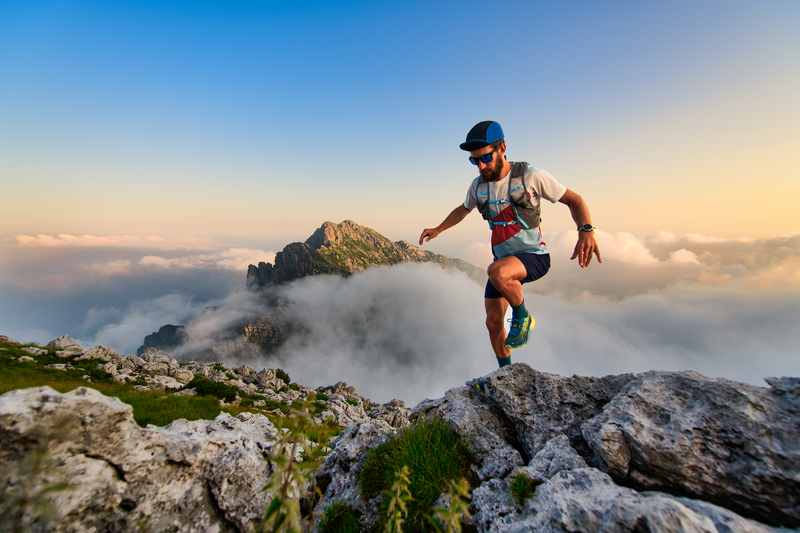
95% of researchers rate our articles as excellent or good
Learn more about the work of our research integrity team to safeguard the quality of each article we publish.
Find out more
FOCUSED REVIEW article
Front. Psychol. , 30 May 2017
Volume 8 - 2017 | https://doi.org/10.3389/fpsyg.2017.00860
This article mentions parts of:
Forever Young(er): potential age-defying effects of long-term meditation on gray matter atrophy
Over the last decade, an increasing number of studies has reported a positive impact of meditation on cerebral aging. However, the underlying mechanisms for these seemingly brain-protecting effects are not well-understood. This may be due to the fact, at least partly, that systematic empirical meditation research has emerged only recently as a field of scientific scrutiny. Thus, on the one hand, critical questions remain largely unanswered; and on the other hand, outcomes of existing research require better integration to build a more comprehensive and holistic picture. In this article, we first review theories and mechanisms pertaining to normal (brain) aging, specifically focusing on telomeres, inflammation, stress regulation, and macroscopic brain anatomy. Then, we summarize existing research integrating the developing evidence suggesting that meditation exerts positive effects on (brain) aging, while carefully discussing possible mechanisms through which these effects may be mediated.
Life expectancy has been rising consistently for more than a century, and it is expected that this trend will continue (Oeppen and Vaupel, 2002). In the United States, the percentage of the population aged 65 and older is projected to grow from 13.7 to 20.9% between 2012 and 2050. Furthermore, over the same time span, the percentage of the population aged 85 and older is likely to increase from 1.9 to 4.5% (Ortman et al., 2014). On a broader geographical scale, the percentage of the population aged 60 and older is estimated to double worldwide by 2050 (WHO, 2015). While this is generally good news, old age is also a major risk factor for dementia and other neurodegenerative diseases, and it is therefore expected that the prevalence of age-related pathological conditions will rise in upcoming years. For example, over 35 million people were suffering from dementia worldwide in 2010, and this number is expected to triple by 2050 (WHO, 2012). In other words, a longer life expectancy might come at the expense of a healthy life. Thus, in the current absence of a general cure for age-related pathological conditions or disease-modifying treatments for dementia, it is important to direct more attention to the development of effective preventative approaches to brain aging and neurodegeneration.
Recent research revealed a reduced negative correlation between age and brain gray matter in long-term meditation practitioners compared to age-matched controls (Luders et al., 2015). We interpreted these findings as possibly indicating that meditators' brains are less affected by aging processes Importantly, this is not an isolated observation and, over the past decade, scientific evidence suggesting that meditation may help us to hold on longer to young(er) brains, bodies, and minds has been accumulating (Epel et al., 2009; Gard et al., 2014; Koike and Cardoso, 2014; Luders, 2014; Marciniak et al., 2014; Luders and Cherbuin, 2016). Thus, in light of a steadily increasing life expectancy, meditation could be an effective means to better maintain brain tissue, preserve cognitive, and emotional reserves, and to diminish the risk of dementia and other age-related neurodegenerative diseases. However, before implementing prevention and/or intervention strategies, systematic, rigorous studies are clearly necessary to fill existing research gaps. Moreover, findings already present seem to require a better integration to construct a solid basis on which to further develop this field. Thus, in the first part of this article (Section Effects and Mechanisms of Aging), we review and discuss relevant theories and biological mechanisms implicated in aging and likely to be modulated by meditative practice. In the second part (Section Effects and Possible Mechanisms of Meditation), we summarize and attempt to integrate the increasing evidence suggesting that meditation exerts positive effects on (brain) aging; possible mechanisms through which these effects may be mediated are discussed. Note that, throughout the text, the term “meditation” is used to refer to any type of mind-body practice (for details on different types of meditation as well as mindfulness practices, please refer to Lutz et al., 2008; Lippelt et al., 2014; Marciniak et al., 2014).
Aging is the result of a multitude of complex direct and indirect mechanisms. While many of these mechanisms are still not fully understood, others have already been well-characterized (for review, see Lopez-Otin et al., 2013). Given the extensive literature on this topic, a comprehensive summary of the mechanisms and effects of normal brain aging is beyond the scope of this review. Instead, here we focus on selected aging-related processes for which effects of meditation have been reported, such as related to telomeres, inflammation, stress regulation, and macroscopic brain anatomy.
In vitro, diploid cells can only replicate a finite number of times before cellular senescence and programmed cell death occur (Hayflick and Moorhead, 1961). This limited cell life-cycle is due to the progressive shortening of telomeres—the terminal sections of the chromosomal deoxyribonucleic acid (DNA) strands in eukaryotic cells that cannot be replicated completely during mitosis (Blackburn et al., 2006, 2015). In addition to this programmed mechanism, it has been established that superoxide and other free radicals, such as reactive oxygen species (ROS), damage cellular structures, including DNA (Lopez-Otin et al., 2013). ROS are constantly produced by normal cellular metabolic processes or immune responses, and may also result from exogenous sources (e.g., radiation, chemicals, etc.). Within the cell, ROS result in oxidation and subsequent alterations of membranes, organelles, proteins, and the DNA, potentially impairing physiological functioning overall. Particularly in the DNA, such oxidations can result in devastating alterations of function. While most of the DNA is consistently restored, telomeres are covered by shelterin proteins that limit repair. Thus, it has been proposed that telomeres encode the cumulative DNA damage and with it the probability of devastating mutations (Blackburn et al., 2015). Importantly, cumulative damage of the telomeres has the same effects as progressive shortening and leads to altered gene transcription, altered cell functioning as well as mitochondrial dysfunction, and ultimately programmed cell death.
Interestingly, telomere attrition also increases ROS production via altered signaling and consequent mitochondrial dysfunction, which results in an increased release of ROS into the cell (Sahin et al., 2011). While an increase of ROS will initially result in increased compensatory and repair mechanisms, those mechanisms will fail above a certain threshold of ROS (Hekimi et al., 2011; Lopez-Otin et al., 2013). Thus, shortened or damaged telomeres can actively induce an environment that will lead to further telomere attrition, cellular senescence, cell cycle arrest, and ultimately apoptosis. While telomere attrition may play a protective role in avoiding mutations and DNA damage, telomeres can nevertheless be repaired and elongated by a specific enzyme, telomerase, which is enriched in a variety of stem cells (Blackburn et al., 2015). Although telomerase can help repair accumulated DNA damage and re-extend shortened telomeres, this enzyme is low (sometimes even undetectable) in many human cells, consequently resulting in unremitting telomere attrition (Blackburn et al., 2006, 2015; Lopez-Otin et al., 2013).
KEY CONCEPT 1. Telomere attrition
Telomeres are known to shorten with every cell division. Further, DNA damage in this region is not readily repaired, but accumulates over time. This attrition of the telomeres probably encodes the potential accumulated risk for mutations and dysfunction over the lifetime of a cell. Increasing telomere attrition results in increased repair mechanisms, and finally cell death.
Aging does not only affect intra-cellular biochemical processes, but also presents with changes in cell signaling, including endocrine, neuroendocrine, and neuronal communication (Lopez-Otin et al., 2013). One of these changes manifests as a weakened immune response as well as increased inflammatory signaling and has been described as central to aging. Age-associated inflammation was reported to inhibit epidermal stem cell function (Doles et al., 2012) and also has been discussed as an underlying factor of diseases, such as type 2 diabetes, atherosclerosis, and obesity (Lopez-Otin et al., 2013). In other words, while inflammation is a central factor of the immune response and essential for tissue regeneration and healing, this complex response becomes less specific and less effective with age. The increased inflammatory signaling that accompanies aging in mammals has been coined “inflammaging” (Salminen et al., 2012a) and represents a smoldering pro-inflammatory phenotype. Several reports point to the pro-inflammatory transcription factor NF-κB (Nuclear Factor κB) as a central element of inflammaging (Helenius et al., 1996; Adler et al., 2007; Salminen et al., 2008; Haustead et al., 2016). Moreover, inflammaging can be fueled by increased ROS and pro-inflammatory pathways. However, cause and effect on the cellular level are deeply intertwined (Salminen et al., 2012a,b; Lopez-Otin et al., 2013) and point to a complex relationship between cellular senescence, telomeres, and inflammation: As described in Section Telomeres, increased ROS—resulting, for example, from inflammatory processes, mitochondrial dysfunction, or indirectly from telomere attrition itself—may lead to increased oxidative stress and associated activation of the pro-inflammatory NF-κB pathway. Telomere attrition itself leads to the release of shelterin proteins, among them RAP1 (Ras-proximate-1), that normally cover the telomeres. Set free, RAP1 activates the NF-κB pathway, thus further increasing a pro-inflammatory state (Ye et al., 2014).
KEY CONCEPT 2. Inflammation
A more pro-inflammatory state along with a less focused (i.e., weaker and less localized) response may be observed in aging, coined “inflammaging.” This state weakens the immune response and wound healing on the one hand, and increases stress on the tissue overall on the other.
Stress enables individuals to effectively respond to threatening situations arising both from their internal environment (e.g., hypotension, pain) or external environment (e.g., cold, predators), and to maintain homeostasis. One mechanism involved in the so-called “stress response” is the hypothalamus-pituitary-adrenal (HPA) axis. The HPA axis describes an endocrine signaling cascade that starts with the release of corticotrophin releasing hormone (CRH) in the hypothalamus, which causes the release of adrenocorticotropic hormone (ACTH) in the pituitary gland, that then triggers the release of glucocorticoids (cortisol in humans) from the adrenal glands. The activation of the HPA axis leads to wide ranging-effects, including raised blood pressure and raised heart rate, increased metabolism, and increased psychological alertness, as well as decreased inflammatory and decreased immune responses. While such modulations are essential to survival and well-being, chronic activation of the stress response leads to adverse outcomes. For example, high basal levels of glucocorticoids, if sustained over time, lead to increased ROS production, and increased risk of DNA and cellular damage (Garcia-Bueno et al., 2008; Joergensen et al., 2011). Moreover, high CRH levels stimulate the production of pro-inflammatory cytokines, thus further increasing oxidative stress (Wang et al., 2003). Importantly, increased stress levels and persistent negative emotions (e.g., as experienced during depression or life adversities), have been linked directly to accelerated biological aging through telomere shortening, DNA replication errors, and cellular damage (Lin et al., 2012; Price et al., 2013; Revesz et al., 2014; Blackburn et al., 2015; Zahran et al., 2015) or increased NF-κB activity (Irwin and Cole, 2011; Cole, 2014; Bower and Irwin, 2016). Furthermore, it has been shown that stress, anxiety, and depression are associated with reduced neuroplasticity and neurogenesis (Mcewen, 2001; Hanson et al., 2011; Liston and Gan, 2011; Besnard and Sahay, 2016), limiting the brain's ability to effectively adapt to new challenges. Finally, increased cortisol levels have been reported to be particularly detrimental in some brain regions (Bentson et al., 1978; Starkman et al., 1992, 1999; Knoops et al., 2010). For example, increased cortisol levels have been found to reduce the volumes of the hippocampus in rodents (Watanabe et al., 1992), primates (Uno et al., 1989), as well as humans (Bentson et al., 1978; Starkman et al., 1992, 1999; Knoops et al., 2010), suggesting a close link between stress, cortisol levels, and loss of brain tissue. Thus, while the stress response is protective in relation to transient threats, its chronic upregulation is detrimental and leads to accelerated (brain) aging.
KEY CONCEPT 3. Glucocorticoids
Glucocorticoid receptors are located inside the cells and, when activated, become transcription factors that change its functioning. On short term, glucocorticoids lead to multiple adaptations throughout the organism that are extremely beneficial during a stress response. However, when increased over a long time span, these changes can have detrimental effects.
A well-documented effect of aging is a progressive decline in brain volume commencing in our early twenties to mid-thirties. While it is not entirely clear how this volume loss is reflected at the microscopic level, significant contributors to tissue loss include decreases in neuron, glia, and spine numbers, reductions in interstitial space (neuropil), shrinkages of dendritic trees, and demyelination (Shen et al., 2008; Glorioso and Sibille, 2011). Importantly, age-related volume changes differ between tissue types and brain regions with different brain structures showing different trajectories. For total brain volume this decline is estimated to range from 0.2% per year in early to mid-adulthood to 0.6% per year in the sixties and seventies (Hedman et al., 2012; Tabatabaei-Jafari et al., 2015). In contrast, the hippocampus, reaches its maximum volume in the mid-twenties and then starts declining at a rate of 0.38% per year until the mid-fifties. The shrinkage rate then increases to 0.98% per year and peaks at 1.12% in the early seventies (Fraser et al., 2015). As for white matter, the age-related volume decrease has been reported to begin later than for gray matter, and maturation processes (e.g., fiber myelination) continue well into the thirties and possibly beyond (Westlye et al., 2010). Despite this continuous decline in brain tissue over time, the brain retains some ability to change and adapt to new demands across the lifespan (Greenwood and Parasuraman, 2010; Valkanova et al., 2014). Indeed, increases in local brain volume have been described in regions involved in the acquisition and performance of new tasks over time frames from several weeks to years, and importantly such positive changes were evident in young adults as well as in the elderly (Draganski et al., 2004, 2006; Boyke et al., 2008; Woollett and Maguire, 2011). This neuroplasticity may allow, at least to some extent, to compensate for age-related volume loss by consolidating and optimizing resources (for review see Greenwood and Parasuraman, 2010). Similarly, factors such as educational attainment have been proposed to stimulate neuroplasticity and neurotrophic mechanisms, thereby enhancing brain and cognitive reserves, which may ultimately manifest as more age-resilient brains (Stern, 2012).
KEY CONCEPT 4. Decline in brain volume
Probably one of the best established effects of aging in brain research is a progressive loss of brain volume. While volume loss may be compensated to some degree via neuroplastic adaptation, it may reach a threshold below which full compensation becomes impossible. A relative preservation of volume might therefore be protective or delay the onset of functional decline.
KEY CONCEPT 5. Neuroplasticity
The brain has the ability to adapt in response to the existence (or absence) of demand. Neuroplasticity could be observed in local volume increases as a response to the learning of new tasks. Neuroplastic changes on a macroscopic level can be observed within weeks to years and can be assumed to be a continuous and fundamental process.
While there is only limited research investigating meditation and biological processes implicated in brain aging and neurodegeneration, it hints at plausible pathways through which meditative practice may exert beneficial effects on (brain) aging. In the next sections, we will review this emerging field of research suggesting an association between meditation and the aforementioned aspects and processes as pertaining to normal aging.
As described above (see Section Telomeres), the enzyme telomerase contributes to repairing accumulated DNA damage and extending shortened telomeres. Interestingly, recent studies have indicated that meditation may increase telomerase activity (Jacobs et al., 2011; Lavretsky et al., 2013; Schutte and Malouff, 2014) and telomere length (Hoge et al., 2013; Alda et al., 2016). While telomere length has only been measured cross-sectionally, telomerase activity has been investigated longitudinally. Altogether, these studies' outcomes are consistent with the notion that meditation is protective against cellular aging. However, some caution is warranted. Telomere length and telomerase activity are measured in leukocytes from blood draws, and therefore these biomarkers may only reflect conditions (and possible meditation-induced effects) in peripheral blood cells, rather than in neurons and more broadly brain tissue. In fact, the role of telomerase in cells of the central nervous system remains obscure. More specifically, even although there is some preliminary evidence that a subunit of telomerase is also expressed in neurons after brain injury, it remains unclear if telomerase is expressed under normal conditions and with what result (Mattson et al., 2002; Zhang et al., 2007; Gonzalez-Giraldo et al., 2016). It should also be noted that while the critical role of telomere attrition is well-established in cell aging, its specific effect on the brain is not entirely clear (Allsopp et al., 1995; Nakamura et al., 2007; Zhang et al., 2010; Eitan et al., 2014). Nevertheless, shorter leukocyte telomere length has been reported to predict dementia, cognitive decline, and mortality, suggesting at least some link between telomere length and brain aging (Eitan et al., 2014). Moreover, as telomeres are particularly sensitive to DNA damage (see Section Telomeres), the positive findings in meditators (i.e., longer telomeres, stronger telomerase activity) may point to less inflammatory signaling (see Section Inflammation), less stress (see Section Stress Regulation), and as a result more functionally intact cells, at least within the blood stream. Unfortunately, more comprehensive investigations exploring these links in controlled prospective intervention studies, optimally over an extended time frame, are entirely missing.
Recent longitudinal studies have revealed that meditation reduces NF-κB activity (Creswell et al., 2012; Bhasin et al., 2013; Black et al., 2013; Bower et al., 2015; Rao et al., 2015; Bower and Irwin, 2016) as well as circulating markers of inflammation, such as C reactive protein and interleukin (IL)-6 (Creswell et al., 2012, 2016; Lengacher et al., 2012; Malarkey et al., 2013). Moreover, the reduced NF-κB activity was accompanied by a meditation-induced increased activity of the anti-inflammatory glucocorticoid receptor, which suggests a higher sensitivity to anti-inflammatory glucocorticoids (Bower et al., 2015; Bower and Irwin, 2016). Overall, these results point to a reduced pro-inflammatory state in active meditation practitioners. However, as for telomerase activity and telomere length (as discussed in Section Effects of Meditation on Telomeres), markers of inflammation are measured in peripheral leukocytes, rather than in neurons of the central nervous system; and although the role of NF-κB in brain cells is better established than for telomeric measures, the interpretation of these findings remains tentative. That is, on the one hand, NF-κB within glia cells was found to exert a pro-inflammatory activity and the potential to cause neuronal degeneration. On the other hand, and outside the realm of inflammation, NF-κB has also been established as an important factor for neuronal development, plasticity, repair, and survival (Mattson, 2005; Mattson and Meffert, 2006; Camandola and Mattson, 2007; Boersma et al., 2011). Thus, a simple extrapolation from findings in peripheral leukocytes to all cells and circumstances may be too simplistic, especially as it is well-known that single factors can result in different effects depending on the circumstances. Overall, however, meditation-induced reduction in NF-κB activity might be beneficial, as inflammatory activity observed in peripheral immune cells (e.g., leucocytes) may be correlated (at least to some degree) with the activity of immune cells in the brain (e.g., microglia). Overall, these reductions of NF-κB activity may potentially reflect a delay of the smoldering pro-inflammatory phenotype “inflammaging” and its detrimental effects (see Section Inflammation), although this latter hypothesis remains mere conjecture until it can be tested directly. Along these same lines, a meditation-induced increase in the activity of the glucocorticoid receptor (Bower et al., 2015; Bower and Irwin, 2016) is likely to increase the anti-inflammatory properties of cortisol. This, in turn, may help reduce cortisol levels overall (because the higher glucocorticoid receptor sensitivity will relay the same anti-inflammatory effects at lower doses of circulating cortisol). As may be inferred from the discussion of the detrimental effects of cortisol (see Section Stress Regulation), decreased cortisol levels are likely to contribute to lower rates of brain shrinkage, and particularly so in the hippocampus. So, altogether, the observed effects of meditation on inflammation processes may have beneficial effects on brain aging. However, comprehensive controlled trials to substantiate these effects are still missing.
Several studies suggest that meditation fosters adaptive emotion regulation and emotional well-being (Moynihan et al., 2013; Grecucci et al., 2015; Zeng et al., 2015), while also reducing stress and cortisol levels (Chiesa and Serretti, 2009; Koike and Cardoso, 2014; Ray et al., 2014; Sharma and Rush, 2014; Turan et al., 2015). Such effects were not only observed cross-sectionally when comparing meditators with non-meditators but also longitudinally in randomized controlled trials exploring the effects of meditation. These findings may reflect improved voluntary regulation of mental activity, shifting focus and attention, as well as reduction in rumination (Holzel et al., 2011; Wolkin, 2015). As discussed above (see Section Stress Regulation), stress reduction may play a central part in the modulating effects of meditation on brain aging, especially as chronically increased cortisol levels have been associated with increased ROS, NF-κB activity, and brain volume loss, as well as reduced telomere length and neuroplastic capacity (see Section Inflammation and Stress Regulation). Thus, while stress is not a mechanism of aging per se, it can directly affect a multitude of aging processes. Unfortunately, a comprehensive assessment of the effects of meditation on perceived stress, objective biomarkers of stress (including cortisol levels etc.), changes in telomere length, NF-κB activity, and brain tissue over an extended time is not yet available. This severely limits the level of inference that can be drawn at this point. More specifically, while links and interactions between meditation, stress regulation, and mechanisms of aging appear likely, they have not been assessed directly and remain a hypothesis that awaits to be tested in future studies.
A limited number of cross-sectional neuroimaging studies has investigated the possible modulating impact of meditation on age-related changes in the macrostructure of the brain (Luders, 2014; Luders and Cherbuin, 2016). These investigations revealed less pronounced negative correlations between chronological age and various cerebral measures (e.g., local and global gray matter volumes or indicators of white matter fiber integrity) in meditators than in controls (Lazar et al., 2005; Pagnoni and Cekic, 2007; Luders et al., 2011, 2015; Kurth et al., 2015; Laneri et al., 2016). Moreover, when estimating the age of brains using automated pattern recognition, the brains of meditators appeared to be several years younger than the brains of age-matched controls (Luders et al., 2016). These findings seem to suggest that meditation may slow down age-related brain degeneration. However, the cross-sectional nature of these studies precludes an inference on causality. That is, it remains unclear whether meditation induced the observed differences, whether the differences existed already before people started meditating, whether there is interplay between the two, or whether the differences are due to more complex interactions with other factors. Assuming the observed differences are the result of meditation, a combination of processes is likely to be implicated (Kurth et al., 2015; Luders et al., 2015; Luders and Cherbuin, 2016): For example, through a variety of mechanisms, such as upregulating telomerase activity, down-regulating pro-inflammatory processes, and decreasing the effects of chronic stress (see Sections Effects of Meditation on Telomeres, Effects of Meditation on Inflammation, and Effects of Meditation on Stress Regulation), meditation may prevent or at least reduce the normal age-related brain decline by averting neurotoxic effects on the brain. However, these effects might even go beyond the mere prevention of tissue loss and actually present as tissue gain, at least in the short term. For example, it has been reported that loss of hippocampal tissue originally induced by high cortisol levels was restored when cortisol levels were reduced (Starkman et al., 1999). Similarly, relative tissue increases may occur due to anabolic training effects, similar to the neuroplasticity observed in imaging studies (see Section Macroscopic Brain Anatomy). In fact, several longitudinal studies revealed changes in local brain tissue after 4–8 weeks of intense meditation and, overall, these changes presented as increases (Fox et al., 2014). Nevertheless, some tissue decreases were also evident in brain regions known to modulate stress and fear responses and/or when effects of meditation were examined in pathological states (Holzel et al., 2010; Kurth et al., 2014). Although a time frame of several weeks is likely too short to draw sensible conclusions with respect to the impact of meditation in the framework of aging, the existing short-term longitudinal studies already demonstrate meditation-induced neuroplastic changes in brain structure. Controlled long-term longitudinal studies might reveal even more pronounced changes, especially if conducted over several years. Ideally, such trials will also obtain detailed measures of stress, inflammation, and telomere attributes to facilitate the interpretability of findings.
Aging is happening to each and all of us every single day. It is an ongoing process regardless of our birth dates, but the obvious sequels become most evident late in life. Aging is complex and our understanding of it still resembles an incomplete puzzle. However, we know that throughout the life span, there are multiple factors that may influence the dynamics of aging, and meditation may be one of them. Without a doubt, the accumulating scientific evidence is very encouraging, especially given that meditation is relatively easy to integrate in everyone's every-day life. Nevertheless, numerous open questions exist for diverse aspects, ranging from missing answers on the biological level, over the specifics of an effective (personalized) meditation intervention, to the general question of causality. Moreover, preliminary evidence for possible age-defying effects of meditation mostly stems from cross-sectional studies and/or from using indirect markers associated with aging. In contrast, controlled longitudinal studies between meditation and diminished brain aging are still missing. Admittedly, since aging is a rather slow and dynamic process, such prospective trials constitute challenging long-term endeavors but they seem necessary eventually to prove a causal relationship. In conclusion, before constructing effective prevention and/or intervention plans leveraging the potential of meditation to affect multiple pathways involved in (brain) aging, future research is clearly necessary to continue collecting still missing pieces and painting a more complete picture.
All authors FK, NC, EL contributed equally in writing and revising the manuscript.
The authors declare that the research was conducted in the absence of any commercial or financial relationships that could be construed as a potential conflict of interest.
Adler, A. S., Sinha, S., Kawahara, T. L., Zhang, J. Y., Segal, E., and Chang, H. Y. (2007). Motif module map reveals enforcement of aging by continual NF-kappaB activity. Genes Dev. 21, 3244–3257. doi: 10.1101/gad.1588507
Alda, M., Puebla-Guedea, M., Rodero, B., Demarzo, M., Montero-Marin, J., Roca, M., et al. (2016). Zen meditation, length of telomeres, and the role of experiential avoidance and compassion. Mindfulness 7, 651–659. doi: 10.1007/s12671-016-0500-5
Allsopp, R. C., Chang, E., Kashefi-Aazam, M., Rogaev, E. I., Piatyszek, M. A., Shay, J. W., et al. (1995). Telomere shortening is associated with cell division in vitro and in vivo. Exp. Cell Res. 220, 194–200. doi: 10.1006/excr.1995.1306
Bentson, J., Reza, M., Winter, J., and Wilson, G. (1978). Steroids and apparent cerebral atrophy on computed tomography scans. J. Comput. Assist. Tomogr. 2, 16–23. doi: 10.1097/00004728-197801000-00003
Besnard, A., and Sahay, A. (2016). Adult hippocampal neurogenesis, fear generalization, and stress. Neuropsychopharmacology 41, 24–44. doi: 10.1038/npp.2015.167
Bhasin, M. K., Dusek, J. A., Chang, B. H., Joseph, M. G., Denninger, J. W., Fricchione, G. L., et al. (2013). Relaxation response induces temporal transcriptome changes in energy metabolism, insulin secretion and inflammatory pathways. PLoS ONE 8:e62817. doi: 10.1371/journal.pone.0062817
Black, D. S., Cole, S. W., Irwin, M. R., Breen, E., St Cyr, N. M., Nazarian, N., et al. (2013). Yogic meditation reverses NF-κB and IRF-related transcriptome dynamics in leukocytes of family dementia caregivers in a randomized controlled trial. Psychoneuroendocrinology 38, 348–355. doi: 10.1016/j.psyneuen.2012.06.011
Blackburn, E. H., Epel, E. S., and Lin, J. (2015). Human telomere biology: a contributory and interactive factor in aging, disease risks, and protection. Science 350, 1193–1198. doi: 10.1126/science.aab3389
Blackburn, E. H., Greider, C. W., and Szostak, J. W. (2006). Telomeres and telomerase: the path from maize, Tetrahymena and yeast to human cancer and aging. Nat. Med. 12, 1133–1138. doi: 10.1038/nm1006-1133
Boersma, M. C., Dresselhaus, E. C., De Biase, L. M., Mihalas, A. B., Bergles, D. E., and Meffert, M. K. (2011). A requirement for nuclear factor-kappaB in developmental and plasticity-associated synaptogenesis. J. Neurosci. 31, 5414–5425. doi: 10.1523/JNEUROSCI.2456-10.2011
Bower, J. E., Crosswell, A. D., Stanton, A. L., Crespi, C. M., Winston, D., Arevalo, J., et al. (2015). Mindfulness meditation for younger breast cancer survivors: a randomized controlled trial. Cancer 121, 1231–1240. doi: 10.1002/cncr.29194
Bower, J. E., and Irwin, M. R. (2016). Mind-body therapies and control of inflammatory biology: a descriptive review. Brain Behav. Immun. 51, 1–11. doi: 10.1016/j.bbi.2015.06.012
Boyke, J., Driemeyer, J., Gaser, C., Buchel, C., and May, A. (2008). Training-induced brain structure changes in the elderly. J. Neurosci. 28, 7031–7035. doi: 10.1523/JNEUROSCI.0742-08.2008
Camandola, S., and Mattson, M. P. (2007). NF-kappa B as a therapeutic target in neurodegenerative diseases. Expert Opin. Ther. Targets 11, 123–132. doi: 10.1517/14728222.11.2.123
Chiesa, A., and Serretti, A. (2009). Mindfulness-based stress reduction for stress management in healthy people: a review and meta-analysis. J. Altern. Complement. Med. 15, 593–600. doi: 10.1089/acm.2008.0495
Cole, S. W. (2014). Human social genomics. PLoS Genet. 10:e1004601. doi: 10.1371/journal.pgen.1004601
Creswell, J. D., Irwin, M. R., Burklund, L. J., Lieberman, M. D., Arevalo, J. M., Ma, J., et al. (2012). Mindfulness-based stress reduction training reduces loneliness and pro-inflammatory gene expression in older adults: a small randomized controlled trial. Brain Behav. Immun. 26, 1095–1101. doi: 10.1016/j.bbi.2012.07.006
Creswell, J. D., Taren, A. A., Lindsay, E. K., Greco, C. M., Gianaros, P. J., Fairgrieve, A., et al. (2016). Alterations in resting-state functional connectivity link mindfulness meditation with reduced interleukin-6: a randomized controlled trial. Biol. Psychiatry 80, 53–61. doi: 10.1016/j.biopsych.2016.01.008
Doles, J., Storer, M., Cozzuto, L., Roma, G., and Keyes, W. M. (2012). Age-associated inflammation inhibits epidermal stem cell function. Genes Dev. 26, 2144–2153. doi: 10.1101/gad.192294.112
Draganski, B., Gaser, C., Busch, V., Schuierer, G., Bogdahn, U., and May, A. (2004). Neuroplasticity: changes in grey matter induced by training. Nature 427, 311–312. doi: 10.1038/427311a
Draganski, B., Gaser, C., Kempermann, G., Kuhn, H. G., Winkler, J., Buchel, C., et al. (2006). Temporal and spatial dynamics of brain structure changes during extensive learning. J. Neurosci. 26, 6314–6317. doi: 10.1523/JNEUROSCI.4628-05.2006
Eitan, E., Hutchison, E. R., and Mattson, M. P. (2014). Telomere shortening in neurological disorders: an abundance of unanswered questions. Trends Neurosci. 37, 256–263. doi: 10.1016/j.tins.2014.02.010
Epel, E., Daubenmier, J., Moskowitz, J. T., Folkman, S., and Blackburn, E. (2009). Can meditation slow rate of cellular aging? Cognitive stress, mindfulness, and telomeres. Ann. N.Y. Acad. Sci. 1172, 34–53. doi: 10.1111/j.1749-6632.2009.04414.x
Fox, K. C., Nijeboer, S., Dixon, M. L., Floman, J. L., Ellamil, M., Rumak, S. P., et al. (2014). Is meditation associated with altered brain structure? A systematic review and meta-analysis of morphometric neuroimaging in meditation practitioners. Neurosci. Biobehav. Rev. 43, 48–73. doi: 10.1016/j.neubiorev.2014.03.016
Fraser, M. A., Shaw, M. E., and Cherbuin, N. (2015). A systematic review and meta-analysis of longitudinal hippocampal atrophy in healthy human ageing. Neuroimage 112, 364–374. doi: 10.1016/j.neuroimage.2015.03.035
Garcia-Bueno, B., Caso, J. R., and Leza, J. C. (2008). Stress as a neuroinflammatory condition in brain: damaging and protective mechanisms. Neurosci. Biobehav. Rev. 32, 1136–1151. doi: 10.1016/j.neubiorev.2008.04.001
Gard, T., Holzel, B. K., and Lazar, S. W. (2014). The potential effects of meditation on age-related cognitive decline: a systematic review. Ann. N.Y. Acad. Sci. 1307, 89–103. doi: 10.1111/nyas.12348
Glorioso, C., and Sibille, E. (2011). Between destiny and disease: genetics and molecular pathways of human central nervous system aging. Prog. Neurobiol. 93, 165–181. doi: 10.1016/j.pneurobio.2010.11.006
Gonzalez-Giraldo, Y., Forero, D. A., Echeverria, V., Gonzalez, J., Avila-Rodriguez, M., Garcia-Segura, L. M., et al. (2016). Neuroprotective effects of the catalytic subunit of telomerase: a potential therapeutic target in the central nervous system. Ageing Res. Rev. 28, 37–45. doi: 10.1016/j.arr.2016.04.004
Grecucci, A., Pappaianni, E., Siugzdaite, R., Theuninck, A., and Job, R. (2015). Mindful emotion regulation: exploring the neurocognitive mechanisms behind mindfulness. Biomed Res. Int. 2015:670724. doi: 10.1155/2015/670724
Greenwood, P. M., and Parasuraman, R. (2010). Neuronal and cognitive plasticity: a neurocognitive framework for ameliorating cognitive aging. Front. Aging Neurosci. 2:150. doi: 10.3389/fnagi.2010.00150
Hanson, N. D., Owens, M. J., and Nemeroff, C. B. (2011). Depression, antidepressants, and neurogenesis: a critical reappraisal. Neuropsychopharmacology 36, 2589–2602. doi: 10.1038/npp.2011.220
Haustead, D. J., Stevenson, A., Saxena, V., Marriage, F., Firth, M., Silla, R., et al. (2016). Transcriptome analysis of human ageing in male skin shows mid-life period of variability and central role of NF-κB. Sci. Rep. 6:26846. doi: 10.1038/srep26846
Hayflick, L., and Moorhead, P. S. (1961). The serial cultivation of human diploid cell strains. Exp. Cell Res. 25, 585–621. doi: 10.1016/0014-4827(61)90192-6
Hedman, A. M., Van Haren, N. E., Schnack, H. G., Kahn, R. S., and Hulshoff Pol, H. E. (2012). Human brain changes across the life span: a review of 56 longitudinal magnetic resonance imaging studies. Hum. Brain Mapp. 33, 1987–2002. doi: 10.1002/hbm.21334
Hekimi, S., Lapointe, J., and Wen, Y. (2011). Taking a “good” look at free radicals in the aging process. Trends Cell Biol. 21, 569–576. doi: 10.1016/j.tcb.2011.06.008
Helenius, M., Hanninen, M., Lehtinen, S. K., and Salminen, A. (1996). Changes associated with aging and replicative senescence in the regulation of transcription factor nuclear factor-kappa B. Biochem. J. 318(Pt 2), 603–608. doi: 10.1042/bj3180603
Hoge, E. A., Chen, M. M., Orr, E., Metcalf, C. A., Fischer, L. E., Pollack, M. H., et al. (2013). Loving-kindness meditation practice associated with longer telomeres in women. Brain Behav. Immun. 32, 159–163. doi: 10.1016/j.bbi.2013.04.005
Holzel, B. K., Carmody, J., Evans, K. C., Hoge, E. A., Dusek, J. A., Morgan, L., et al. (2010). Stress reduction correlates with structural changes in the amygdala. Soc. Cogn. Affect. Neurosci. 5, 11–17. doi: 10.1093/scan/nsp034
Holzel, B. K., Lazar, S. W., Gard, T., Schuman-Olivier, Z., Vago, D. R., and Ott, U. (2011). How does mindfulness meditation work? proposing mechanisms of action from a conceptual and neural perspective. Perspect. Psychol. Sci. 6, 537–559. doi: 10.1177/1745691611419671
Irwin, M. R., and Cole, S. W. (2011). Reciprocal regulation of the neural and innate immune systems. Nat. Rev. Immunol. 11, 625–632. doi: 10.1038/nri3042
Jacobs, T. L., Epel, E. S., Lin, J., Blackburn, E. H., Wolkowitz, O. M., Bridwell, D. A., et al. (2011). Intensive meditation training, immune cell telomerase activity, and psychological mediators. Psychoneuroendocrinology 36, 664–681. doi: 10.1016/j.psyneuen.2010.09.010
Joergensen, A., Broedbaek, K., Weimann, A., Semba, R. D., Ferrucci, L., Joergensen, M. B., et al. (2011). Association between urinary excretion of cortisol and markers of oxidatively damaged DNA and RNA in humans. PLoS ONE 6:e20795. doi: 10.1371/journal.pone.0020795
Knoops, A. J., Gerritsen, L., Van Der Graaf, Y., Mali, W. P., and Geerlings, M. I. (2010). Basal hypothalamic pituitary adrenal axis activity and hippocampal volumes: the SMART-Medea study. Biol. Psychiatry 67, 1191–1198. doi: 10.1016/j.biopsych.2010.01.025
Koike, M. K., and Cardoso, R. (2014). Meditation can produce beneficial effects to prevent cardiovascular disease. Horm. Mol. Biol. Clin. Investig. 18, 137–143. doi: 10.1515/hmbci-2013-0056
Kurth, F., Cherbuin, N., and Luders, E. (2015). Reduced age-related degeneration of the hippocampal subiculum in long-term meditators. Psychiatry Res. 232, 214–218. doi: 10.1016/j.pscychresns.2015.03.008
Kurth, F., Luders, E., Wu, B., and Black, D. S. (2014). Brain gray matter changes associated with mindfulness meditation in older adults: an exploratory pilot study using voxel-based morphometry. Neuro 1, 23–26. doi: 10.17140/NOJ-1-106
Laneri, D., Schuster, V., Dietsche, B., Jansen, A., Ott, U., and Sommer, J. (2016). Effects of long-term mindfulness meditation on brain's white matter microstructure and its aging. Front. Aging Neurosci. 7:254. doi: 10.3389/fnagi.2015.00254
Lavretsky, H., Epel, E. S., Siddarth, P., Nazarian, N., Cyr, N. S., Khalsa, D. S., et al. (2013). A pilot study of yogic meditation for family dementia caregivers with depressive symptoms: effects on mental health, cognition, and telomerase activity. Int. J. Geriatr. Psychiatry 28, 57–65. doi: 10.1002/gps.3790
Lazar, S. W., Kerr, C. E., Wasserman, R. H., Gray, J. R., Greve, D. N., Treadway, M. T., et al. (2005). Meditation experience is associated with increased cortical thickness. Neuroreport 16, 1893–1897. doi: 10.1097/01.wnr.0000186598.66243.19
Lengacher, C. A., Kip, K. E., Barta, M., Post-White, J., Jacobsen, P. B., Groer, M., et al. (2012). A pilot study evaluating the effect of mindfulness-based stress reduction on psychological status, physical status, salivary cortisol, and interleukin-6 among advanced-stage cancer patients and their caregivers. J. Holist. Nurs. 30, 170–185. doi: 10.1177/0898010111435949
Lin, J., Epel, E., and Blackburn, E. (2012). Telomeres and lifestyle factors: roles in cellular aging. Mutat. Res. 730, 85–89. doi: 10.1016/j.mrfmmm.2011.08.003
Lippelt, D. P., Hommel, B., and Colzato, L. S. (2014). Focused attention, open monitoring and loving kindness meditation: effects on attention, conflict monitoring, and creativity - A review. Front. Psychol. 5:1083. doi: 10.3389/fpsyg.2014.01083
Liston, C., and Gan, W. B. (2011). Glucocorticoids are critical regulators of dendritic spine development and plasticity in vivo. Proc. Natl. Acad. Sci. U.S.A. 108, 16074–16079. doi: 10.1073/pnas.1110444108
Lopez-Otin, C., Blasco, M. A., Partridge, L., Serrano, M., and Kroemer, G. (2013). The hallmarks of aging. Cell 153, 1194–1217. doi: 10.1016/j.cell.2013.05.039
Luders, E. (2014). Exploring age-related brain degeneration in meditation practitioners. Ann. N.Y. Acad. Sci. 1307, 82–88. doi: 10.1111/nyas.12217
Luders, E., and Cherbuin, N. (2016). Searching for the philosopher's stone: promising links between meditation and brain preservation. Ann. N.Y. Acad. Sci. 1373, 38–44. doi: 10.1111/nyas.13082
Luders, E., Cherbuin, N., and Gaser, C. (2016). Estimating brain age using high-resolution pattern recognition: younger brains in long-term meditation practitioners. Neuroimage 134, 508–513. doi: 10.1016/j.neuroimage.2016.04.007
Luders, E., Cherbuin, N., and Kurth, F. (2015). Forever young(er): potential age-defying effects of long-term meditation on gray matter atrophy. Front. Psychol. 5:1551. doi: 10.3389/fpsyg.2014.01551
Luders, E., Clark, K., Narr, K. L., and Toga, A. W. (2011). Enhanced brain connectivity in long-term meditation practitioners. Neuroimage 57, 1308–1316. doi: 10.1016/j.neuroimage.2011.05.075
Lutz, A., Slagter, H. A., Dunne, J. D., and Davidson, R. J. (2008). Attention regulation and monitoring in meditation. Trends Cogn. Sci. 12, 163–169. doi: 10.1016/j.tics.2008.01.005
Malarkey, W. B., Jarjoura, D., and Klatt, M. (2013). Workplace based mindfulness practice and inflammation: a randomized trial. Brain Behav. Immun. 27, 145–154. doi: 10.1016/j.bbi.2012.10.009
Marciniak, R., Sheardova, K., Cermakova, P., Hudecek, D., Sumec, R., and Hort, J. (2014). Effect of meditation on cognitive functions in context of aging and neurodegenerative diseases. Front. Behav. Neurosci. 8:17. doi: 10.3389/fnbeh.2014.00017
Mattson, M. P. (2005). NF-kappaB in the survival and plasticity of neurons. Neurochem. Res. 30, 883–893. doi: 10.1007/s11064-005-6961-x
Mattson, M. P., Chan, S. L., and Duan, W. (2002). Modification of brain aging and neurodegenerative disorders by genes, diet, and behavior. Physiol. Rev. 82, 637–672. doi: 10.1152/physrev.00004.2002
Mattson, M. P., and Meffert, M. K. (2006). Roles for NF-kappaB in nerve cell survival, plasticity, and disease. Cell Death Differ. 13, 852–860. doi: 10.1038/sj.cdd.4401837
Mcewen, B. S. (2001). Plasticity of the hippocampus: adaptation to chronic stress and allostatic load. Ann. N.Y. Acad. Sci. 933, 265–277. doi: 10.1111/j.1749-6632.2001.tb05830.x
Moynihan, J. A., Chapman, B. P., Klorman, R., Krasner, M. S., Duberstein, P. R., Brown, K. W., et al. (2013). Mindfulness-based stress reduction for older adults: effects on executive function, frontal alpha asymmetry and immune function. Neuropsychobiology 68, 34–43. doi: 10.1159/000350949
Nakamura, K., Takubo, K., Izumiyama-Shimomura, N., Sawabe, M., Arai, T., Kishimoto, H., et al. (2007). Telomeric DNA length in cerebral gray and white matter is associated with longevity in individuals aged 70 years or older. Exp. Gerontol. 42, 944–950. doi: 10.1016/j.exger.2007.05.003
Oeppen, J., and Vaupel, J. W. (2002). Demography. Broken limits to life expectancy. Science 296, 1029–1031. doi: 10.1126/science.1069675
Ortman, J. M., Velkoff, V. A., and Hogan, H. (2014). An Aging Nation: The Older Population in the United States. Current Population Reports [Online], P25–P1140.
Pagnoni, G., and Cekic, M. (2007). Age effects on gray matter volume and attentional performance in Zen meditation. Neurobiol. Aging 28, 1623–1627. doi: 10.1016/j.neurobiolaging.2007.06.008
Price, L. H., Kao, H. T., Burgers, D. E., Carpenter, L. L., and Tyrka, A. R. (2013). Telomeres and early-life stress: an overview. Biol. Psychiatry 73, 15–23. doi: 10.1016/j.biopsych.2012.06.025
Rao, K. S., Chakraharti, S. K., Dongare, V. S., Chetana, K., Ramirez, C. M., Koka, P. S., et al. (2015). Antiaging effects of an intensive mind and body therapeutic program through enhancement of telomerase activity and adult stem cell counts. J. Stem Cells 10, 107–125.
Ray, I. B., Menezes, A. R., Malur, P., Hiltbold, A. E., Reilly, J. P., and Lavie, C. J. (2014). Meditation and coronary heart disease: a review of the current clinical evidence. Ochsner J. 14, 696–703.
Revesz, D., Verhoeven, J. E., Milaneschi, Y., De Geus, E. J., Wolkowitz, O. M., and Penninx, B. W. (2014). Dysregulated physiological stress systems and accelerated cellular aging. Neurobiol. Aging 35, 1422–1430. doi: 10.1016/j.neurobiolaging.2013.12.027
Sahin, E., Colla, S., Liesa, M., Moslehi, J., Muller, F. L., Guo, M., et al. (2011). Telomere dysfunction induces metabolic and mitochondrial compromise. Nature 470, 359–365. doi: 10.1038/nature09787
Salminen, A., Huuskonen, J., Ojala, J., Kauppinen, A., Kaarniranta, K., and Suuronen, T. (2008). Activation of innate immunity system during aging: NF-κB signaling is the molecular culprit of inflamm-aging. Ageing Res. Rev. 7, 83–105. doi: 10.1016/j.arr.2007.09.002
Salminen, A., Kaarniranta, K., and Kauppinen, A. (2012a). Inflammaging: disturbed interplay between autophagy and inflammasomes. Aging 4, 166–175. doi: 10.18632/aging.100444
Salminen, A., Ojala, J., Kaarniranta, K., and Kauppinen, A. (2012b). Mitochondrial dysfunction and oxidative stress activate inflammasomes: impact on the aging process and age-related diseases. Cell. Mol. Life Sci. 69, 2999–3013. doi: 10.1007/s00018-012-0962-0
Schutte, N. S., and Malouff, J. M. (2014). A meta-analytic review of the effects of mindfulness meditation on telomerase activity. Psychoneuroendocrinology 42, 45–48. doi: 10.1016/j.psyneuen.2013.12.017
Sharma, M., and Rush, S. E. (2014). Mindfulness-based stress reduction as a stress management intervention for healthy individuals: a systematic review. J. Evid. Based Complement. Altern. Med. 19, 271–286. doi: 10.1177/2156587214543143
Shen, S., Sandoval, J., Swiss, V. A., Li, J., Dupree, J., Franklin, R. J., et al. (2008). Age-dependent epigenetic control of differentiation inhibitors is critical for remyelination efficiency. Nat. Neurosci. 11, 1024–1034. doi: 10.1038/nn.2172
Starkman, M. N., Gebarski, S. S., Berent, S., and Schteingart, D. E. (1992). Hippocampal formation volume, memory dysfunction, and cortisol levels in patients with Cushing's syndrome. Biol. Psychiatry 32, 756–765. doi: 10.1016/0006-3223(92)90079-F
Starkman, M. N., Giordani, B., Gebarski, S. S., Berent, S., Schork, M. A., and Schteingart, D. E. (1999). Decrease in cortisol reverses human hippocampal atrophy following treatment of Cushing's disease. Biol. Psychiatry 46, 1595–1602. doi: 10.1016/S0006-3223(99)00203-6
Stern, Y. (2012). Cognitive reserve in ageing and Alzheimer's disease. Lancet Neurol. 11, 1006–1012. doi: 10.1016/S1474-4422(12)70191-6
Tabatabaei-Jafari, H., Shaw, M. E., and Cherbuin, N. (2015). Cerebral atrophy in mild cognitive impairment: a systematic review with meta-analysis. Alzheimers Dement (Amst.) 1, 487–504. doi: 10.1016/j.dadm.2015.11.002
Turan, B., Foltz, C., Cavanagh, J. F., Wallace, B. A., Cullen, M., Rosenberg, E. L., et al. (2015). Anticipatory sensitization to repeated stressors: the role of initial cortisol reactivity and meditation/emotion skills training. Psychoneuroendocrinology 52, 229–238. doi: 10.1016/j.psyneuen.2014.11.014
Uno, H., Tarara, R., Else, J. G., Suleman, M. A., and Sapolsky, R. M. (1989). Hippocampal damage associated with prolonged and fatal stress in primates. J. Neurosci. 9, 1705–1711.
Valkanova, V., Eguia Rodriguez, R., and Ebmeier, K. P. (2014). Mind over matter–what do we know about neuroplasticity in adults? Int. Psychogeriatr. 26, 891–909. doi: 10.1017/S1041610213002482
Wang, W., Ji, P., and Dow, K. E. (2003). Corticotropin-releasing hormone induces proliferation and TNF-alpha release in cultured rat microglia via MAP kinase signalling pathways. J. Neurochem. 84, 189–195. doi: 10.1046/j.1471-4159.2003.01544.x
Watanabe, Y., Gould, E., and Mcewen, B. S. (1992). Stress induces atrophy of apical dendrites of hippocampal CA3 pyramidal neurons. Brain Res. 588, 341–345. doi: 10.1016/0006-8993(92)91597-8
Westlye, L. T., Walhovd, K. B., Dale, A. M., Bjornerud, A., Due-Tonnessen, P., Engvig, A., et al. (2010). Life-span changes of the human brain white matter: diffusion tensor imaging (DTI) and volumetry. Cereb. Cortex 20, 2055–2068. doi: 10.1093/cercor/bhp280
WHO (2015). WHO: Number of People Over 60 Years Set to Double by 2050; Major Societal Changes Required [Online]. Geneva: WHO (Accessed 2016).
Wolkin, J. R. (2015). Cultivating multiple aspects of attention through mindfulness meditation accounts for psychological well-being through decreased rumination. Psychol. Res. Behav. Manag. 8, 171–180. doi: 10.2147/PRBM.S31458
Woollett, K., and Maguire, E. A. (2011). Acquiring “the Knowledge” of London's layout drives structural brain changes. Curr. Biol. 21, 2109–2114. doi: 10.1016/j.cub.2011.11.018
Ye, J., Renault, V. M., Jamet, K., and Gilson, E. (2014). Transcriptional outcome of telomere signalling. Nat. Rev. Genet. 15, 491–503. doi: 10.1038/nrg3743
Zahran, S., Snodgrass, J. G., Maranon, D. G., Upadhyay, C., Granger, D. A., and Bailey, S. M. (2015). Stress and telomere shortening among central Indian conservation refugees. Proc. Natl. Acad. Sci. U.S.A. 112, E928–E936. doi: 10.1073/pnas.1411902112
Zeng, X., Chiu, C. P., Wang, R., Oei, T. P., and Leung, F. Y. (2015). The effect of loving-kindness meditation on positive emotions: a meta-analytic review. Front. Psychol. 6:1693. doi: 10.3389/fpsyg.2015.01693
Zhang, D., Cheng, L., Craig, D. W., Redman, M., and Liu, C. (2010). Cerebellar telomere length and psychiatric disorders. Behav. Genet. 40, 250–254. doi: 10.1007/s10519-010-9338-0
Keywords: aging, brain, meditation, mindfulness, MRI
Citation: Kurth F, Cherbuin N and Luders E (2017) Promising Links between Meditation and Reduced (Brain) Aging: An Attempt to Bridge Some Gaps between the Alleged Fountain of Youth and the Youth of the Field. Front. Psychol. 8:860. doi: 10.3389/fpsyg.2017.00860
Received: 15 October 2016; Accepted: 10 May 2017;
Published: 30 May 2017.
Edited by:
Barbara Tomasino, University of Udine, ItalyReviewed by:
Maarten A. Immink, University of South Australia, AustraliaCopyright © 2017 Kurth, Cherbuin and Luders. This is an open-access article distributed under the terms of the Creative Commons Attribution License (CC BY). The use, distribution or reproduction in other forums is permitted, provided the original author(s) or licensor are credited and that the original publication in this journal is cited, in accordance with accepted academic practice. No use, distribution or reproduction is permitted which does not comply with these terms.
*Correspondence: Zmt1cnRoQHVjbGEuZWR1
†These authors have contributed equally to this work.
Disclaimer: All claims expressed in this article are solely those of the authors and do not necessarily represent those of their affiliated organizations, or those of the publisher, the editors and the reviewers. Any product that may be evaluated in this article or claim that may be made by its manufacturer is not guaranteed or endorsed by the publisher.
Research integrity at Frontiers
Learn more about the work of our research integrity team to safeguard the quality of each article we publish.