than for the younger group in improving muscle strength, ES = 2.17, 95% CI = 1.57–2.76, and ES = 0.95, 95% CI = 0.74–1.17, respectively, and the difference between the two groups was statistically significant, Q1, 23 = 14.15, p = 0.0002. In addition, both qualitative (Figure 4) and quantitative (Q21 = 40.64, p < 0.01, I2 = 50.8%) results suggest heterogeneity for the young adults. In contrast, such heterogeneity did not occur for the older adults (Q4 = 2.17, p = 0.25, I2 = 26.4%). The little heterogeneity for the older adults suggests that all the estimated points of treatment for the elderly individuals might come from the same population (Higgins et al., 2003).
Neural deficit, such as the number of motor units in the muscle that cannot be recruited by maximal voluntary effort, has been reported to be a major factor causing strength loss. For example, a study by Clark et al. (2006) investigated adaptations in neural control of the plantar flexor muscles after 4 weeks of unilateral lower limb suspension. Clark et al. (2006) found that, with regards to relative contribution of neural and muscular factors to strength loss, neural deficits in central activation explained 48% of the variability in strength loss, whereas muscular factors explained 39% of the variability. Central neural degeneration or neural deficit is a significant underlying mechanism of aging-related muscle weakness. It has been shown that neural deficit, for the elderly is greater than young adults (Campbell et al., 1973; Mamone, 2013). MIT, which increases central neural drive, may be a way to reverse the degeneration to improve strength. Therefore, it was suggested that MIT in elderly could reduce neural deficit by improving the cortical-to-muscle drive, therefore improving motor unit recruitment/activation and strength. The brain signal data of the study by Mamone (2013) showed that EEG frequency power associated with MVC of the elderly was significantly greater than young adults following an 8-week MIT. The EEG power has consistently shown a positive relationship with contractile force of a muscle group (Ranganathan et al., 2004; Mamone, 2013; Yao et al., 2013). Additionally, it has been shown that the level of functional connectivity between the brain (EEG) and muscle (EMG) signals increases with muscle force in old and young (Bayram et al., 2015), suggesting that the strength of the connectivity or coupling is important for muscle output. As it has been well-documented that the contractile force of a muscle is determined by the number of recruited motor units and the firing rate of the recruited motor units and both the recruitment and firing rate are modulated by commands from the central nervous system; the greater beneficial effect of MIT on muscle strength for elderly than young adults demonstrated in the current review might be explained at least partially by the greater EEG power for the elderly followed MIT (Mamone, 2013), and perhaps improved brain-to-muscle signal connectivity (Bayram et al., 2015) following the MIT.
Muscle subgroup effects of MIT based on MVC force
Another interesting finding from the current review is that the estimated effect of MIT for finger muscles (ES = 1.64, 95% CI = 1.06–2.22) was significantly greater than the large UE muscles (ES = 0.86, 95% CI = 0.56–1.16), Q1,14 = 5.38, p = 0.02. This finding is consistent with the one from Ranganathan et al.’s (2004) study in which they directly compared MIT effects on the improvement of muscle strength of little finger abductor muscle (ABD group) and elbow flexor muscles (ELB group). After 12-weeks training, they (Ranganathan et al., 2004) found that the ABD group had increased their finger abduction strength by 35% (P < 0.005) and the ELB group augmented their elbow flexion strength by 13.5% (P < 0.001).
A potential explanation for the difference in ES of MIT between finger muscles and large muscles of the upper extremities may be due to the difference in the characteristics of motor unit behaviors between the two types of muscles. Literature indicates that muscle force is generated by increasing motor-unit recruitment and discharge rate of the recruited motor units. Studies (Milner-Brown et al., 1973; De Luca et al., 1982) have demonstrated that motor-unit recruitment is completed at about 40% of the MVC for small muscle groups such as finger muscles and at about 80% of the MVC for large muscle groups such as elbow flexor and knee extensor muscles. The force beyond these levels (∼40% of MVC for small muscles and ∼80% for large muscles) is produced purely by increasing discharge rate, which indicates that the central drive may have greater impact on firing rate of finger than larger limb muscles. In addition, outperformance of MIT of finger muscles over large upper extremity muscles may also be due to the relatively large cortical representation of the finger muscles, thus having greater potential to be influenced by MIT, over the large upper extremities. Furthermore, the large upper extremity muscles such as elbow flexor muscles are frequently used for daily activities and may be considered as “highly trained” with little room for neural adaptation-induced strength improvements. It should be noted that the magnitude of force production is directly proportional to the amplitude of the respective brain signal (Dai et al., 2001) and greater power of central motor control network has been observed following MIT (Ranganathan et al., 2004; Mamone, 2013; Yao et al., 2013). Thus, the greater effect of MIT on MVC force for the finger muscles might be explained by their greater representation in the brain (motor cortex). However, since there was no significant difference in ES of MIT between finger muscles (ES = 1.64) and large lower extremity muscles (ES = 1.20), additional factors must exist to account for the difference in the effect of MIT on these different muscle groups. Due to the lack of the literature, these factors cannot be identified and should be addressed in the future research.
Dose-response relationship of MIT to MVC force
The dose-response relationship of MIT to MVC force was examined by detecting mean effects associated with training volume variables (see Table 2). For the training period, no significant difference between groups was found, Q(3) = 2.65, p = 0.45. It (Table 2) shows that 3-weeks or less training period yields mean MIT effect equivalent to those with longer training periods. It is not surprising for the 3-weeks or less training period of MIT yields a substantial improvement of MVC force since studies (Sale, 1986; Enoka, 1997; Chilibeck et al., 1998; Akima et al., 1999) have clearly shown that early strength gains within the first 3 to 4 weeks of a conventional strength training program are mainly attributed to the neural adaptations. Two studies with only one week’s MIT training (Cornwall et al., 1991; Grôspretre et al., 2017) also showed a significant increase in muscle strength compared to the baseline (see Table 1). In the 1-week training by Grôspretre et al. (2017), the MIT group increased both plantar flexion MVC torque and the rate of torque development (RTD) along with the significant increase of EMG activity and V-wave during MVC and of H-reflex at rest. Thus, Grôspretre et al. (2017) attributed the significant enhancement of MVC torque and RTD after 1-week MIT training to the increased cortical descending neural drive and the excitability of spinal networks at rest. In a longitudinal study, Ranganathan et al. (2004) inspected MVC force changes of both little finger abductor (ABD) and elbow flexor (ELB) muscles under MIT intervention over 12 weeks. The maximal gain of MVC was obtained after 4-week training for ABD and 2-week training for ELB. Furthermore, the enhancements of MVC force of both ABD and ELB groups were accompanied with the significant increase of MVC-related cortical potential. Putting together, there is no research evidence to suggest an optimal training period for MIT. It seems that the optimal training is muscle specific. The limited research evidence tentatively suggests that larger muscle groups need one to 2 weeks of MIT training to achieve significant improvement (Ranganathan et al., 2004; Grôspretre et al., 2017) and small finger muscles may need a longer training period, three to 4 weeks, to obtain maximal MIT effect (Ranganathan et al., 2004).
For the training sessions per week, 15 out of 22 studies used five sessions per week. Compared to the less frequent training (3 or 2 sessions per week), the more frequent training (5 or 7 sessions per week) shows a noticeable advantage but the difference between the two was not significant, Q(1, 20) = 1.68, p = 0.20. The estimated MIT effect for 5 or more sessions is ES = 1.39, 95% CI 1.13–1.66 and for 3 or 2 sessions is ES = 1.06, 95% CI 0.62–1.49. Due to the lack of the significant difference between the two groups, thus no recommendation could be made for the optimal training frequency in the current review and further research is needed to address the issue.
Regarding the number of trials per session, most of studies (11) had 30 to 40 trials in a session that also resulted in largest mean MIT effect (ES = 1.48, 95% CI 1.15–1.81). It should be noted that the difference in mean MIT effects among the number of trials per session groups is not significant, Q(3) = 2.80, p = 0.42. Thus, the decision of the number of trials per session should be made based on training period, number of sessions per week, and even individual’s imagery ability as suggested by Paravlic et al. (2018).
For the total number of trials per study, similar to the number of trials per session, the difference in mean MIT effects among the groups is not significant, Q(3) = 2.65, p = 45 and ES for the four groups are remarkably close, ranging from 1.19 to 1.75. Although no optimal total trails in a study could be identified, it should be noted that majority of the included studies had 600 or more total training trials. Thus, it is suggested that a minimum of number of 600 trials might be needed.
Limitations of the current review
Like many other studies with systematic reviews and meta-analyses, the current review has some limitations. The funnel plot (Figure 2) is skewed asymmetrically indicating publication bias. The Egger’s regression test provided further statistical evidence to show bias for the publications, p < 0.001. The publication bias may lead to overestimation of the effectiveness of MIT. The cause of the publication bias may come from the fact that studies reporting statistically significant effect sizes are more likely to be published than studies reporting negative effect size (Samawi, 2021). In addition, inclusion of the articles published in English only may also be a factor to cause the publication bias. Additional limitation is from the lack of number of studies to achieve statistical power. This is true in our subgroup analysis to detect a dose-response relationship of MIT on muscle strength. Thus, it is difficult to identify optimal volumes in the four training variables (Table) examined in the current review because of the absence of statistical evidence (i.e., statistically not significant between subgroups). It should be noted that absence of evidence is not evidence of absence. In other words, no significant difference in effect sizes between subgroups does not automatically mean that the subgroups produce equivalent outcomes. A further limitation to the findings of this review is that no studies comprehensively examined the efficacy of MIT on enhancing muscle strength. In other words, the focus and design of MIT intervention is very different and that makes it difficult, if possible, to make firm conclusions on the issues such as optimal training volumes and types of MIT intervention.
Conclusion
In general, the current review shows that MIT has better estimated effects on enhancing muscle strength compared to no exercise, but inferior to PT. The intervention of the combination of MIT and PT is equivalent to PT alone in enhancing MVC force. The subgroup group analyses further suggest that older adults and small finger muscles may be beneficial more from MIT than young adults and larger muscles. In summary, the current review suggests that MIT is an effective substitute or addition to PT in muscle strength training. Therefore, MIT should be considered and applied in rehabilitation settings when physical training is too demanding for patients with motor disabilities (e.g., poststroke rehabilitation) or high-intensity resistance training is impractical or venerable to injuries (e.g., very old adults).
Data availability statement
The original contributions presented in this study are included in the article/supplementary material, further inquiries can be directed to the corresponding authors.
Author contributions
XL, GY, and WY: conception, design of study, and acquisition of data. XL, GY, WY, SG, AC, and ZY: analysis and/or interpretation of data and drafting the manuscript. All authors contributed to the article and approved the submitted version.
Conflict of interest
The authors declare that the research was conducted in the absence of any commercial or financial relationships that could be construed as a potential conflict of interest.
Publisher’s note
All claims expressed in this article are solely those of the authors and do not necessarily represent those of their affiliated organizations, or those of the publisher, the editors and the reviewers. Any product that may be evaluated in this article, or claim that may be made by its manufacturer, is not guaranteed or endorsed by the publisher.
References
Akima, H., Takahashi, H., Kuno, S. Y., Masuda, K., Masuda, T., Shimojo, H., et al. (1999). Early phase adaptations of muscle use and strength to isokinetic training. Med. Sci. Sports Exerc. 31, 588–594. doi: 10.1097/00005768-199904000-00016
Alenezi, M. (2018). in Motor imagery as a potential tool for improvement of musculoskeletal function in physiotherapy practice. Ph.D. Dissertation, ed. H. Kubis (Bangor: Bangor University).
Altermann, C. D. C., Martins, A. S., Carpes, F. P., and Mello-Carpes, P. B. (2014). Influence of mental practice and movement observation on motor memory, cognitive function and motor performance in the elderly. Braz. J. Phys. Ther. 18, 201–209. doi: 10.1590/S1413-35552012005000150
Amir-Behghadami, M., and Janati, A. (2020). Population, intervention, comparison, outcomes and study (Picos) design as a framework to formulate eligibility criteria in systematic reviews. Emerg. Med. J. 37:387. doi: 10.1136/emermed-2020-209567
Bahari, S. M., Damirchi, A., Rahmaninia, F., and Salehian, M. H. (2011). The effects of mental practice on strength gain and electromyographic changes in elbow flexor muscles. Ann. Biol. Res. 2, 198–207.
Bayram, M., Siemionow, V., and Yue, G. (2015). Weakening of corticomuscular signal coupling during voluntary motor action in aging. J. Gerontol. A Biol. Sci. Med. Sci. 70, 1037–1043. doi: 10.1093/gerona/glv014
Borenstein, M., Hedges, L., Higgins, J., and Rothstein, H. (2009). Introduction to meta-analysis. Chichester: Wiley. doi: 10.1002/9780470743386
Bouguetoch, A., Martin, A., and Grospretre, S. (2021). Insights into the combination of neuromuscular electrical stimulation and motor imagery in a training-based approach. Eur. J. Appl. Physiol. 121, 941–955. doi: 10.1007/s00421-020-04582-4
Campbell, M., Mccomas, A., and Petito, F. (1973). Physiological changes in ageing muscle. J. Neurol. Neurosurg. Psychiatry 36, 174–182. doi: 10.1136/jnnp.36.2.174
Chilibeck, P. D., Calder, A. W., Sale, D. G., and Webber, C. E. (1998). A comparison of strength and muscle mass increases during resistance training in young women. Eur. J. Appl. Physiol. Occup. Physiol. 77, 170–175. doi: 10.1007/s004210050316
Clark, B., Fernhall, B., and Ploutz-Snyder, L. (2006). Adaptations in human neuromuscular function following prolonged unweighting: Ii. Skeletal muscle contractile properties and applied ischemia efficacy. J. Appl. Physiol. 101, 264–272. doi: 10.1152/japplphysiol.01404.2005
Cornwall, M. W., Bruscato, M. P., and Barry, S. (1991). Effect of mental practice on isometric muscular strength. J. Orthop. Sports Phys. Ther. 13, 231–234. doi: 10.2519/jospt.1991.13.5.231
Cuijpers, P., Weitz, E., Cristea, I. A., and Twisk, J. (2017). Pre-post effect sizes should be avoided in meta-analyses. Epidemiol. Psychiatr. Sci. 26, 364–368. doi: 10.1017/S2045796016000809
da Costa, B. R., Nuesch, E., Rutjes, A. W., Johnston, B. C., Reichenbach, S., Trelle, S., et al. (2013). Combining follow-up and change data is valid in meta-analyses of continuous outcomes: A meta-epidemiological study. J. Clin. Epidemiol. 66, 847–855. doi: 10.1016/j.jclinepi.2013.03.009
Dahm, S. F., Weigelt, M., and Rieger, M. (2022). Sequence representations after action-imagery practice of one-finger movements are effector-independent. Psychol. Res. doi: 10.1007/s00426-022-01645-3
Dai, T. H., Liu, J. Z., Sahgal, V., Brown, R. W., and Yue, G. H. (2001). Relationship between muscle output and functional MRI-measured brain activation. Exp. Brain Res. 140, 290–300. doi: 10.1007/s002210100815
Darvishi, M., Ahmadi, S., Hierani, A., and Jabari, N. (2013). Effects of motor imagery and maximal isometric action on grip strength of elderly men. World Appl. Sci. J. 24, 556–560.
De Luca, C., Lefever, R., Mccue, M., and Xenakis, A. (1982). Behavior of human motor units in different muscles during linearly varing contractions. J. Physiol. (London) 329, 113–128. doi: 10.1113/jphysiol.1982.sp014293
de Morton, N. A. (2009). The pedro scale is a valid measure of the methodological quality of clinical trials: A demographic study. Aust. J. Physiother. 55, 129–133. doi: 10.1016/S0004-9514(09)70043-1
de Ruiter, C. J., Hutter, V., Icke, C., Groen, B., Gemmink, A., Smilde, H., et al. (2012). The effects of imagery training on fast isometric knee extensor torque development. J. Sports Sci. 30, 166–174. doi: 10.1080/02640414.2011.627369
Decety, J. (1996). Neural representations for action. Rev. Neurosci. 7, 285–297. doi: 10.1515/REVNEURO.1996.7.4.285
Decety, J., and Grezes, J. (1999). Neural mechanisms subserving the perception of human actions. Trends Cogn. Sci. 3, 172–178. doi: 10.1016/S1364-6613(99)01312-1
Deeks, J., Higgins, J., and Altman, D. (2022). “Chapter 10: Analysing data and undertaking meta-analyses,” in Cochrane handbook for systematic reviews of interventions, version 6.3, eds J. Higgins, J. Thomas, J. Chandler, M. Cumpston, T. Li, M. Page, et al. (London: Cochrane).
Driskell, J. E., Copper, C., and Moran, A. (1994). Does mental practice enhance performance? J. Appl. Psychol. 79, 481–492. doi: 10.1037/0021-9010.79.4.481
Duchateau, J., and Enoka, R. (2011). Human motor unit recordings: Origins and insight into the integrated motor system. Brain Res. 1409, 42–61. doi: 10.1016/j.brainres.2011.06.011
Durg, S., Dhadde, S. B., Vandal, R., Shivakumar, B. S., and Charan, C. S. (2015). Withania somnifera (Ashwagandha) in neurobehavioural disorders induced by brain oxidative stress in rodents: A systematic review and meta-analysis. J. Pharm. Pharmacol. 67, 879–899. doi: 10.1111/jphp.12398
Enoka, R. M. (1997). Neural adaptations with chronic physical activity. J. Biomech. 30, 447–455. doi: 10.1016/S0021-9290(96)00170-4
Enoka, R. M., and Pearson, K. G. (2014). “The motor unit and muscle action,” in Principles of neural science, Fifth Edition, eds E. R. Kandel, J. H. Schwartz, T. M. Jessell, S. A. Siegelbaum, and A. J. Hudspeth (New York, NY: McGraw-Hill Education).
Feltz, D., and Landers, D. M. (1983). The effects of mental practice on motor skill learning and performance: A meta-analysis. J. Sport Psychol. 5, 25–57. doi: 10.1123/jsp.5.1.25
Fletcher, J. (2007). What is heterogeneity and is it important? BMJ 334, 94–96. doi: 10.1136/bmj.39057.406644.68
Fontani, G., Migliorini, S., Benocci, R., Facchini, A., Casini, M., and Corradeschi, F. (2007). Effect of mental imagery on the development of skilled motor actions. Percept. Mot. Skills 105, 803–826. doi: 10.2466/pms.105.3.803-826
Gandevia, S. C. (1999). Mind, muscles and motoneurones. J. Sci. Med. Sports Health 2, 167–180. doi: 10.1016/S1440-2440(99)80171-6
Goudarzian, M., Ghavi, S., Shariat, A., Shirvani, H., and Rahimi, M. (2017). Effects of whole body vibration training and mental training on mobility, neuromuscular performance, and muscle strength in older men. J. Exerc. Rehabil. 13, 573–580. doi: 10.12965/jer.1735024.512
Gould, D., Weinberg, R., and Jackson, A. (1980). Mental preparation strategies, cognitions, and strength performance. J. Sport Psychol. 2, 329–339. doi: 10.1123/jsp.2.4.329
Grôspretre, S., Jacquet, T., Lebon, F., Papaxanthis, C., and Martin, A. (2017). Neural mechanisms of strength increase after one-week motor imagery training. Eur. J. Sport Sci. 18, 209–218. doi: 10.1080/17461391.2017.1415377
Harrer, M., Cuijpers, P., Furukawa, T. A., and Ebert, D. D. (2022). “Chapter 8: Meta-regression,” in Doing meta-analysis with R: A hands-on guide, (London: Chapman & Hall/Crc Press). doi: 10.1201/9781003107347
Herbert, R. D., Dean, C., and Gandevia, S. C. (1998). Effects of real and imagined training on voluntary muscle activation during maximal isometric contractions. Acta Physiol. Scand. 163, 361–368. doi: 10.1046/j.1365-201X.1998.t01-1-00358.x
Hétu, S., Grégoire, M., Saimpont, A., Coll, M. P., Eugène, F., Michon, P. E., et al. (2013). The neural network of motor imagery: An Ale meta-analysis. Neurosci. Biobehav. Rev. 37, 930–949. doi: 10.1016/j.neubiorev.2013.03.017
Higgins, J., Li, T., and Deeks, J. (2022). “Chapter 6. Choosing effect measures and computing estimates of effects,” in Cochrane handbook for systematic reviews of interventions, version 6.3, eds J. Higgins, J. Thomas, J. Chandler, M. Cumpston, T. Li, M. Page, et al. (London: Cochrane).
Higgins, J. P., Thompson, S. G., Deeks, J. J., and Altman, D. G. (2003). Measuring inconsistency in meta-analyses. BMJ 327, 557–560. doi: 10.1136/bmj.327.7414.557
Hinshaw, K. E. (1991). The effects of mental practice on motor skill performance: Critical evaluation and meta-analysis. Imagin. Cogn. Pers. 11, 3–35. doi: 10.2190/X9BA-KJ68-07AN-QMJ8
Hird, J. S., Landers, D. M., Thomas, J. R., and Horan, J. J. (1991). Physical practice is superior to mental practice in enhancing cognitive and motor task performance. J. Sport Exerc. Psychol. 13, 281–293. doi: 10.1123/jsep.13.3.281
Hoffmann, T. C., Glasziou, P. P., Boutron, I., Milne, R., Perera, R., Moher, D., et al. (2014). Better reporting of interventions: Template for intervention description and replication (TidieR) checklist and guide. BMJ 348:g1687. doi: 10.1136/bmj.g1687
Jackson, P. L., Lafleur, M. F., Malouin, F., Richards, C., and Doyon, J. (2001). Potential role of mental practice using motor imagery in neurologic rehabilitation. Arch Phys. Med. Rehabil. 82, 1133–1141. doi: 10.1053/apmr.2001.24286
Jiang, C., Ranganathan, V. K., Zhang, J., Siemionow, V., and Yue, G. H. (2016). Motor effort training with low exercise intensity improves muscle strength and descending command in aging. Medicine (Baltimore) 95:e3291. doi: 10.1097/MD.0000000000003291
Jiang, C.-H., Ranganathan, V., Siemionow, V., and Yue, G. (2017). The level of effort, rather than muscle exercise intensity determines strength gain following a six-week training. Life Sci. 178, 30–34. doi: 10.1016/j.lfs.2017.04.003
Jones, E. J., Bishop, P. A., Woods, A. K., and Green, J. M. (2008). Cross-sectional area and muscular strength: A brief review. Sports Med. 38, 987–994. doi: 10.2165/00007256-200838120-00003
Krüger, B., Hettwer, M., Zabicki, A., De Haas, B., Munzert, J., and Zentgraf, K. (2020). Practice modality of motor sequences impacts the neural signature of motor imagery. Sci. Rep. 10:19176. doi: 10.1038/s41598-020-76214-y
Krzysztofik, M., Wilk, M., Wojdała, G., and Gołaś, A. (2019). Maximizing muscle hypertrophy: A systematic review of advanced resistance training techniques and methods. Int. J. Environ. Res. Public Health 16:4897. doi: 10.3390/ijerph16244897
Land, W. M., Liu, B., Cordova, A., Fang, M., Huang, Y., and Yao, W. X. (2016). Effects of physical practice and imagery practice on bilateral transfer in learning a sequential tapping task. PLoS One 11:e0152228. doi: 10.1371/journal.pone.0152228
Lebon, F., Collet, C., and Guillot, A. (2010). Benefits of motor imagery training on muscle strength. J. Strength Cond. Res. 24, 1680–1687. doi: 10.1519/JSC.0b013e3181d8e936
Leung, M., Spittle, M., and Kidgell, D. (2013). Corticospinal excitability following short-term motor imagery training of a strength task. J. Imagery Res. Sport Phys. Activity 8, 35–44. doi: 10.1515/jirspa-2012-0006
Lindsay, R. S., Larkin, P., Kittel, A., and Spittle, M. (2021). Mental imagery training programs for developing sport-specific motor skills: A systematic review and meta-analysis. Phys. Educ. Sport Pedagogy 1–22. doi: 10.1080/17408989.2021.1991297
Mamone, B. (2013). Motor imagery training facilitates neural adaptations associated with muscle strengthening in aging. Ph.D. Dissertation, ed. G. Yue (Kent, OH: Kent State University).
Manochio, J. P., Lattari, E., Portugal, E. M., Monteiro-Junior, R. S., Paes, F., Budde, H., et al. (2015). From mind to body: Is mental practice effective on strength gains? A meta-analysis. CNS Neurol. Disord. Drug Targets 14, 1145–1151. doi: 10.2174/1871527315666151111125847
Meier, D. (2021). The effect of mental imagery practice on maximal voluntary strength: A meta-analysis and systematic review. San Antonio, TX: The University of Texas at San Antonio.
Milner-Brown, H., Sein, R., and Lee, R. (1975). Synchronization of human motor units: Possible roles of exercise and supraspinal reflexes. Electroencephalogr. Clin. Neurophysiol. 38, 245–254. doi: 10.1016/0013-4694(75)90245-X
Milner-Brown, H., Stein, R., and Yemm, R. (1973). The orderly recruitment of human motor units during voluntary isometric contractions. J. Physiol. (London) 228, 359–370. doi: 10.1113/jphysiol.1973.sp010192
Montuori, S., Curcio, G., Sorrentino, P., Belloni, L., Sorrentino, G., Foti, F., et al. (2018). Functional role of internal and external visual imagery: Preliminary evidences from pilates. Neural Plast. 2018:7235872. doi: 10.1155/2018/7235872
Niazi, S. M., Bai, N., and Shahamat, M. D. (2014). Investigation of effects of imagery training on changes in the electrical activity of motor units of muscles and their strength in the lower extremities. Eur. J. Exp. Biol. 4, 595–599.
Page, M., Higgins, J., and Sterne, J. (2022). “Chapter 13: Assessing risk of bias due to missing results in a synthesis,” in Cochrane handbook for systematic reviews of interventions version 6.3, eds J. Higgins, J. Thomas, J. Chandler, M. Cumpston, T. Li, M. Page, et al. (London: Cochrane).
Page, M. J., Mckenzie, J. E., Bossuyt, P. M., Boutron, I., Hoffmann, T. C., Mulrow, C. D., et al. (2021). The prisma 2020 statement: An updated guideline for reporting systematic reviews. BMJ 372:n71. doi: 10.1136/bmj.n71
Paravlic, A. H., Slimani, M., Tod, D., Marusic, U., Milanovic, Z., and Pisot, R. (2018). Effects and dose-response relationships of motor imagery practice on strength development in healthy adult populations: A systematic review and meta-analysis. Sports Med. 48, 1165–1187. doi: 10.1007/s40279-018-0874-8
Ranganathan, V. K., Siemionow, V., Liu, J. Z., Sahgal, V., and Yue, G. H. (2004). From mental power to muscle power–gaining strength by using the mind. Neuropsychologia 42, 944–956. doi: 10.1016/j.neuropsychologia.2003.11.018
Reiser, M., Büsch, D., and Munzert, J. (2011). Strength gains by motor imagery with different ratios of physical to mental practice. Front. Psychol. 2:194. doi: 10.3389/fpsyg.2011.00194
Reiser, M., and Munzert, J. (2011). Strength gains by motor imagery with different ratios of physical to mental practice. Front. Psychol. 2:194. doi: 10.3389/fpsyg.2011.00194
Rozand, V., Sundberg, C. W., Hunter, S. K., and Smith, A. E. (2020). Age-related deficits in voluntary activation: A systematic review and meta-analysis. Med. Sci. Sports Exerc. 52, 549–560. doi: 10.1249/MSS.0000000000002179
Saimpont, A., Malouin, F., Tousignant, B., and Jackson, P. L. (2013). Motor imagery and aging. J. Mot. Behav. 45, 21–28. doi: 10.1080/00222895.2012.740098
Sale, D. (1986). “Neural adaptation in strength and power training,” in Human muscle power, eds N. L. Jones, N. Mccartney, and A. J. Mccomas (Champaign, IL: Human Kinetics).
Sale, D. G. (1987). Influence of exercise and training on motor unit activation. Exerc. Sport Sci. Rev. 15, 95–151. doi: 10.1249/00003677-198700150-00008
Samawi, H. (2021). “Chapter 9. Publication bias in meta-analysis,” in Applied meta-analysis with R and stata, eds D. Chen and K. Peace (New York, NY: Chapman and Hall/Crc). doi: 10.1201/9780429061240-9
Sawilowsky, S. S. (2009). New effect size rules of thumb. J. Modern Appl. Stat. Methods 8, 597–599. doi: 10.22237/jmasm/1257035100
Semmler, J., and Enoka, R. (2000). “Neural contributions to changes in muscle strength,” in Biomechanics, ed V. M. Zatsiorsky (Hoboken, NJ: Blackwell Science Oxford).
Semmler, J. G., and Nordstrom, M. A. (1998). Motor unit discharge and force tremor in skill- and strength-trained individuals. Exp. Brain Res. 119, 27–38. doi: 10.1007/s002210050316
Shackel, E. M., and Standing, L. G. (2007). Mind over matter: Mental training increases physical strength. North Am. J. Psychol. 9, 189–200.
Shelton, T. O., and Mahoney, M. J. (1978). The content and effect of “Psyching-Up” strategies in weight lifters. Cogn. Ther. Res. 2, 275–284. doi: 10.1007/BF01185789
Sidaway, B., and Trzaska, A. R. (2005). Can mental practice increase ankle dorsiflexor torque? Phys. Ther. 85, 1053–1060. doi: 10.1093/ptj/85.10.1053
Simoneau, E., Martin, A., Porter, M., and Van Hoecke, J. (2006). Strenght training in old age: Adaptation of antagonist muscles at the ankle joint. Muscle Nerve 33, 546–555. doi: 10.1002/mus.20492
Simonsmeier, B. A., Andronie, M., Buecker, S., and Frank, C. (2021). The effects of imagery interventions in sports: A meta-analysis. Int. Rev. Sport Exerc. Psychol. 14, 186–207. doi: 10.1080/1750984X.2020.1780627
Siparsky, P. N., Kirkendall, D. T., and Garrett, W. E. Jr. (2014). Muscle changes in aging: Understanding sarcopenia. Sports Health 6, 36–40. doi: 10.1177/1941738113502296
Slimani, M., Tod, D., Chaabene, H., Miarka, B., and Chamari, K. (2016). Effects of mental imagery on muscular strength in healthy and patient participants: A systematic review. J. Sports Sci. Med. 15, 434–450.
Smith, D., Collins, D., and Holmes, P. (2003). Impact and mechanism of mental practice effects on strength. Int. J. Sport Exerc. Psychol. 1, 293–306. doi: 10.1080/1612197X.2003.9671720
Tod, D., Edwards, C., Mcguigan, M., and Lovell, G. (2015). A systematic review of the effect of cognitive strategies on strength performance. Sports Med. 45, 1589–1602. doi: 10.1007/s40279-015-0356-1
Wright, D. J., and Smith, D. (2009). The effect of pettlep imagery on strength performance. Int. J. Sport Exerc. Psychol. 7, 18–31. doi: 10.1080/1612197X.2009.9671890
Yao, W., Fuglevand, A., and Enoka, R. (2000). Motor unit synchronization increases Emg amplitude and decreases force steadiness in simulated contractions. J. Neurophysiol. 83, 441–452. doi: 10.1152/jn.2000.83.1.441
Yao, W. X. (2004). Roles of motor-unit recruitment in producing force variability of simulated muscle contractions. Mot. Control 8, 64–75. doi: 10.1123/mcj.8.1.64
Yao, W. X., Ranganathan, V. K., Allexandre, D., Siemionow, V., and Yue, G. H. (2013). Kinesthetic imagery training of forceful muscle contractions increases brain signal and muscle strength. Front. Hum. Neurosci. 7:561. doi: 10.3389/fnhum.2013.00561
Yue, G., and Cole, K. J. (1992). Strength increases from the motor program: Comparison of training with maximal voluntary and imagined muscle contractions. J. Neurophysiol. 67, 1114–1123. doi: 10.1152/jn.1992.67.5.1114
Yue, G. H., Ranganathan, V. K., Siemionow, V., Liu, J. Z., and Sahgal, V. (1999). Older adults exhibit a reduced ability to fully activate their biceps brachii muscle. J. Gerontol. A Biol. Sci. Med. Sci. 54, M249–M253. doi: 10.1093/gerona/54.5.M249
Yue, G. H., Ranganathan, V. K., Siemionow, V., Liu, J. Z., and Sahgal, V. (2000). Evidence of inability to fully activate human limb muscle. Muscle Nerve 23, 376–384. doi: 10.1002/(SICI)1097-4598(200003)23:3<376::AID-MUS9>3.0.CO;2-2
Yue, G. H., Wilson, S. L., Cole, K. J., Darling, W. G., and Yuh, W. (1996). Imagined muscle contraction training increases voluntary neural drive to muscle. J. Psychophysiol. 10, 198–208.
Keywords: motor imagery practice, mental practice, movement imagery, muscle strength, performance, strength enhancement, aging
Citation: Liu XJ, Ge S, Cordova A, Yaghi Z, Jiang BY, Yue GH and Yao WX (2023) Elderly may benefit more from motor imagery training in gaining muscle strength than young adults: A systematic review and meta-analysis. Front. Psychol. 13:1052826. doi: 10.3389/fpsyg.2022.1052826
Received: 24 September 2022; Accepted: 05 December 2022;
Published: 04 January 2023.
Edited by:
Ana-Maria Cebolla, Université libre de Bruxelles, BelgiumReviewed by:
Hadi Nobari, University of Granada, SpainJ. Matt McCrary, Hanover University of Music, Drama and Media, Germany
Copyright © 2023 Liu, Ge, Cordova, Yaghi, Jiang, Yue and Yao. This is an open-access article distributed under the terms of the Creative Commons Attribution License (CC BY). The use, distribution or reproduction in other forums is permitted, provided the original author(s) and the copyright owner(s) are credited and that the original publication in this journal is cited, in accordance with accepted academic practice. No use, distribution or reproduction is permitted which does not comply with these terms.
*Correspondence: Guang H. Yue, ✉ Z3l1ZUBrZXNzbGVyZm91bmRhdGlvbi5vcmc=; Wan X. Yao, ✉ d2FueGlhbmcueWFvQHV0c2EuZWR1