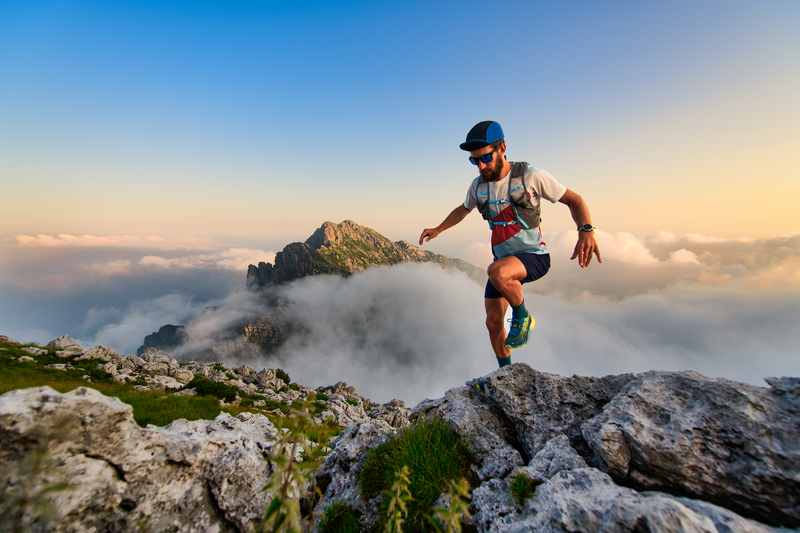
95% of researchers rate our articles as excellent or good
Learn more about the work of our research integrity team to safeguard the quality of each article we publish.
Find out more
ORIGINAL RESEARCH article
Front. Psychol. , 28 May 2012
Sec. Psychology of Language
Volume 3 - 2012 | https://doi.org/10.3389/fpsyg.2012.00161
This article is part of the Research Topic At the doors of lexical access: The importance of the first 250 milliseconds in reading View all 11 articles
Reading is a difficult task that, at a minimum, requires recognizing a visual stimulus and linking it with its corresponding sound and meaning. Neurologically, this involves an anatomically distributed set of brain regions cooperating to solve the problem. It has been hypothesized that the supramarginal gyrus (SMG) contributes preferentially to phonological aspects of word processing and thus plays an important role in visual word recognition. Here, we used chronometric transcranial magnetic stimulation (TMS) to investigate the functional specificity and timing of SMG involvement in reading visually presented words. Participants performed tasks designed to focus on either the phonological, semantic, or visual aspects of written words while double pulses of TMS (delivered 40 ms apart) were used to temporarily interfere with neural information processing in the left SMG at five different time windows. Stimulation at 80/120, 120/160, and 160/200 ms post-stimulus onset significantly slowed subjects’ reaction times in the phonological task. This inhibitory effect was specific to the phonological condition, with no effect of TMS in the semantic or visual tasks, consistent with claims that SMG contributes preferentially to phonological aspects of word processing. The fact that the effect began within 80–120 ms of the onset of the stimulus and continued for approximately 100 ms, indicates that phonological processing initiates early and is sustained over time. These findings are consistent with accounts of visual word recognition that posit parallel activation of orthographic, phonological, and semantic information that interact over time to settle into a distributed, but stable, representation of a word.
From texts to twitter, e-mails to blogs, we live in a society that is dominated by written communication. The ease with which we read masks a complex set of processes necessary to link visual symbols with their sounds and meaning. At a neural level, these processes engage an anatomically distributed set of brain regions that, at a minimum, include broad areas of the ventral occipito-temporal (vOT) cortex, the inferior parietal lobule (IPL), and inferior frontal cortex (Pugh et al., 2001; Shaywitz et al., 2002; Price and Mechelli, 2005). Here we focused on a specific sub-field of the IPL, namely the supramarginal gyrus (SMG), and investigated both its functional contribution to reading and also its time course using transcranial magnetic stimulation (TMS).
The IPL is an anatomically heterogeneous area consisting of several distinct cytoarchitectonic fields (Brodmann, 1909; Von Bonin and Bailey, 1947), each with their own pattern of connectivity (Rushworth et al., 2006; Caspers et al., 2011). The most anterior field corresponds to the SMG, an area strongly linked to phonological processing (Petersen et al., 1988; Booth et al., 2004; Seghier et al., 2004; Zevin and McCandliss, 2005; Prabhakaran et al., 2006; Raizada and Poldrack, 2007; Buchsbaum and D’Esposito, 2008; Obleser and Kotz, 2009; Sharp et al., 2010; Yoncheva et al., 2010). Indeed, neuroimaging evidence demonstrates that SMG responds more strongly during phonological than semantic processing (Demonet et al., 1994; Price et al., 1997; Mummery et al., 1998; Devlin et al., 2003), suggesting a level functional specificity during word recognition. Thus it was surprising that a recent TMS experiment (Stoeckel et al., 2009) found stimulation of the left SMG facilitated both phonological and semantic processing, calling into question the specificity of SMG’s contribution to reading. It is certainly possible that differences between the fMRI and TMS methodologies could yield conflicting results (e.g., Hamidi et al., 2009), thus we were motivated to further investigate the functional specificity of the SMG during word recognition using a more robust stimulation technique than used in the earlier study.
Our second aim was to investigate the temporal dynamics of SMG contributions to language processing. Traditionally, event-related potential (ERP) and magnetoencephalography (MEG) are most commonly used to measure the time course of processing, taking advantage of their outstanding temporal resolution. Several such studies have reported phonological effects occurring 250–350 ms after the appearance of the visual word (Niznikiewicz and Squires, 1996; Bentin et al., 1999; Newman and Connolly, 2004; Grainger et al., 2006; Ashby and Martin, 2008). Sereno and colleagues, however, have argued compellingly that the phonological (and semantic) processing must happen more rapidly, based on the rapidity of eye movements during text reading (Sereno et al., 1998; Sereno and Rayner, 2003). In addition, they have used ERPs to demonstrate that higher order properties of words are accessed as early as 100–200 ms after stimulus onset (Sereno et al., 1998). These findings receive additional support from recent ERP and MEG studies suggesting that phonological processing may begin within the first 100 ms of visual word recognition (Ashby et al., 2009; Wheat et al., 2010). Here, we used chronometric TMS to take advantage of its combined temporal (tens of ms) and spatial resolution (approximately 10 mm) to investigate the timing of SMG involvement in reading.
Forty right-handed, monolingual native English speakers volunteered to participate in this study, and of these 32 (19 women, 13 men; aged 18–41, mean = 25) were included in the main experiment. For the other eight the functional location procedure failed to identify a region of SMG for testing in the main experiment (see Experimental Procedures below). All participants were neurologically normal, with no personal or family history of epilepsy. In addition, none had any form of dyslexia according to self-reports. Each person provided informed consent after the experimental procedures were explained and subjects were paid for their participation. The experiment was approved by the University College London (UCL) Research Ethics Committee.
There were two testing sessions. The first involved a 30-min visit to the Birkbeck-UCL Neuroimaging Centre (BUCNI) to acquire a T1-weighted structural magnetic resonance imaging (MRI) scan [FLASH sequence, repetition time (TR) = 12 ms, echotime (TE) = 5.6 ms, flip angle = 19, resolution = 1 mm × 1 mm × 1 mm] used to anatomically identify the left SMG in each participant. The second session occurred 2–10 days later and involved the main TMS testing which lasted approximately 1 h.
Before a participant arrived for TMS testing, three potential stimulation targets were identified and marked on their MRI scan using the Brainsight frameless stereotaxy system (Rogue Research, Montreal, Canada). The first was located just superior to the termination of the posterior ascending ramus of the Sylvian fissure. The second was placed at the ventral end of the anterior SMG, superior to the Sylvian fissure, posterior to the postcentral sulcus, and anterior to the posterior ascending ramus of the Sylvian fissure. The third was approximately halfway between these sites and at least 10–15 mm from the other two (see Figure 1A). These three sites were chosen within the anterior region of the left SMG since this area has been shown to be sensitive to phonological processing in neuroimaging studies (e.g., Petersen et al., 1988; Price et al., 1997; Devlin et al., 2003; Seghier et al., 2004; Zevin and McCandliss, 2005; Raizada and Poldrack, 2007). Each site was then tested to functionally localize the specific target site where stimulation interfered with phonological processing.
Figure 1. Stimulation sites. (A) Three possible stimulation targets marked within each participant’s left SMG using a frameless stereotaxy system. The first one located just superior to the termination of the posterior ascending ramus of the Sylvian fissure; the second one at the ventral end of the anterior SMG; and the third one approximately halfway between the other two sites. (B) The final testing sites for all 32 participants (white filled circles) and the mean group location (black filled circle) on the averaged brain of all participants normalized to the standard MNI152 space with an affine registration (Jenkinson and Smith, 2001) shown on a parasagittal plane.
Participants performed a visual rhyme judgment task to focus attention on the sounds of the words. Each trial began with a fixation cross centrally presented on the screen for 1000 ms immediately followed by two words that appeared simultaneously above and below the cross and remained on the screen for 500 ms. Subjects were asked to judge whether the two words rhymed or not (e.g., kite-white) during a 2500-ms inter-trial interval (ITI). Responses were indicated by button press using the left and right index fingers. The pairing of yes/no responses with fingers was counter-balanced across participants. Each run included 34 trials and lasted 1:35 min. Repetitive TMS (10 Hz, 500 ms) was delivered randomly on half of the trials with the caveat that they occurred equally often on yes and no trials. Stimulation began 100 ms after the onset of the word pair. The data from the first two trials per run were discarded to allow participants to get past anticipating the first rTMS trial. When TMS consistently slowed median reaction times (RTs) relative to non-TMS trials, that site was used for testing in the main experiment.
At the beginning of testing, participants performed a practice run of the rhyme judgment task where no TMS was delivered to become familiar with the task. Once they felt comfortable, the first testing site was chosen and the participant was introduced to the sensation of rTMS at that site. TMS was introduced by placing the coil on the scalp such that the line of maximum magnetic flux intersected the target site. After familiarization with the sensation, each participant performed two more practice runs with concurrent rTMS. Localization then began at the first testing site and each site was tested using one of five matched stimulus sets. When rTMS facilitated RTs, the next site was tested. When rTMS produced numerically longer (i.e., slower) responses, the site was re-tested using a different stimulus set to determine whether the observed slowdown was consistent. Any site that produced two or more RT slowdowns during the localizer task was selected for stimulation in the main experiment. Note that any numeric increase in RTs, including a single millisecond, was considered a “slowdown,” which is why it was important to show that slowdowns were consistent rather than a result of idiosyncratic factors. In general, the fact that the “wrong” SMG sites typically led to small speedup effects (presumably due to intersensory facilitation), made even small slowdowns convincing as long as they were reproducible. Once a testing site was identified, the localization procedure stopped in order to limit unnecessary stimulation received by subjects. The order of testing the target sites was counter-balanced across participants. If after 10 runs, no site resulted in consistent TMS-induced slowdowns, then the experiment was terminated.
In the main experiment, participants performed three different tasks: (i) a homophone judgment task where they decided whether two words sounded the same; (ii) a synonym judgment task where they decided whether two words meant the same thing; and (iii) a visual judgment control task where they decided whether two consonant letters strings were identical. The first two tasks were designed to emphasize phonological or semantic processing, respectively. The third task was included as a control condition in which stimulation was not expected to affect performance. This task shared visual, decision, and response features of the lexical tasks but no linguistic components. The number of “yes” and “no” responses was equal in all cases.
There were 105 trials per task. The tasks were presented in blocks of 21 trials to minimize task-switching costs. Following a short instruction screen to remind the participant of the task, the first trial in each block was a dummy item and discarded from the analyses to exclude the RT cost of switching tasks. The remaining 20 items in the block constituted the data used for further analysis. A trial commenced with a fixation cross displayed for 500 ms, followed by two letter strings presented above and below the fixation cross for another 500 ms. A blank screen was then presented for a random interval between 1300 and 2300 ms, giving an average duration of 2500 ms per trial. Participants indicated their response with the same button press they used in the functional localizer task. The experiment was divided into three runs of five blocks each lasting approximately 5 min. In between runs, subjects took a self-paced break. The order of tasks was counter-balanced across participants.
A double pulse of TMS was delivered on every trial, at one of five different timing conditions. Pulses occurred at either 40 and 80, 80 and 120, 120 and 160, 160 and 200, or 200 and 240 ms post-stimulus onset. The TMS timings were not randomly distributed; instead, they were ordered in either an ascending or descending staircase in sets of four trials (Figure 2). For instance, the first four trials might have pulses delivered at 40/80 ms, while the next four were at 80/120, etc., such that all 20 trials in the block had TMS delivered at one of the five timing conditions. For the following block (i.e., the next task), the timing went in the opposite direction (i.e., 4 × 200/240 followed by 4 × 160/200, etc.). The aim of this procedure was to avoid any late stimulation trials (e.g., 160/200) randomly following early trials (40/80) because during pilot studies there was some concern that participants were implicitly waiting for the TMS pulse before responding, and thus artificially inflating RTs on those trials. With the current staircase method there was no evidence that participants waited for the TMS before responding. Indeed, subjects reported that they were not aware that stimulation onsets differed. In contrast, when chronometric timings are delivered randomly subjects are typically aware of the different timings.
Figure 2. (A) Within a run, homophones (H), synonyms (S), and consonant strings (C) alternated in 50 s blocks. (B) Each block consisted of 20 trials. Pulses occurred at either 40/80, 80/120, 120/160, 160/240, or 200/240 ms post-stimulus onset. TMS timings were ordered in either an ascending or descending staircase in sets of four trials. H0 and S0 indicate dummy trials. (C) Each trial began with a fixation cross presented for 500 ms. A stimulus was then presented for 250 m, followed by a blank screen displayed for random interval between 1300 and 2300 ms. Stimulation occurred at one of five time windows.
Testing began with a practice run performed without TMS in order to familiarize subjects with the task requirements. It included all three tasks and provided practice in switching between them. Subjects were then familiarized with the sensation of double-pulse TMS at the SMG testing site. Finally, they completed the actual experiment for the given site using one of five different stimulus versions. The order of the versions was counter-balanced among participants. None of stimuli used in practice or in the localization procedure were repeated in the main task.
For the localizer task, word stimuli (n = 160 plus 10 dummy trials) ranged in length from three to eight letters and were divided into five separate lists, matched for concreteness, familiarity, written word frequency, number of letters, and number of syllables [one-way ANOVA, all F(1, 158) < 1.1, p > 0.31]. Concreteness and familiarity ratings were taken from the MRC Psycholinguistic database (Coltheart, 1981), and British English word frequencies came from the Celex database (Baayen and Pipenbrook, 1995). In addition, within each list trials were divided into TMS and no-TMS items that were also matched across these five factors [all t(30) < 1.8, p > 0.1]. It is worth noting that the orthography of the paired words was manipulated such that participants could not perform rhyme judgment based solely on the word’s spelling. The words in rhyming and non-rhyming trials had different spellings in half of the cases (e.g., rhyming: wall-call vs. style-pile; non-rhyming: work-pork vs. egg-pen).
For the main experiment, the word stimuli (200 trials plus 12 dummies trials) ranged in length from 3 to 10 letters and were matched across the homophone and synonym tasks for concreteness, familiarity, written word frequency, number of letters, and number of syllables [all t(198) < 1.66, p > 0.11]. In addition, the consonant strings in the non-lexical task were matched in length to the lexical stimuli. Within each task, the items were divided into five lists, again matched for all factors [all F(4, 95) < 2.1, p > 0.1]. Then, the lists were paired with each of the five time windows such that the lists occurred with equal frequency within each time window across participants.
Stimulation was performed using a Magstim Rapid2 stimulator (Magstim, Carmarthenshire, UK) and a 70-mm diameter figure-of-eight coil. The stimulation intensity was set to 55% of the maximum stimulator output and held constant for all subjects. During the localizer task, trains of five pulses (i.e., 10 Hz for 500 ms) were pseudorandomly delivered at 100, 200, 300, 400, 500 ms post-stimulus onset in half of all trials. During the main task, double pulses were delivered 40 ms apart at five different time windows: 40/80, 80/120, 120/160, 160/200, and 200/240 ms following stimulus onset in each trial. The TMS frequency, intensity, and duration were well within established international safety limits (Wassermann, 1998; Rossi et al., 2009). During testing, a Polaris Vicra infrared camera (Northern Digital, Waterloo, ON, Canada) was used in conjunction with the Brainsight frameless stereotaxy system (Rogue Research, Montreal, Canada) to register the participant’s head to their own MRI scan in order to accurately target stimulation throughout the experiment. All participants used an earplug in their left ear to attenuate the sound of the coil discharge and avoid damage to the ear (Counter et al., 1991). All participants tolerated TMS well. In some cases, stimulation affected the temporalis muscle and produced a small, unilateral facial twitch. Participants described the sensations as “unusual” but not uncomfortable.
Reaction times were recorded from the onset of the stimulus and only correct responses were analyzed. TMS was expected to affect RTs rather than accuracy, as previous studies utilizing similar language tasks and stimuli indicate that TMS rarely affects accuracy (Devlin and Watkins, 2007). For the localizer task, the group analysis compared responses to TMS and no-TMS trials when TMS was delivered to the main testing site vs. when it was delivered to the other SMG targets. For the main task the earliest timing window (i.e., pulses delivered at 40/80 ms) was considered the baseline condition as previous ERP, MEG, and TMS findings (e.g., Khateb et al., 1999; Pammer et al., 2004; Stoeckel et al., 2009) indicate that this is too early for TMS to have an effect on SMG during phonological processing. As a result, within each of the three tasks, each of the four later time windows was compared to the baseline, using two-tailed, planned paired t-tests. Anticipatory responses were defined as RTs ≤300 ms and were trimmed from the data (0.04% of responses). In all analyses, median RTs for correct responses were used in the statistical analyses to minimize the effect of outliers (Ulrich and Miller, 1994).
In order to identify testing sites in terms of standard space coordinates, each participant’s structural scan was registered to the Montreal Neurological Institute-152 template using an affine registration (Jenkinson and Smith, 2001). Note that all stimulation was done in native anatomical space – the standard space coordinates were computed solely for reporting purposes. In addition, for illustrative purposes a group mean structural scan was created in standard space and used as a background image when presenting the stimulation sites in order to accurately reflect the anatomical variability across subjects (Devlin and Poldrack, 2007).
In 8 out of 40 participants, the functional localization process failed and testing ceased after 10 runs. In the remaining 32 participants, an average of five localizer runs per subject (range: 2–10, mean = 6) were required to successfully identify the main SMG testing site. In these participants, rTMS produced a significant inhibitory effect of 44 ms relative to the no-TMS trials [paired t-test; t(31) = 9.8, p < 0.001]. When normalized to reflect between-subject variability in overall RT, this equated to a 6% slowdown in individuals. In contrast, stimulation of the other SMG sites produced a significant facilitation effect of 32 ms [paired t-test; t(31) = 4.9, p < 0.001]. When normalized, this constituted a 4% speed-up in RTs. In other words, there was a clear difference between the final test site and other locations, even though they were only 1–2 cm away and still within anterior SMG. The precise location where stimulation interfered with phonological processing varied across individuals and is illustrated in Figure 1B. Here, white filled circles show where stimulation led to a slowdown for rhyme judgments in each participant. The mean coordinate in standard space was [−52, −37, +32], a region previously implicated in phonological processing (e.g., Price et al., 1997; Devlin et al., 2003; Seghier et al., 2004; Zevin and McCandliss, 2005; Raizada and Poldrack, 2007).
Overall accuracy levels were reasonably high (88%) indicating that participants did not have any difficulty performing the tasks. When accuracy was analyzed with an omnibus 3 × 5 ANOVA with Task (Phonological, Semantic, Visual) and TMS (40/80, 80/120, 120/160, 160/200, 200/240) as independent factors, it revealed a significant main effect of Task [F(2, 63) = 30.4, p < 0.001] indicating that the semantic task (83%) was significantly more difficult than either the phonological task (90%) or the visual task (91%). Neither the main effect of TMS nor its interaction with Task were significant (both F < 1). In other words, there was no evidence that TMS affected accuracy in performing any of the three tasks.
The RT results are shown in Figure 3. From the figure, it is apparent that there was a main effect of Task [F(2, 62) = 98, p < 0.001], with slowest responses on the semantic task (893 ms), followed by the phonological task (803 ms) and then the visual task (665 ms), each of which was significant different from the others (all p < 0.001, after Bonferroni correction for multiple comparisons). Neither the main effect of TMS [F(4, 124) = 1.2, p = 0.31] nor the Task × TMS interaction reached significance [F(8, 248) = 1.26, p = 0.27] in the omnibus ANOVA. Even so, a set of planned comparisons were performed to specifically evaluate whether TMS modified RTs in the phonological and/or semantic task.
Figure 3. Reaction times (RTs) from the onset of the visual stimulus for each of the five stimulation timings for all three tasks in the main experiment. Note the scales of the y-axes are not identical due to different RTs across the three tasks with visual < phonological < semantic. The solid line represents the baseline RTs. Error bars reflect standard error of the mean adjusted to correctly reflect the variance in the within-subject design (Loftus and Masson, 1994).
For the phonological task, a comparison of each time condition to the baseline condition (40/80 ms) indicated inhibitory effects at all four time windows relative to baseline (plotted in Figure 4). We observed RT increases of 30, 30, 25, and 21 ms, although only the first three were significant [80/120: t(31) = 3.9, p = 0.001; 120/160: t(31) = 2.4; p = 0.02; 160/200: t(31) = 2.3, p = 0.03; 200/240: t(31) = 1.6, p = 0.11]. Despite a similar size inhibitory effect, the final time window did not reach statistical significance because of greater inter-subject variability. Specifically, only 20 out of 32 participants were slowed by TMS during the 200/240 time window. In contrast, 26 subjects showed a slowdown in the 80/120 window, 22 subjects in 120/160 window, and 24 subjects in the 160/200 window. In summary, double pulses of TMS delivered to the same site that slowed performance in the rhyme judgment localizer task resulted in significantly longer RTs between 80 and 200 ms post-stimulus onset.
Figure 4. The difference between reaction times for each time window relative to its baseline condition (i.e., the 40/80 time window) is plotted for all three tasks. Dark gray bars represent the phonological task, light gray the semantic task and white the visual control task. *p < 0.05.
In contrast, SMG stimulation had no significant effect on either the semantic or visual judgment task. For the semantic task, there were net slowdowns in each of the time windows relative to the baseline condition (40/80 ms), but none of these were significant [all t(31) < 0.96, p > 0.34]. This was due to considerable inter-subject variability. Specifically, only 18, 15, 19, and 14 participants (out of 32) showed increased RTs in the four respective time windows. For the visual judgment control task, the effects of TMS were variable and none were significant [all t(31) < 1.1, p > 0.3].
To investigate the functional specificity of the slowdowns observed in the phonological test, we compared them to the TMS effects in the semantic and visual tasks. Figure 4 illustrates the difference in RTs between TMS and no-TMS trials per time window. Dark gray, light gray, and white bars show TMS effects for phonological, semantic, and visual tasks, respectively. It is clear from the figure that slowdown in the phonological task was significantly greater than both the semantic [paired t-test: t(31) = 2, p = 0.03] and visual task [t(31) = 3.1, p = 0.002] in the 80/120 time window. In the later time window, however, the phonological TMS effect did not differ statistically from the semantic TMS effect, despite the fact that there were significant slowdowns relative to baseline in the phonological, but not the semantic, task. Relative to the TMS effects in the visual task, TMS produced significantly larger slowdowns in the phonological task in the 120/160 [t(31) = 2.2, p = 0.02] and 160/200 [t(31) = 2.6, p = 0.01] time windows. Finally, there were no significant differences between the TMS effects in the semantic and visual tasks in any time windows [all t(31) < 0.83, p > 0.41].
In the present study TMS was used to investigate functional specificity and timing of phonological processing within the left SMG during reading. There were two main findings. First, the effects of TMS were present for phonological judgments but were not observed for either semantic or visual judgments. Moreover, the effect of TMS was significantly greater for phonological judgments than either semantic or visual judgments in the 80/120 time window. Second, the inhibitory effects of TMS were apparent as early as 80–120 ms following stimulus presentation and were sustained for approximately another 100 ms. Both of these findings are discussed as they pertain to the neural information processing underlying visual word recognition.
The first aim of this study was to investigate the functional specificity of SMG contributions to word recognition. Previous functional imaging studies involving explicit phonological decisions have consistently revealed SMG activation (Petersen et al., 1988; Booth et al., 2004; Seghier et al., 2004; Zevin and McCandliss, 2005; Raizada and Poldrack, 2007; Buchsbaum and D’Esposito, 2008; Yoncheva et al., 2010). Moreover, the region is activated when participants focus on the sounds of words relative to their meaning (Demonet et al., 1994; Price et al., 1997; Mummery et al., 1998; Devlin et al., 2003; McDermott et al., 2003) suggesting that SMG is preferentially engaged by phonological, rather than semantic, processes. Indeed, the current TMS results are consistent with the imaging findings, confirming a causal link between SMG and phonological processing (Hartwigsen et al., 2010a). SMG stimulation increased response latencies in the phonological task but not in the semantic or visual control tasks. Indeed, at the earliest time window (80/120) the effect of TMS on the phonological task (+30 ms) was significantly greater than in the semantic (−1 ms) or the visual (−8 ms) task, suggesting a degree of functional specificity for phonology early in the time course of processing visual words. Moreover, the results imply that the region is not necessary for other types of linguistic processing such as visual word recognition or semantic processing, nor for more domain-general processes such as sustained attention, decision making, action selection, and initiation, etc. A previous study, however, found a different pattern of results where SMG stimulation affected both phonological and semantic processing (Stoeckel et al., 2009). We are cautious about these previous findings for three reasons. First, Stoeckel et al. (2009) reported that TMS facilitated, rather than inhibited, response times – an effect that has no clear physiological basis (Walsh and Pascual-Leone, 2003; Devlin and Watkins, 2007). Second, this facilitation was only present following single pulse stimulation; trains of repetitive TMS delivered to the same site inhibited phonological processing (Stoeckel et al., 2009). Finally, their findings stand in contrast to several previous studies (as well as the current results) that demonstrate stimulation of SMG preferentially interferes with phonological processing (Romero et al., 2006; Hartwigsen et al., 2010a; Pattamadilok et al., 2010). As a result, the weight of evidence from TMS seems to support the imaging findings and suggests that SMG provides a necessary contribution to phonological, but not semantic, processing.
Precisely what aspects of phonological processing are being computed in SMG are open to debate. Studies of speech comprehension, for instance, typically do not show supramarginal activation (Hickok and Poeppel, 2007; Rauschecker and Scott, 2009), even though phonology plays a central role in speech perception. Instead, the region seems to be engaged by more demanding phonological tasks such as rhyme (Petersen et al., 1988; Yoncheva et al., 2010), syllable (Price et al., 1997; Devlin et al., 2003), or phoneme judgments (Zevin and McCandliss, 2005; Raizada and Poldrack, 2007). Pattamadilok et al. (2010) hypothesized that this may be because each of these tasks involves a form of covert articulation where the participant monitors their own inner speech. The SMG is anatomically well situated for this role with reciprocal connections linking it to ventral premotor (PMv) cortex and pars opercularis (POp; Catani et al., 2005; Rushworth et al., 2006; Petrides and Pandya, 2009), two regions involved in articulatory motor planning (Price, 2010). These reciprocal connections between PMv/POp and SMG may form a processing loop for acting on reproducible sound patterns that would provide a simple resonance circuit for temporarily storing these patterns (McClelland and Elman, 1986; Botvinick and Plaut, 2006). Indeed, studies of verbal working memory commonly implicate these regions (Paulesu et al., 1993; Buchsbaum and D’Esposito, 2008; Koelsch et al., 2009) and TMS delivered to PMv/POp also disrupts phonological processing (Nixon et al., 2004; Gough et al., 2005; Romero et al., 2006; Hartwigsen et al., 2010b). In other words, SMG may play an integral role in representing and processing representations for phono-articulatory patterns that contribute to “phonological processing.”
It is important to note, however, that phonological processing is only one of several functions that the SMG contributes to. For instance, the region is also involved in making visually guided hand actions (Rushworth et al., 2001; Binkofski et al., 2004; Price, 2010) and in spatially localizing auditory stimuli (Lewald and Ehrenstein, 2001; Renier et al., 2009). In other words, the apparent functional specificity of the SMG for phonological processing is limited to a very restricted context – namely when processing linguistic information.
The second aim of this study was to investigate the temporal dynamics of SMG contributions to each task by disrupting processing at different time intervals during the first 250 ms of stimulus processing. In the phonological task, a TMS-induced inhibitory effect was present from 80/120 ms post-stimulus onset. Although the detailed mechanisms of action on the cerebral cortex remain unknown (Wagner et al., 2009), it is clear that TMS induces ionic currents in a percentage of neurons in all cortical layers within the stimulated area, leading to inhibitory and excitatory currents within local microcircuits (Esser et al., 2005). These can cause spiking of pyramidal neurons that in turn send a volley of spikes to distal, but anatomically connected regions. Affected neurons then enter a brief refractory state, such that the local physiological effect of a single TMS pulse within the stimulated area lasts approximately 10 ms (Esser et al., 2005), although the distal effects may last for tens of milliseconds. Indeed, chronometric TMS experiments have shown functionally distinct effects of TMS for pulses separated by as little as 40 ms (Amassian et al., 1993; Corthout et al., 1999; Juan and Walsh, 2003; Pitcher et al., 2007). Consequently, it is reasonable to assume that the inhibitory effects of 80/120 stimulation did not last beyond 160 ms post-stimulus onset – earlier than expected based on many ERP findings. For instance, Bentin et al. (1999) used ERPs to measure the time course of phonological processing during a rhyme monitoring task. Both written words and pseudowords produced a negative-going potential beginning as early as 290 ms after the onset of the stimulus, consistent with many similar studies showing phonological effects in the 250- to 300-ms time range (Niznikiewicz and Squires, 1996; Newman and Connolly, 2004; Grainger et al., 2006). Other studies have reported even later phonological effects ranging from 350- to 550-ms (Rugg, 1984; Carreiras et al., 2009). In other words, many studies indicate that the time course of phonological processing in word recognition begins roughly 100 ms later than reported here.
One possible explanation for this apparent discrepancy may have to do with the nature of the different methodologies. ERP and MEG signals reflect the aggregate electromagnetic activity of synchronous neuronal firing and as a result may be less sensitive to the earliest processing dynamics within a region before synchrony has time to develop (Schroeder et al., 1998). In contrast, the effect of TMS occurs immediately with the stimulation pulse and can interfere with neuronal activity that contributes to the build up of the ERP/MEG signal (Walsh and Cowey, 2000). As a result, TMS effects tend to precede those seen in ERP/MEG and correspond more closely to the timings seen in intracellular recording studies (Corthout et al., 2000; Duncan et al., 2010; Schuhmann et al., 2012). In other words, despite its poorer temporal resolution (tens of ms as opposed to ms), TMS may provide more precise information regarding the onset of regional neuronal activity.
Another possible explanation for the relatively late ERP recordings is that the ERP components such as the N250 or N400 index processes based on recurrent feedback rather than the initial information passing through the system (Sereno and Rayner, 2003). When reading text, the eyes fixate on a word for an average of 250–300 ms (Just and Carpenter, 1980; Rayner et al., 1996), indicating that lexical processing must be underway well before the next saccade. Indeed, Sereno et al. (1998) found that during reading, early ERP components such as the P1 and N1 are influenced by factors such as lexicality and frequency, demonstrating that higher order properties of the word are accessed as early as 100–200 ms post-stimulus onset (see also Hauk and Pulvermuller, 2004). In other words, there is growing evidence that non-visual properties of a word become available as early as 100–200 ms from the onset of the visual word (Ashby et al., 2009; Wheat et al., 2010; Reichle et al., 2011; Hauk et al., 2012).
In addition to this rapid onset, we observed that the effects of TMS were sustained through the 160/200 ms time windows. In contrast, most previous chronometric TMS studies of visual processing have demonstrated separate early and late effects of stimulation, suggesting temporally distinct feed-forward and feedback phases of processing (e.g., Corthout et al., 1999). In our data, however, TMS to each of the time windows between 80/120 and 160/200 ms significantly slowed responses, suggesting on-going phonological processing, presumably due to dynamic interactions with regions processing other aspects of the word including visual and semantic information (Cao et al., 2008; Carreiras et al., 2009; Frye et al., 2010). Indeed, the same temporal pattern of disruption was observed in a chronometric TMS study of left vOTcortex – a region critically involved in processing the visual forms of words (Duncan et al., 2010). Taken together, the results suggest continuous and simultaneous communication between vOT and SMG occurring between approximately 100 and 200 ms after the presentation of a visual word. This type of interactive processing (as opposed to strictly feed-forward processing) is a fundamental principle of virtually all computationally explicit cognitive accounts of visual word recognition (McClelland and Rumelhart, 1981; Seidenberg and McClelland, 1989; Plaut et al., 1996; Coltheart et al., 2001; Jacobs et al., 2003; Harm and Seidenberg, 2004; Perry et al., 2007) and is increasingly important for neuro-anatomical models of reading as well (Price and Devlin, 2011; Twomey et al., 2011; Wang et al., 2011; Woodhead et al., 2011). In other words, these data are not only consistent with accounts of visual word recognition that suggest parallel processing of orthographic, phonological (and presumably semantic) information over time and their integration as a result of constant regional interaction in order to achieve stable word representations, but they also provide a tentative time frame for this processing (i.e., 80–200 ms), consistent with estimates of the time available based on both eye movement and ERP data (Sereno and Rayner, 2003).
The authors declare that the research was conducted in the absence of any commercial or financial relationships that could be construed as a potential conflict of interest.
Amassian, V. E., Cracco, R. Q., MacCabee, P. J., Cracco, J. B., Rudell, A. P., and Eberle, L. (1993). Unmasking human visual perception with the magnetic coil and its relationship to hemispheric asymmetry. Brain Res. 605, 312–316.
Ashby, J., and Martin, A. E. (2008). Prosodic phonological representations early in visual word recognition. J. Exp. Psychol. Hum. Percept. Perform. 34, 224–236.
Ashby, J., Sanders, L. D., and Kingston, J. (2009). Skilled readers begin processing sub-phonemic features by 80 ms during visual word recognition: evidence from ERPs. Biol. Psychol. 80, 84–94.
Baayen, R. H., and Pipenbrook, R. (1995). Linguistic Data Consortium. The Celex Lexical Database. Philadelphia, PA: University of Pennsylvania.
Bentin, S., Mouchetant-Rostaing, Y., Giard, M. H., Echallier, J. F., and Pernier, J. (1999). ERP manifestations of processing printed words at different psycholinguistic levels: time course and scalp distribution. J. Cogn. Neurosci. 11, 235–260.
Binkofski, F., Buccino, G., Zilles, K., and Fink, G. R. (2004). Supramodal representation of objects and actions in the human inferior temporal and ventral premotor cortex. Cortex 40, 159–161.
Booth, J. R., Burman, D. D., Meyer, J. R., Gitelman, D. R., Parrish, T. B., and Mesulam, M. M. (2004). Development of brain mechanisms for processing orthographic and phonologic representations. J. Cogn. Neurosci. 16, 1234–1249.
Botvinick, M. M., and Plaut, D. C. (2006). Short-term memory for serial order: a recurrent neural network model. Psychol. Rev. 113, 201–233.
Buchsbaum, B. R., and D’Esposito, M. (2008). The search for the phonological store: from loop to convolution. J. Cogn. Neurosci. 20, 762–778.
Cao, F., Bitan, T., and Booth, J. R. (2008). Effective brain connectivity in children with reading difficulties during phonological processing. Brain Lang. 107, 91–101.
Carreiras, M., Perea, M., Vergara, M., and Pollatsek, A. (2009). The time course of orthography and phonology: ERP correlates of masked priming effects in Spanish. Psychophysiology 46, 1113–1122.
Caspers, S., Eickhoff, S. B., Rick, T., Von Kapri, A., Kuhlen, T., Huang, R., Shah, N. J., and Zilles, K. (2011). Probabilistic fibre tract analysis of cytoarchitectonically defined human inferior parietal lobule areas reveals similarities to macaques. Neuroimage 58, 362–380.
Catani, M., Jones, D. K., and Ffytche, D. H. (2005). Perisylvian language networks of the human brain. Ann. Neurol. 57, 8–16.
Coltheart, M., Rastle, K., Perry, C., Langdon, R., and Ziegler, J. (2001). DRC: a dual route cascaded model of visual word recognition and reading aloud. Psychol. Rev. 108, 204–256.
Corthout, E., Uttl, B., Walsh, V., Hallett, M., and Cowey, A. (1999). Timing of activity in early visual cortex as revealed by transcranial magnetic stimulation. Neuroreport 10, 2631–2634.
Corthout, E., Uttl, B., Walsh, V., Hallett, M., and Cowey, A. (2000). Plasticity revealed by transcranial magnetic stimulation of early visual cortex. Neuroreport 11, 1565–1569.
Counter, S. A., Borg, E., and Lofqvist, L. (1991). Acoustic trauma in extracranial magnetic brain stimulation. Electroencephalogr. Clin. Neurophysiol. 78, 173–184.
Demonet, J. F., Price, C., Wise, R., and Frackowiak, R. S. (1994). Differential activation of right and left posterior sylvian regions by semantic and phonological tasks: a positron-emission tomography study in normal human subjects. Neurosci. Lett. 182, 25–28.
Devlin, J. T., Matthews, P. M., and Rushworth, M. F. (2003). Semantic processing in the left inferior prefrontal cortex: a combined functional magnetic resonance imaging and transcranial magnetic stimulation study. J. Cogn. Neurosci. 15, 71–84.
Devlin, J. T., and Poldrack, R. A. (2007). In praise of tedious anatomy. Neuroimage 37, 1033–1041; discussion 1050–1038.
Devlin, J. T., and Watkins, K. E. (2007). Stimulating language: insights from TMS. Brain 130, 610–622.
Duncan, K. J., Pattamadilok, C., and Devlin, J. T. (2010). Investigating occipito-temporal contributions to reading with TMS. J. Cogn. Neurosci. 22, 739–750.
Esser, S. K., Hill, S. L., and Tononi, G. (2005). Modeling the effects of transcranial magnetic stimulation on cortical circuits. J. Neurophysiol. 94, 622–639.
Frye, R. E., Liederman, J., Hasan, K. M., Lincoln, A., Malmberg, B., McLean, J. III, and Papanicolaou, A. (2010). Diffusion tensor quantification of the relations between microstructural and macrostructural indices of white matter and reading. Hum. Brain Mapp. 32, 1220–1235.
Gough, P. M., Nobre, A. C., and Devlin, J. T. (2005). Dissociating linguistic processes in the left inferior frontal cortex with transcranial magnetic stimulation. J. Neurosci. 25, 8010–8016.
Grainger, J., Kiyonaga, K., and Holcomb, P. J. (2006). The time course of orthographic and phonological code activation. Psychol. Sci. 17, 1021–1026.
Hamidi, M., Tononi, G., and Postle, B. R. (2009). Evaluating the role of prefrontal and parietal cortices in memory-guided response with repetitive transcranial magnetic stimulation. Neuropsychologia 47, 295–302.
Harm, M. W., and Seidenberg, M. S. (2004). Computing the meanings of words in reading: cooperative division of labor between visual and phonological processes. Psychol. Rev. 111, 662–720.
Hartwigsen, G., Baumgaertner, A., Price, C. J., Koehnke, M., Ulmer, S., and Siebner, H. R. (2010a). Phonological decisions require both the left and right supramarginal gyri. Proc. Natl. Acad. Sci. U.S.A. 107, 16494–16499.
Hartwigsen, G., Price, C. J., Baumgaertner, A., Geiss, G., Koehnke, M., Ulmer, S., and Siebner, H. R. (2010b). The right posterior inferior frontal gyrus contributes to phonological word decisions in the healthy brain: evidence from dual-site TMS. Neuropsychologia 48, 3155–3163.
Hauk, O., Coutout, C., Holden, A., and Chen, Y. (2012). The time-course of single-word reading: evidence from fast behavioral and brain responses. Neuroimage 60, 1462–1477.
Hauk, O., and Pulvermuller, F. (2004). Effects of word length and frequency on the human event-related potential. Clin. Neurophysiol. 115, 1090–1103.
Hickok, G., and Poeppel, D. (2007). The cortical organization of speech processing. Nat. Rev. Neurosci. 8, 393–402.
Jacobs, A. M., Graf, R., and Kinder, A. (2003). Receiver operating characteristics in the lexical decision task: evidence for a simple signal-detection process simulated by the multiple read-out model. J. Exp. Psychol. Learn Mem. Cogn. 29, 481–488.
Jenkinson, M., and Smith, S. (2001). A global optimisation method for robust affine registration of brain images. Med. Image Anal. 5, 143–156.
Juan, C. H., and Walsh, V. (2003). Feedback to V1: a reverse hierarchy in vision. Exp. Brain Res. 150, 259–263.
Just, M. A., and Carpenter, P. A. (1980). A theory of reading: from eye fixations to comprehension. Psychol. Rev. 87, 329–354.
Khateb, A., Annoni, J. M., Landis, T., Pegna, A. J., Custodi, M. C., Fonteneau, E., Morand, S. M., and Michel, C. M. (1999). Spatio-temporal analysis of electric brain activity during semantic and phonological word processing. Int. J. Psychophysiol. 32, 215–231.
Koelsch, S., Schulze, K., Sammler, D., Fritz, T., Muller, K., and Gruber, O. (2009). Functional architecture of verbal and tonal working memory: an fMRI study. Hum. Brain Mapp. 30, 859–873.
Lewald, J., and Ehrenstein, W. H. (2001). Spatial coordinates of human auditory working memory. Brain Res. Cogn. Brain Res. 12, 153–159.
Loftus, G. R., and Masson, M. E. J. (1994). Using confidence-intervals in within-subject designs. Psychon. Bull. Rev. 1, 476–490.
McClelland, J. L., and Elman, J. L. (1986). The TRACE model of speech perception. Cogn. Psychol. 18, 1–86.
McClelland, J. L., and Rumelhart, D. E. (1981). An interactive activation model of context effects in letter perception: I. An account of basic findings. Psychol. Rev. 88, 375–407.
McDermott, K. B., Petersen, S. E., Watson, J. M., and Ojemann, J. G. (2003). A procedure for identifying regions preferentially activated by attention to semantic and phonological relations using functional magnetic resonance imaging. Neuropsychologia 41, 293–303.
Mummery, C. J., Patterson, K., Hodges, J. R., and Price, C. J. (1998). Functional neuroanatomy of the semantic system: divisible by what? J. Cogn. Neurosci. 10, 766–777.
Newman, R. L., and Connolly, J. F. (2004). Determining the role of phonology in silent reading using event-related brain potentials. Brain Res. Cogn. Brain Res. 21, 94–105.
Nixon, P., Lazarova, J., Hodinott-Hill, I., Gough, P., and Passingham, R. (2004). The inferior frontal gyrus and phonological processing: an investigation using rTMS. J. Cogn. Neurosci. 16, 289–300.
Niznikiewicz, M., and Squires, N. K. (1996). Phonological processing and the role of strategy in silent reading: behavioral and electrophysiological evidence. Brain Lang. 52, 342–364.
Obleser, J., and Kotz, S. A. (2009). Expectancy constraints in degraded speech modulate the language comprehension network. Cereb. Cortex 20, 633–640.
Pammer, K., Hansen, P. C., Kringelbach, M. L., Holliday, I., Barnes, G., Hillebrand, A., Singh, K. D., and Cornelissen, P. L. (2004). Visual word recognition: the first half second. Neuroimage 22, 1819–1825.
Pattamadilok, C., Knierim, I. N., Kawabata Duncan, K. J., and Devlin, J. T. (2010). How does learning to read affect speech perception? J. Neurosci. 30, 8435–8444.
Paulesu, E., Frith, C. D., and Frackowiak, R. S. (1993). The neural correlates of the verbal component of working memory. Nature 362, 342–345.
Perry, C., Ziegler, J. C., and Zorzi, M. (2007). Nested incremental modeling in the development of computational theories: the CDP+ model of reading aloud. Psychol. Rev. 114, 273–315.
Petersen, S. E., Fox, P. T., Posner, M. I., Mintun, M., and Raichle, M. E. (1988). Positron emission tomographic studies of the cortical anatomy of single-word processing. Nature 331, 585–589.
Petrides, M., and Pandya, D. N. (2009). Distinct parietal and temporal pathways to the homologues of Broca’s area in the monkey. PLoS Biol. 7, e1000170. doi:10.1371/journal.pbio.1000170
Pitcher, D., Walsh, V., Yovel, G., and Duchaine, B. (2007). TMS evidence for the involvement of the right occipital face area in early face processing. Curr. Biol. 17, 1568–1573.
Plaut, D. C., McClelland, J. L., Seidenberg, M. S., and Patterson, K. (1996). Understanding normal and impaired word reading: computational principles in quasi-regular domains. Psychol. Rev. 103, 56–115.
Prabhakaran, R., Blumstein, S. E., Myers, E. B., Hutchison, E., and Britton, B. (2006). An event-related fMRI investigation of phonological-lexical competition. Neuropsychologia 44, 2209–2221.
Price, C. J. (2010). The anatomy of language: a review of 100 fMRI studies published in 2009. Ann. N. Y. Acad. Sci. 1191, 62–88.
Price, C. J., and Devlin, J. T. (2011). The interactive account of ventral occipitotemporal contributions to reading. Trends Cogn. Sci. (Regul. Ed.) 15, 246–253.
Price, C. J., and Mechelli, A. (2005). Reading and reading disturbance. Curr. Opin. Neurobiol. 15, 231–238.
Price, C. J., Moore, C. J., Humphreys, G. W., and Wise, R. J. S. (1997). Segregating semantic from phonological processes during reading. J. Cogn. Neurosci. 9, 727–733.
Pugh, K. R., Mencl, W. E., Jenner, A. R., Katz, L., Frost, S. J., Lee, J. R., Shaywitz, S. E., and Shaywitz, B. A. (2001). Neurobiological studies of reading and reading disability. J. Commun. Disord. 34, 479–492.
Raizada, R. D., and Poldrack, R. A. (2007). Selective amplification of stimulus differences during categorical processing of speech. Neuron 56, 726–740.
Rauschecker, J. P., and Scott, S. K. (2009). Maps and streams in the auditory cortex: nonhuman primates illuminate human speech processing. Nat. Neurosci. 12, 718–724.
Rayner, K., Sereno, S. C., and Raney, G. E. (1996). Eye movement control in reading: a comparison of two types of models. J. Exp. Psychol. Hum. Percept. Perform. 22, 1188–1200.
Reichle, E. D., Tokowicz, N., Liu, Y., and Perfetti, C. A. (2011). Testing an assumption of the E-Z reader model of eye-movement control during reading: using event-related potentials to examine the familiarity check. Psychophysiology 48, 993–1003.
Renier, L. A., Anurova, I., De Volder, A. G., Carlson, S., Vanmeter, J., and Rauschecker, J. P. (2009). Multisensory integration of sounds and vibrotactile stimuli in processing streams for “what” and “where”. J. Neurosci. 29, 10950–10960.
Romero, L., Walsh, V., and Papagno, C. (2006). The neural correlates of phonological short-term memory: a repetitive transcranial magnetic stimulation study. J. Cogn. Neurosci. 18, 1147–1155.
Rossi, S., Hallett, M., Rossini, P. M., and Pascual-Leone, A. (2009). Safety, ethical considerations, and application guidelines for the use of transcranial magnetic stimulation in clinical practice and research. Clin. Neurophysiol. 120, 2008–2039.
Rugg, M. D. (1984). Event-related potentials in phonological matching tasks. Brain Lang. 23, 225–240.
Rushworth, M. F., Behrens, T. E., and Johansen-Berg, H. (2006). Connection patterns distinguish 3 regions of human parietal cortex. Cereb. Cortex 16, 1418–1430.
Rushworth, M. F., Krams, M., and Passingham, R. E. (2001). The attentional role of the left parietal cortex: the distinct lateralization and localization of motor attention in the human brain. J. Cogn. Neurosci. 13, 698–710.
Schroeder, C. E., Mehta, A. D., and Givre, S. J. (1998). A spatiotemporal profile of visual system activation revealed by current source density analysis in the awake macaque. Cereb. Cortex 8, 575–592.
Schuhmann, T., Schiller, N. O., Goebel, R., and Sack, A. T. (2012). Speaking of which: dissecting the neurocognitive network of language production in picture naming. Cereb. Cortex. 22, 701–709.
Seghier, M. L., Lazeyras, F., Pegna, A. J., Annoni, J. M., Zimine, I., Mayer, E., Michel, C. M., and Khateb, A. (2004). Variability of fMRI activation during a phonological and semantic language task in healthy subjects. Hum. Brain Mapp. 23, 140–155.
Seidenberg, M. S., and McClelland, J. L. (1989). A distributed, developmental model of word recognition and naming. Psychol. Rev. 96, 523–568.
Sereno, S. C., and Rayner, K. (2003). Measuring word recognition in reading: eye movements and event-related potentials. Trends Cogn. Sci. (Regul. Ed.) 7, 489–493.
Sereno, S. C., Rayner, K., and Posner, M. I. (1998). Establishing a time-line of word recognition: evidence from eye movements and event-related potentials. Neuroreport 9, 2195–2200.
Sharp, D. J., Awad, M., Warren, J. E., Wise, R. J. S., Vigliocco, G., and Scott, S. K. (2010). The neural response to changing semantic and perceptual complexity during language processing. Hum. Brain Mapp. 31, 365–377.
Shaywitz, B. A., Shaywitz, S. E., Pugh, K. R., Mencl, W. E., Fulbright, R. K., Skudlarski, P., Constable, R. T., Marchione, K. E., Fletcher, J. M., Lyon, G. R., and Gore, J. C. (2002). Disruption of posterior brain systems for reading in children with developmental dyslexia. Biol. Psychiatry 52, 101–110.
Stoeckel, C., Gough, P. M., Watkins, K. E., and Devlin, J. T. (2009). Supramarginal gyrus involvement in visual word recognition. Cortex 45, 1091–1096.
Twomey, T., Kawabata Duncan, K. J., Price, C. J., and Devlin, J. T. (2011). Top-down modulation of ventral occipito-temporal responses during visual word recognition. Neuroimage 55, 1242–1251.
Ulrich, R., and Miller, J. (1994). Effects of truncation on reaction time analysis. J. Exp. Psychol. Gen. 123, 34–80.
Von Bonin, G., and Bailey, P. (1947). The Neocortex of Macaca Mulatta. Urbana, IL: University of Illinois Press.
Wagner, T., Rushmore, J., Eden, U., and Valero-Cabre, A. (2009). Biophysical foundations underlying TMS: setting the stage for an effective use of neurostimulation in the cognitive neurosciences. Cortex 45, 1025–1034.
Walsh, V., and Cowey, A. (2000). Transcranial magnetic stimulation and cognitive neuroscience. Nat. Rev. Neurosci. 1, 73–79.
Walsh, V., and Pascual-Leone, A. (2003). Transcranial Magnetic Stimulation. A Neurochronometrics of Mind. London: The MIT Press.
Wang, X., Yang, J., Shu, H., and Zevin, J. D. (2011). Left fusiform BOLD responses are inversely related to word-likeness in a one-back task. Neuroimage 55, 1346–1356.
Wassermann, E. M. (1998). Risk and safety of repetitive transcranial magnetic stimulation: report and suggested guidelines from the International Workshop on the safety of repetitive transcranial magnetic stimulation, June 5-7, 1996. Electroencephalogr. Clin. Neurophysiol. 108, 1–16.
Wheat, K. L., Cornelissen, P. L., Frost, S. J., and Hansen, P. C. (2010). During visual word recognition, phonology is accessed within 100 ms and may be mediated by a speech production code: evidence from magnetoencephalography. J. Neurosci. 30, 5229–5233.
Woodhead, Z. V., Brownsett, S. L., Dhanjal, N. S., Beckmann, C., and Wise, R. J. (2011). The visual word form system in context. J. Neurosci. 31, 193–199.
Yoncheva, Y. N., Zevin, J. D., Maurer, U., and McCandliss, B. D. (2010). Auditory selective attention to speech modulates activity in the visual word form area. Cereb. Cortex 20, 622–632.
Keywords: reading, phonology, semantics, chronometric TMS, inferior parietal lobe
Citation: Sliwinska MW, Khadilkar M, Campbell-Ratcliffe J, Quevenco F and Devlin JT (2012) Early and sustained supramarginal gyrus contributions to phonological processing. Front. Psychology 3:161. doi: 10.3389/fpsyg.2012.00161
Received: 26 January 2012; Accepted: 05 May 2012;
Published online: 28 May 2012.
Edited by:
Jon Andoni Dunabeitia, Basque Center on Cognition, Brain and Language, SpainReviewed by:
Rajeev D. S. Raizada, Cornell University, USACopyright: © 2012 Sliwinska, Khadilkar, Campbell-Ratcliffe, Quevenco and Devlin. This is an open-access article distributed under the terms of the Creative Commons Attribution Non Commercial License, which permits non-commercial use, distribution, and reproduction in other forums, provided the original authors and source are credited.
*Correspondence: Joseph T. Devlin, Cognitive, Perceptual and Brain Sciences, University College London, Gower Street, London WC1E 6BT, UK. e-mail:am9lLmRldmxpbkB1Y2wuYWMudWs=
Disclaimer: All claims expressed in this article are solely those of the authors and do not necessarily represent those of their affiliated organizations, or those of the publisher, the editors and the reviewers. Any product that may be evaluated in this article or claim that may be made by its manufacturer is not guaranteed or endorsed by the publisher.
Research integrity at Frontiers
Learn more about the work of our research integrity team to safeguard the quality of each article we publish.