- 1Departamento de Ciencias de la Vida, Facultad de Ciencias, Universidad de Alcalá, Madrid, Spain
- 2Departamento de Ecología y Genética Forestal, Instituto de Ciencias Forestales (ICIFOR), Instituto Nacional de Investigación y Tecnología Agraria y Alimentaria - Consejo Superior de Investigaciones Científicas (ICIFOR-INIA, CSIC), Madrid, Spain
- 3Instituto Universitario de Investigación en Gestión Forestal Sostenible (iuFOR), Universidad de Valladolid, Palencia, Spain
- 4Departamento de Producción Vegetal y Recursos Forestales, Escuela Técnica Superior de Ingenierías Agrarias (ETSIIAA), Universidad de Valladolid, Palencia, Spain
- 5MARE – Marine and Environmental Sciences Centre, ARNET – Aquatic Research Network, Department of Life Sciences, University of Coimbra, Coimbra, Portugal
- 6Departamento de Genética, Escuela Técnica Superior de Ingeniería Agronómica y de Montes (ETSIAM), Universidad de Córdoba, Córdoba, Spain
- 7Forest Research Centre, Associate Laboratory TERRA, School of Agriculture, University of Lisbon, Lisbon, Portugal
- 8Ingeniería Forestal y Medio Natural, Centro Universitario de Plasencia, Instituto Universitario de Investigación de la Dehesa (INDEHESA), Universidad de Extremadura, Plasencia, Spain
Alders are widely distributed riparian trees in Europe, North Africa and Western Asia. Recently, a strong reduction of alder stands has been detected in Europe due to infection by Phytophthora species (Stramenopila kingdom). This infection causes a disease known as alder dieback, characterized by leaf yellowing, dieback of branches, increased fruit production, and bark necrosis in the collar and basal part of the stem. In the Iberian Peninsula, the drastic alder decline has been confirmed in the Spanish Ulla and Ebro basins, the Portuguese Mondego and Sado basins and the Northern and Western transboundary hydrographic basins of Miño and Sil, Limia, Douro and Tagus. The damaging effects of alder decline require management solutions that promote forest resilience while keeping genetic diversity. Breeding programs involve phenotypic selection of asymptomatic individuals in populations where severe damage is observed, confirmation of tree resistance via inoculation trials under controlled conditions, vegetative propagation of selected trees, further planting and assessment in areas with high disease pressure and different environmental conditions and conservation of germplasm of tolerant genotypes for reforestation. In this way, forest biotechnology provides essential tools for the conservation and sustainable management of forest genetic resources, including material characterization for tolerance, propagation for conservation purposes, and genetic resource traceability, as well as identification and characterization of Phytophthora species. The advancement of biotechnological techniques enables improved monitoring and management of natural resources by studying genetic variability and function through molecular biology methods. In addition, in vitro culture techniques make possible large-scale plant propagation and long-term conservation within breeding programs to preserve selected outstanding genotypes.
Introduction
Alders are deciduous riparian trees distributed mostly in the Mediterranean, temperate and boreal zones of the Northern Hemisphere (Bjelke et al., 2016) up to the Himalayas and the Andes. These trees compose the genus Alnus (Family Betulaceae), represented by more than 40 species. In the Iberian Peninsula, two different species coexist: Alnus glutinosa (L.) Gaertn. (common name: common alder) and Alnus lusitanica Vít, Douda & Mandák (common name: Iberian alder; Vít et al., 2017). Common alder is the most widespread alder species in Europe and Western Asia, playing a significant ecological role as a key component of the riparian vegetation along streams (Claessens et al., 2010; Smeriglio et al., 2022). However, in the Iberian Peninsula, the presence of A. glutinosa is limited to the northeast region. Conversely, A. lusitanica is the most representative and widespread alder species with a large distribution in the northwest region (Gomes Marques et al., 2024a; Martín et al., 2024).
Alders are actinorhizal plants that fix atmospheric nitrogen, therefore contributing very significantly to nitrogen dynamics at the local and landscape scales. In addition, they stabilize streams and riverbanks, functioning as a protective barrier against flooding, preventing waterlogging of crops and surrounding areas, and mitigating the damage caused after periods of widespread rainfall. Also, alders contribute to the rapid colonization of abandoned sites and the maintenance of biodiversity by providing refuge for terrestrial and aquatic organisms (Compton et al., 2003; Wipfli and Musslewhite, 2004; Claessens et al., 2010; Handa et al., 2014). This combination of attributes, along with the provision and cultural ecosystem services that are derived from streams and rivers, makes alder replacement by other species very difficult.
Over the recent decades, different alder species have been severely impacted by decline and mortality events caused by abiotic factors, such as extended periods of drought followed by flooding, as well as biotic factors (Ferreira et al., 2022; Gomes Marques et al., 2022), which accumulate to already existing long-term pressures on rivers (Vörösmarty et al., 2010). Along with global change, an increase in outbreaks of invasive pathogenic fungi and oomycetes has severely affected native plants worldwide (Fisher et al., 2020; Gomes Marques et al., 2024b).
Recently, a strong reduction of alder stands has been detected in Europe due to infection by the Phytophthora alni species complex Brasier & S.A. Kirk (Bjelke et al., 2016). This complex includes a group of pathogens that cause Phytophthora disease of alder, also known as alder dieback, affecting different organs of the aerial part of the tree and roots (Gibbs et al., 1999). Although P. ×alni (Husson et al., 2015) is the most aggressive species within the complex, a possible synergy between Phytophthora species in the damage caused to alders is unknown. The aggressiveness is favored by mild winters and warm, but not too hot, summers (Bjelke et al., 2016; Gomes Marques et al., 2024b; Horta Jung et al., 2024). Also, global environmental changes may promote shifts in the pathogen distribution and impact. Their dispersal occurs primarily along water currents which transport thousands of infective zoospores, thus constituting an important route of dissemination of Phytophthora species. Due to the dendritic structure of river networks, Phytophthora can spread rapidly to new areas, notably downstream (Bjelke et al., 2016). The Phytophthora zoospores usually infect the host through the root system, mainly fine roots, or by wounds at the base of the trunk and ascend through its tissues causing lesions in the cambium (Brasier and Kirk, 2001; Černý and Strnadová, 2012). Thus, alders may be subjected to multiple infections over time due to their proximity to the river and the contact of their tissues with surface runoff. Therefore, changes in phytosanitary state and vigor, as well as the degree of tree survival over time are conditioned by environmental factors, including the concentration of inoculum in the soil, the soil type, the water flow velocity, and the geomorphic position of the tree in relation to water (Gomes Marques et al., 2024b).
The wide distribution of alders, with isolated local populations, has resulted in a high genetic diversity that allows them to respond differently to selection pressures related to stand structure (like canopy composition and density) and edaphic, abiotic and biotic factors. In the same way, it has also resulted in inbreeding and moderate local differentiation, partly associated with the ease of seed dispersal through river channels (Štochlová et al., 2012). Given the local adaptation of alders, the identification/selection of more tolerant or resistant genotypes in distant stretches of the same river should seek to retain the adaptive traits so that they can thrive in different environmental conditions. Even when infected, some alders may look asymptomatic and can remain so for a long time (Elegbede et al., 2010). This would also contribute to broadening the genetic base of resistance, thereby reducing the risk of the pathogen overcoming resistance (Sniezko and Koch, 2017). For these reasons, it is very important to understand and protect the existing diversity and to identify resistant genotypes with as much genetic variation as possible and from suitable sources, so they can be used for riverbank restoration.
In this review, the impacts of alder dieback in the Iberian Peninsula and the potential breeding strategies for alder resistance to Phytophthora are summarized. New approaches to improve the resistance selection process and breeding are also described.
Alder genetic diversity
During the last two decades, several studies have used molecular markers to address the genetic diversity and structure of European alders (Mingeot et al., 2010; De Kort et al., 2014a, De Kort et al., 2014b; Beatty et al., 2015; Cubry et al., 2015; Havrdová et al., 2015; Gryta et al., 2017). Although traditionally considered diploid species, recent studies using cytometry and molecular markers revealed variation in ploidy level (Lepais et al., 2013; Mandák et al., 2016). This way, Mandák et al. (2016) identified three main groups across Europe in what was before considered to be A. glutinosa. In addition to the most common and widely distributed diploid (2n = 2x = 28) A. glutinosa, two tetraploid (2n = 4x = 56) clusters were identified: one in western Balkan Peninsula (A. rohlenae Vít, Douda & Mandák) and the other in the Iberian Peninsula and North Africa (A. lusitanica) (Vít et al., 2017; Gomes Marques et al., 2024a). The proposed post-glacial recolonization of Europe by alders would have taken place from multiple refuges in the north of the Iberian, Apennine and Balkan Peninsulas, composed of diploid populations (A. glutinosa), with no involvement of tetraploid populations, which may have originated later (Comes and Kadereit, 1998; Havrdová et al., 2015; Mandák et al., 2016; Šmíd et al., 2020).
In the Iberian Peninsula, the Iberian alder (A. lusitanica) and the common alder (A. glutinosa) are native tree species occurring in riparian and wetland forest communities. Both species contribute to shaping the characteristics, communities and functioning of stream ecosystems (Table 1). The most representative and widespread alder is the tetraploid A. lusitanica, which is widespread from Morocco, and shows three main genetic groups with a clear geographical distribution in the northern, western and central and southern regions (Figure 1A; Martín et al., 2024), under Atlantic and continental climates. The common alder is present in the Ebro basin and some northeast Cantabrian and Catalonian basins (Figure 1A). The Ebro River represents the northeast limit for the distribution of this species (Sanna et al., 2023; Martín et al., 2024), but also a contact zone between A. lusitanica and A. glutinosa. Even so, to date, there is no evidence of gene flow between these species, as evidenced by the absence of triploid individuals among the samples prospected in this area (Martín et al., 2024). In turn, although rare, in the Balkans, triploid individuals have been identified in the overlapping distribution areas of A. glutinosa and the tetraploid A. rohlenae (Mandák et al., 2016; Šmíd et al., 2020). Based on these findings, future studies might also identify triploids in the area where A. lusitanica and A. glutinosa meet in the Iberian Peninsula.
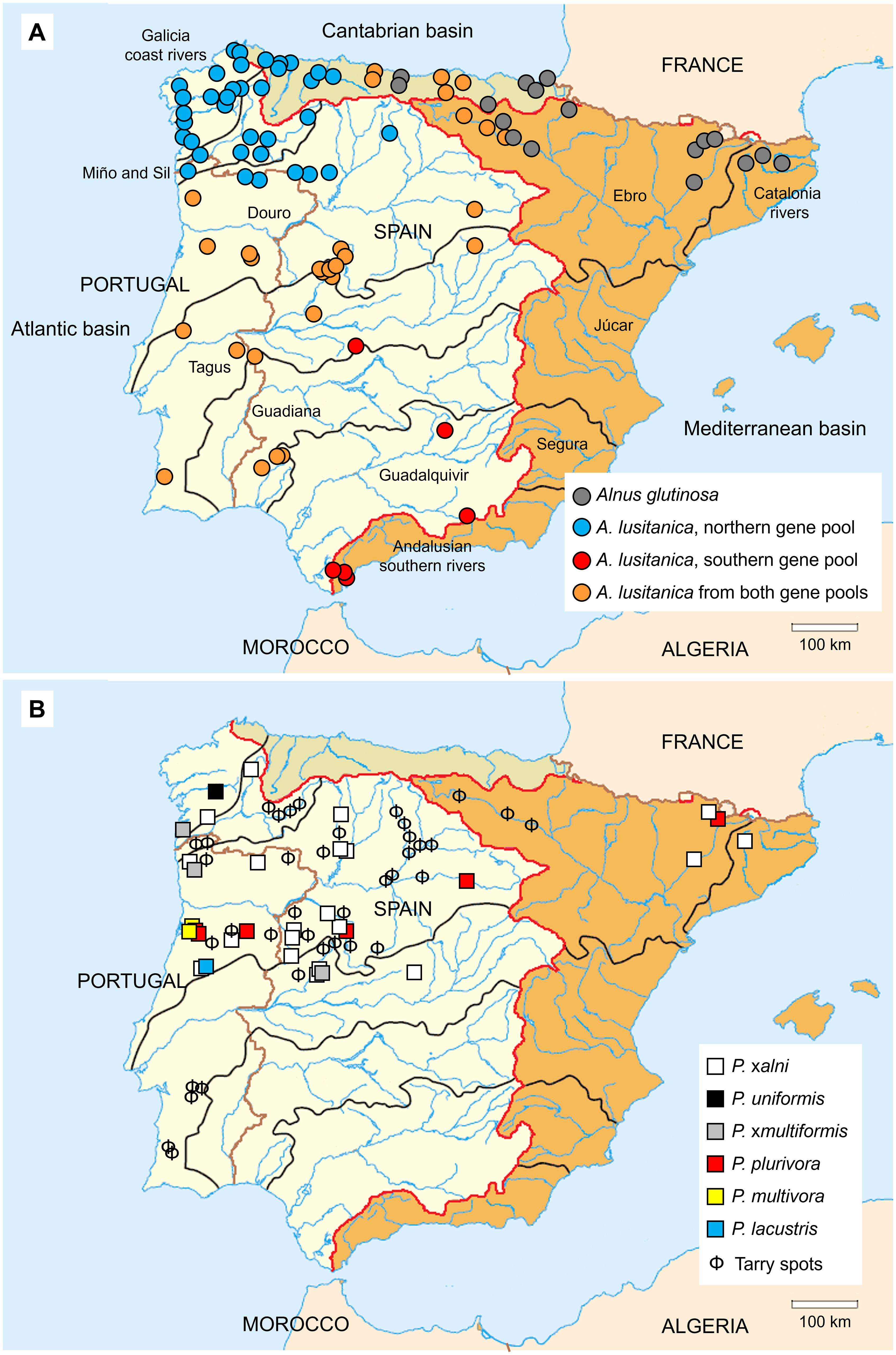
Figure 1. (A) Distribution of Alnus species within the Iberian Peninsula according to ploidy assessment (Havrdová et al., 2015; Vít et al., 2017; Gomes Marques et al., 2022; Sanna et al., 2023) and to genetic structure analyses by using microsatellite markers (Gomes Marques et al., 2024a; Martín et al., 2024). (B) Location of Phytophthora species isolated from unhealthy alder trees (Solla et al., 2010; Pintos-Varela et al., 2010, 2012, 2016; Haque et al., 2014; Jung et al., 2016; Kanoun-Boulé et al., 2016; Ferreira et al., 2022; Bregant et al., 2023; Vieites-Blanco et al., 2023; Gomes Marques et al., 2024b; Gomes Marques, 2024) and stands with characteristic tarry spots on alder trunks from which Phytophthora was not isolated yet. Edges of major river basins are highlighted in red and black, main rivers in blue and country borders in brown.
Ecological importance of Alnus lusitanica and Alnus glutinosa in Iberian riparian and wetland ecosystems
According to the European Habitats Directive 92/43/EEC, alders are considered key components of alluvial forests, which are priority habitats for biodiversity conservation (priority habitat 91E0*). Indeed, alders are trees that live close to water and so have high ecological importance (Table 1).
The extensive root system of alders contributes to defining the morphology of the stream channel, thus creating important habitats for other organisms (Figure 2A). Moreover, woody adventitious roots provide habitat, refuge and feeding grounds for aquatic organisms (Claessens et al., 2010). Their root system also provides an important ecosystem service by contributing to stabilize riverbanks. Alders are also nitrogen-fixing trees owing to their symbiotic association with the nitrogen-fixing bacteria Frankia alni (Voronin) Von Tubeuf in root nodules, which increases soil nitrogen availability (Teklehaimanot and Mmolotsi, 2007; Claessens et al., 2010). As a result, alder trees in the watershed could significantly increase nitrogen levels in streams. This was verified in the USA, where redder alder (A. rubra Bong.) cover in the watershed was linked to higher nitrogen concentrations in stream water (Compton et al., 2003; Shaftel et al., 2012). Increases in soil nitrogen availability in the presence of alder may also increase the nutrient concentrations of leaf litter of non-nitrogen-fixing species (Shainsky and Rose, 1995; Rhoades et al., 2001).
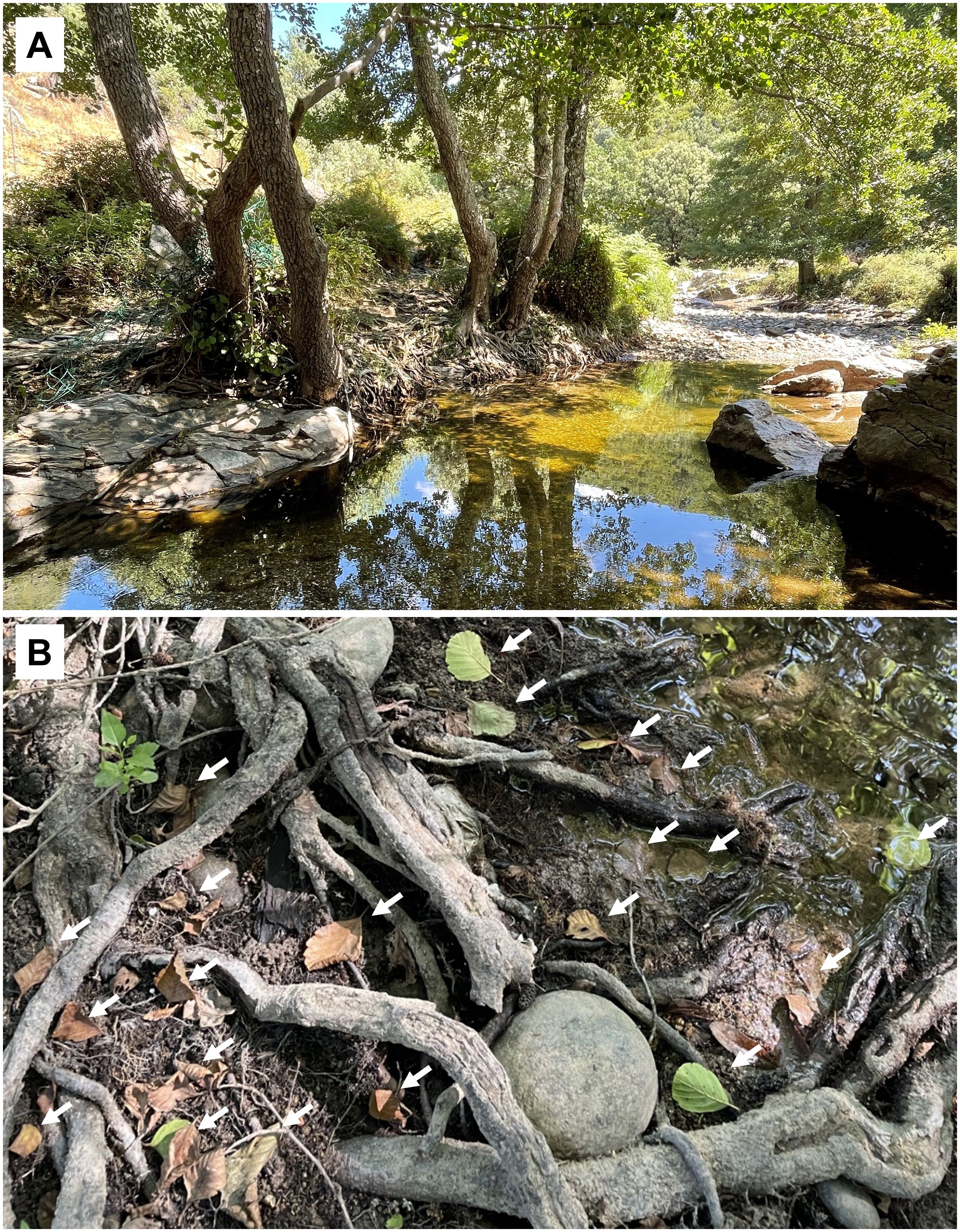
Figure 2. (A) Alnus glutinosa trees provide streambank stabilization. (B) Leaf litter of A. lusitanica, indicated by arrows. Both species show root systems that tolerate submersion, with long tap roots that ensure anchorage during water level decline.
Alder trees do not tolerate shade, making them pioneer species, and the choice to help restore degraded riparian habitats. The shade provided by these trees during summer limits instream primary production while contributes to keeping stream water cool (Bjelke et al., 2016). Furthermore, the alder canopy provides habitat, refuge, food and feeding grounds to terrestrial and aquatic species during their terrestrial life stage, which may provide food inputs to the stream ecosystem when falling into the water (Wipfli, 1997).
Moreover, leaf litter of Iberian and common alders (Figure 2B) is soft and has high nitrogen concentration and low concentration of recalcitrant carbon, in comparison with leaf litter of other Iberian native tree species (Jabiol et al., 2019; Ferreira et al., 2022). This makes it a very palatable food resource for aquatic microbial decomposers and macroinvertebrate detritivores, which prefer alder leaf litter to more recalcitrant one (Graça and Cressa, 2010; Alonso et al., 2021), resulting in its fast decomposition (Feio et al., 2010; Woodward et al., 2012). Especially in autumn, alder leaf litterfall offers an important food supply to aquatic food webs (Pozo et al., 1997), providing a high-quality food resource for the early stages of detritivores aquatic insects (Molinero and Pozo, 2006). In addition, alder leaf litter often stimulates the decomposition of litter mixtures (Alonso et al., 2021), not only because it is a fast-decomposing leaf litter itself (Rubio-Ríos et al., 2023), but also because it stimulates the decomposition of more recalcitrant leaf litter due to the likely reduction in nutrient limitation (Ferreira et al., 2012; Alonso et al., 2024).
Alder Phytophthora species in Spain and Portugal
Riparian forests are particularly vulnerable to pathogen invasions, which spread rapidly along rivers, taking advantage of the current and becoming more effective once they reach stagnant waters (Bjelke et al., 2016). Since the 1990s, the principal pathogens associated with alder disease have been the oomycete of the genus Phytophthora. The first observation of the pathogen infecting alder trees in Europe was in the UK in 1993 (Brasier et al., 1995), and it rapidly spread being quickly detected in a large part of Europe (Brasier et al., 2022). Although the mechanisms behind the rapid spread of the pathogen across Europe are not well understood, it is known that water enhances the pathogen’s sporulation, spread and infection via zoospores (Chen et al., 2022). Furthermore, other authors consider that human activities have significantly contributed to the rapid spread of the disease. For instance, plants already infected by Phytophthora from nurseries may have been used for the reforestation of riparian forests, allowing the introduction of new pathogens (Gibbs et al., 2003; Eschen et al., 2015; Jung et al., 2016; Tremblay et al., 2018; Mora-Sala et al., 2022).
In the Iberian Peninsula, the first reports of outbreaks of Phytophthora associated with alders date from 2009 in Spain and from 2016 in Portugal (Pintos-Varela et al., 2010; Solla et al., 2010; Kanoun-Boulé et al., 2016). The main pathogens causing the disease in alder trees are grouped in the P. alni complex, which includes P. ×alni, P. uniformis and P. ×multiformis, described as species by Husson et al. (2015) (Bjelke et al., 2016; Nave et al., 2021; Trzewik et al., 2021; Bregant et al., 2023). Over the years, new species of Phytophthora (Table 2; Figure 1B) have been detected and isolated from diseased alders presenting symptoms similar to the disease caused by P. alni complex infection (Figure 3). In particular, P. plurivora Jung & Burgess (Jung and Burgess, 2009) has been isolated from bark cankers on several occasions, being the second most isolated species in the Iberian Peninsula (Vieites-Blanco et al., 2023).
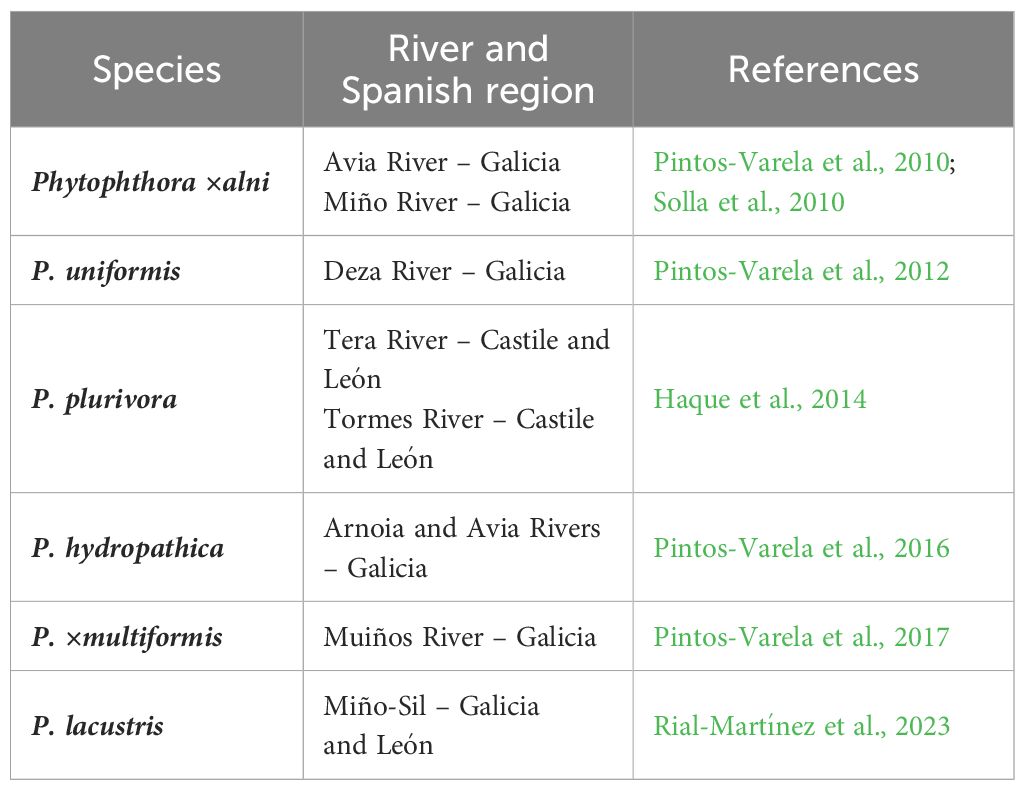
Table 2. First reported distribution of different species of Phytophthora associated with alder in Spain.
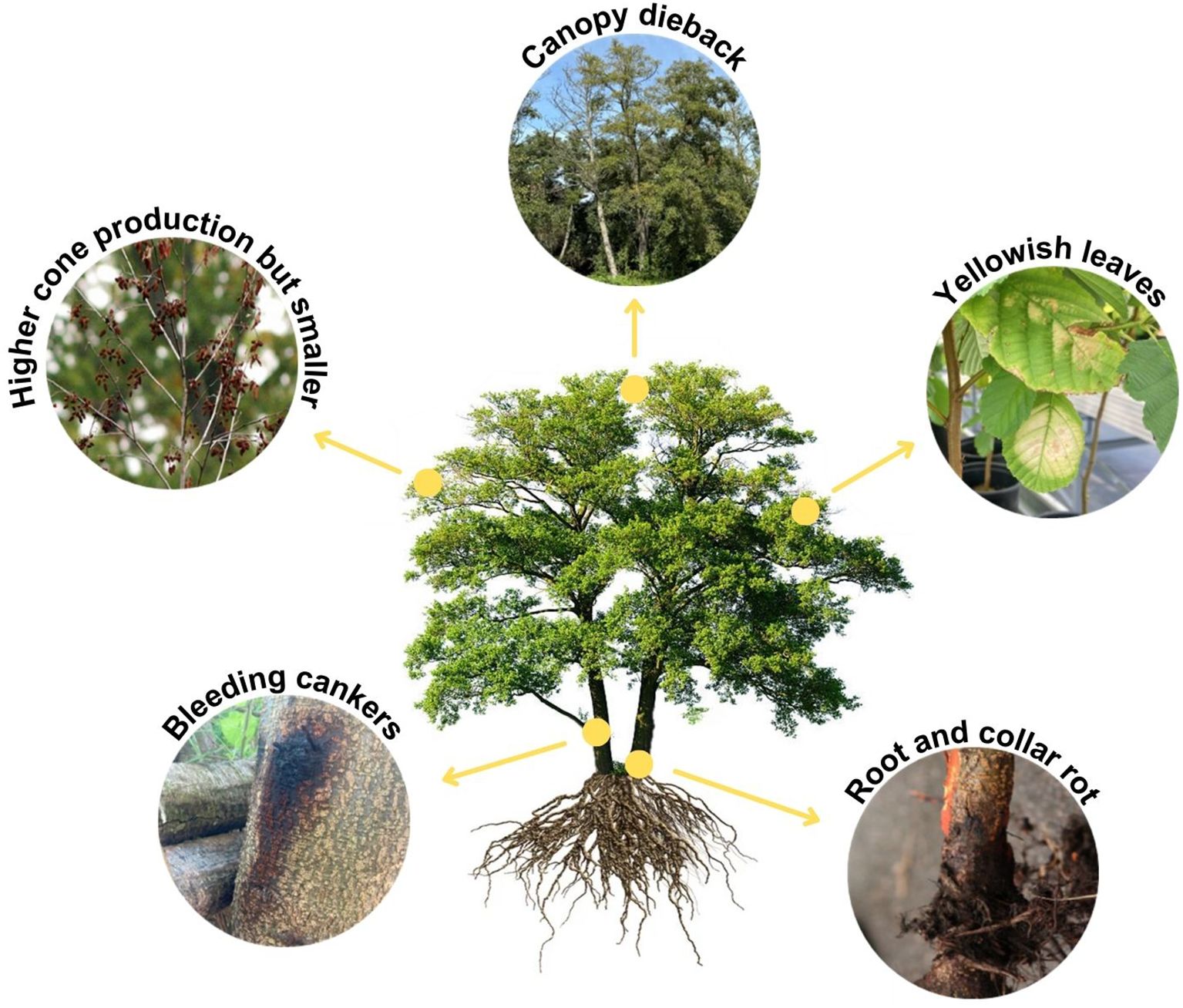
Figure 3. Representative scheme of the main symptoms observed in alder trees infected by different Phytophthora species.
All these Phytophthora taxa (Table 2) generate common symptoms in alders (Figure 3) including small and yellowish leaves, increased cone production but cones smaller than the ones from healthy alders, canopy dieback, growth reduction, bark necrosis, bleeding cankers, exudations in the collar and basal part of the stem, root and collar rot, and tree mortality (Bjelke et al., 2016; Corcobado et al., 2023; Handa et al., 2014; Redondo et al., 2015a). Nonetheless, the pathogenicity of the different Phytophthora species varies. Different methods have been described to study the pathogenicity of Phytophthora species under controlled conditions (Haque et al., 2015; Chandelier et al., 2016). Within the P. alni complex, P. ×alni is considered the most aggressive (Haque et al., 2015). However, studies comparing the pathogenicity between P. plurivora and P. ×alni (the most pathogenic species isolated from alders in the Iberian Peninsula; Jung et al., 2013; Haque et al., 2015; Ferreira et al., 2022; Horta Jung et al., 2024) suggest that the oomycete with the highest pathogenicity on alder is P. plurivora (Zamora-Ballesteros et al., 2017; Corcobado et al., 2023; Vieites-Blanco et al., 2023). This difference in pathogenicity may depend on many factors, such as the alder species and their defense mechanisms, the environmental factors of different riparian forests and the isolates of the Phytophthora pathogen tested and their infection capacity. It is important to note that the higher pathogenicity of P. plurivora compared to P. ×alni may be due to two factors. First, infection of P. plurivora causes a low response in alder, which implies a low defense against this pathogen. Secondly, P. plurivora colonizes the xylem and phloem, in contrast to P. ×alni which primarily occurs in the phloem. The ability to invade the xylem may provide P. plurivora with a competitive advantage over P. ×alni (Vieites-Blanco et al., 2023). Phytophthora lacustris Brasier, Cacciola, Nechwatal, Jung & Bakonyi (Figure 1B, Table 2, Rial-Martínez et al., 2023) and P. hydropathica Hong & Gallegly (Table 2, Pintos-Varela et al., 2016) were also detected in alder trees or river water associated with riparian alder, but their pathogenicity remains to be demonstrated.
Ecological impact of alder disease caused by Phytophthora species in riparian and wetland ecosystems
Given the ecological importance of alders (Table 1), their disappearance from riparian forests, due to Phytophthora occurrence, will likely alter the plant community diversity and structure, and affect the characteristics of streams, because of modified channel morphology, decreased bank stability and increased water temperature (Figure 4A). Root rot caused by the pathogen will result in reduced tree stability, and fallen trees in riverbanks will cause accelerated erosion, affecting river geomorphological conditions and increasing damages caused by flooding in crops and farms (Figure 4B). Also, with alder death, the loss of woody adventitious roots will reduce the instream habitat available to aquatic organisms, and the loss of wide-canopy trees will reduce the habitat availability to terrestrial organisms. Moreover, the disappearance of alder leaf litter from autumn leaf fall will reduce the supply of high-quality organic matter, disrupting the stream detrital pathway (Alonso et al., 2021). Consequently, instream nutrient cycling will be impaired due to the decreased decomposition rate of leaf litter, caused by the loss of high-quality alder leaf litter (Rubio-Ríos et al., 2023). Additionally, the absence of alder will stop contributing to the increase of the quality of leaf litter from non-nitrogen-fixing species (Rhoades et al., 2001), which may decompose slower due to the loss of the stimulatory effect of alder litter presence on the decomposition of other low-quality leaf litter in mixtures (Ferreira et al., 2012; Alonso et al., 2024).
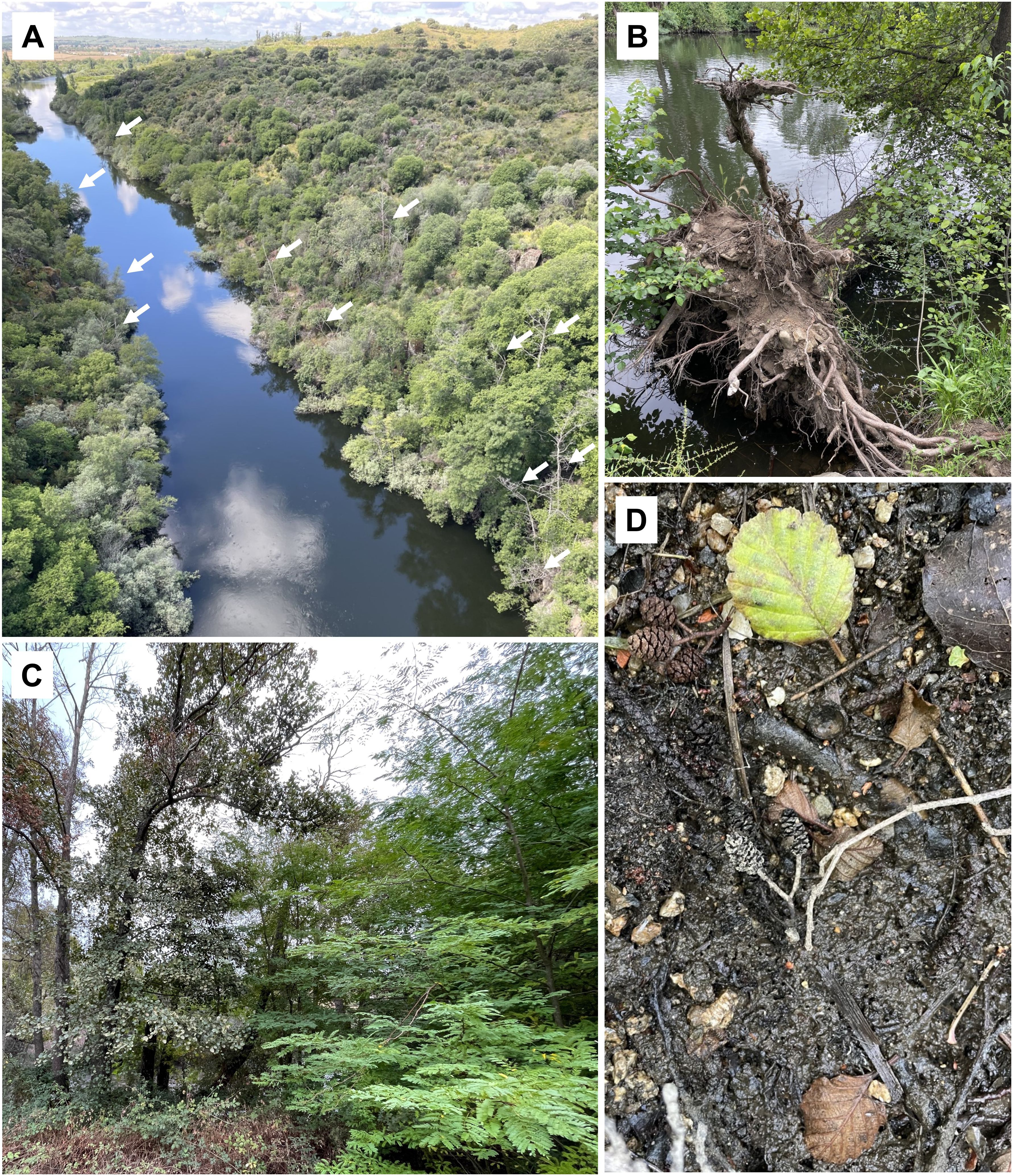
Figure 4. (A) Widespread mortality of Alnus glutinosa trees (white arrows) induced by Phytophthora species along Alagón River (Valdeobispo, Spain). (B) Unhealthy alder trees are prone to fall allowing increased soil erosion. (C) The gap created by tree mortality due to P. ×alni infection will most likely allow rapid colonization of invasive species, such as Gledistia triacanthos tree on the left. (D) Phytophthora-infested soil might not allow seed germination, impeding the successful recruitment of alders; the image shows cones full of viable seeds fallen from alder trees in an infested soil where no regeneration has been registered.
Even before alder trees completely disappear from streamside forests due to the disease, sick alder trees might already be affecting the functioning of the stream ecosystem. For instance, diseased alder trees generally have sparse and small-sized leaves (Jung et al., 2018), and their leaves have lower nitrogen and higher phosphorus concentrations compared with leaves from healthy trees (Ferreira et al., 2022). As aforementioned, these changes will reduce the amount of leaf litter inputs to streams and likely affect the instream cycling of litter-derived nutrients. Microbial-mediated leaf litter decomposition was faster for Iberian alder trees infected with P. alni complex than for healthy trees, probably because of the higher litter phosphorus concentration in diseased trees (Ferreira et al., 2022).
On the other hand, the mortality of alder trees by Phytophthora often generates landscape gaps, which trigger germination and proliferation of some exotic invasive species (Figure 4C), such as Acacia dealbata Link and A. melanoxylon R. Br. in specific areas (Portela-Pereira et al., 2022), leading to biodiversity homogenization. Dieback of mother trees induced by Phytophthora may hamper forest succession by reducing the rate of successful recruitment events, thus compromising the long-term sustainability of the community (Rodríguez-González et al., 2010). Moreover, soil and water infestation by Phytophthora species may impede the successful regeneration of alders, by producing damage to the embryo and radicle of seeds during germination, as reported in other Phytophthora-infested ecosystems (Martín-García et al., 2015; Figure 4D). Thus, direct mortality along with indirect effects on the alder life cycle might lead to non-linear changes in community composition threatening biodiversity hosted by alder-dominated forests (Biurrun et al., 2021).
Resistance and tolerance responses against Phytophthora
Identifying natural resistant genotypes and understanding the underlying mechanisms of resistance are essential for developing effective management and conservation strategies (Redondo et al., 2020). Resistance and tolerance responses of alders to Phytophthora infection are complex and multifaceted, involving a combination of genetic, physiological, biochemical and environmental factors (Gomes Marques et al., 2022; Avila-Quezada and Rai, 2023; Macháčová et al., 2024). Alders have physical barriers as structural defenses, like bark and lignified cell walls (Schmitt et al., 2021). However, Phytophthora can penetrate the host through its fine roots and then spread upwards to the trunk, or directly infect the trunk through existing wounds during flooding (Oßwald et al., 2014). This renders the physical barriers of the alder trees ineffective against infection by Phytophthora.
Alders have also developed defense mechanisms that define their tolerance or resistance and the level of pathogenicity of the oomycete. For example, trees can use tylose production, lignin deposition and/or callose production and deposition around sieve plates to prevent the infection of vascular systems (Redondo et al., 2015a; van den Berg et al., 2018). On alders, Phytophthora was found to affect the epidermis, cortex and vascular cylinder of roots, thus altering fibers and vessels, which leads to a detectable increase in tylose production (Vieites-Blanco et al., 2023). Indeed, some authors relate the presence of tyloses to a defense mechanism to hamper the advance of mycelium (Narayan et al., 2022; Martín and López, 2023). However, Vieites-Blanco et al. (2023) considered that the production of tyloses could be an indicator of damage rather than of plant resistance. In turn, callose formation in the crib plates is associated with plant resistance to Phytophthora. Thus, van den Berg et al. (2018) observed a lack of callose formation on susceptible roots with invasive hyphae, and Vieites-Blanco et al. (2023) detected differences in callose formation in alders infected with less aggressive pathogens vs. absence of callose with the more aggressive pathogens.
A direct relationship has been observed between the geographical distribution of Phytophthora species and subspecies and the climatic conditions. Efforts to create a comprehensive and reliable map of the global spread of various Phytophthora species have revealed that their distribution is related with the abiotic conditions of the studied areas. For example, in southern Sweden, a temperature-related barrier separates the survival of two species: P. uniformis, which can withstand lower temperatures, and P. ×alni which is only found in southern regions with milder climatic conditions (Redondo et al., 2015b, Redondo et al., 2020; Teshome et al., 2020).
In addition, biological barriers, such as microbial communities, are very important in enhancing plant defenses. Forest trees maintain close relationships with a wide variety of microorganisms that are essential for maintaining tree health, optimizing nutrient availability and supporting overall ecosystem functions (Fuller et al., 2023). Compared to crops planted yearly, the microbes that live around trees, both helpful and harmful, usually have more steady and stable interactions. This stability is largely due to the deep root systems of trees, which create a more stable environment for microbial communities (Mercado-Blanco et al., 2018). Beneficial microbiota, including plant growth-promoting rhizobacteria (PGPR), plant growth-promoting fungi (PGPF) and biocontrol agents, can significantly influence the metabolic processes of alder trees. These microorganisms enhance growth, improve performance and increase the trees’ resistance to various stresses in an economically efficient manner (Tian et al., 2020). Furthermore, in the search for resistant or tolerant genotypes to the pathogen Phytophthora, it was observed that progenies of alders from an area invaded by P. uniformis were less susceptible to P. ×alni than progenies from a pathogen-free zone. This could suggest an epigenetic regulation in some of the mechanisms of alder resistance to Phytophthora, as recently described in citrus (Rodrigues da Silva et al., 2021). Also, these studies provide valuable information, as they confirm that responses to infection can be inherited and highlight the importance of studying both surviving and healthy trees (not just the diseased ones), since they provide adaptation potential to the future generation. However, they also stress the need to study the stability of these responses over time and generations.
Through research, several potential biological control agents (BCAs) have been identified to reduce pathogenic spread and associated symptoms including dieback and root rot. The most commonly used BCAs belong to the genera Pseudomonas, Bacillus and Trichoderma. For instance, a study by Zaspel et al. (2014) demonstrated the advantages of root treatment with Pseudomonas veronii Coroler, Elomari, Hoste, Gillis, Izard, Leclerc for enhancing alder rooting. The study found that this bacterium can induce tolerance in some P. ×alni-infected alders, allowing them to survive without exhibiting symptoms caused by the pathogen. Moreover, while studies of BCAs on alder are limited, there are many registered microorganisms with antagonistic activity on other Phytophthora pathogens whose benefits have been successfully demonstrated. For example, Pseudomonas putida (Trevisan) Migula and P. chlororaphis (Guignard and Sauvageau, 1894; Bergey et al., 1930) were used as BCA of Phytophthora root rot in citrus orchids (Steddom et al., 2002) and in P. palmivora E.J. Butler infected-cacao plants, respectively (Acebo-Guerrero et al., 2015). In the same way, Bacillus amyloliquefaciens (Fukomoto) Priest, Goodfellow, Shute & Berkeley induced systemic resistance against P. cactorum (Lebert & Cohn) J. Schroüt (Lee et al., 2015). In addition, Trichoderma virens (J.H. Miller, Giddens & A.A. Foster) Arx, T. harzianum Rifai, T. asperellum Samuels, Lieckfeldt & Nirenberg and T. spirale Bissett showed antagonistic effects against P. palmivora in cacao plants (Mpika et al., 2009). T. saturnisporum Hammill also showed antagonistic effects against several Phytophthora spp (Diánez Martínez et al., 2016; Mercado-Blanco et al., 2018). These findings suggest a promising avenue for biocontrol against Phytophthora species, underscoring the importance of investigating the alder microbiome. Such research aims to identify potential microorganisms capable of inhibiting or parasitizing the pathogen decreasing its pathogenicity, thereby enhancing the host chances of survival and resilience (Redondo et al., 2020).
Breeding programs and approaches to fight against diseases and pests in forest tree species that may guide research on alder
Forest tree breeding is a laborious and time-consuming process, strongly limited by the long breeding cycle of most tree species. An important factor in maintaining the long-term viability of the alder populations in Europe is the development or maintenance of natural resistance to Phytophthora. Additionally, the presence of asymptomatic alders surviving in highly affected areas could suggest the potential resistance to alder dieback in some common alder genotypes (Jung and Blaschke, 2004). Traditional approaches for breeding are based on the selection and mating of elite trees carrying the desirable traits. It is crucial to identify asymptomatic genotypes, which may be resistant, within natural populations affected by the pathogen. These genotypes should be tested for resistance under controlled conditions, typically using a combination of short- and long-term assessments. This includes planting the selected genotypes in areas with high disease pressure and varying environmental conditions (Sniezko, 2006; Sniezko and Koch, 2017). The confirmed resistant trees can then be used to develop a pool of resistant germplasm, for reforestation or restoration purposes, by clonal propagation and/or by crossing with other resistant trees (Keriö et al., 2019).
Forest biotechnology provides tools for conserving and sustainably managing of natural genetic resources, as well as for optimizing and speeding up genetic improvement programs. The development and use of biotechnological techniques provide, on the one hand, information for better management of natural resources, through the study of the genetic variability of forest tree resources and their functional characterization, employing molecular biology techniques (Díaz-Sala, 2014, 2019). On the other hand, in vitro culture techniques make possible large-scale plant propagation within breeding programs to, among others, preserve selected outstanding genotypes that are difficult to conserve by other methods. The application of tissue culture techniques has also led to the development of cryopreservation, as an additional strategy for conservation widely used in the agricultural sector. Vegetative propagation and in vitro propagation allow the development of clonal trials for phenotypic evaluation, the multiplication and maintenance of the genotypes of interest, the regeneration of a high number of plants in breeding programs and guarantee the health and availability of forest material quickly at any time and season of the year (Díaz-Sala, 2016).
Large-scale vegetative propagation
Alders are easily propagated by seeds, and this is the most used method when a high number of specimens is required. However, due to the variation resulting from sexual reproduction, the use of seeds as a means of propagation to produce plants expressing a desirable trait, such as disease resistance, is limited, and vegetative propagation methods would be more appropriate. An alternative to sexual reproduction, for capturing genetic gains, is the vegetative propagation of trees that show desirable traits. Vegetative propagation also allows the preservation of non-additive gene effects, which result from gene interactions. These effects are usually not passed on through sexual reproduction but can produce exceptional individuals. In horticulture, mass vegetative propagation of selected phenotypes has been used for centuries. However, woody species have specific characteristics that make mass propagation not widely used in forestry, despite the need to propagate elite genotypes by these methods (Greenwood and Weir, 1995). The high heterozygosity of forest species, combined with the significant non-additive genetic effects influencing various traits of interest, requires the use of vegetative propagation to achieve optimal genetic gains while preserving the genotype’s identity and biodiversity.
Alders can be propagated vegetatively by rooting woody cuttings. However, rooting success is highly dependent on the genotype, tree age, collection season, type of cuttings and treatments used. Even though, annual softwood cuttings were found more appropriate for rooting and vegetative propagation of mature common alder trees (Radwan et al., 1989; Novotná and Štochlová, 2012). Also, cuttings collected during the breakdown of endogenous dormancy of the mother plant (December to February), or before the onset of dormancy (July to September), seem to be more successful for rooting of A. glutinosa (Novotná and Štochlová, 2012).
Alders can also be propagated by using in vitro tissue culture techniques (Tremblay and Lalonde, 1984; Périnet and Tremblay, 1987; Corredoira et al., 2011, Corredoira et al., 2013; Bajji et al., 2013; San José et al., 2013). Indeed, in vitro propagation and conservation of alders have been carried out by somatic embryogenesis and subsequent cryopreservation of induced somatic embryos (Corredoira et al., 2013; San José et al., 2015b). However, at present, the establishment of axillary micropropagation systems, by sequential subcultures and subsequent rooting of the shoots obtained, is the most widely used method, as it guarantees a rapid large-scale multiplication and genetic stability (Tremblay and Lalonde, 1984; Périnet and Tremblay, 1987; Corredoira et al., 2011; San José et al., 2012, San José et al., 2013). However, the effect of the genotype, the tree age and phytosanitary conditions, the stage of development and the status of the explant material are major factors affecting culture establishment (Tremblay and Lalonde, 1984; Périnet and Tremblay, 1987; Bajji et al., 2013; San José et al., 2013). Remarkably, the use of explants from forced-to-flush axillary shoots, from mature branches under controlled conditions, avoids the huge contamination problems when explants taken directly from the field are used for the initiation of in vitro cultures (Corredoira et al., 2011; San José et al., 2013). A specific culture medium for woody species, supplemented with cytokinins and auxins, is required for the successful establishment and multiplication of juvenile and adult tree explants by shoot cultures (Bajji et al., 2013; San José et al., 2013). In addition, the carbohydrate source seems to play an important role in both multiplication and rooting phases of shoots (San José et al., 2011, San José et al., 2013). In the presence of exogenous auxin, primarily indole-3-butyric acid, or even in its absence, alder induces adventitious roots in stems developed in vitro (Corredoira et al., 2011; San José et al., 2012, San José et al., 2013). Recently, the use of in vitro systems of temporary immersion in a liquid medium has been described as an alternative strategy for the improvement of alder multiplication (San José et al., 2020). In the same way, the use of double-phase culture systems, by adding liquid medium (Rodriguez et al., 1991), improves the multiplication rates and plant vigor, while reducing the management costs. The storage of A. glutinosa shoot cultures under minimum growth conditions allows maintaining cultures for extended periods (up to 18 months before subculturing), which results in a cost-efficient storage of alder germplasm, thus contributing to improved conservation of alder genetic diversity (San José et al., 2015a).
Recent advances to improve the efficiency of tree breeding
Although forest tree breeding usually lasts for decades, recent advancements in methods and strategies have introduced tools to accelerate and refine this process. Genomic Selection (GS), Marker-Assisted Selection (MAS), Genome-Wide Association Studies (GWAS) and Quantitative Trait Loci (QTL) have revolutionized tree breeding by enabling the precise identification and manipulation of genes associated with resistance to pests and diseases (Fan et al., 2024; Fernandes et al., 2024; Jacobs et al., 2024; Sharma et al., 2024). These approaches have great potential to augment and help advance tree improvement programs, through early, indirect selection of improved genotypes (Sniezko and Koch, 2017). Moreover, technologies such as the CRISPR/Cas9 genome-editing tool allow for precise alterations in tree genomes, opening the door for obtaining individuals with specific resistances to pests and diseases. This technology, combined with GS and MAS, could help to accelerate breeding cycles and improve the genetic gain per generation (Poovaiah et al., 2021). In addition, modern High-Throughput Phenotyping (HTP) technologies, like cameras, sensors, Unmanned Aerial Vehicles (UAV), robotics and computers, allow the collection of reliable phenotypic data of thousands of individuals with unprecedented speed and accuracy, i.e. automated phenotyping (aka phenomics) (Spalding and Miller, 2013). For instance, these technologies (e.g. UAV) might be used to identify volatiles or any other chemical signal linked with resistant phenotypes (Quintana-Rodríguez et al., 2015) and/or with the maturity stage of the pest (Mamidala et al., 2013; Shen et al., 2021). For instance, UAVs were used to monitor the health status of trees affected by alder dieback in Northern Portugal (Guerra-Hernández et al., 2021). Recent advances in Artificial Intelligence may also leverage the linking of phenotypes to genomic features (Rairdin et al., 2022), which is particularly challenging in the current big data era in plant biology (Deng et al., 2023), as well as the phenotype/disease identification/diagnosis (Ferentinos, 2018; Wang et al., 2022) and the GS prediction models (Sharma et al., 2024).
Case studies in breeding against forest pests and diseases
The Emerald Ash Borer (EAB, Agrilus planipennis Fairmaire) case, a prime example of an invasive pest introduced through globalization, is an example of how multiple biotechnological approaches to genetic breeding can help in disease control in forest species. Native to Northeast Asia, EAB was first found in the USA in 2002 and, since then, it has devastated ash populations (Fraxinus spp.), including green ash (F. pennsylvanica Marsh), and forested ecosystems, with severe economic and ecological impacts (Herms and McCullough, 2014). Thus, EAB is the most damaging invasive forest insect pest ever to have invaded North America, threatening nearly all native species of Fraxinus with functional extinction (Poland and McCullough, 2006; Herms and McCullough, 2014; Aubin et al., 2015; Stanley et al., 2023). Breeding programs have focused on identifying and propagating EAB-resistant ash trees. Research has identified specific genetic markers associated with resistance, enabling the use of MAS to accelerate breeding efforts (Cobo-Simón et al., 2021; Huff et al., 2022). In addition, ongoing genomic studies aim to understand the mechanisms of resistance and enhance the resilience of ash populations (Huff et al., 2022; Battisti and Larsson, 2023). However, further knowledge of the ash genome is of vital importance to understanding the genetic basis of ash resistance. In this sense, the recently published genomes of F. excelsior L. and F. pennsylvanica can be very useful (Sollars et al., 2017; Huff et al., 2022). Another approach has optimized the Agrobacterium-mediated transformation system to introduce a Bt toxin gene into transgenic F. nigra Marshall susceptible shoots (Lee and Pijut, 2018). From another perspective, Merkle et al. (2023) reported the application of somatic embryogenesis to clonally propagate progeny of lingering F. americana L. and F. pennsylvanica parents, which could provide EAB-resistant ash varieties for forest and urban tree restoration. Moreover, transcriptome and proteomic analyses also provide information and powerful tools to advance pedigree-based breeding and selection programs as well as the management of standing populations (Neale and Kremer, 2011). Recently, Chiu et al. (2023) identified a unique set of genes linked to three different levels of increasing EAB infestation of F. pennsylvanica. Moreover, studies on resistance variability and candidate genes for ash tree stress defense can help breeding for resistance to EAB (Lane et al., 2016; Kelly et al., 2020). Although there is still much progress to be made in the fight against EAB, the combined use of several biotechnological approaches could offer new hope for the survival of North American Fraxinus species.
Several other case studies highlight the success of modern forest breeding programs. For instance, breeding programs for Norway spruce (Picea abies [L.] H. Karst) and various pine species (Pinus spp.) have shown significant improvements in growth and resistance traits using GS techniques (Sharma et al., 2024). These programs use genetic data to predict and select the best candidates for breeding, enhancing resistance to common pests and diseases such as bark beetles (Westbrook et al., 2013), whose impacts are magnified by climate change. These efforts have resulted in more resilient tree populations capable of withstanding pest invasions and environmental stresses (Lenz et al., 2020; Sharma et al., 2024).
American chestnut (Castanea dentata (Marshall) Borkh.) was nearly eradicated by chestnut blight (Cryphonectria parasitica (Murrill) Barr). Breeding programs have aimed to restore this iconic species through hybridization with blight-resistant Chinese chestnut (C. mollissima Blume). American chestnut is also highly susceptible to the soil-borne pathogen P. cinnamomi Rands, which causes root rot. This pathogen is spreading towards northern regions of North America due to climate change. Breeding programs aim to combine resistance to chestnut blight and Phytophthora, by breeding blight-resistant hybrids with P. cinnamon-resistant American chestnuts. Recent advances in GS,CRISPR/Cas9 technology (Westbrook et al., 2019a, Westbrook et al., 2019b; Fernandes et al., 2022; Battisti and Larsson, 2023) and transcriptomics, particularly comparative transcriptome analysis (Barakat et al., 2009, Barakat et al., 2012; Nie et al., 2023), have furthered these efforts, leading to crucial findings to assist successful breeding and offering hope for the re-establishment of American chestnut populations.
In European chestnut species, breeding for resistance to ink disease, caused by P. cinnamomi, allowed for the development of tolerant hybrid rootstocks in Europe, by crossing the local C. sativa Mill. with the two Asian tolerant species, C. crenata Sieb. and Zucc. and C. mollissima (Miranda-Fontaíña et al., 2007; Santos et al., 2015, Santos et al., 2017). Furthermore, the selection of P. cinnamomi-tolerant chestnut trees has been evaluated using EST-SSRs, among which the CsPT_0005 locus could be applied in marker-assisted selection to predict P. cinnamomi resistance in non-inoculated C. sativa trees (Alcaide et al., 2020).
In Spain, a breeding program is currently underway to obtain Quercus ilex L. and Q. suber L. varieties tolerant to P. cinnamomi. It includes activities such as the identification of trees in affected areas and selection of symptomless individuals, population variability studies, propagation of symptomless specimens identified in affected areas, evaluation of the suitability of tolerant material as rootstocks, tolerance trials “in vitro”, in the nursery and the field, installation of seed orchards of selected clonal materials, search for molecular markers linked to resistance/tolerance responses, and studies of the biological component of the soil (Tapias et al., 2006, Tapias et al., 2008; Pérez et al., 2020; Martínez et al., 2023).
Genetic improvement has also proven to be effective as a control tool against the pine wood nematode (Bursaphelenchus xylophilus (Steiner and Buhrer) Nickle). Designed to combat this pathogen, the genetic breeding program from the Forestry Research Center of Lourizán (Spain) has successfully developed resistant varieties of Pinus pinaster Aiton, that are now offered for sale in nurseries (Díaz-Vázquez et al., 2020). For this purpose, researchers studied variation in susceptibility to the nematode across several pine species, as well as among P. pinaster provenances from the Iberian Peninsula and France, and among half-sib families within the P. pinaster and P. radiata D. Don genetic improvement programs (Díaz-Vázquez et al., 2020; Menéndez-Gutiérrez et al., 2018, Menéndez-Gutiérrez et al., 2021).
The breeding program for Chamaecyparis lawsoniana (A. Murray bis) Parl. (Port-Orford cedar or Lawson’s cypress) resistance to P. lateralis Tucker & Milbrath, in the USA, is one of the most promising resistance selection efforts for forest trees around the world (Keriö et al., 2019). P. lateralis nearly decimated C. lawsoniana, a keystone tree species in the Pacific Northwest and one of the most valued landscape tree species in the Northern Hemisphere. Currently, containerized seed orchards with the best parents and progeny are maintained for resistance testing. Seed production and field plantings indicate high survival rates of the resistant planting stock (Hansen et al., 2012).
Unfortunately, there are relatively few examples of tree breeding programs focused on Phytophthora resistance in wild forest species, compared to those with higher economic significance, such as crops. In contrast, agricultural species have benefited from genomic techniques such as QTL mapping, GWAS, and genome-wide extreme phenotyping (XP-GWAS). These approaches have been instrumental in identifying significant QTLs and single nucleotide polymorphisms (SNPs) associated with resistance to Phytophthora (Siviero et al., 2006; Siddique et al., 2019; de Ronne et al., 2022; Ro et al., 2022; Li et al., 2023; Lin et al., 2023). For example, underway breeding programs have allowed obtaining citrus rootstocks resistant to P. nicotianae Breda de Hann, and the use of molecular technologies, linkage maps and QTL information has improved the efficiency of various citrus breeding programs, by decreasing evaluation times for a high number of genotypes and providing study targets (Lima et al., 2018).
Since the disease caused by Phytophthora was first detected in European alders in the 1990s, it has resulted in severe losses in timber production, and severe damage to alder stands in forests and along riverbanks with negative effects on flood defense and biodiversity loss. Forest management strategies and fungicides are the usual way of coping with this disease, but the control of the pathogen with chemical treatments outside nurseries is not allowed. Therefore, breeding for resistance can be one of the most effective strategies to control the disease. Furthermore, the need to preserve genetic diversity and the presence of tolerant alders support the need for breeding for resistance. However, until recently, the only known breeding program for common alder resistant to P. ×alni is the RESISTANT ALDER project, conducted at the Institute of Forest Genetics in Waldsieversdorf (Germany). This project ended in 2017, resulting in the selection of only four clones with lower susceptibility to P. ×alni.
In response to the widespread alder mortality in the Iberian Peninsula, comprehensive programs have recently been launched to address the alder decline in Spanish and Portuguese riparian forest ecosystems. The ALNUS project in Portugal (2018-2022; https://www.isa.ulisboa.pt/proj/alnus/project/) launched the study of alder populations’ resilience to Phytophthora and climate change. In Spain, the RETAIN (2022-2024) and ATLANTES (2022-2025) projects, involving several research groups from different universities and research institutes, with the collaboration of public bodies in charge of alder management (Ministry for Ecological Transition and the Demographic Challenge, River Basin Authority and Regional Government of Castile-La Mancha), initiated an ambitious regional and national program to cope with alder decline in Spanish riparian forest ecosystems (Figure 5). This program involves, on one side, the determination of the current distribution and damage of alder dieback, as well as the characterization of the Phytophthora species complex causing the disease, to improve our understanding of host-pathogen interactions. On the other side, it plans to carry out an in-depth characterization of the existing genetic variability of alders from the Iberian Peninsula and the interactions in the Alnus-Phytophthora pathosystem, along with the identification and large-scale propagation of resistant or less susceptible trees while maintaining biodiversity. The genetic and ecological characterization of an admixture of species in an Alnus stand, as well as of their offspring in response to abiotic and biotic factors are also included in the program. This information will allow, when planning a restoration program, to consider any genetic structure at a local scale and differentiation at a river catchment (Rodríguez-González et al., 2019), provenance region or national scale. Maintaining genetic diversity within forest populations during breeding for resistance is crucial to ensure resilience against future threats (Blows and Hoffmann, 2005; Bijlsma and Loeschcke, 2012; Hamilton et al., 2017; Sjöman et al., 2024). Alimpić et al. (2022) concluded that a combination of in situ and ex situ measures and/or integrative conservation of riparian ecosystems is the most appropriate option for conserving the genetic diversity of riparian tree species. In addition, in the Iberian Peninsula, where two species (A. glutinosa and A. lusitanica), hard to distinguish, coexist, traceability may be of particular interest to river managers.
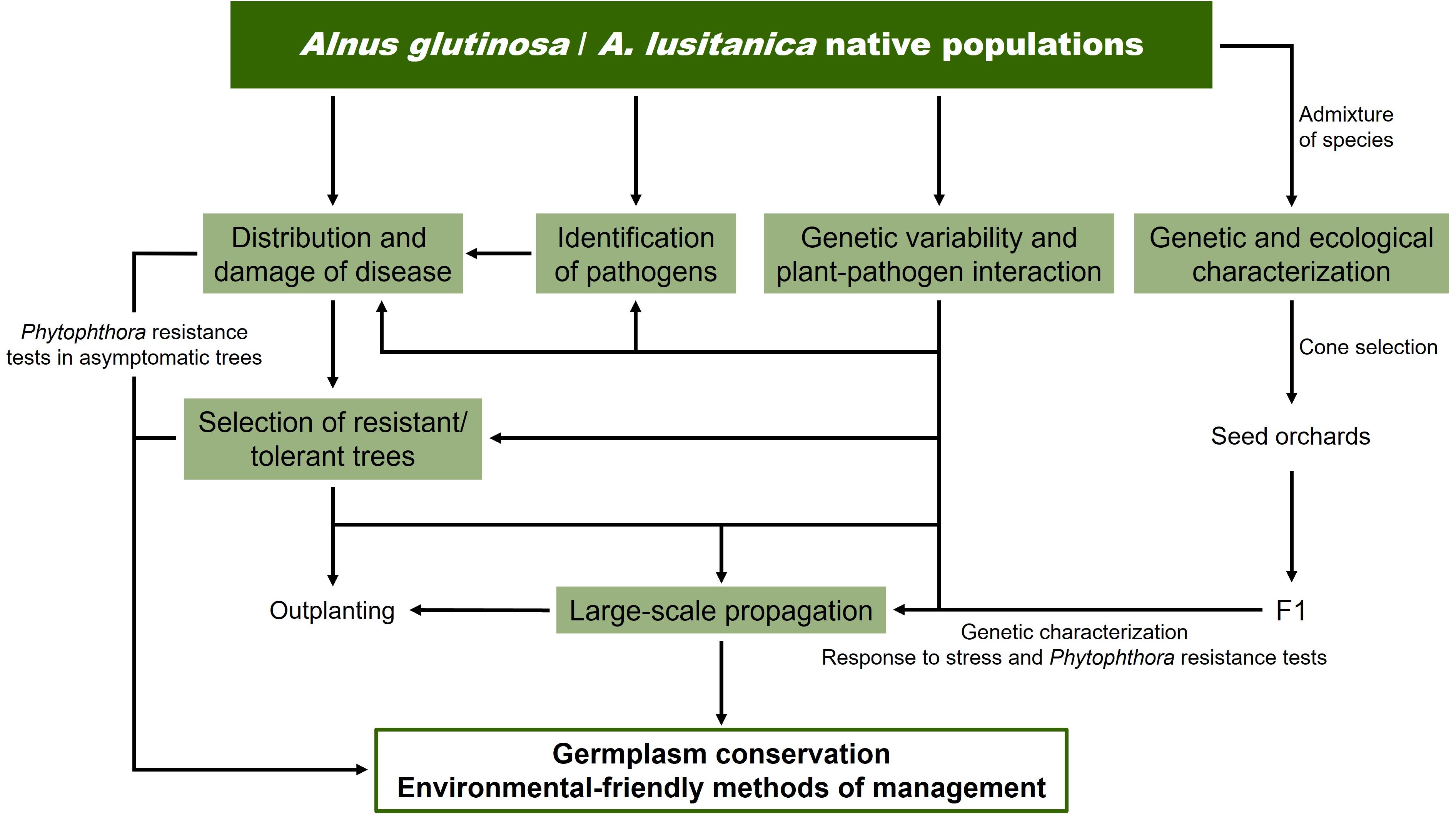
Figure 5. Program launched in RETAIN and ATLANTES projects to tackle alder decline in Spanish riparian forest ecosystems. Information on the program launched in the Portuguese ALNUS project can be found at https://www.isa.ulisboa.pt/proj/alnus/project/.
Conclusions and future perspective
Alders play significant ecological, commercial and recreational roles. However, a strong reduction of alder stands has been detected in Europe due to infection by Phytophthora spp. Continuous tree germplasm screening, effective procedures for the evaluation of the disease, accurate identification of Phytophthora species and identification of tolerant genotypes for large-scale propagation are crucial for the development of breeding programs that will preserve tree genetic diversity. The advances in genetic screening and genomic technologies, reference genomes and bioinformatic tools, including the promising application of Artificial Intelligence techniques in molecular biology (e.g. AlphaFold; Tunyasuvunakool et al., 2021; Abramson et al., 2024), as well as the genomics of Phytophtora-alder interactions and the novel high-throughput phenotyping techniques, will help to identify resistance gene(s), QTLs and pathogen effectors. This knowledge will allow the application of genomic-assisted breeding, gene silencing and gene editing to improve Phytophthora resistance in alder.
Author contributions
DC: Writing – original draft, Writing – review & editing. AP: Writing – original draft, Writing – review & editing. MV: Writing – original draft, Writing – review & editing. MG: Writing – original draft, Writing – review & editing, Conceptualization. NM: Writing – original draft, Writing – review & editing. PR: Writing – original draft, Writing – review & editing. IC-S: Writing – original draft, Writing – review & editing. AD-G: Writing – original draft, Writing – review & editing. AB: Writing – original draft, Writing – review & editing. VF: Writing – original draft, Writing – review & editing, Funding acquisition. MM: Writing – original draft, Writing – review & editing, Funding acquisition. PR-G: Funding acquisition, Writing – original draft, Writing – review & editing. AS: Writing – original draft, Writing – review & editing, Conceptualization, Funding acquisition. MC: Conceptualization, Funding acquisition, Writing – original draft, Writing – review & editing. JD-C: Conceptualization, Funding acquisition, Writing – original draft, Writing – review & editing. JC: Conceptualization, Writing – original draft, Writing – review & editing. CD-S: Conceptualization, Funding acquisition, Supervision, Writing – original draft, Writing – review & editing.
Funding
The author(s) declare financial support was received for the research, authorship, and/or publication of this article. This work has been funded by MCIN/AEI/10.13039/501100011033/European Union “NextGenerationEU”/PRTR (TED2021-130790B-C31 and TED2021-130790B-C32), by Junta de Comunidades de Castilla-La Mancha/EU-FEDER (SBPLY/21/180501/000258), by the Directorate General of Biodiversity Forest and Desertification from the Ministry for the Ecological Transition and the Demographic Challenge through the National Rural Development Program 2014-2020 which is co-financed by 75% from the European Agricultural Fund for Rural Development (FEADER) and by the Portuguese Foundation for Science and Technology (Forest Research Centre by UIDP/00239/2020 and UIDB/00239/2020; Associate Laboratory TERRA by LA/P/0092/2020; MARE by UIDP/04292/2020 and UIDB/04292/2020, ARNET by LA/P/0069/2020, and VF by CEECIND/02484/2018).
Conflict of interest
The authors declare that the research was conducted in the absence of any commercial or financial relationships that could be construed as a potential conflict of interest.
The author(s) declared that they were an editorial board member of Frontiers, at the time of submission. This had no impact on the peer review process and the final decision
Publisher’s note
All claims expressed in this article are solely those of the authors and do not necessarily represent those of their affiliated organizations, or those of the publisher, the editors and the reviewers. Any product that may be evaluated in this article, or claim that may be made by its manufacturer, is not guaranteed or endorsed by the publisher.
References
Abramson, J., Adler, J., Dunger, J., Evans, R., Green, T., Pritzel, A., et al. (2024). Accurate structure prediction of biomolecular interactions with AlphaFold 3. Nature 630, 493–500. doi: 10.1038/s41586-024-07487-w
Acebo-Guerrero, Y., Hernández-Rodríguez, A., Vandeputte, O., Miguélez-Sierra, Y., Heydrich-Pérez, M., Ye, L., et al. (2015). Characterization of Pseudomonas chlororaphis from Theobroma cacao L. rhizosphere with antagonistic activity against Phytophthora palmivora (Butler). J. Appl. Microbiol. 119, 1112–1126. doi: 10.1111/jam.12910
Alcaide, F., Solla, A., Cherubini, M., Mattioni, C., Cuenca, B., Camisón, Á., et al. (2020). Adaptive evolution of chestnut forests to the impact of ink disease in Spain. J. Syst. Evol. 58, 504–516. doi: 10.1111/jse.12551
Alimpić, F., Milovanović, J., Pielech, R., Hinkov, G., Jansson, R., Dufour, S., et al. (2022). The status and role of genetic diversity of trees for the conservation and management of riparian ecosystems: a European experts' perspective. J. Appl. Ecol. 59, 2476–2485. doi: 10.1111/1365-2664.14247
Alonso, A., Boyero, L., Solla, A., Ferreira, V. (2024). Dieback and replacement of riparian trees may impact stream ecosystem functioning. Microb. Ecol. 87, 32. doi: 10.1007/s00248-024-02343-w
Alonso, A., Pérez, J., Monroy, S., Lopez-Rojo, N., Basaguren, A., Bosch, J., et al. (2021). Loss of key riparian plant species impacts stream ecosystem functioning. Ecosystems 24, 1436–1449. doi: 10.1007/s10021-020-00592-7
Aubin, I., Cardou, F., Ryall, K., Kreutzweiser, D., Scarr, T. (2015). Ash regeneration capacity after emerald ash borer (Eab) outbreaks: some early results. For. Chron. 91, 291–298. doi: 10.5558/tfc2015-050
Avila-Quezada, G. D., Rai, M. (2023). Novel nanotechnological approaches for managing Phytophthora diseases of plants. Trends Plant Sci. 28, 1070–1080. doi: 10.1016/j.tplants.2023.03.022
Bajji, M., Thunissen, C., Druart, P. (2013). Adventitious shoot regeneration from in vitro juvenile explants of black alder (Alnus glutinosa [L.] Gaertn.). Biotechnol. Agron. Soc Environ. 17, 12–19.
Barakat, A., DiLoreto, D. S., Zhang, Y., Smith, C., Baier, K., Powell, W. A., et al. (2009). Comparison of the transcriptomes of American chestnut (Castanea dentata) and Chinese chestnut (Castanea mollissima) in response to the chestnut blight infection. BMC Plant Biol. 9, 51. doi: 10.1186/1471-2229-9-51
Barakat, A., Staton, M., Cheng, C. H., Park, J., Yassin, N. B. M., Ficklin, S., et al. (2012). Chestnut resistance to the blight disease: insights from transcriptome analysis. BMC Plant Biol. 12, 38. doi: 10.1186/1471-2229-12-38
Battisti, A., Larsson, S. (2023). “Climate change and forest insect pests,” in Forest Entomology and Pathology. Eds. Allison, J. D., Slippers, B., Wingfield, M. J. (Springer, Cham, Switzerland), 773–787. doi: 10.1007/978-3-031-11553-0_22
Beatty, G., Montgomery, W., Tosh, D., Provan, J. (2015). Genetic provenance and best practice woodland management: a case study in native alder (Alnus glutinosa). Tree Genet. Genomes 11, 92. doi: 10.1007/s11295-015-0919-1
Bergey, D. H., Harrison, F. C., Breed, R. S., Hammer, B. W., Huntoon, F. M. (1930). Bergey's manual of determinative bacteriology. 3rd ed (Baltimore: The Williams and Wilkins Co.).
Bijlsma, R., Loeschcke, V. (2012). Genetic erosion impedes adaptive responses to stressful environments. Evol. Appl. 5, 117–129. doi: 10.1111/j.1752-4571.2011.00214.x
Biurrun, I., Portela-Pereira, E., Mendías, C. S., Rodríguez-González, P. M. (2021). “Osmundo-Alnion glutinosae (Br.-Bl. et al. 1956) Dierschke et Rivas-Mart. in Rivas-Mart. 1975: Floodplain forests of the western (Atlantic half of the Iberian Peninsula,” in Interpretative manual of European riparian forests and shrublands. Eds. Mandžukovski, D., Čarni, A., Sotirovski, K. (Ss Cyril and Methodius University, Skopje, North Macedonia), 21–26.
Bjelke, U., Boberg, J., Oliva, J., Tattersdill, K., McKie, B. G. (2016). Dieback of riparian alder caused by the Phytophthora alni complex: projected consequences for stream ecosystems. Freshw. Biol. 61, 565–579. doi: 10.1111/fwb.12729
Blows, M. W., Hoffmann, A. A. (2005). A reassessment of genetic limits to evolutionary change. Ecology 86, 1371–1384. doi: 10.1890/04-1209
Brasier, C. M., Kirk, S. A. (2001). Comparative aggressiveness of standard and variant hybrid alder Phytophthoras, Phytophthora cambivora and other Phytophthora species on bark of Alnus, Quercus and other woody hosts. Plant Pathol. 50, 218–229. doi: 10.1046/j.1365-3059.2001.00553.x
Brasier, C. M., Rose, J., Gibbs, J. N. (1995). An unusual Phytophthora associated with widespread alder mortality in Britain. Plant Pathol. 44, 999–1007. doi: 10.1111/j.1365-3059.1995.tb02658.x
Brasier, C., Scanu, B., Cooke, D., Jung, T. (2022). Phytophthora: an ancient, historic, biologically and structurally cohesive and evolutionarily successful generic concept in need of preservation. IMA Fungus 13, 12. doi: 10.1186/s43008-022-00097-z
Bregant, C., Batista, E., Hilário, S., Linaldeddu, B. T., Alves, A. (2023). Phytophthora species involved in Alnus glutinosa decline in Portugal. Pathogens 12, 276. doi: 10.3390/pathogens12020276
Černý, K., Strnadová, V. (2012). Winter survival of Phytophthora alni subsp. alni in aerial tissues of black alder. J. For. Sci. 58, 328–336. doi: 10.17221/11/2012-JFS
Chandelier, A., Husson, C., Druart, P., Maçais, B. (2016). Assessment of inoculation methods for screening black alder resistance to Phyophthora xalni. Plant Pathol. 65, 441–450. doi: 10.1111/ppa.12418
Chen, Q., Bakhshi, M., Balci, Y., Broders, K. D., Cheewangkoon, R., Chen, S. F., et al. (2022). Genera of phytopathogenic fungi: GOPHY 4. Stud. Mycol. 101, 417–564. doi: 10.3114/sim.2022.101.06
Chiu, C. C., Pelletier, G., Stival Sena, J., Roux-Dalvai, F., Prunier, J., Droit, A., et al. (2023). Integrative analysis of green ash phloem transcripts and proteins during an emerald ash borer infestation. BMC Plant Biol. 23, 123. doi: 10.1186/s12870-023-04108-y
Claessens, H., Oosterbaan, A., Savill, P., Rondeux, J. (2010). A review of the characteristics of black alder (Alnus glutinosa (L.) Gaertn.) and their implications for silvicultural practices. Forestry 83, 163–175. doi: 10.1093/forestry/cpp038
Cobo-Simón, I., Bennett, J., Fritz, A., Romero-Severson, J., Reid, M., Koch, J., et al. (2021). “Breeding resistant ash for North America: identifying genetic signatures associated with the lingering phenotype in green ash (Fraxinus pennsylvanica),” in Botany 2021 Conference, Virtual. Available at: https://2021.botanyconference.org/engine/search/index.php?func=detail&aid=483.
Comes, H. P., Kadereit, J. W. (1998). The effect of Quaternary climatic changes on plant distribution and evolution. Trends Plant Sci. 3, 432–438. doi: 10.1016/S1360-1385(98)0127-2
Compton, J. E., Church, M. R., Larned, S. T., Hogsett, W. E. (2003). Nitrogen export from forested watersheds in the Oregon Coast Range: the role of N2-fixing red alder. Ecosystems 6, 773–785. doi: 10.1007/s10021-002-0207-4
Corcobado, T., Cech, T. L., Daxer, A., Ďatková, H., Janoušek, J., Patra, S., et al. (2023). Phytophthora, Nothophytophthora and Halophytophthora diversity in rivers, streams and riparian alder ecosystems of Central Europe. Mycol. Prog. 22, 50. doi: 10.1007/s11557-023-01898-1
Corredoira, E., Janeiro, L. V., San José, M. C. (2011). Aplicación de técnicas de cultivo in vitro en la propagación del aliso con vistas a su conservación. Recursos Rurais 7, 49–57. doi: 10.15304/rr.id92
Corredoira, E., Valladares, S., Martínez, M. T., Vieitez, A. M., San José, M. C. (2013). Somatic embryogenesis in Alnus glutinosa (L.) Gaertn. Trees 27, 1597–1608. doi: 10.1007/s00468-013-0907-8
Cubry, P., Gallagher, E., O’Connor, E., Kelleher, C. (2015). Phylogeography and population genetics of black alder (Alnus glutinosa (L.) Gaertn.) in Ireland: putting it in a European context. Tree Genet. Genomes 11, 99. doi: 10.1007/s11295-015-0924-4
De Kort, H., Mergeay, J., Vander Mijnsbrugge, K., Decocq, G., Maccherini, S., Kehlet Bruun, H. H., et al. (2014b). An evaluation of seed zone delineation using phenotypic and population genomic data on black alder Alnus glutinosa. J. Appl. Ecol. 51, 1218–1227. doi: 10.1111/1365-2664.12305
De Kort, H., Vandepitte, K., Bruun, H. H., Closset-Kopp, D., Honnay, O., Mergeay, J. (2014a). Landscape genomics and a common garden trial reveal adaptive differentiation to temperature across Europe in the tree species Alnus glutinosa. Mol. Ecol. 23, 4709–4721. doi: 10.1111/mec.12813
Deng, C. H., Naithani, S., Kumari, S., Cobo-Simón, I., Quezada-Rodríguez, E. H., Skrabisova, M., et al. (2023). Genotype and phenotype data standardization, utilization and integration in the big data era for agricultural sciences. Database, 2023, baad088. doi: 10.1093/database/baad088
de Ronne, M., Santhanam, P., Cinget, B., Labbé, C., Lebreton, A., Ye, H., et al. (2022). Mapping of partial resistance to Phytophthora sojae in soybean PIs using whole-genome sequencing reveals a major QTL. Plant Genome 15, e20184. doi: 10.1002/tpg2.20184
Diánez Martínez, F., Santos, M., Carretero, F., Marín, F. (2016). Trichoderma saturnisporum, a new biological control agent. J. Sci. Food Agric. 96, 1934–1944. doi: 10.1002/jsfa.7301
Díaz-Sala, C. (2014). Direct reprogramming of adult somatic cells toward adventitious root formation in forest tree species: the effect of the juvenile-adult transition. Front. Plant Sci. 5. doi: 10.3389/fpls.2014.00310
Díaz-Sala, C. (2016). “Physiological, cellular, molecular and genomic analysis of the effect of maturation on propagation capacity,” in Vegetative Propagation of Forest Trees. Eds. Park, Y. S., Bonga, J. M., Moon, H. K. (National Institute of Forest Science, Seoul), 75–96.
Díaz-Sala, C. (2019). Molecular dissection of the regenerative capacity of forest tree species: special focus on conifers. Front. Plant Sci. 9. doi: 10.3389/fpls.2018.01943
Díaz-Vázquez, R., Menéndez, M., Prada, E. (2020). La mejora genética como herramienta de control frente al nematodo del pino. Primeros pinos tolerantes catalogados en España. Foresta 78, 84–88.
Elegbede, C. F., Pierrat, J. C., Aguayo, J., Husson, C., Halkett, F., Marçais, B. A. (2010). Statistical model to detect asymptomatic infectious individuals with an application in the Phytophthora alni-induced alder decline. Phytopathology 100, 1262–1269. doi: 10.1094/PHYTO-05-10-0140
Eschen, R., Rigaux, L., Sukovata, L., Vettraino, A. M., Marzano, M., Grégoire, J.-C. (2015). Phytosanitary inspection of woody plants for planting at European Union entry points: a practical enquiry. Biol. Invasions 17, 2403–2413. doi: 10.1007/s10530-015-0883-6
Fan, S., Georgi, L. L., Hebard, F. V., Zhebentyayeva, T., Yu, J., Sisco, P. H., et al. (2024). Mapping QTLs for blight resistance and morpho-phenological traits in inter-species hybrid families of chestnut (Castanea spp.). Front. Plant Sci. 8. doi: 10.3389/fpls.2024.1365951
Feio, M. J., Alves, T., Boavida, M., Medeiros, A., Graça, M. A. S. (2010). Functional indicators of stream health: ariver-basin approach. Freshw. Biol. 55, 1050–1065. doi: 10.1111/j.1365-2427.2009.02332.x
Ferentinos, K. (2018). Deep learning models for plant disease detection and diagnosis. Comp. Electron. Agr. 145, 311–318. doi: 10.1016/j.compag.2018.01.009
Fernandes, P., Colavolpe, M. B., Serrazina, S., Costa, R. L. (2022). European and American chestnuts: an overview of the main threats and control efforts. Front. Plant Sci. 13. doi: 10.3389/fpls.2022.951844
Fernandes, P., Pimentel, D., Ramiro, R. S., do Céu Silva, M., Fevereiro, P., Costa, R. L. (2024). Dual transcriptomic analysis reveals early induced Castanea genes and Phytophthora cinnamomi effectors. Front. Plant Sci. 15. doi: 10.3389/fpls.2024.1439380
Ferreira, V., Encalada, A. C., Graça, M. A. S. (2012). Effects of litter diversity on decomposition and biological colonization of submerged litter in temperate and tropical streams. Freshw. Sci. 31, 945–962. doi: 10.1899/11-062.1
Ferreira, V., Pazianoto, L. H., Solla, A. (2022). Invasive forest pathogens affect the characteristics, microbial colonisation, and decomposition of leaf litter in streams. Freshw. Biol. 67, 416–429. doi: 10.1111/fwb.13851
Fisher, M. C., Gurr, S. J., Cuomo, C. A., Blehert, D. S., Jin, H., Stukenbrock, E. H., et al. (2020). Threats posed by the fungal kingdom to humans, wildlife, and agriculture. mBio 11. doi: 10.1128/mBio.00449-20
Fuller, E., Germaine, K. J., Rathore, D. S. (2023). The good, the bad, and the useable microbes within the common alder (Alnus glutinosa) microbiome-potential bio-agents to combat alder dieback. Microorganisms 11, 2187. doi: 10.3390/microorganisms11092187
Gibbs, J. N., Lipscombe, M. A., Peace, A. J. (1999). The impact of Phytophthora disease on riparian populations of common alder (Alnus glutinosa) in southern Britain. Eur. J. For. Pathol. 29, 39–50. doi: 10.1046/j.1439-0329.1999.00129.x
Gibbs, J. N., Van Dijk, C., Webber, J. F. (2003). Phytophthora disease of alder in Europe. Forestry Commission Bulletin 126 (Edinburgh, UK: Forestry Commission).
Gomes Marques, I., Solla, A., David, T. S., Rodríguez-González, P. M., Garbelotto, M. (2022). Response of two riparian woody plants to Phytophthora species and drought. For. Ecol. Manage. 518, 120281. doi: 10.1016/j.foreco.2022.120281
Gomes Marques, I., Vieites-Blanco, C., Barrento, M. J., Semedo, J. N., Rodrigues, A. P., Scotti-Campos, P., et al. (2024a). Phenotypic variation and genetic diversity in European Alnus species. Forestry, cpae039. doi: 10.1093/forestry/cpae039
Gomes Marques, I., Vieites-Blanco, C., Rodríguez-González, P. M., Segurado, P., Marques, M., Barrento, M. J., et al. (2024b). The ADnet Bayesian belief network for alder decline: Integrating empirical data and expert knowledge. Sci. Total Environ. 947, 173619. doi: 10.1016/j.scitotenv.2024.173619
Gomes Marques, I. (2024). Resilience of alder in response to global change stressors. PhD thesis. School of Agriculture, University of Lisbon.
Graça, M. A. S., Cressa, C. (2010). Leaf quality of some tropical and temperate tree species as food resource for stream shredders. Int. Rev. Hydrobiol. 95, 27–41. doi: 10.1002/iroh.200911173
Greenwood, M. S., Weir, R. J. (1995). Genetic variation in rooting ability of loblolly pine cuttings: effect of auxin and family on rooting by hypocotyl cuttings. Tree Physiol. 15, 41–45. doi: 10.1093/treephys/15.1.41
Gryta, H., Van de Paer, C., Manzi, S., Holota, H., Roy, M., Besnard, G. (2017). Genome skimming and plastid microsatellite profiling of alder trees (Alnus spp., Betulaceae): phylogenetic and phylogeographical prospects. Tree Genet. Genomes 13, 1–14. doi: 10.1007/s11295-017-1204-2
Guerra-Hernández, J., Díaz Varela, R. A., Álvarez González, J. G., Rodríguez González, P. M. (2021). Assessing a novel modelling approach with high resolution UAV imagery for monitoring health status in priority riparian forests. For. Ecosyst. 61, 8. doi: 10.1186/s40663-021-00342-8
Guignard, L., Sauvageau, C. (1894). Sur un nouveau microbe chromogene, le Bacillus chlororaphis. C. R. Soc Biol. Paris Ser. 10 1, 841–843.
Hamilton, J. A., Royauté, R., Wright, J. W., Hodgskiss, P., Ledig, F. T. (2017). Genetic conservation and management of the California endemic, Torrey pine (Pinus torreyana Parry): Implications of genetic rescue in a genetically depauperate species. Ecol. Evol. 7, 7370–7381. doi: 10.1002/ece3.3306
Handa, I. T., Aerts, R., Berendse, F., Berg, M. P., Bruder, A., Butenschoen, O., et al. (2014). Consequences of biodiversity loss for litter decomposition across biomes. Nature 509, 218–221. doi: 10.1038/nature13247
Hansen, E. M., Reeser, P., Sutton, W., Sniezko, R. A. (2012). “Methods for screening Port-Orford-Cedar for resistance to Phytophthora lateralis,” in Proceedings of the fourth international workshop on the genetics of host-parasite interactions in forestry: disease and insect resistance in forest trees. Trees. Gen. Tech. Rep. PSW-GTR-240 (Pacific Southwest Research Station, Forest Service, U.S. Department of Agriculture, Albany, CA), 181–188.
Haque, M. M., Martínez-Álvarez, P., Lomba, J. M., Martín-García, J., Diez, J. J. (2014). First report of Phytophthora plurivora causing collar rot on common alder in Spain. Plant Dis. 98, 425–425. doi: 10.1094/PDIS-07-13-0784-PDN
Haque, M. M. U., Martín-García, J., Diez, J. J. (2015). Variation in pathogenicity among the three subspecies of Phytophthora alni on detached leaves, twigs and branches of Alnus glutinosa. For. Pathol. 45, 484–491. doi: 10.1111/efp.12198
Havrdová, A., Douda, J., Krak, K., Vít, P., Hadincová, V., Zákravský, P., et al. (2015). Higher genetic diversity in recolonized areas than in refugia of Alnus glutinosa triggered by continent-wide lineage admixture. Mol. Ecol. 24, 4759–4777. doi: 10.1111/efp.12239
Herms, D. A., McCullough, D. G. (2014). Emerald ash borer invasion of North America: history, biology, ecology, impacts, and management. Annu. Rev. Entomol. 59, 13–30. doi: 10.1146/annurev-ento-011613-162051
Horta Jung, M., Maia, C., Mora-Sala, B., Abad-Campos, P., Schena, L., Mosca, S., et al. (2024). High diversity of Phytophthora species in natural ecosystems and nurseries of Portugal: Detrimental side effect of plant introductions from the age of discovery to modern globalization. Plant Pathol. 00, 1–33. doi: 10.1111/ppa.14022
Huff, M., Seaman, J., Wu, D., Zhebentyayeva, T., Kelly, L. J., Faridi, N., et al. (2022). A high-quality reference genome for Fraxinus pennsylvanica for ash species restoration and research. Mol. Ecol. Resour. 22, 1284–1302. doi: 10.1111/1755-0998.13545
Husson, C., Aguayo, J., Revellin, C., Frey, P., Ioos, R., Marçais, B. (2015). Evidence for homoploid speciation in Phytophthora alni supports taxonomic reclassification in this species complex. Fungal Genet. Biol. 77, 12–21. doi: 10.1016/j.fgb.2015.02.013
Jabiol, J., Lecerf, A., Lamothe, S., Gessner, M. O., Chauvet, E. (2019). Litter quality modulates effects of dissolved nitrogen on leaf decomposition by stream microbial communities. Microb. Ecol. 77, 959–966. doi: 10.1007/s00248-019-01353-3
Jacobs, D. C., Revord, R. S., Capik, J. M., Mehlenbacher, S. A., Thomas, J. M. (2024). Variable response of eastern filbert blight resistance sources in New Jersey. Front. Plant Sci. 15. doi: 10.3389/fpls.2024.1419265
Jung, T., Blaschke, M. (2004). Phytophthora root and collar rot of alders in Bavaria: distribution, modes of spread and possible management strategies. Plant Pathol. 53, 197–208. doi: 10.1111/j.0032-0862.2004.00957.x
Jung, T., Burgess, T. I. (2009). Re-evaluation of Phytophthora citricola isolates from multiple woody hosts in Europe and North America reveals a new species, Phytophthora plurivora sp. nov. Persoonia - Mol. Phylogeny Evol. Fungi 22, 95–110. doi: 10.3767/003158509X442612
Jung, T., Cech, T., Vannini, A. (2013). “The impact of invasive Phytophthora species on European forests,” in Phytophthora: a global perspective. Ed. Lamour, K. (CABI Digital Library), 146–158. doi: 10.1079/9781780640938.0146
Jung, T., Orlikowski, L., Henricot, B., Abad-Campos, P., Aday, A. G., Aguín Casal, O., et al. (2016). Widespread Phytophthora infestations in European nurseries put forest, semi-natural and horticultural ecosystems at high risk of Phytophthora diseases. For. Pathol. 46, 134–163. doi: 10.1111/efp.12239
Jung, T., Pérez-Sierra, A., Durán, A., Jung, M. H., Balci, Y., Scanu, B. (2018). Canker and decline diseases caused by soil-and airborne Phytophthora species in forests and woodlands. Persoonia: Mol. Phylogeny Evol. Fungi 40, 182–220. doi: 10.3767/persoonia.2018.40.08
Kanoun-Boulé, M., Vasconcelos, T., Gaspar, J., Vieira, S., Dias-Ferreira, C., Husson, C. (2016). Phytophthora × alni and Phytophthora lacustris associated with common alder decline in Central Portugal. For. Pathol. 46, 174–176. doi: 10.1111/efp.12273
Kelly, L. J., Plumb, W. J., Carey, D. W., Mason, M. E., Cooper, E. D., Crowther, W., et al. (2020). Convergent molecular evolution among ash species resistant to the emerald ash borer. Nat. Ecol. Evol. 4, 1116–1128. doi: 10.1038/s41559-020-1209-3
Keriö, S., Daniels, H. A., Gómez-Gallego, M., Tabima, J. F., Lenz, R. R., Søndreli, K. L., et al. (2019). From genomes to forest management – tackling invasive Phytophthora species in the era of genomics. Can. J. Plant Pathol. 42, 1–29. doi: 10.1080/07060661.2019.1626910
Lane, T., Best, T., Zembower, N., Davitt, J., Henry, N., Xu, Y., et al. (2016). The green ash transcriptome and identification of genes responding to abiotic and biotic stresses. BMC Genomics 17, 702. doi: 10.1186/s12864-016-3052-0
Lee, B. D., Dutta, S., Ryu, H., Yoo, S. J., Suh, D. S., Park, K. (2015). Induction of systemic resistance in Panax ginseng against Phytophthora cactorum by native Bacillus amyloliquefaciens HK34. J. Ginseng Res. 39, 213–220. doi: 10.1016/j.jgr.2014.12.002
Lee, J. H., Pijut, P. M. (2018). Optimization of Agrobacterium-mediated genetic transformation of Fraxinus nigra and development of black ash for possible emerald ash borer resistance. Plant Cell Tiss. Organ Cult. 134, 217–229. doi: 10.1007/s11240-018-1414-9
Lenz, P. R. N., Nadeau, S., Mottet, M.-J., Perron, M., Isabel, N., Beaulieu, J., et al. (2020). Multi-trait genomic selection for weevil resistance, growth, and wood quality in Norway spruce. Evol. Appl. 13, 76–94. doi: 10.1111/eva.12823
Lepais, O., Muller, S., Ben Saad-Limam, S., Benslama, M., Rhazi, L., Belouahem-Abed, D., et al. (2013). High genetic diversity and distinctiveness of rear-edge climate relicts maintained by ancient tetraploidisation for Alnus glutinosa. PLoS One 8, e75029. doi: 10.1371/JOURNAL.PONE.0075029
Li, W., Zheng, X., Cheng, R., Zhong, C., Zhao, J., Liu, T. H., et al. (2023). Soybean ZINC FINGER PROTEIN03 targets two SUPEROXIDE DISMUTASE1s and confers resistance to Phytophthora sojae. Plant Physiol. 192, 633–647. doi: 10.1093/plphys/kiad083
Lima, R. P. M., Máximo, H. J., Merfa, M. V., Dalio, R. J. D., Cristofani-Yaly, M., MaChado, M. A. (2018). Genetic tools and strategies for citrus breeding aiming at resistant rootstocks to gummosis disease. Trop. Plant Pathol. 43, 279–288. doi: 10.1007/s40858-018-0229-x
Lin, Y.-C., Mansfeld, B. N., Tang, X., Colle, M., Chen, F., Weng, Y., et al. (2023). Identification of QTL associated with resistance to Phytophthora fruit rot in cucumber (Cucumis sativus L.). Front. Plant Sci. 14. doi: 10.3389/fpls.2023.1281755
Macháčová, M., Tomášková, I., Corcobado, T., Nagy, Z., Milanović, S., Janoušek, J., et al. (2024). Response of Alnus glutinosa to Phytophthora bark infections at ambient and elevated CO2 levels. Front. For. Glob. Change 7. doi: 10.3389/ffgc.2024.1379791
Mamidala, P., Wijeratne, A. J., Wijeratne, S., Poland, T., Qazi, S. S., Doucet, D., et al. (2013). Identification of odor-processing genes in the emerald ash borer, agrilus planipennis. PLoS One 8, e56555. doi: 10.1371/journal.pone.0056555
Mandák, B., Vít, P., Krak, K., Trávníček, P., Havrdová, A., Hadincová, V., et al. (2016). Flow cytometry, microsatellites and niche models reveal the origins and geographical structure of Alnus glutinosa populations in Europe. Ann. Bot. 117, 107–120. doi: 10.1093/AOB/MCV158
Martín, J. A., López, R. (2023). Biological deterioration and natural durability of wood in Europe. Forests 14, 283. doi: 10.3390/f14020283
Martín, M. A., Moreno, R., Die, J. V., Cabrera, A., Castro, P., Pérez, M. D., et al. (2024). Distribution, diversity and genetic structure of alders (Alnus lusitanica and A. glutinosa) in Spain. For. Ecol. Manage. 562, 121922. doi: 10.1016/j.foreco.2024.121922
Martínez, M. T., Cuenca, B., Mosteiro, F., Piñeiro, P., Pérez, F., Solla, A., et al. (2023). Screening of cork oak for resistance to Phytophthora cinnamomi and micropropagation of tolerant seedlings. Horticulturae 9, 692. doi: 10.3390/horticulturae9060692
Martín-García, J., Solla, A., Corcobado, T., Siasou, E., Woodward, S. (2015). Influence of temperature on germination of Quercus ilex in Phytophthora cinnamomi, P. gonapodyides, P. quercina and P. psychrophila infested soils. For. Pathol. 45, 215–223. doi: 10.1111/efp.12159
Menéndez-Gutiérrez, M., Alonso, M., Díaz, R. (2021). Assessing genetic variation in resistance to pinewood nematode (Bursaphelenchus xylophilus) in Pinus radiata D. Don half-sib families. Forests 12, 1474. doi: 10.3390/f12111474
Menéndez-Gutiérrez, M., Alonso, M., Toval, G., Díaz, R. (2018). Testing of selected Pinus pinaster half-sib families for tolerance to pinewood nematode (Bursaphelenchus xylophilus). Forestry: Int. J. For. Res. 91, 38–48. doi: 10.1093/forestry/cpx030
Mercado-Blanco, J., Abrantes, I., Barra Caracciolo, A., Bevivino, A., Ciancio, A., Grenni, P., et al. (2018). Belowground microbiota and the health of tree crops. Front. Microbiol. 9. doi: 10.3389/fmicb.2018.01006
Merkle, S. A., Koch, J. L., Tull, A. R., Dassow, J. E., Carey, D. W., Barnes, B. F., et al. (2023). Application of somatic embryogenesis for development of emerald ash borer-resistant white ash and green ash varietals. New For. 54, 697–720. doi: 10.1007/s11056-022-09903-3
Mingeot, D., Baleux, R., Watillon, B. (2010). Characterization of microsatellite markers for black alder (Alnus glutinosa [L.] Gaertn). Conserv. Genet. Resour. 2, 269–271. doi: 10.1007/s12686-010-9188-3
Miranda-Fontaíña, M. E., Fernández-López, J., Vettraino, A. M., Vannini, A. (2007). Resistance of Castanea clones to Phytophthora cinnamomi: testing and genetic control. Silvae Genetica 56, 11–21. doi: 10.1515/sg-2007-0002
Molinero, J., Pozo, J. (2006). Organic matter, nitrogen and phosphorus fluxes associated with leaf litter in two small streams with different riparian vegetation: a budget approach. Arch. Hydrobiol. 166, 363–386. doi: 10.1127/0003-9136/2006/0166-0363
Mora-Sala, B., León, M., Pérez-Sierra, A., Abad-Campos, P. (2022). New reports of Phytophthora species in plant nurseries in Spain. Pathogens 11, 826. doi: 10.3390/pathogens11080826
Mpika, J., Kébé, I. B., Issali, A. E., N’guessan, F. K., Druzhinina, S., Komon-Zélazowska, M., et al. (2009). Antagonist potential of Trichoderma indigenous isolates for biological control of Phytophthora palmivora the causative agent of black pod disease on cocoa (Theobroma cacao L.) in Côte d’Ivoire. Afr. J. Biotechnol. 8, 5280–5293. doi: 10.4314/ajb.v8i20.65962
Narayan, H., Srivasatava, P., Chandra Bhatt, S., Joshi, D., Soni, R. (2022). “Plant pathogenesis and disease control,” in Plant Protection: From Chemicals to Biologicals. Eds. Soni, R., Suyal, D. C., Goel, R. (De Gruyter, Berlín, Boston, MA), 95–114. doi: 10.1515/9783110771558-005
Nave, C., Schwan, J., Werres, S., Riebesehl, J. (2021). Alnus glutinosa threatened by alder Phytophthora: a histological study of roots. Pathogens 10, 977. doi: 10.3390/pathogens10080977
Neale, D. B., Kremer, A. (2011). Forest tree genomics: growing resources and applications. Nat. Rev. Genet. 12, 111–122. doi: 10.1038/nrg2931
Nie, X., Zhao, S., Hao, Y., Gu, S., Zhang, Y., Qi, B., et al. (2023). Transcriptome analysis reveals key genes involved in the resistance to Cryphonectria parasitica during early disease development in Chinese chestnut. BMC Plant Biol. 23, 79. doi: 10.1186/s12870-023-04072-7
Novotná, K., Štochlová, P. (2012). Selection of the best method for vegetative propagation of mature Alnus glutinosa (L.) Gaertn. trees resistant to Phytophthora alni. Acta Univ. Agric. silvic. Mendel. Brun. 60, 105–110. doi: 10.11118/actaun201260010105
Oßwald, W., Fleischmann, F., Rigling, D., Coelho, A. C., Cravador, A., Diez, J., et al. (2014). Strategies of attack and defence in woody plant–Phytophthora interactions. For. Path. 44, 169–190. doi: 10.1111/efp.12096
Pérez, F., Cuenca, B., Ruíz-Gómez, F. J., Rey, M. D., Galea, M. R., Arrillaga, I., et al. (2020). Programa de mejora y conservación de los recursos genéticos de la encina y el alcornoque frente al síndrome de la seca. Foresta 78, 56–61.
Périnet, F., Tremblay, F. M. (1987). Commercial micropropagation of five Alnus species. New For. 3, 225–230. doi: 10.1007/BF00118760
Pintos-Varela, C., Martínez, C. R., Aguín Casal, O., Vázquez, J. P. M., Yebra, A. A. (2012). First report of Phytophthora alni subsp. uniformis on black alder in Spain. Plant Dis. 96, 589. doi: 10.1094/PDIS-10-11-0891-PDN
Pintos-Varela, C., Rial, C., Aguín, O., Ferreiroa, V., Mansilla, J. P. (2016). First report of Phytophthora hydropathica in river water associated with riparian alder in Spain. New Dis. Rep. 33, 25. doi: 10.5197/j.2044-0588.2016.033.025
Pintos-Varela, C., Rial-Martínez, C., Aguín-Casal, O., Mansilla-Vázquez, J. P. (2017). First report of Phytophthora × multiformis on Alnus glutinosa in Spain. Plant Dis. 101, 261. doi: 10.1094/PDIS-08-16-1092-PDN
Pintos-Varela, C., Rial Martínez, C., Mansilla Vázquez, J. P., Aguín Casal, O. (2010). First report of Phytophthora rot on alders caused by Phytophthora alni subsp. alni in Spain. Plant Dis. 94, 273. doi: 10.1094/PDIS-94-2-0273A
Poland, T. M., McCullough, D. G. (2006). Emerald ash borer: invasion of the urban forest and the threat to North America’s ash resource. J. For. 104, 118–124. doi: 10.1093/jof/104.3.118
Poovaiah, C., Phillips, L., Geddes, B., Reeves, C., Sorieul, M., Thorlby, G. (2021). Genome editing with CRISPR/Cas9 in Pinus radiata (D. Don). BMC Plant Biol. 21, 363. doi: 10.1186/s12870-021-03143-x
Portela-Pereira, E., Monteiro, P., Rodríguez-González, P. M. (2022). Control of invasive plant species in wetland forests (91E0*). Biol. Life Sci. Forum 13, 84. doi: 10.3390/blsf2022013084
Pozo, J., González, E., Díez, J. R., Molinero, J., Elósegui, A. (1997). Inputs of particulate organic matter to streams with different riparian vegetation. J. N. Am. Benthol. Soc 16, 602–611. doi: 10.2307/1468147
Quintana-Rodríguez, E., Morales-Vargas, A. T., Molina-Torres, J., Ádame-Alvarez, R. M., Acosta-Gallegos, J. A., Heil, M. (2015). Plant volatiles cause direct, induced and associational resistance in common bean to the fungal pathogen Colletotrichum lindemuthianum. J. Ecol. 103, 250–260. doi: 10.1111/1365-2745.12340
Radwan, M. A., Max, T. A., Johnson, D. W. (1989). Softwood cuttings for propagation of red alder. New For. 3, 21–30. doi: 10.1007/BF00128898
Rairdin, A., Fotouhi, F., Zhang, J., Mueller, D. S., Ganapathysubramanian, B., Singh, A. K., et al. (2022). Deep learning-based phenotyping for genome wide association studies of sudden death syndrome in soybean. Front. Plant Sci. 13. doi: 10.3389/fpls.2022.966244
Redondo, M. A., Boberg, J., Olsson, C. H. B., Oliva, J. (2015b). Winter conditions correlate with Phytophthora alni subspecies distribution in southern Sweden. Phytopathology 105, 1191–1197. doi: 10.1094/PHYTO-01-15-0020-R
Redondo, M. A., Pérez-Sierra, A., Abad-Campos, P., Torres, L., Solla, A., Reig-Armiñana, J., et al. (2015a). Histology of Quercus ilex roots during infection by Phytophthora cinnamomi. Trees 29, 1943–1957. doi: 10.1007/s00468-015-1275-3
Redondo, M. A., Stenlid, J., Oliva, J. (2020). Genetic variation explains changes in susceptibility in a naïve host against an invasive forest pathogen: the case of alder and the Phytophthora alni complex. Phytopathology 110, 517–525. doi: 10.1094/PHYTO-07-19-0272-R
Rhoades, C., Oskarsson, H., Binkley, D., Stottlemyer, B. (2001). Alder (Alnus crispa) effects on soils in ecosystems of the Agashashok River valley, northwest Alaska. Ecoscience 8, 89–95. doi: 10.1080/11956860.2001.11682634
Rial-Martínez, C., Souto-Herrero, M., Piñón-Esteban, P., García-González, I., Aguín-Casal, O., Salinero-Corral, C., et al. (2023). First report of root rot caused by Phytophthora lacustris on alder (Alnus lusitanica) in Spain. Plant Dis. 107, 3322. doi: 10.1094/PDIS-04-23-0793-PDN
Ro, N., Haile, M., Hur, O., Geum, B., Rhee, J., Hwang, A., et al. (2022). Genome-wide association study of resistance to Phytophthora capsici in the pepper (Capsicum spp.) collection. Front. Plant Sci. 13. doi: 10.3389/fpls.2022.902464
Rodrigues da Silva, A., da Costa Silva, D., Dos Santos Pinto, K. N., Santos Filho, H. P., Coelho Filho, M. A., Dos Santos Soares Filho, W., et al. (2021). Epigenetic responses to Phytophthora citrophthora gummosis in citrus. Plant Sci. 313, 111082. doi: 10.1016/j.plantsci.2021.111082
Rodriguez, R., Diíaz-Sala, C., Cuozzo, L., Ancora, G. (1991). Pear in vitro propagation using a double-phase culture system. HortScience 26, 62–64. doi: 10.21273/HORTSCI.26.1.62
Rodríguez-González, P. M., García, C., Albuquerque, A., Monteiro-Henriques, T., Faria, C., Guimarães, J. B., et al. (2019). A spatial stream-network approach assists in managing the remnant genetic diversity of riparian forests. Sci. Rep. 9, 6741. doi: 10.1038/s41598-019-43132-7
Rodríguez-González, P. M., Stella, J. C., Campelo, F., Ferreira, M. T., Albuquerque, A. (2010). Subsidy or stress? Tree structure and growth in wetland forests along a hydrological gradient in Southern Europe. For. Ecol. Manage. 259, 2015–2025. doi: 10.1016/j.foreco.2010.02.012
Rubio-Ríos, J., Salinas-Bonillo, M. J., Pérez, J., Fenoy, E., Boyero, L., Casas, J. J. (2023). Alder stands promote N-cycling but not leaf litter mass loss in Mediterranean streams flowing through pine plantations. For. Ecol. Manage. 542, 121072. doi: 10.1016/j.foreco.2023.121072
San José, M. C., Blázquez, N., Cernadas, M. J., Janeiro, L. V., Cuenca, B., Sánchez, C., et al. (2020). Temporary immersion systems to improve alder micropropagation. Plant Cell Tiss. Organ Cult. 143, 265–275. doi: 10.1007/s11240-020-01937-9
San José, M. C., Corredoira, E., Janeiro, L. V. (2011). Efecto de los carbohidratos sobre la micropropagacion de Alnus glutinosa (L.) Gaertn. Span. J. Rural Dev. 2, 9–18. doi: 10.5261/2011.GEN4.02
San José, M. D. C., Corredoira, E., Oliveira, H., Santos, C. (2015b). Cryopreservation of somatic embryos of Alnus glutinosa (L.) Gaertn. and confirmation of ploidy stability by flow cytometry. Plant Cell Tiss. Org. Cult. 123, 489–499. doi: 10.1007/s11240-015-0853-9
San José, M. C., Janeiro, L. V., Corredoira, E. (2013). Micropropagation of threatened black alder. Silva Fennica 47, 892. doi: 10.14214/sf.892
San José, M. C., Janeiro, L. V., Corredoira, E. (2015a). Simple strategy for the in vitro conservation of Alnus glutinosa (L.) Gaertn. germplasm. Trees-Struct. Funct. 29, 539–549. doi: 10.1007/s00468-014-1133-8
San José, M. C., Romero, L., Janeiro, L. V. (2012). Effect of indole-3-butyric acid on root formation in Alnus glutinosa microcuttings. Silva Fenn. 46, 643–654. doi: 10.14214/sf.916
Sanna, M., González Toral, C., Nava, H. S., Cuesta, C., Loidi, J., Herrera, M., et al. (2023). Contribution to the knowledge of the distribution of Alnus species in southern Europe based on cpDNA. Nat. Cantab. 11, 41–52.
Santos, C., MaChado, H., Correia, I., Gomes, F., Gomes-Laranjo, J., Costa, R. (2015). Phenotyping Castanea hybrids for Phytophthora cinnamomi resistance. Plant Pathol. 64, 901–910. doi: 10.1111/ppa.12313
Santos, C., Nelson, C. D., Zhebentyayeva, T., MaChado, H., Gomes-Laranjo, J., Costa, R. L. (2017). First interspecific genetic linkage map for Castanea sativa x Castanea crenata revealed QTLs for resistance to Phytophthora cinnamomi. PLoS One 12, e0184381. doi: 10.1371/journal.pone.0184381
Schmitt, U., Singh, A. P., Kim, Y. S. (2021). “Wood as an ecological niche for microorganisms: wood formation, structure, and cell wall composition,” in Forest Microbiology: Tree Microbiome - Phyllosphere, Endosphere and Rhizosphere. Eds. Asiegbu, F. O., Kovalchuk, A. (Elsevier, San Diego, CA, USA), 17–34. doi: 10.1016/B978-0-12-822542-4.00010-3
Shaftel, R. S., King, R. S., Back, J. A. (2012). Alder cover drives nitrogen availability in Kenai lowland headwater streams, Alaska. Biogeochemistry 107, 135–148. doi: 10.1007/s10533-010-9541-3
Shainsky, L. J., Rose, C. L. (1995). Effects of competition on the foliar chemistry of young Douglas-fir in monoculture and mixed stands with young red alder. Can. J. For. Res. 25, 1969–1977. doi: 10.1139/x95-212
Sharma, U., Sankhyan, H. P., Kumari, A., Thakur, S., Thakur, L., Mehta, D., et al. (2024). Genomic selection: a revolutionary approach for forest tree improvement in the wake of climate change. Euphytica 220, 9. doi: 10.1007/s10681-023-03263-5
Shen, S., Fan, Z., Zhang, X., Kong, X., Liu, F., Zhang, Z., et al. (2021). The characteristics of chemosensory and opsin genes in newly emerged and sexually mature Agrilus planipennis, an important quarantine forest beetle. Front. Genet. 11. doi: 10.3389/fgene.2020.604757
Siddique, M. I., Lee, H. Y., Ro, N. Y., Han, K., Venkatesh, J., Solomon, A. M., et al. (2019). Identifying candidate genes for Phytophthora capsici resistance in pepper (Capsicum annuum) via genotyping-by-sequencing-based QTL mapping and genome-wide association study. Sci. Rep. 9, 9962. doi: 10.1038/s41598-019-46342-1
Siviero, A., Cristofani, M., Furtado, E. L., Garcia, A. A., Coelho, A. S., MaChado, M. A. (2006). Identification of QTLs associated with citrus resistance to Phytophthora gummosis. J. Appl. Genet. 47, 23–28. doi: 10.1007/BF03194595
Sjöman, H., Watkins, H., Kelly, L. J., Hirons, A., Kainulainen, K., Martin, K. W. E., et al. (2024). Resilient trees for urban environments: the importance of intraspecific variation. Plants People Planet 6, 1180–1189. doi: 10.1002/ppp3.10518
Smeriglio, A., D’Angelo, V., Cacciola, A., Ingegneri, M., Raimondo, F. M., Trombetta, D., et al. (2022). New insights on phytochemical features and biological properties of Alnus glutinosa stem bark. Plants 11, 2499. doi: 10.3390/plants11192499
Šmíd, J., Douda, J., Krak, K., Mandák, B. (2020). Analyses of hybrid viability across a hybrid zone between two Alnus species using microsatellites and cpDNA markers. Genes 11, 770. doi: 10.3390/GENES11070770
Sniezko, R. A. (2006). Resistance breeding against nonnative pathogens in forest trees: current successes in North America. Can. J. Plant Pathol. 28, S270–S279. doi: 10.1080/07060660609507384
Sniezko, R. A., Koch, J. (2017). Breeding trees resistant to insects and diseases: putting theory into application. Biol. Invasions 19, 3377–3400. doi: 10.1007/s10530-017-1482-5
Solla, A., Pérez-Sierra, A., Corcobado, T., Haque, M. M., Diez, J. J., Jung, T. (2010). Phytophthora alni on Alnus glutinosa reported for the first time in Spain. Plant Pathol. 59, 798–798. doi: 10.1111/j.1365-3059.2009.02254.x
Sollars, E., Harper, A., Kelly, L., Sambles, C. M., Ramirez-Gonzalez, R. H., Swarbreck, D., et al. (2017). Genome sequence and genetic diversity of European ash trees. Nature 541, 212–216. doi: 10.1038/nature20786
Spalding, E. P., Miller, N. D. (2013). Image analysis is driving a renaissance in growth measurement. Curr. Opin. Plant Biol. 16, 100–104. doi: 10.1016/j.pbi.2013.01.001
Stanley, R. K., Carey, D. W., Mason, M. E., Doran, A., Wolf, J., Otoo, K. O., et al. (2023). Emerald ash borer (Agrilus planipennis) infestation bioassays and metabolic profiles of green ash (Fraxinus pennsylvanica) provide evidence for an induced host defensive response to larval infestation. Front. For. Glob. Change 6. doi: 10.3389/ffgc.2023.1166421
Steddom, K., Becker, O., Menge, J. A. (2002). Repetitive applications of the biocontrol agent Pseudomonas putida 06909-rif/nal and effects on populations of Phytophthora parasitica in citrus orchards. Phytopathology 92, 850–856. doi: 10.1094/PHYTO.2002.92.8.850
Štochlová, P., Novotná, K., Černý, K. (2012). Factors affecting the development of Phytophthora alni ssp. alni infections in Alnus glutinosa L. J. For. Sci. 58, 123–130. doi: 10.17221/26/2011-JFS
Tapias, R., Fernández, M., Moreira, A. C., Sánchez, E., Cravador, A. (2006). “Posibilidades de la variabilidad genética de encinas y alcornoques en la conservación y recuperación de bosques amenazados por la “seca”,” in Boletín informativo CIDEU. Ed. Tapias, R. (Huelva, Spain: CIDEU-Universidad de Huelva), 45–51.
Tapias, R., Moreira, A. C., Fernández, M., Saenz, A., Domingos, A. C., Melo, E., et al. (2008). “Variability in the tolerance/resistance of Quercus suber L. seedlings to Phytophthora cinnamomi Rands: evaluation of survival,” in Suberwood: new challenges for the integration of cork oak forests and products. Eds. Vázquez-Piqué, J., Pereira, H., González-Pérez, A. (Huelva, Spain: Universidad de Huelva Publicaciones), 237–246.
Teklehaimanot, Z., Mmolotsi, R. M. (2007). Contribution of red alder to soil nitrogen input in a silvopastoral system. Biol. Fert. Soils 43, 843–848. doi: 10.1007/s00374-006-0163-9
Teshome, D. T., Zharare, G. E., Naidoo, S. (2020). The threat of the combined effect of biotic and abiotic stress factors in forestry under a changing climate. Front. Plant Sci. 11. doi: 10.3389/fpls.2020.601009
Tian, L., Lin, X., Tian, J., Ji, L., Chen, Y., Tran, L.-S. P., et al. (2020). Research advances of beneficial microbiota associated with crop plants. Int. J. Mol. Sci. 21, 1792. doi: 10.3390/ijms21051792
Tremblay, É.D., Duceppe, M.-O., Bérubé, J. A., Kimoto, T., Lemieux, C., Bilodeau, G. J. (2018). Screening for exotic forest pathogens to increase survey capacity using metagenomics. Phytopathology 108, 1509–1521. doi: 10.1094/PHYTO-02-18-0028-R
Tremblay, F. M., Lalonde, M. (1984). Requirements for in vitro propagation of seven nitrogen-fixing Alnus species. Plant Cell Tiss. Organ Cult. 3, 189–199. doi: 10.1007/BF00040337
Trzewik, A., Maciorowski, R., Orlikowska, T. (2021). Pathogenicity of Phytophthora× alni isolates obtained from symptomatic trees, soil and water against alder. Forests 13, 20. doi: 10.3390/f13010020
Tunyasuvunakool, K., Adler, J., Wu, Z., Green, T., Zielinski, M., Žídek, A., et al. (2021). Highly accurate protein structure prediction for the human proteome. Nature 596, 590–596. doi: 10.1038/s41586-021-03828-1
van den Berg, N., Christie, J. B., Aveling, T. A. S., Engelbrecht, J. (2018). Callose and β-1,3-glucanase inhibit Phytophthora cinnamomi in a resistant avocado rootstock. Plant Pathol. 67, 1150–1160. doi: 10.1111/ppa.12819
Vieites-Blanco, C., Colangelo, M., Camarero, J. J., Caballol, M., García Breijo, F. J., Štraus, D., et al. (2023). Pathogenicity of Phytophthora and Halophytophthora species on black alder and the host histological response. Mycol. Prog. 22, 71. doi: 10.1007/s11557-023-01923-3
Vít, P., Douda, J., Krak, K., Havrdová, A., Mandák, B. (2017). Two new polyploid species closely related to Alnus glutinosa in Europe and North Africa – an analysis based on morphometry, karyology, flow cytometry and microsatellites. Taxon 66, 567–583. doi: 10.12705/663.4
Vörösmarty, C., McIntyre, P., Gessner, M., Dudgeon, D., Prusevich, A., Green, P., et al. (2010). Global threats to human water security and river biodiversity. Nature 467, 555–561. doi: 10.1038/nature09440
Wang, Y., Wang, Y., Zhao, J. (2022). MGA-YOLO: alightweight one-stage network for apple leaf disease detection. Front. Plant Sci. 13. doi: 10.3389/fpls.2022.927424
Westbrook, J. W., James, J. B., Sisco, P. H., Frampton, J., Lucas, S., Jeffers, S. N. (2019a). Resistance to Phytophthora cinnamomi in American chestnut (Castanea dentata) backcross populations that descended from two Chinese chestnut (Castanea mollissima) sources of resistance. Plant Dis. 103, 1631–1641. doi: 10.1094/PDIS-11-18-1976-RE
Westbrook, J. W., Resende, M. F. R., Munoz, P., Walker, A. R., Wegrzyn, J. L., Nelson, C. D., et al. (2013). Association genetics of oleoresin flow in loblolly pine: discovering genes and predicting phenotype for improved resistance to bark beetles and bioenergy potential. New Phytol. 199, 89–100. doi: 10.1111/nph.12240
Westbrook, J. W., Zhang, Q., Mandal, M. K., Jenkins, V. E., Barth, L. E., Jenkins, J. W., et al. (2019b). Optimizing genomic selection for blight resistance in American chestnut backcross populations: a trade-off with American chestnut ancestry implies resistance is polygenic. Evol. Appl. 13, 31–47. doi: 10.1111/eva.12886
Wipfli, M. S. (1997). Terrestrial invertebrates as salmonid prey and nitrogen sources in streams: Contrasting old-growth and young-growth riparian forests in southeastern Alaska, USA. Can. J. Fish. Aquat. Sci. 54, 1259–1269. doi: 10.1139/f97-034
Wipfli, M. S., Musslewhite, J. (2004). Density of red alder (Alnus rubra) in headwaters influences invertebrate and detritus subsidies to downstream fish habitats in Alaska. Hydrobiologia 520, 153–163. doi: 10.1023/B:HYDR.0000027734.95586.24
Woodward, G., Gessner, M. O., Giller, P. S., Gulis, V., Hladyz, S., Lecerf, A., et al. (2012). Continental-scale effects of nutrient pollution on stream ecosystem functioning. Science 336, 1438–1440. doi: 10.1126/science.1219534
Zamora-Ballesteros, C., Haque, M. M. U., Diez, J. J., Martín-García, J. (2017). Pathogenicity of Phytophthora alni complex and P. plurivora in Alnus glutinosa seedlings. For. Pathol. 47, e12299. doi: 10.1111/efp.12299
Keywords: alder decline, environmentally friendly management, forest diseases, forest trees, oomycetes, riparian ecosystems
Citation: Cordeiro D, Pizarro A, Vélez MD, Guevara MÁ, de María N, Ramos P, Cobo-Simón I, Diez-Galán A, Benavente A, Ferreira V, Martín MÁ, Rodríguez-González PM, Solla A, Cervera MT, Diez-Casero JJ, Cabezas JA and Díaz-Sala C (2024) Breeding Alnus species for resistance to Phytophthora disease in the Iberian Peninsula. Front. Plant Sci. 15:1499185. doi: 10.3389/fpls.2024.1499185
Received: 20 September 2024; Accepted: 20 November 2024;
Published: 09 December 2024.
Edited by:
Rita Lourenço Costa, National Institute for Agricultural and Veterinary Research (INIAV), PortugalReviewed by:
Alfredo Cravador, University of Algarve, PortugalGlória Catarina Pinto, University of Aveiro, Portugal
Copyright © 2024 Cordeiro, Pizarro, Vélez, Guevara, de María, Ramos, Cobo-Simón, Diez-Galán, Benavente, Ferreira, Martín, Rodríguez-González, Solla, Cervera, Diez-Casero, Cabezas and Díaz-Sala. This is an open-access article distributed under the terms of the Creative Commons Attribution License (CC BY). The use, distribution or reproduction in other forums is permitted, provided the original author(s) and the copyright owner(s) are credited and that the original publication in this journal is cited, in accordance with accepted academic practice. No use, distribution or reproduction is permitted which does not comply with these terms.
*Correspondence: Carmen Díaz-Sala, Y2FybWVuLmRpYXpzYWxhQHVhaC5lcw==