- 1Laboratory for Multimodal Neuroimaging, Department of Psychiatry and Psychotherapy, Philipps-University Marburg, Marburg, Germany
- 2Center for Mind, Brain and Behavior, Philipps-University Marburg, Marburg and Justus-Liebig University Giessen, Giessen, Germany
- 3Core-Facility Brainimaging, Faculty of Medicine, Philipps-University Marburg, Marburg, Germany
- 4Department of Child and Adolescent Psychiatry, Psychosomatics and Psychotherapy, Philipps-University Marburg, Marburg, Germany
- 5Bender Institute of Neuroimaging, Justus-Liebig University Giessen, Giessen, Germany
Face processing is mediated by a distributed neural network commonly divided into a “core system” and an “extended system.” The core system consists of several, typically right-lateralized brain regions in the occipito-temporal cortex, including the occipital face area (OFA), the fusiform face area (FFA) and the posterior superior temporal sulcus (pSTS). It was recently proposed that the face processing network is initially bilateral and becomes right-specialized in the course of the development of reading abilities due to the competition between language-related regions in the left occipito-temporal cortex (e.g., the visual word form area, VWFA) and the FFA for common neural resources. In the present pilot study, we assessed the neural face processing network in 12 children (aged 7–9 years) and 10 adults with functional magnetic resonance imaging (fMRI). The hemispheric lateralization of the core face regions was compared between both groups. The study had two goals: First, we aimed to establish an fMRI paradigm suitable for assessing activation in the core system of face processing in young children at the single subject level. Second, we planned to collect data for a power analysis to calculate the necessary group size for a large-scale cross-sectional imaging study assessing the ontogenetic development of the lateralization of the face processing network, with focus on the FFA. It was possible to detect brain activity in the core system of 75% of children at the single subject level. The average scan-to-scan motion of the included children was comparable to adults, ruling out that potential activation differences between groups are caused by unequal motion artifacts. Hemispheric lateralization of the FFA was 0.07 ± 0.48 in children (indicating bilateral activation) and −0.32 ± 0.52 in adults (indicating right-hemispheric dominance). These results thus showed, as expected, a trend for increased lateralization in adults. The estimated effect size for the FFA lateralization difference was d = 0.78 (indicating medium to large effects). An adequately powered follow-up study (sensitivity 0.8) testing developmental changes of FFA lateralization would therefore require the inclusion of 18 children and 26 adults.
Introduction
Face processing is mediated by a distributed neural network. This network is, as first outlined in the Haxby model (Haxby et al., 2000), often divided into a “core system” and an “extended system” (Bernstein and Yovel, 2015). The core system consists of several bilateral brain regions in the occipito-temporal cortex. These regions include the fusiform face area (FFA) in the middle fusiform gyrus, the occipital face area (OFA) in the lateral inferior occipital gyrus and the posterior superior temporal sulcus (pSTS). The OFA has often been associated with the processing of single physical features of faces including the eyes, the mouth and the nose (Gschwind et al., 2012), while the FFA is responsible for the analysis of invariant aspects of the face, as for example face identity (Rossion, 2015). The pSTS is involved in the processing of dynamic changeable facial features, for instance eye-gaze, mouth movements and facial expressions (Ishai et al., 2005). Beyond the core system, there are a number of additional (non-face-specific) regions that contribute to face processing, e.g., the inferior frontal gyrus (IFG), the orbitofrontal cortex (OFC), the amygdalae and the insula (Haxby et al., 2000; Aylward et al., 2005; Fairhall and Ishai, 2007; Gobbini and Haxby, 2007; Ishai, 2008; Haist et al., 2013). This extended system of face processing tends to be task-specific and comes into play if additional information is extracted from faces, e.g., emotions, biographical information and/or attractiveness.
The neural face processing network is distributed across both hemispheres, but typically shows a right-hemispheric dominance in adults. This finding first originated from studies of patients with acquired prosopagnosia, i.e., the inability to recognize the identity of faces following brain damage. A large proportion of patients suffering from acquired prosopagnosia had lesions in the posterior right hemisphere (for an overview, see Bukowski et al., 2013). Although bilateral lesions often lead to more severe impairments than unilateral damage, unilateral-right damage is often sufficient to cause these impairments. Over the last 20 years, the face processing network has been extensively investigated in adults, in particular with functional magnetic resonance imaging (fMRI). Functional neuroimaging studies confirmed the right-hemispheric dominance of the face-processing network. The right hemisphere typically shows stronger response to face stimuli, both in terms of the spatial extent of the activation and the strength of activity (Hemond et al., 2007; Willems et al., 2010; Bukowski et al., 2013; Frässle et al., 2016a, c).
However, far less is known about its ontogenetic development. Some studies reported a pattern of increasing face-selectivity in the FFA (e.g., Gathers et al., 2004; Aylward et al., 2005; Golarai et al., 2007; Peelen et al., 2009; Haist et al., 2013). Nonetheless, it is still a matter of debate at what age this face selectivity develops (Gathers et al., 2004; Aylward et al., 2005; Golarai et al., 2007). Other studies reported a developmental shift from a more distributed activation pattern in children to a more focused activation pattern in adults (e.g., Passarotti et al., 2003; Scherf et al., 2007). Less research has been performed on the development of the OFA. A positive correlation between the intensity of right OFA activation and age was found (Joseph et al., 2011). This finding is in line with an earlier study that found lower intensity of face-preferential activation within the right-hemispheric OFA for children (6–10 years) compared to adolescents (11–14 years) and adults (Scherf et al., 2007). Findings on pSTS engagement during face processing in children are mixed. Some studies reported no (Joseph et al., 2011) or reduced (Scherf et al., 2007) pSTS recruitment in children. Other studies found no activation differences between children (of at least 7 years) and adults (Golarai et al., 2007; Cohen Kadosh et al., 2011). Other studies even reported stronger pSTS recruitment in children compared to adults (Haist et al., 2013). All three regions of the core face processing network, i.e., OFA, FFA as well as pSTS showed a smaller size in 5–7-year-old children compared to adults (Cohen et al., 2019). While face perception in general is reported to mature early in development (at or even before 5 years of age), the face-specific memory is assumed to mature later, at around 10 years of age (Weigelt et al., 2014). For adults, a “coarse-to-fine” processing of face dimensions has been suggested with facial gender and age information emerging before identity information (Dobs et al., 2019). Taken together, the empirical evidence on the functional neuroanatomy of the core face processing network in children is inconsistent, indicating large variability in terms of the localization of the brain regions of the core-system and its activation strength.
The present study focused on the development of hemispheric lateralization. It has been speculated that right-hemispheric lateralization of the core face processing network is emerging during development from childhood to adulthood. However, it remains unclear, at what age right-dominance emerges and which factors drive this specialization. Recent neuroimaging studies suggested that right-hemispheric specialization for face processing is initiated when children learn to read and further increases through adolescence (Dehaene and Cohen, 2011; Dundas et al., 2013; Behrmann and Plaut, 2015). The development of reading abilities, typically starting at the age of six, is neuroanatomically associated with the visual word form area (VWFA). The VWFA is considered to be an essential area for reading and is hypothesized to be involved in identifying words and letters from lower-level shape images, prior to association with phonology or semantics (Price and Devlin, 2003; Dehaene and Cohen, 2011). It is thought to be highly competitive with the FFA for common neural resources during childhood (Cantlon et al., 2011; Dundas et al., 2013). VWFA and FFA show similar positions in the fusiform gyrus, with a slightly more anterior location of the FFA compared to the VWFA (Dien, 2009). Individuals with left-lateralization of language areas (as seen in most right-handers) show a gradual VWFA lateralization to the left hemisphere in order to optimize the connectivity between orthographical representations, whereas the FFA shows a right-lateralization (Dehaene and Cohen, 2011; Behrmann and Plaut, 2015; Gerrits et al., 2019). In contrast, individuals with right-lateralization of language areas have a higher probability of an atypical VWFA right-lateralization and FFA left-lateralization in course of development (Gerrits et al., 2019). Furthermore, recent literature revealed that the VWFA is mostly left-lateralized in readers of alphabetic writing systems (e.g., English and German), while readers of non-alphabetic writing systems (e.g., Chinese) often exhibit bilateral engagement of the left and right VWFA (Carlos et al., 2019). Alphabetic orthographies may bias readers toward analytic visual strategies, whereas non-alphabetic orthographies may bias readers toward holistic visual strategies that emphasize the overall structure of a word. This finding is in line with previous face processing literature that assumes that the shift of the face processing network to the right hemisphere is driven by the differences in the face processing style of children and adults. The right hemisphere is believed to be involved in a holistic processing of faces, whereas the left hemisphere is more specialized in the processing of single features (Hillger and Koenig, 1991; Rhodes et al., 1993; Meng et al., 2012). It is suggested that adults encode faces using a holistic strategy based on the configural information of the face, i.e., the spatial relations among the different facial features (Schwarzer, 2000; Aylward et al., 2005; Dundas et al., 2013). Children younger than 10 years tend to encode faces using an analytic strategy by analyzing distinctive facial features (Schwarzer, 2000). This analytic strategy would suggest a stronger recruitment of the left hemisphere during face processing in children compared to adults (Meng et al., 2012). The use of a holistic versus analytic strategy and therefore right- or left-lateralization of FFA and VWFA might further be driven by different reading instruction methods in early readers (Carlos et al., 2019). However, as only right-handed subjects of the alphabetic writing system (i.e., German) were included in the present study, a typical left-lateralization of the VWFA was expected. Saygin et al. (2016) previously investigated the development of the VWFA from childhood to adulthood. It has been shown that VWFA connectivity patterns arise in early development, before children are able to read (around 5 years) and instruct the subsequent functional VWFA development during the process of learning how to read between 5 and 8 years (Saygin et al., 2016). Whereas the VWFA of 5-year-old children that could not yet read was neither orthographically selective, nor selective for faces, their left FFA already showed a strong selectivity for faces over letters even at 5 years of age (Saygin et al., 2016). Compared to the FFA, only few studies investigated developmental changes of OFA and pSTS lateralization during face processing. In analogy to the shift of FFA lateralization, OFA and pSTS lateralization are also expected to be subject to a developmental shift from a more bilateral activation to right-specialization during development, especially as brain regions closely interacting with each other benefit from being located close to one another to minimize signal propagation distance between those regions (Behrmann and Plaut, 2015).
The present study was explicitly designed as a pilot study. The first goal was to establish an fMRI paradigm suitable for assessing activation in the core system of face processing in young children at the single subject level. The second goal was to collect data for an informed power analysis to calculate the necessary group size for a large-scale cross-sectional imaging study assessing the ontogenetic development of the face processing network. The central question was the development of the lateralization of the FFA. We hypothesized that children would show a more bilateral brain activation pattern. In exploratory analyses, we also compared hemispheric lateralization of OFA and pSTS activity as well as the activation strength itself between both groups. We hypothesized that, similar to the FFA activity, the activation pattern for OFA and pSTS would be more bilateral in children. We further hypothesized that children would show, due to still developing neural specialization, reduced activity in all brain regions of the core system (i.e., bilateral OFA, FFA, and pSTS).
Materials and Methods
Subjects
Participants were recruited through distribution of flyers and bulletins in public places and advertisement through the student mailing list of the Philipps-University of Marburg, Germany. Ten adults (three females, seven males; 24–45 years; mean age 32.1 ± 6.1 years) and 12 children were initially recruited for the study. Three children were excluded from the final analysis. One child aborted the measurements prematurely due to anxiety. The other two children were excluded due to high motion during the scanning session (see section “Behavioral and Motion Analysis”). The final children sample therefore comprised nine children (two females, nine males), aged 7 years, 11 months to 9 years, 8 months (9.0 ± 0.7 years). Of those nine children, one child had the age of 7, three were aged 8 years and five were 9 years old.
All participants had normal or corrected-to-normal vision. Subjects with corrected-to-normal vision wore ophthalmic lens during the MR scanning session that were individually adjusted to their respective visual acuity prior to lying into the MR scanner. Before the procedure, parents were asked to confirm that their children had no history of psychiatric or neurological disorders, as was assured with adult subjects, as well. Self-reported right-handedness was used as selection criterion during the recruitment process. To ensure right-handedness, subjects were asked to complete the Edinburgh Handedness Inventory Questionnaire (Oldfield, 1971). According to this questionnaire, all children were right-handed with a mean laterality quotient (LQ) of +89.8. In the adult sample 9 out of 10 subjects were right-handed with a mean LQ of +88.3. One adult subject turned out to be left-handed with a LQ of −33.3. We included this subject for the first analysis in which we assessed in how many subjects it was possible to localize the core system’s brain regions on the individual subject level. We excluded this subject from the second analysis in which we compared adults and children, since it is known that activation strength and hemispheric lateralization can be influenced by handedness (Willems et al., 2010; Bukowski et al., 2013; Frässle et al., 2016a).
In the present study, we wanted to ensure that no children are included that show autistic traits. They were therefore asked to complete the Autism Spectrum Quotient Questionnaire (AQ; Baron-Cohen et al., 2001) and the Empathy Quotient Questionnaire (EQ; child-version: Empathy-Systemizing-Quotient Questionnaire/EQ-SQ-Child; Baron-Cohen and Wheelwright, 2004). The evaluation of EQ and AQ showed that all children scored in normal range. We also administered the same screening procedure to the adult subjects. Two of the adult subjects achieved a slightly below-average EQ score and one of these subjects was additionally slightly above-average in the AQ score. We decided to include these subjects in further analyses since deviations in EQ and AQ score were not defined as exclusion criteria for adults. Additionally, we ensured that these subjects showed the typical activation pattern in the core system of face processing. To assess overall cognitive abilities in children, the brief version of the standardized Wechsler Non-verbal Scale of Ability Test (Wechsler and Naglieri, 2006) was applied. As none of the children displayed major cognitive deficits, all nine children subjects were included in further analyses.
All subjects provided written informed consent after they were apprised in detail about the experimental set up and the study procedure. The study was approved by the local Ethics Committee of the Department of Psychology of the Justus-Liebig University in Giessen, Germany (reference number 2018-0024). In case of minor subjects, their parents provided informed consent. After study participation, adult subjects received an allowance of 10 Euros and children could choose between different toys.
MRI Investigation of Children
The children were invited to visit the MR scanner facilities a couple of days in advance to the scanning session to familiarize themselves with the MR scanner environment, to reduce anxiety and discomfort and to minimize occurrence of strong motion artifacts. The individual training session started with a chair circle, including the participating child, one or both of its parents, two instructors and a professional radiographer, who conducted the actual scanning session. A playful theoretical introduction gave insights into the experimental procedure and the fMRI method. The coloring book Paula in der Röhre [Bayer Health Care (Hg), 2008] was used for explaining the procedure. In this booklet the fMRI technique and aspects to consider when lying in the MR scanner are taught in a child-friendly narrative. The book was sent to the child prior to the training session with the appeal to take a look at it. In the training session, the story of the booklet was discussed with the child and possible questions were answered. Children were made aware of the extreme importance of lying still during the scanning session, by making use of the comparison between motion artifacts and blurry photographs (Wilke et al., 2018). In addition, children were shown some exemplary images of face and house stimuli from the fMRI paradigm to give them an impression of the experimental task.
The fMRI study was embedded in a child-oriented setting, putting the whole experiment in a frame story. The child was told to imagine being an astronaut who is flying in a rocket (i.e., the MR scanner). To motivate the child, a cuddly toy was brought into the story, which accompanies the child as a co-astronaut during the whole training and actual scanning session. By using the notion of the narrow interior of the rocket, the child should lose discomfort induced by the tightness inside the MR scanner. Intense background noise was explained as noise produced by the rocket when speeding up. The importance of lying still inside the MR scanner was further underlined by the story as resting still is crucial for a smooth steering of the rocket. To bring the stimuli of the fMRI paradigm into the frame story, children were told that on their journey through the universe they encounter people living in their houses on different planets, showing different reactions when seeing the rocket passing.
After introducing the child to the frame story, she/he had the possibility to inspect the scanning room and the MR scanner. Together with the radiographer, the child could first view the scanner from the outside. Afterward, the child was invited to lie in the scanner to get a feeling for the tightness of the tube. The head coil was also mounted for test purposes, being explained as the helmet of the astronaut. On the day of the scanning session, children were reminded of the frame story and of things to consider when lying inside the scanner.
Experimental Paradigm
For MRI data acquisition, participants laid in supine position in the scanner with their head first. Light inside and outside the scanner was switched off to strengthen the children’s feeling of being situated in a spacecraft in the universe. A response box with one button was fixated on the right thigh of the subjects for conducting a one-back matching task during the fMRI paradigm. To prevent motion artifacts, soft foam rubber pads were used for head fixation. Stimuli were presented via an MRI-compatible LCD screen that was positioned behind the MR scanner. Subjects viewed the paradigm through a 45° tilted mirror which was fixated at the head coil. All stimuli were presented using the software package Presentation (version 20.2, Neurobehavioral Systems, San Francisco, CA, United States).
The face processing network was investigated using a face localizer paradigm in which subjects viewed either gray-scale faces with neutral, sad or fearful expressions in the activation condition or houses in the control condition in a blocked design (see Figure 1 for details). To ensure attention, participants were instructed to indicate via button press with the right index finger when a stimulus was shown twice consecutively.
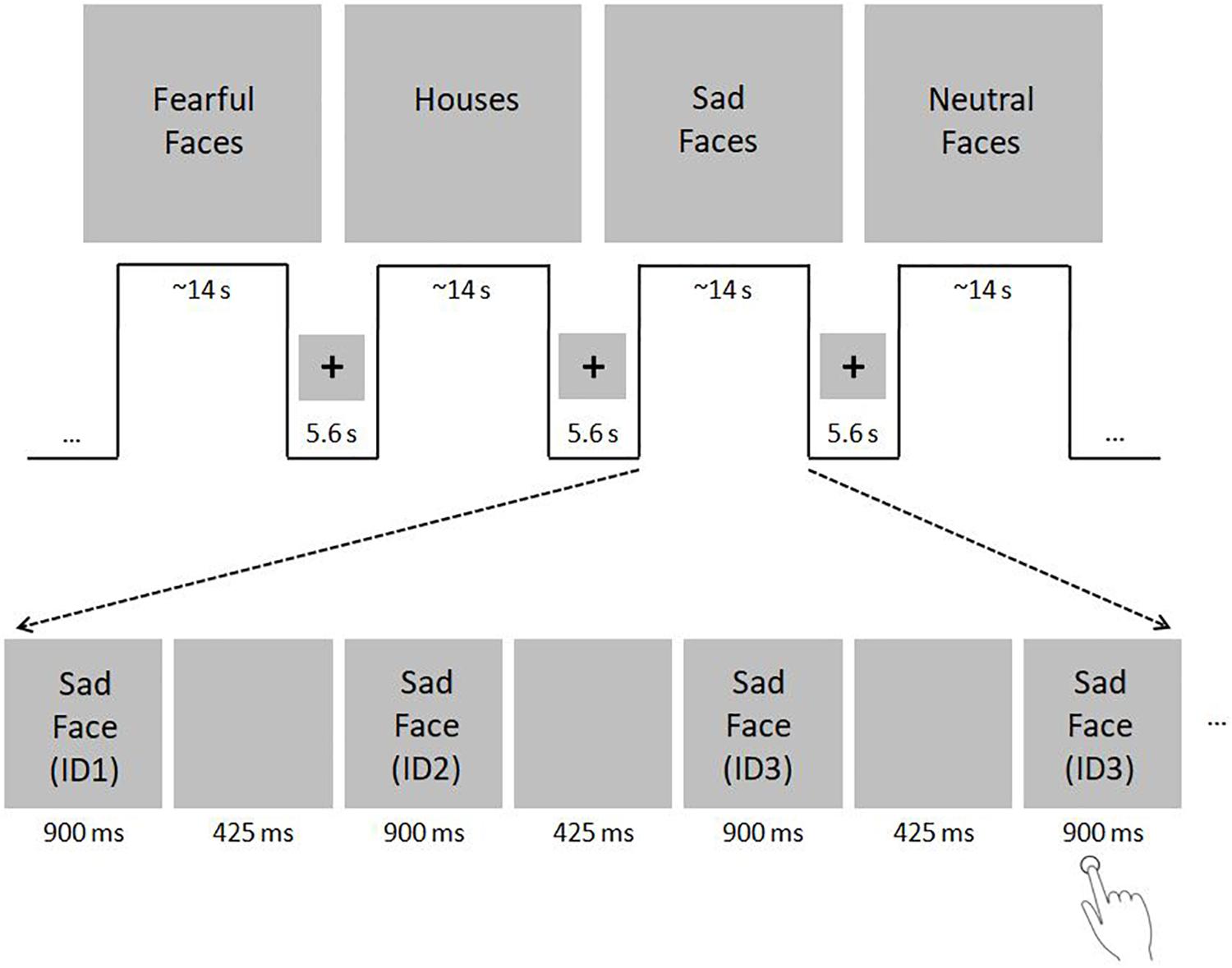
Figure 1. fMRI paradigm. Subjects viewed either gray-scale faces with neutral, sad or fearful faces in the activation condition and houses in the control condition in a blocked design. Face stimuli were selected from the Karolinska Directed Emotional Faces (KDEF) dataset (http://www.emotionlab.se/resources/kdef; permission for publication of KDEF stimuli was kindly provided by the Karolinska Institutet, Department of Neuroscience, Section of Psychology, Stockholm, Sweden; Lundqvist et al., 1998). House stimuli were selected from the internet. To ensure equalized size of face and house images, stimuli were resized to 500 × 400 pixel using ImageMagick for Linux. For purpose of color and luminance matching, stimuli were transferred into gray-scale images and adjusted for mean luminance using the SHINE toolbox for MATLAB (Willenbockel et al., 2010). The paradigm consisted of two sessions, including 16 blocks each (4 blocks with neutral, sad and fearful faces, respectively; 4 house blocks). The sessions were divided by a short break of 20 s. Each block included 11 stimuli that were presented for 900 ms with an inter-stimulus interval of 425 ms. Blocks lasted ∼14 s each and were separated with blank periods (duration: 5.6 s) in which only a centered fixation cross was shown. The order of blocks remained the same across all subjects, whereas the order of images in each block was pseudo-randomized. To ensure attention, subjects were asked to indicate via button press with the right index finger when a stimulus was shown twice consecutively. Within one block, either two or three stimulus-pairs arose, which sum up to 40 target events in the whole fMRI paradigm. The total duration of the fMRI paradigm was ∼11 min. The fMRI paradigm used in the present study was a slightly modified version of the standard paradigm used in our group (e.g., Frässle et al., 2016b) to adapt for the assessment of children. First, to minimize the total scanning time, the number of blocks was reduced from 44 to 32. Second, the stimulus presentation time was tripled to 900 ms. Third, the number of stimuli per block was reduced from 20 to 11. Fourth, the number of different face identities was reduced from 30 to 20 (10 female and 10 male identities). The number of different houses was also reduced from 30 to 20, to match it with the number of face stimuli.
MRI Data Acquisition
Subjects were scanned on a 3-Tesla MR scanner (Siemens Prisma 3-Tesla Magnetom) at the Bender Institute of Neuroimaging (BION) at the Justus-Liebig University of Giessen, Germany. All MRI data were acquired using a 64-channel head matrix receive coil.
First, a high-resolution anatomical image was acquired using a T1-weighted magnetization prepared rapid gradient echo (MPRAGE) sequence. The following parameters were applied: acquisition time (TA) 4:29 min, repetition time (TR) 1580 ms, echo time (TE) 2.30 ms, field of view (FOV) 240 mm, 176 slices, slice thickness (ST) 0.94 mm, resolution 0.9 × 0.9 × 0.9 mm, phase encoding direction (PE) anterior ≫ posterior, distance factor (DF) 50%, flip angle 8°, bandwidth 200 Hz/Px, sagittal ascending acquisition.
Second, functional images were collected using a T2∗-weighted gradient echo-planar imaging (EPI) sequence sensitive to Blood Oxygen Level Dependent (BOLD) contrast. The following parameters were used: TA 11:14 min, TR 1780 ms, TE 36 ms, FOV 256 mm, 20 slices per slab, ST 2.4 mm, resolution 2.0 × 2.0 × 2.4 mm, PE anterior ≫ posterior, DF 20%, flip angle 70°, bandwidth 1396 Hz/Px, ascending acquisition. We did not measure the whole brain, but only a slab (Figure 2). Reducing the coverage allows reduction of the voxel size and therefore an increased spatial resolution. The measurement of a slab of the brain is believed to facilitate the measurement of small regions (e.g., amygdalae; Morawetz et al., 2008). The slab was manually orientated, using the structural T1-weighted image. We aimed to cover on the one hand all three regions of the core face processing network, i.e., bilateral OFA, FFA, and pSTS. On the other hand, we also aimed, as part of a related project, to measure activity in parts of the extended system, in particular the amygdala, insula, cingulate gyrus, and inferior frontal gyrus. These brain regions are known to play an essential role in emotion processing across development.
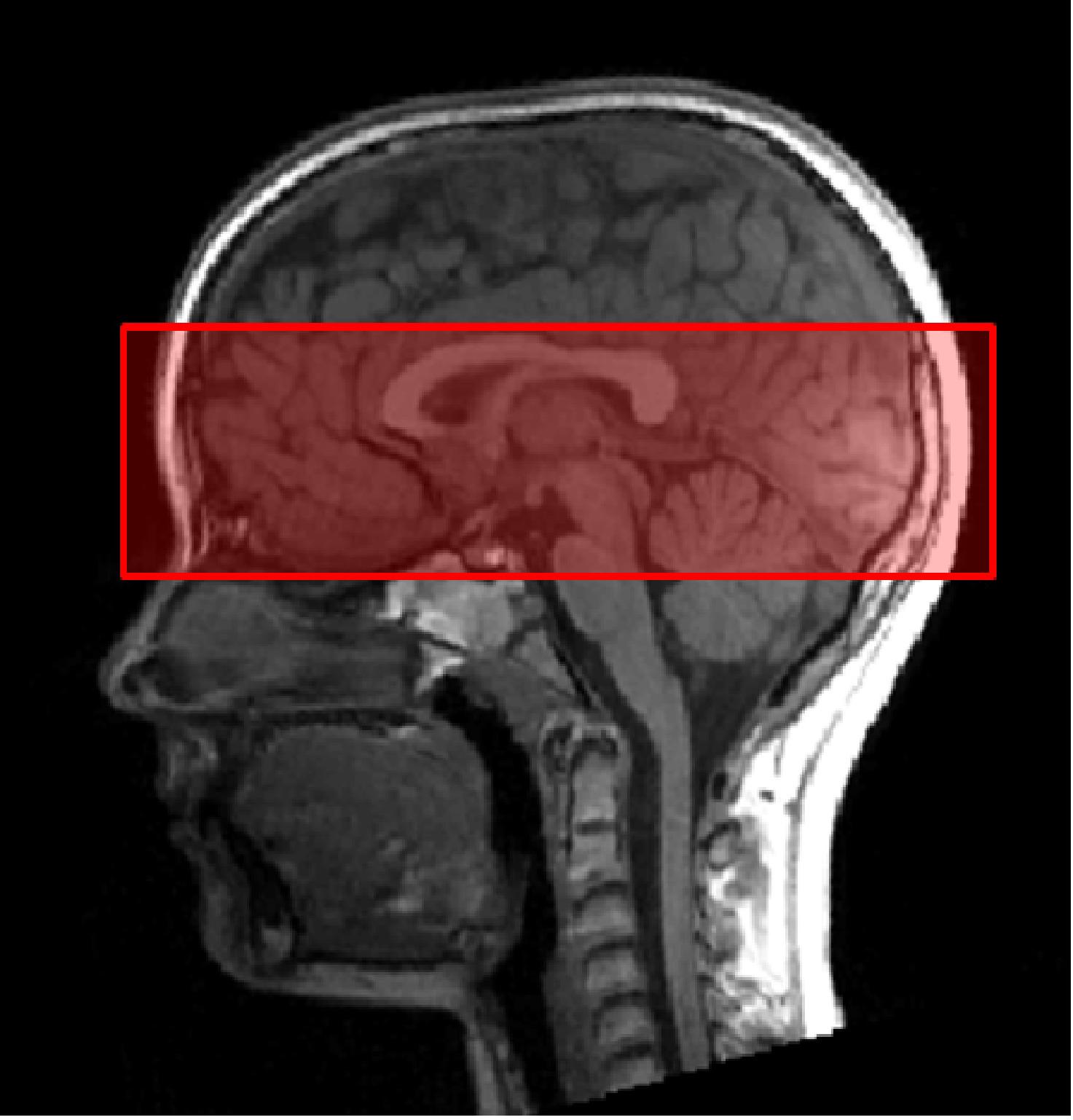
Figure 2. Slab orientation covering bilateral OFA, FFA, and pSTS. The slab was oriented at the lowest part of the occipital pole and the lowest part of the prefrontal cortex (PFC), using the middle view of the structural image.
MRI Data Analysis
MRI data were analyzed using Statistical Parametric Mapping (SPM12, version 7219, Wellcome Trust Centre for Neuroimaging, London, United Kingdom), based on MATLAB (version 9.1, R2016b).
Preprocessing: To control for head movements, functional images from both sessions were realigned to the mean image. Realigned images were coregistered with the high-resolution anatomical image and then spatially normalized into the Montreal Neurological Institute (MNI) standard space using the unified segmentation-normalization of the anatomical image. Normalized functional images were spatially smoothed using an isotropic 6 mm full width at half maximum Gaussian kernel.
Statistical analysis was performed in a two-level, mixed-effects procedure. At the individual subject level, voxel-wise BOLD activity was modeled by a General Linear Model (GLM). Each condition of the face-localizer paradigm, i.e., neutral, sad and fearful faces, respectively, as well as houses, was modeled as a block regressor. This regressor was convolved with the hemodynamic response function implemented in SPM12. The regressors for the two sessions were entered into two separate sessions in one GLM (i.e., were not concatenated). In addition, the six realignment parameters of each session were included in the GLM design matrix as nuisance regressors to control for movement-related artifacts not accounted for by the realignment during preprocessing. A high-pass filter (cut-off frequency: 1/128 Hz) was used to account for low-frequency noise. Individual BOLD activity related to face processing was identified by a contrast comparing faces (irrespective of emotional content) against houses (Δβ = βfaces – βhouses), averaged across sessions (i.e., setting for both sessions the contrast weight “1” on each face condition and “−3” on the house condition). In the following, we will refer to this contrast as “faces > houses.” To assess brain activation at the group level, the “faces > houses” contrast images were entered separately for children and adults into one-sample t-tests. Anatomical localization of the activated brain regions was achieved using the WFU-Pickatlas (Maldjian et al., 2003).
Quality control: First, a motion analysis was performed to rule out that potential activation differences between children and adults were caused by unequal motion artifacts. The motion analysis was performed by MotionEstimator, developed by one of the authors (RK). MotionEstimator calculates location differences between two scans.1 As cut-off criterion for exclusion from further analysis, we chose a mean scan-to-scan motion exceeding 0.35 mm (Power et al., 2015). Second, further quality control was conducted using the software package MRIQC (Magnetic Resonance Imaging Quality Control2, 3). MRIQC assesses both structural T1-weighted MR images and BOLD-images of the brain by calculating a set of quality measures from each image (Esteban et al., 2017). MRIQC uses 14 Image Quality Metrics (IQMs) that characterize each image in 56 features. The tool also includes a visual reporting system in order to manually investigate potential quality issues in single subjects.
Analysis Strategy
First aim of the study was to assess whether it is possible to detect brain activity in the core system of face processing in children at the single subject level. We proceeded in two steps. First, we analyzed the group activation pattern for the contrast “faces > houses” separately for adults and children using one-sample t-tests. Second, we analyzed the individual activation patterns.
In these activation patterns, we determined whether brain activity could be found in the left OFA, right OFA, left FFA, right FFA, left pSTS and right pSTS. The brain activation patterns were first thresholded at a conservative threshold of p < 0.05, corrected for multiple comparisons at the whole brain level (family wise error, FWE, corrected at the voxel level). If brain activity was not found in all regions of the core system at this threshold, the p-value was subsequently lowered to more liberal thresholds (p < 0.001 and p < 0.05, respectively, uncorrected for multiple comparisons) (see Schuster et al., 2017 for an extensive discussion of this procedure).
To assess whether or not a specific activation can be attributed to the core system of face processing, we created three anatomically defined regions of interest (ROIs) including bilateral OFA, FFA, and pSTS, respectively, using the WFU-Pickatlas (Maldjian et al., 2003). OFA-ROI masks were created choosing the inferior occipital gyrus in the brain atlas IBASPM116 (as implemented in the WFU-Pickatlas). FFA-ROI masks were built choosing the fusiform gyrus. pSTS-ROI masks were created choosing the superior and middle temporal gyrus. Activation clusters that appeared inside one of the ROI masks were considered as potential candidates of core system brain activity. To verify the correct anatomical localization, both the anatomical localization on the canonical single-subject T1-image (implemented in SPM12) and the positions of the activated brain regions in the occipito-temporal lobe relative to each other were used. This identification procedure was performed by four individual raters (authors FH, ID, RK, KZ) separately to maximize accuracy and minimize error-proneness due to inter-rater differences.
Second aim of the study was to compare hemispheric lateralization and activation strength of brain regions in the core system of face processing between adults and children. Central focus was the assessment of differences in the hemispheric lateralization of FFA activity.
Activation strength: We decided against applying the standard approach for a group analysis, i.e., assessing voxel-wise differences in normalized functional images between both groups using a two-sample t-test, since the normalization procedure might have introduced systematic differences between these groups. Instead, we created for each subject individual spherical masks (radius 6 mm) centered at the corresponding local maximum of the six brain regions of the core system. Activation strength was calculated as the mean value of all voxels inside the respective mask for the weighted β-image (contrast “faces > houses”).4 Activation differences between both groups were assessed with Welch-tests in R.5
Lateralization: The degree of regional face-sensitive hemispheric lateralization was assessed by a lateralization index (LI) (Jansen et al., 2006). The LI is given by the following expression
where AL and AR refer to values of fMRI-measured activity for homologous ROIs within the left (L) and right (R) hemisphere. The LI yields values between 1 and −1. In the present study, an LI > 0.20 was considered to represent left-hemispheric dominance and an LI < −0.20 right-hemispheric dominance. An LI between −0.20 and 0.20 was denoted as bilateral (Springer et al., 1999).6
For calculation of the LI, we used the bootstrap procedure implemented in the LI tool-box extension (Wilke and Schmithorst, 2006; Wilke and Lidzba, 2007). This method takes 100 bootstrapped samples (resampling ratio k = 0.25) for the ROIs in the left and right hemisphere for 20 equally sized thresholds ranging from 0 to the maximum t-value. This results in 10,000 possible LI combinations, from which only the central 50% are kept to exclude statistical outliers. For each subject, a representative LI is then calculated by weighting these central 50% LIs with their respective threshold. Using this procedure, the LI was computed for face-sensitive activation in the OFA, FFA and pSTS. To specify the ROIs for LI calculation, individual masks for each subject’s bilateral OFA, FFA and pSTS were generated using the WFU-Pickatlas. Individual masks were created as spheres of 10 mm radius around the previously identified MNI-coordinates of each ROI. Lateralization differences between both groups were assessed with Welch-tests.
Power analysis: Since we did not have empirical estimates for the expected effect size of the FFA lateralization differences between children and adults, the present results were intended to build the basis for an informed power analysis yielding the necessary group size for a larger follow-up study. The power analysis was performed using the software G∗Power (version 3.1; Faul et al., 2009). The sample effects detected in the present study were used as an estimate of the population value of the effect to be detected in consecutive studies (Anderson et al., 2017). The effect size was calculated from the mean effect of the children sample, the mean of the adult sample and the standard deviation of each group. For calculation, statistical power was set to 0.8 (80%) and the alpha error probability was set to α = 0.05 (p < 0.05). We used an unbalanced adults/children allocation ratio of 1.4. To achieve the same power, unbalanced designs have to include more subjects than balanced study designs. We nevertheless decided to perform the power analysis for this design, since it allowed us to reduce the number of children (difficult to recruit) at the cost of including overall more adult subjects (easy to recruit).
Behavioral and Motion Analysis
Analysis of the behavioral data was performed for all included subjects (adults: 10, children: 9). The one-back matching task successfully kept the attention of both groups who showed a mean performance of >90% accuracy (adults: 97.90% ± 2.82, children: 94.27% ± 10.65).
Motion analysis was performed for all 10 adults and 11 children. None of the adult subjects showed a mean scan-to-scan motion exceeding the defined cut-off score of >0.35 mm (Figure 3, bottom). They showed an averaged mean scan-to-scan motion of 0.11 mm ± 0.05 mm in the first run and 0.12 mm ± 0.06 mm in the second run. However, two children showed a mean scan-to-scan motion exceeding > 0.35 mm in the second run (C03, C10; Figure 3, top). They were therefore excluded from further analyses. Average mean scan-to-scan motion of the remaining 9 children was 0.09 mm ± 0.03 mm in the first run and 0.11 mm ± 0.05 mm in the second run. An independent samples t-test revealed no significant difference in the mean scan-to-scan motion of the first and second run between the two groups (first run: p = 0.329, second run: p = 0.656), and was therefore characterized as “comparable.” This rules out that potential activation differences between children and adults are caused by unequal motion artifacts.
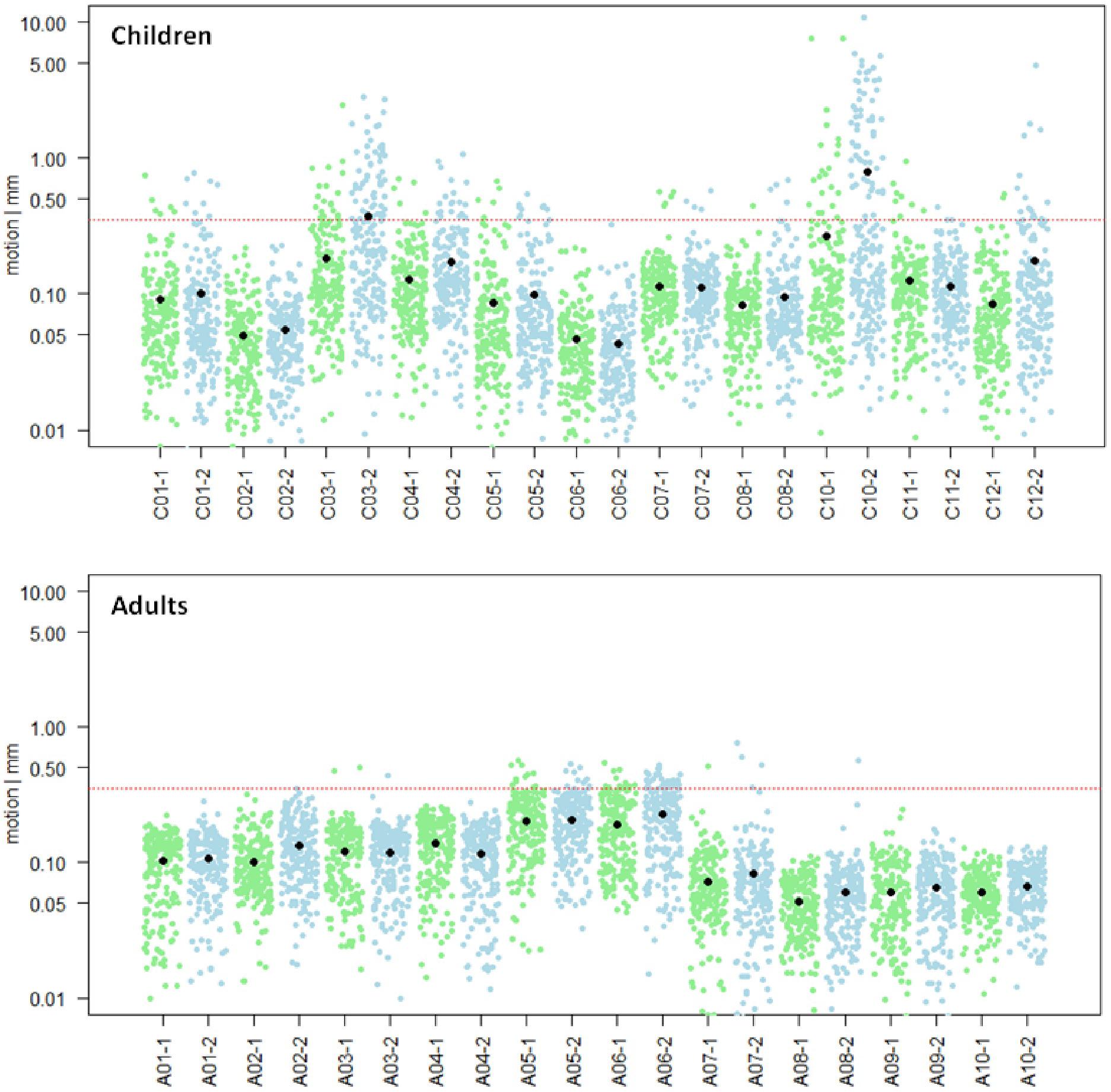
Figure 3. Motion analysis. Mean scan-to-scan motion of the child (n = 11, top) and the adult (n = 10, bottom) sample, separately for each subject and each session. The first session is depicted in green, the second session in blue. On the x-axis, the subject and session are specified; on the y-axis, the mean scan-to-scan motion is given in logarithmic representation to show normal distribution of data points. The red line marks the cut-off score of a mean scan-to-scan motion threshold of 0.35 mm. The black dots represent the mean scan-to-scan motion. Child C03 and Child C10 were excluded from further analyses due to motion exceeding the predefined session-specific mean scan-to-scan motion threshold of >0.35 mm in the second session.
Results
Do Children Activate the Core System of Face Processing?
Our first aim was to assess whether it is possible to detect brain activity in the core system of face processing in children at the single subject level. For illustrational purposes, a representative brain activation pattern is shown in Figure 4. At the group level, we found in the adults group clearly discernible face-sensitive brain activity in bilateral OFA and bilateral pSTS (p < 0.001, uncorrected). In the children group, we found brain activity in the right OFA and bilateral pSTS at p < 0.001, uncorrected. The left OFA was activated at p < 0.05, uncorrected. In contrast, the left and right FFA was not activated in both groups, not even at a liberal threshold of p < 0.05, uncorrected (see discussion for an explanation). At the individual subject level, all regions of the core system of face processing could be identified in almost all subjects. In most cases, activity was found even at conservative statistical thresholds, i.e., at p < 0.05, FWE corrected for multiple comparisons at the whole-brain level. These results suggest that the core face processing regions, i.e., bilateral OFA, FFA and pSTS can be portrayed at the single-subject level in children and adults, with 100% ROI identification scores of OFA in both samples and slightly lower ROI identification scores of bilateral FFA and pSTS (see Figure 5 for details).
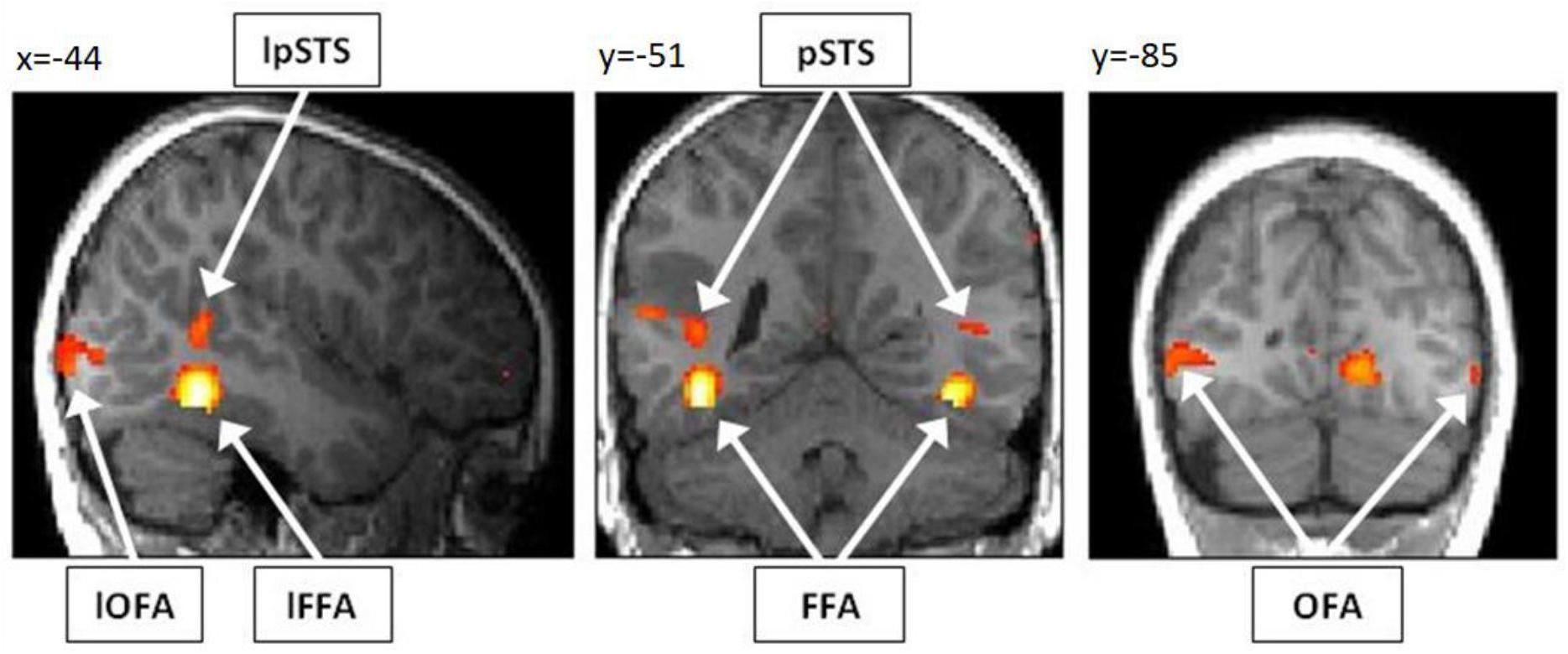
Figure 4. Activation pattern of a representative child (7 years, male) for the contrast “faces > houses.” As overlay T1 image, the normalized and smoothed structural T1 scan of the respective child was used. The subject shows clearly discernible activity in bilateral OFA, FFA and pSTS. For illustrational purposes, the activation pattern is thresholded at p < 0.001, uncorrected for multiple comparisons. Note, however, that all six activations were significant for multiple comparisons (p < 0.05 FWE corrected) at the predefined ROI masks for bilateral OFA, FFA, and pSTS. OFA, occipital face area; FFA, fusiform face area; pSTS, posterior superior temporal sulcus; l, left.
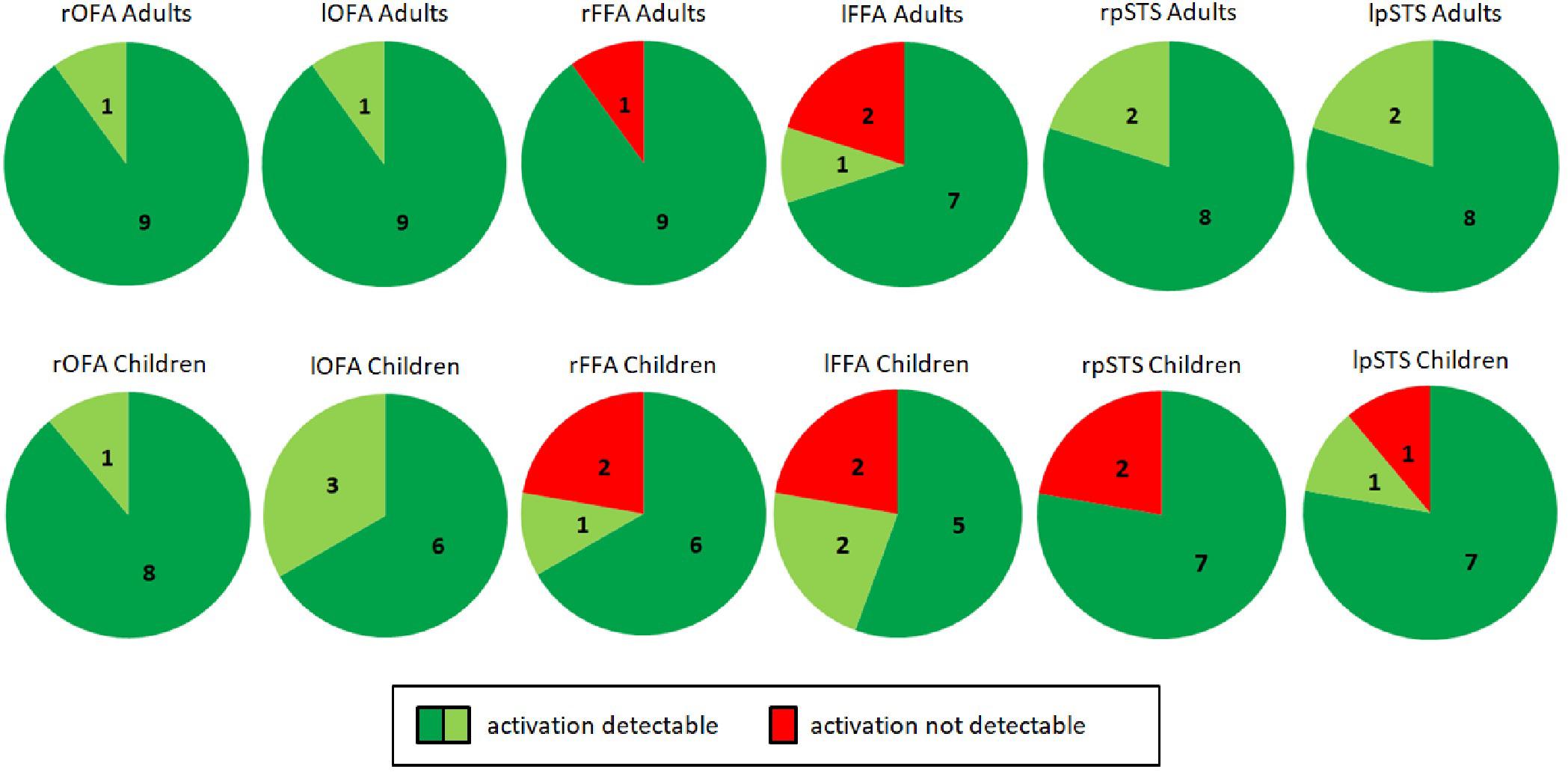
Figure 5. Identification of bilateral OFA, FFA, and pSTS in adults (top, n = 10) and children (bottom, n = 9). Dark green indicates activity detectable at a statistical threshold of p < 0.05, FWE corrected for multiple comparisons at the whole brain level. Light green indicates activity detectable at a statistical threshold of p < 0.001/p < 0.05 uncorrected. Red indicates no detectable activity. In all adults, brain activity could be detected in the right and left OFA as well as in the right and left pSTS. Activity could not be detected in the right FFA for one subject (A02) and the left FFA for two subjects (A02, A07). This was caused by the positioning of the measured volume (see section “Discussion”). As for adults, in all children brain activity could be detected in the right and left OFA. Activity was not detected for two children in the right pSTS (C04, C06) and for one child in the left pSTS (C04). Activity was also not detected for two children in the right FFA (C07, C08) and for two children in the left FFA (C01, C08). Missing FFA activity was again caused by the positioning of the measured volume. OFA, occipital face area; FFA, fusiform face area; pSTS, posterior superior temporal sulcus; l, left; r, right.
Do Children and Adults Differ in Brain Activation and Hemispheric Lateralization?
Our second aim was to compare hemispheric lateralization and brain activity in the core system of face processing between adults and children, with a main focus on the lateralization of FFA activity. The mean activation is summarized separately for both groups for the left and right OFA, FFA and pSTS in Figure 6 (top). Mean activity was, as expected, stronger in bilateral OFA and FFA for adults than for children (albeit the FFA differences were only marginal). In contrast, mean activity for bilateral pSTS was unexpectedly higher for children compared to adults. However, none of the differences reached statistical significance (Table 1). The mean lateralization is summarized in Figure 6 (bottom). For adults, the mean LI was bilateral for OFA (0.15 ± 0.45, range: −0.57–0.64) and right-dominant for FFA (−0.32 ± 0.52, range: −0.96–0.49) and pSTS (−0.64 ± 0.27, range: −0.93–−0.17). For children, the LI was bilateral for OFA (−0.04 ± 0.48, range: −0.64–0.76) and FFA (0.07 ± 0.48, −0.57–0.76), but right-dominant for pSTS (−0.27 ± 0.46, range: −0.85–0.58). Again, none of the differences reached statistical significance (Table 1).
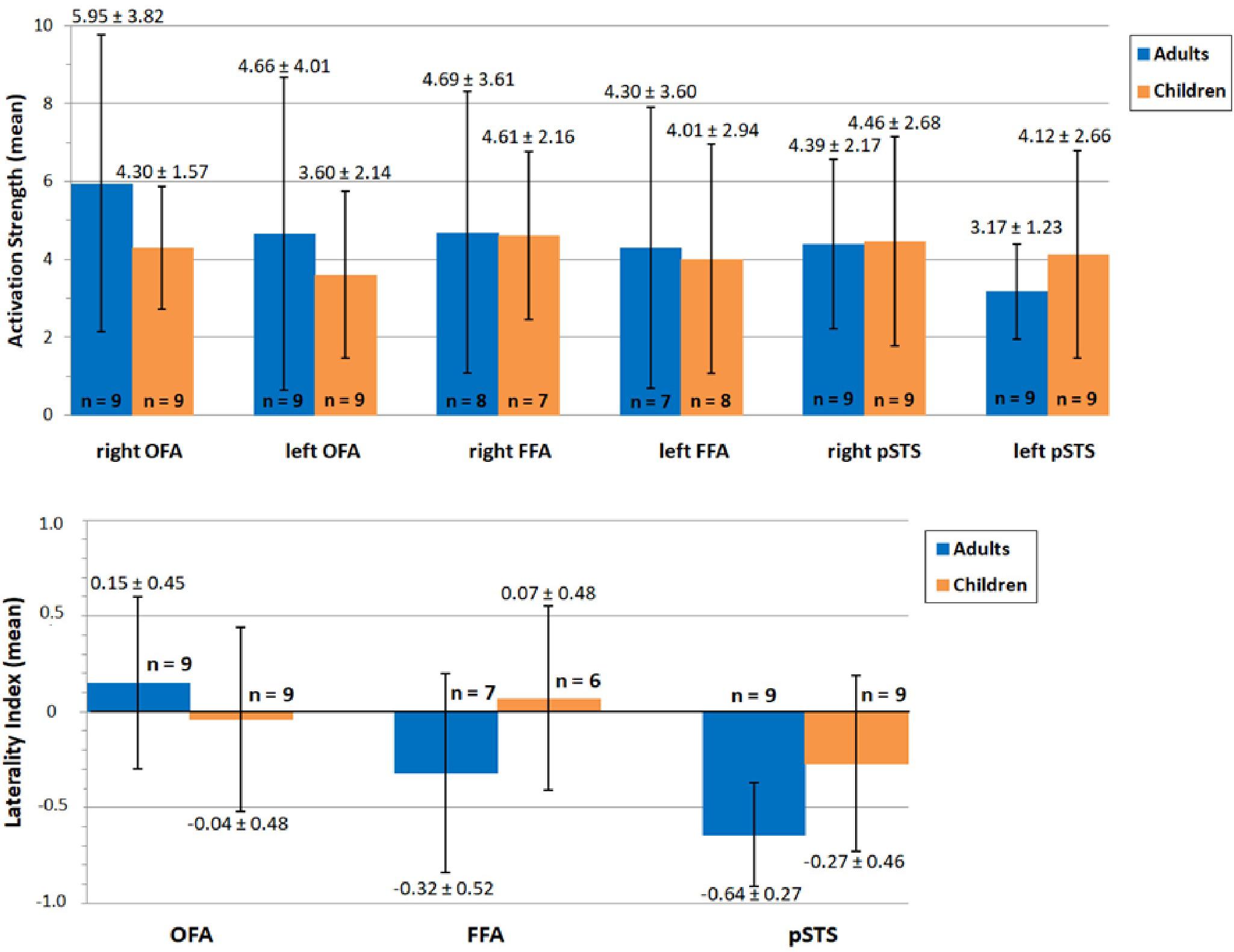
Figure 6. Mean activation (top) and lateralization (bottom) of OFA, FFA and pSTS during face processing in adults (blue) and children (orange). Values of mean activation/lateralization ± SD are specified above/below error bars. The number of included subjects is depicted at the bottom of/above each bar. None of the differences reached statistical significance (p > 0.05). OFA, occipital face area; FFA, fusiform face area; pSTS, posterior superior temporal sulcus.

Table 1. T- and p-values of the Welch t-test, comparing activation and lateralization differences between adults and children for OFA, FFA, and pSTS.
The effect size for the FFA lateralization difference between children and adults was estimated as d = 0.78. Based on this estimate, we performed a power analysis to calculate the group size for a sufficiently powered follow-up study (statistical power 0.8, alpha error probability 0.05, unbalanced adults/children allocation ratio of 1.4). This analysis yielded a necessary sample size of n = 44 (18 children, 26 adults).
Discussion
The first goal of the present study was to establish an fMRI paradigm suitable for assessing activation in the core system of face processing in young children. We showed that it is possible to localize the core system’s brain regions in young children even at the single subject level. The second goal was to collect data for an informed power analysis to calculate the necessary group size for a large-scale cross-sectional imaging study assessing the ontogenetic development of the face processing network, in particular with regard to the lateralization of the FFA. Our results showed the expected right-hemispheric lateralization in adults. In contrast, the children’s brain activation pattern was more bilateral. The effect size of the FFA lateralization difference was estimated as d = 0.78, yielding a necessary sample size of n = 44 (18 children, 26 adults) for an adequately powered follow-up study. In the following, we will discuss these findings in more detail.
Do Children Activate the Core System of Face Processing?
Our first aim was to set up a paradigm for assessing activation in the core system of face processing in children at the single subject level. We modified the “standard” fMRI face processing paradigm that we (and others) are using in adults to make it more suitable for the measurement of young children. Much effort was spent on the thorough preparation of the children for the MRI scanning session. The MRI scanning session was put in a child-appropriate frame story. Children slipped into the role of an astronaut on a journey through the universe. Only one child aborted the measurement prematurely due to anxiety, while the other children completed the measurements without problems. When asked after data acquisition, children did not report any feeling of anxiety, but rather curiosity about the device.
Children typically have more difficulties in staying motionless during data acquisition than adults. One of the biggest problems in fMRI of young children is therefore motion artifacts. A recent study from Wilke et al. (2018) reported for instance a positive correlation between proceeding scanning time and the occurrence of motion artifacts in children. We therefore shortened the paradigm, compared to the standard task used in our lab in adults, and additionally split it into two parts with a break of 20 s in-between, in order to have the possibility to analyze only data of the first part, if motion artifacts would have increased with proceeding time in the second part. In fact, both parts of the experiment of 9 out of 11 children data could be used thanks to a thorough preparation in advance. The first appointment with the children and their caregivers was crucial, as it gave us the opportunity to convey the importance of lying still to the children. In addition, the few days between the first and second appointment enabled the children to internalize the fMRI procedure. Using a thorough motion analysis, 9 out of 11 children passed the stringent, a priori chosen motion threshold. The overall motion of the included subjects was comparable between children and adults. This rules out that potential activation differences between children and adults were caused by unequal motion artifacts.7
The left and right OFA was identified in all children and adults. Also the left and right FFA could be localized in most subjects. At first view, it was puzzling that both the left and right FFA were not activated (even at liberal thresholds) at the group level, but in most participants, both adults and children, at the individual subject level even at conservative thresholds. A more detailed analysis showed that this finding could be explained by the positioning of the measured brain volume. As described in the methods section, we did not measure the whole brain, but a “slab” (Figure 2). The slab was manually positioned with help of the high-resolution structural image. In previous studies of our research group, we used a lateral view of the structural image and oriented the slab at the lowest part of the occipital pole and the lowest part of the inferior temporal gyrus. This positioning ensured that all three regions of the core face processing network (i.e., bilateral OFA, FFA, and pSTS) could be measured. In the present study, however, we also aimed, as part of another project, to cover parts of the extended system that are known to play an essential role in emotion processing (in particular the amygdala, insula, cingulate gyrus, and inferior frontal gyrus). We therefore used the middle view of the structural image and oriented the slab at the lowest part of the occipital pole and the lowest part of the prefrontal cortex. Due to the different positioning procedure, the FFA was now located at the border of the measured brain volume and was accidentally cropped off in two adults and two children. Since SPM12 does not depict group brain activity in voxels in which at least one subject was not assessed, we were not able to detect activity at the group level. The goal of choosing the defined slab to cover the above-mentioned areas of the extended face processing network in high resolution was a compromise that made it difficult to analyze the data at group level due to “cutting off” the FFA in a few subjects. Future studies therefore have to make sure that all relevant brain regions are included in the measured brain volume. This can be achieved either by measuring a larger brain volume [at the cost of a higher acquisition time per volume (i.e., higher TR) and/or a lower spatial resolution] or choosing a different positioning procedure (orienting the slab at the lowest part of the occipital pole and the lowest part of the inferior temporal gyrus using a lateral view of the structural image). However, if possible, it is advisable to narrow down the target areas and the focus of the study prior to fMRI data acquisition. Another possibility to prevent partial cropping of regions of interest (especially the IFG and the dorsal part of the pSTS) would be to adopt a multi-band imaging protocol instead of using a slab of the brain for data acquisition.
pSTS activation was identified in all adults, but not in two children. Differently from the FFA, missing activity could not be explained by the positioning of the slab since the pSTS was clearly within the measured brain volume for all subjects. It has been previously reported that it is more difficult to localize the pSTS than OFA and FFA if static stimuli (as in the present study) are used, since the pSTS has been associated with the processing of changeable facial features (e.g., gaze direction, lip movements; Ishai et al., 2005; Fox et al., 2009). More data is needed to further investigate whether the non-identification of pSTS activity in two subjects is well in line with previous studies using static face localizers (Rhodes et al., 2009; Schultz and Pilz, 2009) or whether it hints at different processing strategies between children and adults.
Do Children and Adults Differ in Hemispheric Lateralization?
Our second aim was to compare brain activation in the core system between children and adults. The central question was the development of the lateralization of the FFA. We hypothesized that children would show a more bilateral brain activation pattern. In exploratory analyses, we also compared hemispheric lateralization of OFA and pSTS activity as well as the activation strength itself between both groups. We hypothesized that children would show reduced hemispheric lateralization also for OFA and pSTS activity as well as reduced activity in all brain regions of the core system (i.e., bilateral OFA, FFA, pSTS).
In fMRI, activation differences between two groups are typically assessed by voxel-wise comparisons of normalized functional images. However, in the present study this analysis would have required the use of the same template (e.g., the MNI template) to normalize the data of both children and adults. Since the brains of children and adults largely differ in size and form, this normalization process might have introduced systematic differences between both groups. It would not have been possible to exclude that potential group differences between children and adults both in location and activation strength might simply be related to differences in the normalization process. To surpass this problem, we determined the location of all core system’s brain regions individually for each subject and calculated the activation strength from these regions. Our group comparison thus avoided a potentially biased voxel-wise comparison.
Since we determined the brain activity at the individual level, we could have completely omitted the normalization process. We nevertheless decided to introduce a normalization step and assess brain activity in the normalized images since it helped us to assign activations with more certainty to specific face regions. For the decision whether specific brain activation is for instance assigned to the OFA or the FFA, we could additionally rely on previously published data that is available typically only for normalized data.8
The main focus of the present study was the assessment of the development of hemispheric lateralization, in particular of the FFA. Our results showed a trend for increased lateralization of all three regions, with the largest effect found for the FFA. The estimated effect size (d = 0.78) denotes medium to large effects. Using our results as basis for an informed power analysis, we estimated that an adequately powered (sensitivity 0.8) follow-up study testing developmental changes of FFA lateralization would require the inclusion of 18 children and 26 adults. Our results also showed, as expected, a trend for weaker activity of children’s bilateral OFA. This finding is in line with theories postulating an increase of face-selective activation in the core system due to an age-related increase of functional specialization (Scherf et al., 2007; Joseph et al., 2011). In contrast, the FFA activity between adults and children was comparable. Unexpectedly, we found for the pSTS a trend for higher activity in children compared to adults (even if we included the children without detectable pSTS activation in the comparison, see results). There are several possible explanations for this hyperactivity. On the one hand, it might be related to the development of the pSTS as a heteromodal association area which builds an interface between sensory signals and hierarchical higher areas (Gogtay et al., 2004). In case of the face-processing network, the bilateral pSTS builds an interface between the core and the extended face-processing network (Hoffman and Haxby, 2000). It might be argued that bilateral pSTS hyperactivation in children is driven through the close link to the hyperactivated extended face-processing network. On the other hand, pSTS hyperactivation may be, at least in part, explained by change of focus of attention. It can be speculated that the stronger pSTS activity in children is driven by a focus of attention on changeable aspects of the face. Overall, the observed trend for stronger pSTS activation is an interesting finding which deserves further investigation.
Summary and Outlook
Taken together, the results of the present pilot study showed that it is possible to localize the core system in children at the single subject level. They further showed, at a trend level, a developmental shift from bilateral FFA activity in children to a right-hemispheric lateralization in adults. The estimated effect size for the FFA lateralization difference was d = 0.78 (indicating medium to large effects). Since this examination was designed as a pilot study, we only tested small samples of children and adults which represents a limitation for the data interpretation. An adequately powered follow-up study (sensitivity 0.8) testing developmental changes of FFA lateralization is planned and would therefore require the inclusion of 18 children and 26 adults. In the planned study we also aim to expand the test battery. By combining the face processing paradigm with a language task, we will also be able to assess the hypothesis that due to competition between language-biased left-hemispheric VWFA specialization and face representation in the left hemisphere, face representation that was initially bilateral is driven to become right-specialized in the course of development (Behrmann and Plaut, 2015).
Data Availability Statement
The datasets generated for this study are available on request to the corresponding author.
Ethics Statement
The studies involving human participants were reviewed and approved by Local ethics committee of the Department of Psychology of the Justus-Liebig University in Giessen, Germany. Written informed consent to participate in this study was provided by the participants’ legal guardian/next of kin.
Author Contributions
FH and ID: concept of the experiment, data collection, MR image processing, data analysis, interpretation of the data, and writing and revision of the manuscript. RK: data analysis, provision of the software motionEstimator, interpretation of the data, and revision of the manuscript. IT: data analysis, interpretation of the data, and revision of the manuscript. KZ: design of the fMRI protocol, data analysis, interpretation of the data, and revision of the manuscript. OS: data analysis and revision of the manuscript. JS: design of the fMRI protocol, data analysis, and revision of the manuscript. IK-B: financially enabled the study and revision of the manuscript. RS: financially enabled the study and revision of the manuscript. AJ: group leader, financially enabled the study, concept of the experiment, data analysis, interpretation of the data, and drafting and revision of the manuscript. All authors contributed to the article and approved the submitted version.
Funding
This work was supported by a Research Grant of the University Medical Center Giessen and Marburg (UKGM) to AJ, IK-B, and RS (project no. 7/2019-MR).
Conflict of Interest
The authors declare that the research was conducted in the absence of any commercial or financial relationships that could be construed as a potential conflict of interest.
Acknowledgments
This manuscript has been released as a pre-print at bioRxiv (https://www.biorxiv.org/content/10.1101/818310v1) (Hildesheim et al., 2019). We are very grateful for the help of Mechthild Wallnig with the MRI data collection.
Footnotes
- ^ https://github.com/kesslerr/motionEstimator
- ^ http://Openneuro.org
- ^ https://mriqc.readthedocs.io/en/stable/
- ^ Using this approach, it was of course only possible to calculate brain activity for those subjects in which we were able to find brain activity that was clearly attributable to a core system’s area. We will discuss in the results section the consequences both for the assessment of group differences and the estimation of unbiased effect size measures.
- ^ www.r-project.org/
- ^ It is to some degree arbitrary which cut-off value is used to consider an effect as bilateral. In the literature, the threshold is usually set to 0.2 (Springer et al., 1999; Deblaere et al., 2004; Jansen et al., 2006), but values like 0.1 (Yuan et al., 2006), 0.15 (Baciu et al., 2005), 0.25 (Pujol et al., 1999; Baciu et al., 2005), and even 0.3 (Benbadis et al., 1998) have also been used in previous work (Seghier, 2008). We chose as cut-off 0.2 since it is the most commonly applied criterion for bilateralism. This choice does not influence the results of our analyses since they consider hemispheric lateralization as continuous variable. The cut-off value is thus mainly used for descriptive purposes.
- ^ We post hoc analyzed the data of the two excluded children. One child (C03) moved so much that the measured volume was shifted outside the brain regions we were interested in, making it impossible to assess activity in the core system of face processing. The other child (C10) did not show any activity in the core system, also at liberal thresholds (p = 0.05 uncorrected).
- ^ At this point, we would like to add some further methodological remarks on the comparison of brain activity measures between children and adults. One important aspect is that children typically have smaller brains than adults. The FFA of children, as assessed by its absolute anatomical volume, is therefore most likely also smaller than the FFA of adults. This can have consequences for the comparison of brain activity measures between both groups. When one is interested in the comparison of the activation strength (rather than the extent of brain activity) between children and adults, one typical approach, the calculation of the mean beta value in a sphere with a given radius, can introduce a bias for instance when the sphere captures only the strongest activated voxels in adults, but–due to the smaller volume of the FFA–also non-activated voxels in children. This approach will most certainly introduce a bias in particular when using non-normalized images.
In the present study, we compared children and adults with regard to the activation strength and, as main aim, the hemispheric lateralization. Hemispheric lateralization was assessed on the basis of the number of activated voxel in spherical ROIs. We used individually tailored spheres that were sufficiently large to capture the activated cluster, but also sufficiently small to ensure that there is no overlap with other activated clusters. The lateralization index is calculated as comparison of left- and right-hemispheric activity. This procedure thus avoids the typical biases when comparing brain activity of children and adults. Brain activity between children and adults was assessed by the mean beta value in spherical ROIs with a radius of 6 mm. We avoided potential biases by assessing brain activity in normalized images and by using individually tailored masks that were centered for each subject at the corresponding local maximum of each cluster. It can, however, not be fully excluded that even after normalization specific brain regions are still smaller in children than in adults due to the children’s overall smaller brain volume. This might lead to an underestimation of the activation strength of children in comparison to adults. This is, however, of lower overall interest for the present study since it is focused on the development of hemispheric lateralization. If we had been interested mainly in the activation strength, one might have introduced further correction procedures (Golarai et al., 2007; Wilke et al., 2017).
References
Anderson, S. F., Kelley, K., and Maxwell, S. E. (2017). Sample-size planning for more accurate statistical power: a method adjusting sample effect sizes for publication bias and uncertainty. Psychol. Sci. 28, 1547–1562. doi: 10.1177/0956797617723724
Aylward, E. H., Park, J. E., Field, K. M., Parsons, A. C., Richards, T. L., Cramer, S. C., et al. (2005). Brain activation during face perception: evidence of a developmental change. J. Cogn. Neurosci. 17, 308–319. doi: 10.1162/0898929053124884
Baciu, M. V., Watson, J. M., Maccotta, L., McDermott, K. B., Buckner, R. L., and Gilliam, F. G. (2005). Evaluating functional MRI procedures for assessing hemispheric language dominance in neurosurgical patients. Neuroradiology 47, 835–844. doi: 10.1007/s00234-005-1431-3
Baron-Cohen, S., and Wheelwright, S. (2004). The empathy quotient: an investigation of adults with asperger syndrome or high functioning autism, and normal sex differences. J. Autism. Dev. Disord. 34, 163–175. doi: 10.1023/b:jadd.0000022607.19833.00
Baron-Cohen, S., Wheelwright, S., Skinner, R., Martin, J., and Clubley, E. (2001). The autism-spectrum quotient (AQ): evidence from asperger syndrome/high-functioning autism, males and females, scientists and mathematicians. J. Autism. Dev. Disord. 31, 5–17. doi: 10.1023/A:1005653411471
Bayer Health Care (Hg) (2008). Paula in der. (Röhre). Ein Malbuch für Kinder zur MRT Untersuchung. Berlin: Detailseite zuletzt geändert am.
Behrmann, M., and Plaut, D. C. (2015). A vision of graded hemispheric specialization. Ann. N. Y. Acad. Sci. 1359, 30–46. doi: 10.1111/nyas.12833
Benbadis, S. R., Binder, J. R., Swanson, S. J., Fischer, M., Hammeke, T. A., and Morris, G. L. (1998). Is speech arrest during Wada testing a valid method for determining hemispheric representation of language? Brain Lang. 65, 441–446. doi: 10.1006/brln.1998.2018
Bernstein, M., and Yovel, G. (2015). Two neural pathways of face processing: a critical evaluation of current models. Neurosci. Biobehav. Rev. 55, 536–546. doi: 10.1016/j.neubiorev.2015.06.010
Bukowski, H., Dricot, L., Hanseeuw, B., and Rossion, B. (2013). Cerebral lateralization of face-sensitive areas in left-handers: only the FFA does not get it right. Cortex 49, 2583–2589. doi: 10.1016/j.cortex.2013.05.002
Cantlon, J. F., Pinel, P., Dehaene, S., and Pelphrey, K. A. (2011). Cortical representations of symbols, objects, and faces are pruned back during early childhood. Cereb. Cortex 21, 191–199. doi: 10.1093/cercor/bhq078
Carlos, B. J., Hirshorn, E. A., Durisko, C., Fiez, J. A., and Coutanche, M. N. (2019). Word inversion sensitivity as a marker of visual word form area lateralization: an application of a novel multivariate measure of laterality. NeuroImage 191, 493–502. doi: 10.1016/j.neuroimage.2019.02.044
Cohen, M. A., Dilks, D. D., Koldewyn, K., Weigelt, S., Feather, J., Kell, A. J. E., et al. (2019). Representational similarity precedes category selectivity in the developing ventral visual pathway. Neuroimage 197, 565–574. doi: 10.1016/j.neuroimage.2019.05.010
Cohen Kadosh, K., Cohen Kadosh, R., Dick, F., and Johnson, M. H. (2011). Developmental changes in effective connectivity in the emerging core face network. Cereb. Cortex 21, 1389–1394. doi: 10.1093/cercor/bhq215
Deblaere, K., Boon, P. A., Vandemaele, P., Tieleman, A., Vonck, K., and Vingerhoets, G. (2004). MRI language dominance assessment in epilepsy patients at 1.0 T: region of interest analysis and comparison with intracarotid amytal testing. Neuroradiology 46, 413–420. doi: 10.1007/s00234-004-1196-0
Dehaene, S., and Cohen, L. (2011). The unique role of the visual word form area in reading. Trends Cogn. Sci. 15, 254–262. doi: 10.1016/j.tics.2011.04.003
Dien, J. (2009). A tale of two recognition systems: implications of the fusiform face area and the visual word form area for lateralized object recognition models. Neuropsychologia 47, 1–16. doi: 10.1016/j.neuropsychologia.2008.08.024
Dobs, K., Isik, L., Pantazis, D., and Kanwisher, N. (2019). How face perception unfolds over time. Nat. Commun. 10:1258. doi: 10.1038/s41467-019-09239-1
Dundas, E. M., Plaut, D. C., and Behrmann, M. (2013). The joint development of hemispheric lateralization for words and faces. J. Exp. Psychol. Gen. 142, 348–358. doi: 10.1037/a0029503
Esteban, O., Birman, D., Schaer, M., Koyejo, O. O., Poldrack, R. A., and Gorgolewski, K. J. (2017). MRIQC: predicting quality in manual MRI assessment protocols using no-reference image quality measures. PLoS One 12:e0184661. doi: 10.1371/journal.pone.0184661
Fairhall, S. L., and Ishai, A. (2007). Effective connectivity within the distributed cortical network for face perception. Cereb. Cortex 17, 2400–2406. doi: 10.1093/cercor/bhl148
Faul, F., Erdfelder, E., Buchner, A., and Lang, A. G. (2009). Statistical power analyses using G∗Power 3.1: tests for correlation and regression analyses. Behav. Res. Methods 41, 1149–1160. doi: 10.3758/brm.41.4.1149
Fox, C. J., Iaria, G., and Barton, J. J. (2009). Defining the face processing network: optimization of the functional localizer in fMRI. Hum. Brain Mapp. 30, 1637–1651. doi: 10.1002/hbm.20630
Frässle, S., Krach, S., Paulus, F. M., and Jansen, A. (2016a). Handedness is related to neural mechanisms underlying hemispheric lateralization of face processing. Sci. Rep. 6, 1–17. doi: 10.1038/srep27153
Frässle, S., Paulus, F. M., Krach, S., and Jansen, A. (2016b). Test-retest reliability of effective connectivity in the face perception network. Hum. Brain Mapp. 37, 730–744. doi: 10.1002/hbm.23061
Frässle, S., Paulus, F. M., Krach, S., Schweinberger, S. R., Stephan, K. E., and Jansen, A. (2016c). Mechanisms of hemispheric lateralization: asymmetric interhemispheric recruitment in the face perception network. NeuroImage 124, 977–988. doi: 10.1016/j.neuroimage.2015.09.055
Gathers, A. D., Bhatt, R., Corbly, C. R., Farley, A. B., and Joseph, J. E. (2004). Developmental shifts in cortical loci for face and object recognition. Neuroreport 15, 1549–1553. doi: 10.1097/01.wnr.0000133299.84901.86
Gerrits, R., Van der Haegen, L., Brysbaert, M., and Vingerhoets, G. (2019). Laterality for recognizing written words and faces in the fusiform gyrus covaries with language dominance. Cortex 117, 196–204. doi: 10.1016/j.cortex.2019.03.010
Gobbini, M. I., and Haxby, J. V. (2007). Neural systems for recognition of familiar faces. Neuropsychologia 45, 32–41. doi: 10.1016/j.neuropsychologia.2006.04.015
Gogtay, N., Giedd, J. N., Lusk, L., Hayashi, K. M., Greenstein, D., Vaituzis, A. C., et al. (2004). Dynamic mapping of human cortical development during childhood through early adulthood. PNAS 101, 8174–8179. doi: 10.1073/pnas.0402680101
Golarai, G., Ghahremani, D. G., Whitfield-Gabrieli, S., Reiss, A., Eberhardt, J. L., Gabrieli, J. D., et al. (2007). Differential development of high-level visual cortex correlates with category-specific recognition memory. NatureNeuroscience 10, 512–522. doi: 10.1038/nn1865
Gschwind, M., Pourtois, G., Schwartz, S., Ville, D., and van de Vuilleumier, P. (2012). White-matter connectivity between face-responsive regions in the human brain. Cereb. Cortex 22, 1564–1576. doi: 10.1093/cercor/bhr226
Haist, F., Adamo, M., Wazny, J. H., Lee, K., and Stiles, J. (2013). The functional architecture for face processing expertise: FMRI evidence of the developmental trajectory of the core and the extended face systems. Neuropsychologia 51, 2893–2908. doi: 10.1016/j.neuropsychologia.2013.08.005
Haxby, J. V., Hoffman, E. A., and Gobbini, M. I. (2000). The distributed human neural system for face perception. Trends Cogn. Sci. 4, 223–233. doi: 10.1016/s1364-6613(00)01482-0
Hemond, C. C., Kanwisher, N. G., and Op de Beeck, H. P. (2007). A preference for contralateral stimuli in human object- and face-selective cortex. PLoS One 2:e574. doi: 10.1371/journal.pone.0000574
Hildesheim, F. E., Debus, I., Kessler, R., Thome, I., Zimmermann, K. M., Steinsträter, O., et al. (2019). The ontogenetic development of hemispheric lateralization during face processing: a functional magnetic resonance imaging pilot study in 7- to 9-year-old children. bioRxiv [Preprint]. doi: 10.1101/818310
Hillger, L. A., and Koenig, O. (1991). Separable mechanisms in face processing: evidence from hemispheric specialization. J. Cogn. Neurosci. 3, 42–58. doi: 10.1162/jocn.1991.3.1.42
Hoffman, E. A., and Haxby, J. V. (2000). Distinct representations of eye gaze and identity in the distributed human neural system for face perception. NatureNeuroscience 3, 80–84. doi: 10.1038/71152
Ishai, A. (2008). Let’s face it: it’s a cortical network. NeuroImage 40, 415–419. doi: 10.1016/j.neuroimage.2007.10.040
Ishai, A., Schmidt, C. F., and Boesiger, P. (2005). Face perception is mediated by a distributed cortical network. Brain Res. Bull. 67, 87–93. doi: 10.1016/j.brainresbull.2005.05.027
Jansen, A., Menke, R., Sommer, J., Förster, A. F., Bruchmann, S., Hempleman, J., et al. (2006). The assessment of hemispheric lateralization in functional MRI – Robustness and reproducibility. NeuroImage 33, 204–217. doi: 10.1016/j.neuroimage.2006.06.019
Joseph, J. E., Gathers, A. D., and Bhatt, R. S. (2011). Progressive and regressive developmental changes in neural substrates for face processing: testing specific predictions of the interactive specialization account. Dev. Sci. 14, 227–241. doi: 10.1111/j.1467-7687.2010.00963.x
Lundqvist, D., Flykt, A., and Öhman, A. (1998). The Karolinska Directed Emotional Faces – KDEF, CD ROM from Department of Clinical Neuroscience, Psychology section. Solna: Karolinska Institutet. doi: 10.1037/t27732-000
Maldjian, J. A., Laurienti, P. J., Kraft, R. A., and Burdette, J. H. (2003). An automated method for neuroanatomic and cytoarchitectonic atlas-based interrogation of fMRI data sets. NeuroImage 19, 1233–1239. doi: 10.1016/s1053-8119(03)00169-1
Meng, M., Cherian, T., Singal, G., and Sinha, P. (2012). Lateralization of face processing in the human brain. Proc. R. Soc. 279, 2052–2061. doi: 10.1098/rspb.2011.1784
Morawetz, C., Holz, P., Lange, C., Baudewig, J., Weniger, G., Irle, E., et al. (2008). Improved functional mapping of the human amygdala using a standard functional magnetic resonance imaging sequence with simple modifications. Magn. Reson. Imaging 26, 45–53. doi: 10.1016/j.mri.2007.04.014
Oldfield, R. C. (1971). The assessment and analysis of handedness: the edinburgh inventory. Neuropsychologia 9, 97–113. doi: 10.1016/0028-3932(71)90067-4
Passarotti, A. M., Paul, B. M., Bussiere, J. R., Buxton, R. B., Wong, E. C., and Stiles, J. (2003). The development of face and location processing: an fMRI study. Dev. Sci. 6, 100–117. doi: 10.1111/1467-7687.00259
Peelen, M. V., Glaser, B., Vuilleumier, P., and Eliez, S. (2009). Differential development of selectivity for faces and bodies in the fusiform gyrus. Dev. Sci. 12, F16–F25. doi: 10.1111/j.1467-7687.2009.00916.x
Power, J. D., Schlaggar, B. L., and Petersen, S. E. (2015). Recent progress and outstanding issues in motion correction in resting state fMRI. NeuroImage 0, 536–551. doi: 10.1016/j.neuroimage.2014.10.044
Price, C. J., and Devlin, J. T. (2003). The myth of the visual word form area. NeuroImage 19, 473–481. doi: 10.1016/s1053-8119(03)00084-3
Pujol, J., Deus, J., Losilla, J. M., and Capdevila, A. (1999). Cerebral lateralization of language in normal left-handed people studied by functional MRI. Neurology 52, 1038–1043. doi: 10.1212/wnl.52.5.1038
Rhodes, G., Brake, S., and Atkinson, A. P. (1993). What’s lost in inverted faces? Cognition 47, 25–57. doi: 10.1016/0010-0277(93)90061-y
Rhodes, G., Michie, P. T., Hughes, M. E., and Byatt, G. (2009). The fusiform face area and occipital face area show sensitivity to spatial relations in faces. Eur. J. Neurosci. 30, 721–733. doi: 10.1111/j.1460-9568.2009.06861.x
Rossion, B. (2015). “Face perception,” in Brain Mapping: An Encyclopedic Reference, 2nd Edn, ed. A. W. Toga (Amsterdam: Elsevier), 515–522. doi: 10.1016/B978-0-12-397025-1.00037-3
Saygin, Z. M., Osher, D. E., Norton, E. S., Youssoufian, D. A., Beach, S. D., Feather, J., et al. (2016). Connectivity precedes function in the development of the visual word form area. Nat. Neurosci. 19, 1250–1255. doi: 10.1038/nn.4354
Scherf, K. S., Behrmann, M., Humphreys, K., and Luna, B. (2007). Visual category-selectivity for faces, places and objects emerges along different developmental trajectories. Dev. Sci. 10, F15–F30. doi: 10.1111/j.1467-7687.2007.00595.x
Schultz, J., and Pilz, K. S. (2009). Natural facial motion enhances cortical responses to faces. Exp. Brain Res. 194, 465–475. doi: 10.1007/s00221-009-1721-9
Schuster, V., Herholz, P., Zimmermann, K. M., Westermann, S., Frässle, S., and Jansen, A. (2017). Comparison of fMRI paradigms assessing visuospatial processing: robustness and reproducibility. PLoS One 12:e0186344. doi: 10.1371/journal.pone.0186344
Schwarzer, G. (2000). Development of face processing: the effect of face inversion. Child Dev. 71, 391–401. doi: 10.1111/1467-8624.00152
Seghier, M. L. (2008). Laterality index in functional MRI: methodological issues. Magn. Reson. Imaging 26, 594–601. doi: 10.1016/j.mri.2007.10.010
Springer, J. A., Binder, J. R., Hammeke, T. A., Swanson, S. J., Frost, J. A., Bellgowan, P. S. F., et al. (1999). Language dominance in neurologically normal and epilepsy subjects. A functional MRI study. Brain 122, 2033–2045. doi: 10.1093/brain/122.11.2033
Wechsler, D., and Naglieri, J. A. (2006). Wechsler Nonverbal Scale of Ability. San Antonio, TX: Harcourt Assessment. doi: 10.1037/t15176-000
Weigelt, S., Koldewyn, K., Dilk, D. D., Balas, B., McKone, E., and Kanwisher, N. (2014). Domain-specific development of face memory but not face perception. Dev. Sci. 17, 47–58. doi: 10.1111/desc.12089
Wilke, M., Altaye, M., and Holland, S. K. (2017). CerebroMatic: a versatile toolbox for spline-based MRI template creation. Front. Comput. Neurosci. 11:5. doi: 10.3389/fncom.2017.00005
Wilke, M., Groeschel, S., Lorenzen, A., Rona, S., and Schuhmann, M. U. (2018). Clinical application of advanced MR methods in children: points to consider. Ann. Clin. Transl. Neurol. 5, 1434–1455. doi: 10.1002/acn3.658
Wilke, M., and Lidzba, K. (2007). LI-tool: a new toolbox to assess lateralization in functional MR-data. J. Neurosci. Methods 163, 128–136. doi: 10.1016/j.jneumeth.2007.01.026
Wilke, M., and Schmithorst, V. J. (2006). A combined bootstrap / histogram analysis approach for computing a lateralization index from neuroimaging data. NeuroImage 33, 522–530. doi: 10.1016/j.neuroimage.2006.07.010
Willems, R. M., Peelen, M. V., and Hagoort, P. (2010). Cerebral lateralization of face-selective and body-selective visual areas depends on handedness. Cereb. Cortex 20, 1719–1725. doi: 10.1093/cercor/bhp234
Willenbockel, V., Sadr, J., Fiset, D., Horne, G. O., Gosselin, F., and Tanaka, J. W. (2010). Controlling low-level image properties: the SHINE toolbox. Behav. Res. Methods 42, 671–684. doi: 10.3758/brm.42.3.671
Keywords: face processing, magnetic resonance imaging, lateralization, children, visual word form area, fusiform face area, occipital face area, posterior superior temporal sulcus
Citation: Hildesheim FE, Debus I, Kessler R, Thome I, Zimmermann KM, Steinsträter O, Sommer J, Kamp-Becker I, Stark R and Jansen A (2020) The Trajectory of Hemispheric Lateralization in the Core System of Face Processing: A Cross-Sectional Functional Magnetic Resonance Imaging Pilot Study. Front. Psychol. 11:507199. doi: 10.3389/fpsyg.2020.507199
Received: 25 February 2020; Accepted: 10 September 2020;
Published: 02 October 2020.
Edited by:
Sara Palermo, University of Turin, ItalyReviewed by:
Vadim Axelrod, Bar-Ilan University, IsraelRosalba Morese, University of Italian Switzerland, Switzerland
Vincenza Tarantino, University of Palermo, Italy
Copyright © 2020 Hildesheim, Debus, Kessler, Thome, Zimmermann, Steinsträter, Sommer, Kamp-Becker, Stark and Jansen. This is an open-access article distributed under the terms of the Creative Commons Attribution License (CC BY). The use, distribution or reproduction in other forums is permitted, provided the original author(s) and the copyright owner(s) are credited and that the original publication in this journal is cited, in accordance with accepted academic practice. No use, distribution or reproduction is permitted which does not comply with these terms.
*Correspondence: Andreas Jansen, YW5kcmVhcy5qYW5zZW5Ac3RhZmYudW5pLW1hcmJ1cmcuZGU=
†These authors have contributed equally to this work