- 1Laboratorio de Enzimología de Parásitos, Departamento de Biología, Facultad de Ciencias, Universidad de Los Andes, Mérida, Venezuela
- 2Dipartimento di Scienze e Tecnologie Ambientali Biologiche e Farmaceutiche, Università degli Studi della Campania, Caserta, Italy
- 3Grupo de Polímeros, Departamento de Química, Universidad de Los Andes, Mérida, Venezuela
- 4Department of Agricultural, Food and Environmental Sciences, Marche Polytechnic University, Ancona, Italy
The agricultural use of synthetic pesticides, fertilizers, and growth regulators may represent a serious public health and environmental problem worldwide. All this has prompted the exploration of alternative chemical compounds, leading to exploring the potential of chitosan and PGPB in agricultural systems as a potential biotechnological solution to establish novel agricultural production practices that not only result in fewer adverse impacts on health and the environment but also improve the resilience and growth of the plants. In this work, an analysis of the impact of plant growth-promoting bacteria (PGPB) and chitosan on plant growth and protection has been conducted, emphasizing the crucial bioactivities of the resistance of the plants to both biotic and abiotic stressors. These include inducing phytohormone production, mobilization of insoluble soil nutrients, biological nitrogen fixation, ethylene level regulation, controlling soil phytopathogens, etc. Moreover, some relevant aspects of chitin and chitosan are discussed, including their chemical structures, sources, and how their physical properties are related to beneficial effects on agricultural applications and mechanisms of action. The effects of PGPB and chitosan on photosynthesis, germination, root development, and protection against plant diseases have been compared, emphasizing the intriguing similarities and synergistic effects observed in some of these aspects. Although currently there are limited studies focused on the combined application of PGPB and chitosan, it would be important to consider the similarities highlighted in this work, and those that may emerge in future studies or through well-designed investigations, because these could permit advancing towards a greater knowledge of these systems and to obtain better formulations by combining these bioproducts, especially for use in the new contexts of sustainable agriculture. Thus, it seems feasible to augur a promising near future for these combinations, considering the wide range of possibilities offered by chitinous biomaterials for the development of innovative formulations, as well as allowing different application methods. Likewise, the studies related to the PGPB effects on plant growth appear to be expanding due to ongoing research to test on plants the impacts of microorganisms derived from different environments, whether known or recently discovered, making it a very exciting field of research.
1 Introduction
Bacteria present in the soil can have beneficial, neutral, or harmful effects on plants. Many rhizosphere bacteria have additionally demonstrated distinctive abilities to promote plant growth in environments that generate strong abiotic stresses, such as arid or seasonally dry regions, whose soils usually experience drought, salinity, or high temperatures (Khan et al., 2020). Likewise, some bacteria can promote plant growth in other extreme conditions, i.e., regions of high altitudes and low temperatures (Kadıoglu et al., 2018). Plant-associated bacteria that have a positive influence on the growth and development of plants are collectively called plant growth-promoting bacteria (Khatoon et al., 2022; Ajijah et al., 2023). They can promote plant growth directly, playing an active role in nutrient solubilization (e.g., P, K, Fe, Ca, and Mg) or atmospheric nitrogen fixation thus facilitating their acquisition, or producing phytohormones able to stimulate plant growth and development. In addition, they can act as biocontrol agents antagonizing other microorganisms that affect plant health and vigor. Among the responses they can generate are:
Mobilization of insoluble nutrients towards the rhizosphere through the secretion of compounds such as the so-called siderophores, which act as chelating agents for metals such as Fe (e.g.), making them more available for plants (He et al., 2020). They are produced especially by Gram-negative bacteria and can be classified according to their iron-binding moieties: carboxylate, catecholate, hydroxamate, and phenolate moieties). Siderophores which are specific for Fe(III), by forming stable aqueous complexes with it, display a similar or higher affinity for Mn(III) and other transition metals like Zn2+ or Cu2+ (Li et al., 2020b). In contaminated soils, siderophores can also chelate Cd2+ or other toxic metals like Al3+, Co2+, Pb2+, etc., reducing their bioavailability for plants and favoring their growth in these less favorable conditions (Guo et al., 2020). Strikingly, a similar mode of action has also been observed for materials with chelating activity such as chitosan (Heidari et al., 2020), with metal chelation standing out as a proposed mechanism to explain the protective role of chitosan favoring plant growth (Chakraborty et al., 2020). Chitosan metal-binding capacity increases at higher pH levels due to unprotonated amine groups, facilitating interaction with and absorption of metal ions (Abdellatef et al., 2022). On the other hand, the effects of chitosan as a chelating agent on plant growth under stress conditions, such as in the case of plants growing in soil contaminated by metals, are not easy to predict and depend on many factors. A good example of this situation is provided by the results obtained during the application of foliar treatments with chitosan to sunflower plants growing in soils “contaminated” separately with lead and cadmium (added as cations in aqueous solution and in equivalent quantities). While one of the treatments attenuates the effects of both cations on root length to the same extent (73%), a similar treatment, using a higher dose of chitosan, produces a lower attenuation of the effect of Pb (31%) than for Pb (75%) (Cartaya-Rubio et al., 2023).
Nitrogen-fixing bacteria, forming symbiotic relationships with agricultural plants and able to reduce atmospheric nitrogen (N2) to ammonium in a reaction catalyzed by nitrogenase, belong to various genera, including Azotobacter, Azospirillum, Bacillus, Bradyrhizobium, Pseudomonas, and Rhizobium (Zaidi et al., 2015). In rhizobia, flavonoid compounds released by legume roots interact specifically with the transcriptional regulator NodD whose activation triggers the expression of nodulation genes necessary for synthesizing lipo-chitooligosaccharides known as Nod factors. These latter, chitin-derivatives obtained by replacing the N-acetyl groups with fatty acids at the reducing ends, act as plant-specific bioactive molecules (Lerouge et al., 1990) signaling the establishment of the symbiotic association with legumes (Long, 1996). It is also interesting that arbuscular mycorrhizal fungi use these same types of molecules to initiate their symbiotic relationship with plants (Limpens et al., 2015).
Induction of the production of phytohormones such as gibberellins (GAs) and auxins, among which indole-acetic acid (IAA) stands out. In some cases, such as with Pseudomonas monteilii, it has been found that induction of IAA synthesis can be increased when other materials are added, such as chitosan nanoparticles (Panichikkal et al., 2022). The excessive application of chitosan in the rhizosphere of Arabidopsis has been also observed to cause stress in these plants, which is made evident by the arrest in the root growth due to the accumulation of auxins, mainly IAA (Lopez-Moya et al., 2019). Therefore, it is extremely important to control the concentrations of chitosan used in agricultural practices to inhibit the growth of plant pathogens, while simultaneously favoring the proliferation of beneficial organisms without compromising plant growth.
Modulation of ethylene (C2H4), a gaseous phytohormone playing a pivotal role in regulating several aspects of plant development and physiology, such as seed germination, cell expansion, leaf and petal abscission, fruit ripening and abscission, organ senescence, and responses to environmental stresses (Iqbal et al., 2017). Moreover, ethylene influences tissue differentiation, root and shoot formation, and branching and root elongation (Glick, 2014). Thus, during the establishment of symbiotic plant-rhizobium or plant-mycorrhizal fungus relationships, a local decrease in ethylene concentration may occur facilitating symbiosis (Gamalero and Glick, 2015). On the other hand, chitosan has also been shown to have a regulatory effect on the production of ethylene in plants, in some stages of their agricultural production (Czékus et al., 2021; Prusky and Romanazzi, 2023). Thus, its application has a notable influence on the maturation of some climacteric fruits, both in pre- and post-harvest treatments. For instance, the ethylene concentration in banana (Musa acuminata) fruits sharply increases at the onset of their climacteric period, leading to a rapid ripening process with the consequent decrease in fruit shelf life. However, the postharvest application of chitosan as an edible coating on fruits allows them to extend their shelf life and maintain their sensory quality (Lustriane et al., 2018; Romanazzi and Moumni, 2022). Likewise, it has recently been found that a similar treatment with chitosan 1.25% w/v on banana fruits, with storage for 11 days, led to a distinctive accumulation of the metabolite 1-aminocyclopropane-1-carboxylic acid (ACC), the immediate precursor of ethylene, which is responsible for the ripening process of climacteric fruit (Parijadi et al., 2022).
Biological control of soil plant pathogens, competing with them for space and nutrients, either by sequestering essential metals for their development (Olanrewaju et al., 2017), or by releasing substances toxic to them, which may include enzymatic proteins such as chitinases (Velásquez et al., 2019; Liu et al., 2019a), or antimicrobial peptides such as bacteriocins, i.e., turicin (Cesa-Luna et al., 2020), or other antibiotics (Matilla and Krell, 2017). On the other hand, one of the control mechanisms of phytopathogenic microorganisms usually associated with chitosan has been precisely the chelation of metals necessary for plant pathogens, especially those associated with molecules present in the cell walls of bacteria (Chandrasekaran et al., 2020).
Similarly, and as briefly outlined in the previous part, chitosan and some of its related materials can also produce stimulating effects on plant growth without compromising environmental sustainability (Shahrajabian et al., 2021), including in many cases a significant improvement in plant resilience under stress conditions (Hidangmayum et al., 2019; Malerba and Cerana, 2020).
Thus, in general terms, it has been stated that both chitosan and PGPB have adequate potential to enhance agricultural productivity in many current stressed environments and in future more extreme scenarios that are predicted due to climate change. In this work, a review of different studies related to the effects of PGPB and chitosan (usually applied independently) on plant growth is carried out. It is intended to organize information that can help to justify some similarities observed in the bioactivities of PGPB and chitosan, which had gone unnoticed. Thus, the knowledge derived from studies of this type can help develop strategies that use these combined systems, which should be very useful in the new contexts of sustainable agriculture, especially under stress conditions.
2 Chitosan and its beneficial effects on plants
Chitosan is the main derivative of chitin, one of the more abundant natural biopolymers. Chitin is present in a wide diversity of organisms, including insects, crustaceans, mollusks, fishes, fungi, algae, etc (Lárez-Velásquez, 2023), and its deacetylation process yields chitosan (Figure 1). The beneficial effects of chitin and chitosan on agricultural uses have been known for a long time (Mitchell and Alexander, 1961; Nichols and Hadwiger, 1979). The main modes of action of these biopolymers in agricultural applications have been profusely reviewed (Velásquez et al., 2019; Riseh et al., 2022; Boamah et al., 2023) and some attempts are being made to organize the accumulated knowledge on the subject (Velásquez et al., 2019; Shamshina et al., 2020). Figure 2 schematically shows the main proposed modes of action by which chitinous materials promote beneficial effects in plants.
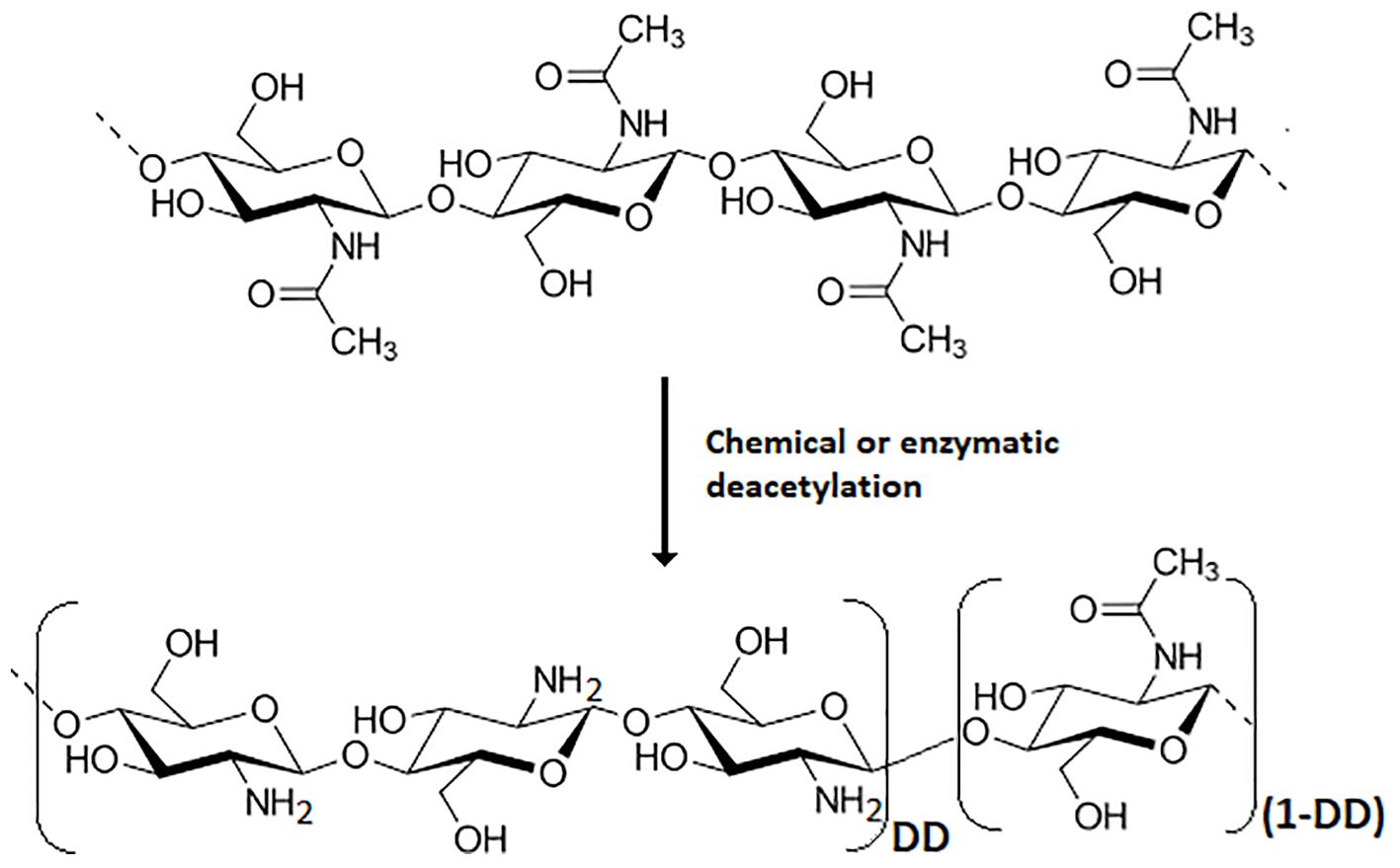
Figure 1. Chemical structure of chitin and its usual deacetylation reactions to obtain chitosan, which is defined by a deacetylation degree (DD) ≥ 50%.
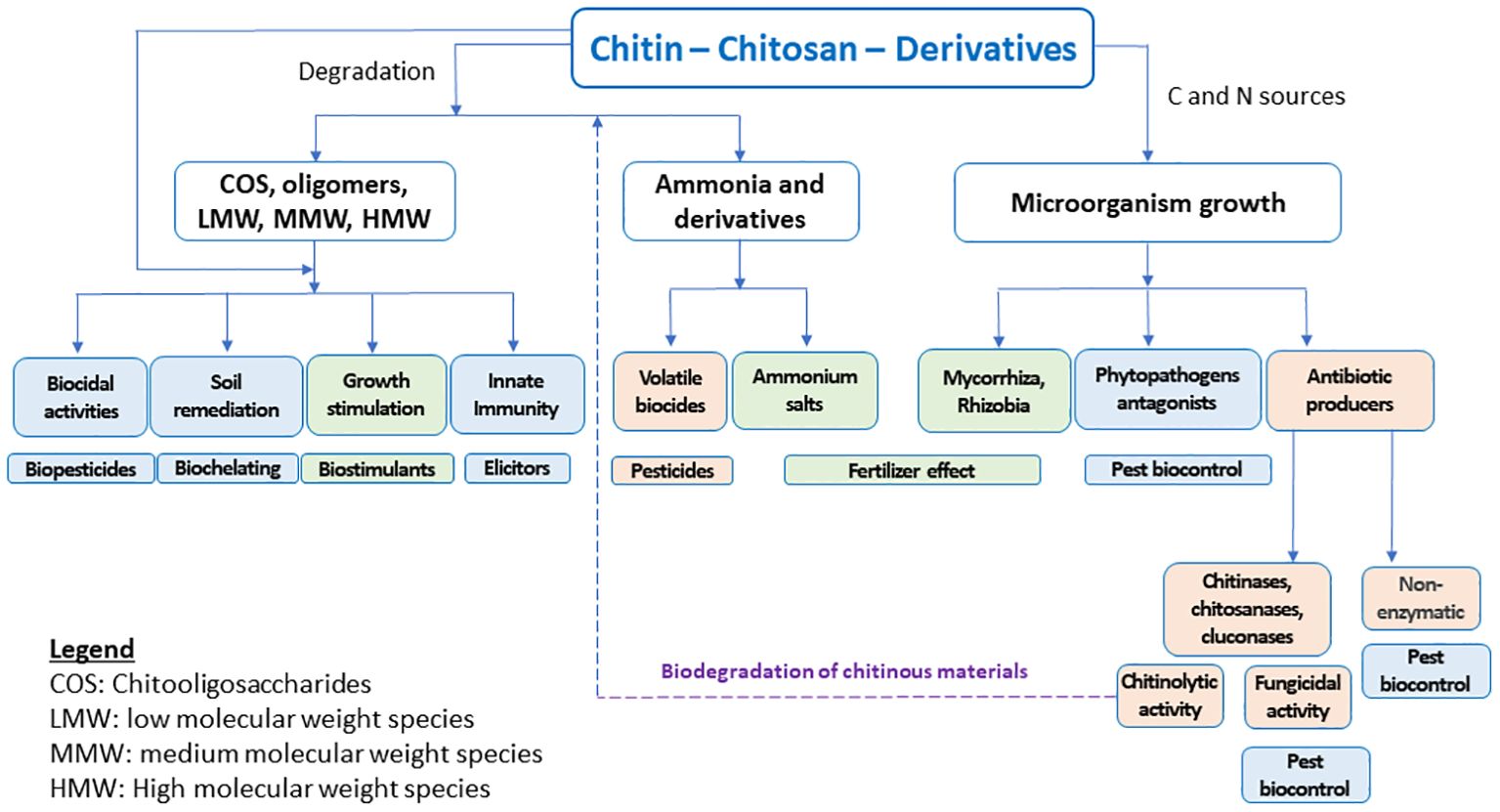
Figure 2. The main modes of action associated with the protection and plant growth provided by chitinous materials.
In general, there are three types of factors that affect the bioactivity of chitosan: (a) those related to the chitosan molecules themselves such as the source and method employed to obtain it, the molecular weight (MW), the degree of deacetylation (DD), the acetylation pattern (AP), the polyelectrolyte associated counterion, the concentration, application method), (b) those associated with the kind and development stage of the pathogenic organism subjected to its action (bacteria, virus, fungi, insects) and (c) those that have to do with the environment where the processes are carried out (pH, temperature, ionic strength, presence of others chemical substances or live organisms, etc.). A few examples of proven agricultural applications, and the underlying mechanisms of action, are the following:
Phytopathogen control: Chitosan biocidal activity against phytopathogens has been amply proven and reviewed (Ke et al., 2021; Riseh et al., 2022), with different mechanisms having been proposed to explain its effects. Its applications have been studied to control diseases caused by viruses, bacteria, fungi, insects, etc., and its biocidal action has been found to depend largely on its cationic polyelectrolyte properties (acid medium). The main mechanisms proposed for its bioactivities concerning diseases caused by fungi are the disruption or alteration of the fungal plasma membrane, the alteration of gene expression, the inhibition of RNA and protein synthesis, and the blocking of Ca2+ channel (Lárez-Velásquez and Rojas-Avelizapa, 2020; Karamchandani et al., 2022). Chitosan control of bacterial phytopathogens has also been profusely proven (Badawy and Rabea, 2016; Khairy et al., 2022), with reports indicating higher effectivity for Gram-negative than for Gram-positive bacteria (Tarakanov et al., 2023).
Soil conditioning and bioremediation: Chitin is a recognized amendment that, in addition to providing nitrogen, can help in soil conditioning by freeing it from nematodes, either by promoting the growth of chitinolytic microorganisms that feed on their eggs or by killing them with the ammonia, or its derivatives, released during its decomposition (Sharp, 2013). In turn, metal chelation stands out as one of the proposed mechanisms to explain the protective role of chitosan on plant growth (Chakraborty et al., 2020), including phytoremediation species (Shirkhani et al., 2021).
Biostimulation and preservation of seeds: chitosan-based coatings have been applied to seed protection (Moumni et al., 2023). This type of application is not limited only to taking advantage of its fungicidal activity (Chookhongkha et al., 2013), or its bioactivity as a germination elicitor (Khaptsev et al., 2021), but can additionally serve as a vehicle for the controlled release of other agrochemicals (Godínez-Garrido et al., 2021), and, even though it has been little used for preparation of synthetic seeds (Kulus, 2019), seems to have the necessary potential for it.
Inducer of resistance to diseases caused by phytopathogens: chitosan is a recognized elicitor of the acquired resistance system of plants (Singh et al., 2018) through a phenomenon known as defense priming. Studies on these topics include the induction of suberization during the wound healing process in potato tubers (Liu et al., 2019b), the production of secondary metabolites, including phytoalexins (Eilenberg et al., 2010), lignin (Ali et al., 2014), phenolic compounds (Acemi et al., 2021), among others. These activities have been reported to be controlled by MW and DD of the applied chitosan (Hua et al., 2019).
Growth biostimulant: chitosan has been extensively explored in agriculture as a potent bio-stimulant, with many successful applications (Shahrajabian et al., 2021). Thus, its effect on the development of the root system under water deficiency is noticeable (Almeida et al., 2020). Likewise, chitosan remarkably promotes the production of secondary metabolites in plants, i.e., the menthol production in mint crops (Goudarzian et al., 2020). Other successful applications include the foliar treatment of tomato crops under salt stress, using solutions prepared with citric or ascorbic acid, which increases the production of some metabolites and osmolytes, thus helping to reactivate their developments (Attia et al., 2021).
Postharvest protection: chitosan applications can simultaneously fulfill diverse functions such as antimicrobial agent, elicitor, physical isolation (given its ability to form semi-permeable films) (Romanazzi et al., 2018). Likewise, chitosan mixtures with other natural products have successfully been studied seeking synergistic effects and reducing the use of synthetic substances, i.e., chitosan/Aloe vera gels to delay the post-harvest decay of mango fruits (Shah and Hashmi, 2020) and chitosan/carnauba wax/oregano oil to protect cucumber (Gutiérrez-Pacheco et al., 2020).
Finally, to close this brief section on chitinous materials and their main agricultural uses, it is necessary to mention their oligomeric derivatives, better known as "chitooligosaccharides" (COS). These materials have attracted attention in recent years due to the development of new methods to prepare some of them with controlled chemical structures (Sreekumar et al., 2022), which has opened the door, with great expectations, for the understanding of which structures induce specific bioprocesses in pathogens and plants, as well as in other areas such as medicine, i.e., for the treatment of cancer (Sun et al., 2024).
3 PGPBs and chitosan to promote plant growth under stress conditions
Some PGPBs generate more specific responses than those mentioned in the previous section, i.e., the production of exo-polysaccharides and/or compatible osmolytes such as sugars (threose), amino acids (proline), betaines, etc., to preserve cellular osmotic balance (Selim et al., 2019). Usually, these PGPBs are known for their capacity to enhance plant tolerance to various types of common abiotic stresses. Likewise, chitosan has shown positive effects on the growth of plants under stress conditions, and its beneficial effects have been reported to alleviate saline stress when used as part of nanomodified biomaterial systems (Balusamy et al., 2022). Similarly, osmo-protection effects have been reported during chitosan foliar treatment of Puccinellia distans, a grass that usually grows in high salinity conditions. These positive effects were more noticeable under drastic saline conditions (Oddo et al., 2019). Some comparisons of the effects of chitosan and PGPB on processes associated with promoting plant growth are shown in Figure 3.
3.1 Photosynthesis
Under stress conditions, the plant photosynthetic process can be severely affected by secondary osmotic and oxidative stress, decreasing transpiration and gas exchanges, and damaging membranes and cell organelles, altering the electron transport process and further generating reactive oxygen species (Hasanuzzaman et al., 2021). Thus, in drought conditions, plants experience a decrease in their chlorophyll content (Ghotbi-Ravandi et al., 2014), among other factors; this triggers an upregulation mechanism to synthesize chlorophyll in greater quantities as a usual strategy of plants to increase their tolerance to drought while reduces the amount of recent photosynthates exported to other organs (Fang and Xiong, 2015). This, in turn, causes changes in the plant organ's metabolic profile, as evidenced in various studies. In this sense, Tripathi et al. (2016) have identified up to 163 metabolites changing their abundance in the roots of plants subjected to water stress after removal from a hydroponic system. From the foregoing, it can be inferred that a diversity of compounds participates in processes such as the maintenance of photosynthesis and cell osmolarity, the slowing down of leaf senescence, and the promotion of root growth, which allow the plants to survive under stress conditions. Moreover, the different allocation of photosynthates, along with the need for osmoregulation, leads to a re-modulation of metabolic pathways. Among the new compounds synthesized and accumulated, sugars, polyols, amino acids, alkaloids, ions, etc., can be mentioned, which are usually produced by upregulated processes (Naylor and Coleman-Derr, 2018). Likewise, an increase in the synthesis of polymers in the cell walls is also generated, which helps to strengthen them and maintain cell turgor (Le Gall et al., 2015). Accordingly, some transcriptomic studies have identified numerous cell wall modifier genes, including some related to xylotransferases involved in the biosynthesis of xyloglucans, which appear upregulated in plants under stress (Tenhaken, 2015). Other biopolymers such as expansin, pectin, lignin, and suberin have also been reported to be upregulated under such conditions (Naylor and Coleman-Derr, 2018).
Some authors have reported the beneficial effect of PGPB on photosynthesis, mainly due to the stimulation of chlorophyll production and photosynthetic efficiency in plants (Erman et al., 2022; Katsenios et al., 2022). In addition, PGPBs produce siderophores that facilitate the capture of metal micronutrients, such as iron (Fe3+) and phosphorus (P) (Souza et al., 2015), essentials for the photosynthesis process (Schmidt et al., 2020; Kayoumu et al., 2023) (Figure 3A).
Regarding the chitosan effects on photosynthesis, it has been proposed that this biopolymer and its oligosaccharides improve photosynthesis by increasing the activities of various enzymes related to carbon and nitrogen metabolism, as well as the light-dependent and -independent photosynthetic reactions (Ahmed et al., 2020) (Figure 3B). Likewise, it has been reported that chitin and chitosan hexamers promote a higher rate of photosynthetic reactions (Li et al., 2020a). In the case of the Fabaceae known as fenugreek (Trigonella foenumgraecum), pretreatment of the seeds with chitosan mitigates the effect of salt stress during plant growth, as it was determined by assessment of the relative water content and photosynthetic pigments (Mosapour-Yahyaabadi et al., 2016). Nevertheless, some contradictory results have also been reported regarding the effects of chitosan on photosynthesis, which could be attributed to some factors such as the method and timing of treatment application (Hidangmayum et al., 2019), which are critical in agricultural applications of this material. Likewise, it is also important to consider the characteristics of each applied chitosan because these can exert a notable influence on the results obtained. As previously indicated, appreciable differences in bioactivities may be observed in chitosan of a “similar” composition (Lárez-Velásquez and Rojas-Avelizapa, 2020).
Further promising positive effects of chitosan on photosynthesis in plants under abiotic stresses include the induction of resistance to conditions of water deficit, as evidenced by the increase in photosynthetic rate and harvest yield in maize plants foliar sprayed with N-succinyl chitosan and N,O-dicarboxy-methylated chitosan, or their combination (Rabêlo et al., 2019). Chitosan has also been used as a carrier for the controlled release of biostimulants to induce plant resistance to different stresses. One such application is the formulation of chitosan-selenium nanoparticles and their application to mitigate cadmium stress in Balsam of Moldavia (Dracocephalum moldavica) plants. After treatment, an increase in photosynthetic pigments, among other parameters studied, was observed (Azimi et al., 2021). This study showed, additionally, that even when the application of chitosan alone does not produce beneficial effects, a synergistic effect occurs when the two components are used together in the form of nanoparticles. In addition, chitosan alleviates water stress in plants by inducing the production of secondary metabolites, such as γ-aminobutyric acid (GABA) (Geng et al., 2020), a key factor for osmotic adjustment and antioxidant response in plants subjected to abiotic stresses (Carillo, 2018). GABA synthesis, catalyzed by glutamate decarboxylase (GAD), consumes protons, buffering acidosis and regulating cytoplasmic pH under stress conditions. It acts as a ROS scavenger, playing a crucial role in protecting and stabilizing macromolecules, proteins, and membranes. Moreover, upon relief from stress conditions, it exerts an anaplerotic function via the GABA shunt, being converted into Krebs cycle intermediates, thus enhancing the production of NADH and ATP (Ciriello et al., 2024).
3.2 Germination
Seed pretreatment with PGPB can promote germination and seedling development in seedbeds (Widawati and Suliasih, 2018; Raja et al., 2019; Rozier et al., 2019). It is known that phytohormones, such as abscisic acid, IAA, GAs, ethylene, cytokinins, brassinosteroids, etc., produced by plants or PGPBs, significantly affect the germination process either positively or negatively. They interact synergistically thus exerting their effects, i.e., some plant genes are necessary for the activity of a phytohormone, while other genes are activated by phytohormones. Since PGPBs play an important role in the production of phytohormones (Bákonyi et al., 2013), they can be used as effective treatments to promote germination and, therefore, crop production (Miransari and Smith, 2014) (Figure 3A). Similarly, the positive effects of chitosan on seed germination have been known for decades (Jiang et al., 1994) and have been extensively documented in recent times (Divya et al., 2019; Li et al., 2019; Godínez-Garrido et al., 2022), including reports with positive results for germination under stress conditions (Odat et al., 2021). Despite this, contrasting findings have also emerged in recent studies, depending on the degree of acetylation and the concentration of chitosan used (Gün Polat et al., 2022). Likewise, it has been found that chitosan can favor some parameters related to rate and time of germination (Figure 3B) and has no positive effects on others (percentage of germination), as observed, for example, during the germination of maize seeds under controlled low-temperature stress conditions (Guan et al., 2009).
3.3 Effects on the roots
Some PGPBs can significantly affect the growth and root architecture of plants under drought stress, improving the plant's ability to uptake water and nutrients, as also observed in plants treated with bio-products such as chitosan (Hidangmayum et al., 2019) (Figures 3A, B). For PGPB, the improvement of the root system could be related to the bacterial production of phytohormones, as reported for some species of bacteria producing IAA in green soybean or mung bean crops (Chandra et al., 2018). However, it is also important to consider the possible effect of other naturally occurring factors, i.e. chitin oligosaccharide present in the soil, able to act as bio-stimulants and exert a positive effect on root development (Winkler et al., 2017). Similarly, various studies have reported the positive effects of PGPBs on enhancing plant growth under conditions of metal stress that are usually found in contaminated soils, which usually affect the root system (Figures 3A, B). Some of these studies are summarized in Table 1.
Although studies on the effect of chitosan on root growth have yielded contradictory results, some showing a decrease in root growth in crops of plants with adventitious roots (Baque et al., 2012), and opposite results in plants inoculated with the nematode Meloidogyne incognita (Khalil and Badawy, 2012). In other studies, it has been proposed that the effect of chitosan on root growth is dependent on the mode of application (dose, frequency, formulation, etc.). Indeed, chitosan represents a useful experimental tool to manipulate root development, among other parameters associated with plant growth (Lopez-Moya et al., 2017). Results from other investigations corroborate this proposition. Studies on Prunus davidiana seedlings evidenced that root growth was proportional to the concentration of carboxymethyl chitosan added up to a concentration of 2 g/L, whereas root growth decreased at higher values (Xu et al., 2020). Furthermore, in studies with corn seedlings exposed to cadmium stress, chitosan treatment significantly increased the growth rate of the root system and compensated for its functional impairments (Qu et al., 2019). Table 1 also summarizes several observations from studies on the efficacy of chitosan in relieving metal stress usually found in contaminated agricultural soils.
3.4 Protection against diseases
Among the biotic factors that produce the greatest stress in the plants, fungal diseases pose a significant threat due to the significant losses that they can cause in the yield of the crops, in addition to the potentially toxic compounds produced during their infection (Adeyeye, 2016). A recent study with isolates of endophytic bacteria (Bacillus velezensis and Pseudomonas putida) demonstrated their significant ability to inhibit the growth of Magnaporthe oryzae (Do, 2022), a phytopathogenic fungus responsible for the blast infection in rice, an essential crop for human food security. The positive effects of some PGPBs, such as Bacillus subtilis, have also been reported on plants facing biotic stress caused by other plant pathogens such as Fusarium oxysporum and Rosellinia necatrix (Hashem et al., 2019). Bacillus subtilis can generate biofilms on plant roots, and chitosan has excellent filmogenic properties, creating barriers against some plant pathogens (de Oliveira and de Oliveira Junior, 2020) (Figure 3A). Additionally, some positive results from PGPB studies have been reported regarding the control of weeds associated with crops such as wheat (Abbas et al., 2017; Mustafa et al., 2019).
Similarly, the fungicidal activity of chitosan has been studied for a long time (Allan and Hadwiger, 1979; Romanazzi et al., 2018). In a study comparing the effects of separate treatments with PGPBs and chitosan on the inhibition of the growth of the pathogen responsible for root rot in tomatoes, under similar growth conditions, high molecular weight chitosan was more effective (El-Mohamedy et al., 2013). Unfortunately, combinations of both treatments were not tested in this study. Furthermore, the antiviral and nematocidal activities of chitosan have also been widely recognized (Abd El-Aziz and Khalil, 2020).
3.5 Synergistic effects
At this point, it is important to consider that studies based on combined treatments of chitosan and PGPB have been scarce. However, the few studies carried out have found significant synergistic effects between PGPB and chitosan. These studies have shown that the combination of both not only improves disease resistance but also nutrient absorption and plant growth, as well as resistance to different stress conditions. This combined approach seems to take advantage of the individual properties of both agents to offer greater benefits than those achieved when used individually, offering, in this sense, an effective alternative to the chemicals usually used in current agriculture. Some systems that have shown synergistic effects for chitosan and PGPB are discussed below.
3.5.1 Improved disease resistance
A PGPB consortium, formed by Azotobacter vinelandii and Bacillus megaterium adsorbed on chitosan nanoparticles (PGPB/npChitosan), alleviates the Fusarium oxysporum infection damage in Solanum lycopersicum (tomato) and Citrullus lanatus (watermelon) plants (Pavlicevic et al., 2023), which has been attributed to the PGPB/npChitosan stimulating increased expression of stress-related genes and the activity of antioxidative enzymes in both plants, compared to treatments with PGPB-free or only npChitosan. Additionally, the observed synergy in this system could be also promoted by the following contributions: (a) PGPBs can directly inhibit pathogens through the production of antimicrobial metabolites and antibiotics (Ngalimat et al., 2021), i.e., Azotobacter species usually produce antibiotics with a structure like anisomycin, a well-known fungicidal agent (Sumbul et al., 2020), as well as other metabolites exhibiting antifungal activity (Chetverikov and Loginov, 2009); in addition, Bacillus megaterium is recognized as an antibacterial and antifungal antibiotic producing PGPB (Xie et al., 2021); (b) chitosan reduces the severity of the infection caused by Fusarium oxysporum by negatively regulating the expression of at least 62 genes in this pathogen, many of which are involved in processes such as plant cell wall degradation, protein, and DNA biosynthesis, as well as some transport of proteins, has also been reported (Elagamey et al., 2022); in addition, it, acting as a bioinoculant carrier, contributes to greater long-term survival of PGPB, enhancing their competition. In fact, it has been documented that chitosan macro beads supported the bacteria survival for over a year, demonstrating its potential as a material for the development of supporting biofertilizers (Perez et al., 2018; Fernández et al., 2022).
Likewise, PGPB-chitosan treatments have also been evaluated in the clubroot disease, caused by the obligate protist Plasmodiophora brassicae Woronin (Kang et al., 2024). Although the bacteria Paenibacillus polymyxa ZF129 is known to be effective in controlling clubroot disease, it has limited stability in the soil; interestingly, when P. polymyxa ZF129 cells were immobilized in chitosan/carrageenan macro-beads (Chit/Carrg, ratio 1/1) a greater survival rate was observed and the control efficiency of ZF129 against clubroot disease improved markedly, with the disease index decreasing from ~94 to ~22, corresponding to a control efficacy around 76. Also, the control index of disease control, which was significantly higher than treatment with ZF129 culture alone (59.76 ± 4.43 %), showed that macro-beads improved the control efficiency of ZF129 culture on clubroot by 16.57 %. Thus, the primary contribution of Chit/Carrg is the protection of the bacterial inoculum from adverse soil conditions and native microflora, facilitating its successful establishment in the rhizosphere and ensuring its effectiveness for a longer period.
Similarly, the encapsulation of Bacillus licheniformis in alginate-chitosan nanoparticles supplemented with rice starch had greater antifungal activity against the Sclerotium rolfsii infection of Capsicum annuum (chili) plants (Panichikkal et al., 2022). These findings demonstrate that the encapsulates of PGPB bacteria with bioactive polymers such as chitosan are phytopathogen biocontrol systems with promising opportunities in current agriculture.
3.5.2 Optimized nutrient uptake
The combined impact of PGPB and chitosan on nutrient uptake by Zea mays L. (corn) has been investigated by Agbodjato et al. (2016). This study unveiled that the Azospirillum lipoferum-Pseudomonas fluorescens-Pseudomonas putida-chitosan combination increases the nitrogen and potassium content in the aerial biomass of maize plants, compared to the control and treatment involving only A. lipoferum and P. fluorescens. Notably, the nitrogen content in the aerial part of the plants increased up to 41.61% compared to the control, after the soil inoculation with the combination of the three bacteria and chitosan. Furthermore, this treatment also induced an increase in potassium content of 6.34% and 27.16% in the aerial and underground parts of the maize plant, respectively. In summary, this combination of PGPB-chitosan has shown promising results in enhancing the mineral nutrition of maize plants, particularly in nitrogen and potassium uptake, which could be related to the ability of PGPB to fix nitrogen, solubilize phosphate, and enhance the availability of other mineral nutrients, thereby making them more accessible and substantially promoting the plant growth and yield (Hasan et al., 2024). On the other hand, chitosan can contribute to soil structure improvement. Particularly, studies using chitosan with lower MW and higher DD demonstrated to reduce porosity, increase mechanical stability, and enhance the wettability of soils; these effects, linked to the creation of electrostatic bonds and/or adhesive bonds between positively charged chitosan particles and negatively charged soil components, results in heightened mechanical stability and water retention, thereby aiding in the retention and availability of nutrients (Adamczuk and Jozefaciuk, 2022). Alternatively, chitosan and these bacteria could facilitate, independent or combined, nutrient uptake by chelating certain minerals, such as Cu and Mn (Verma and Quraishi, 2021), essential for nutrition from plants (White and Brown et al., 2010).
3.5.3 Alleviating stress
In plants, salt stress induces a variety of physiological and metabolic alterations. Some processes, such as germination, photosynthesis, and growth are significantly affected (Hasanuzzaman and Fujita, 2022). The synergy between chitosan and PGPR significantly enhances plant resistance to salt stress. In a study where chitosan-immobilized Methylobacterium oryzae CBMB20 was employed as a bioinoculant to improve Solanum lycopersicum plant growth, under salt stress, an effective increasing in the plant dry weight was observed. The oxidative stress induced by salinity was also alleviated by up regulating the activities of antioxidant enzymes. Additionally, the accumulation of osmolytes such as proline was observed, along with a reduction in the excess of some Na+ influx into plant cells under these stress conditions, compared to both the control group and treatments involving only M. oryzae CBMB20 (in free form) and only chitosan (Chanratana et al., 2019). These effects could be explained by some of the mechanisms proposed for the relief of salt stress in plants by PGPB and chitosan (Kumar et al., 2020; Alenazi et al., 2024). Thus, chitosan can improve the resistance of different crops to diverse abiotic stresses by modulation of signaling pathways, i.e., the application of nano chitosan in certain crops leads to an increase in antioxidative metabolism, resulting in elevated activities of the antioxidant enzymes such catalase, superoxide dismutase, peroxidase and glutathione, reductase (Quitadamo et al., 2021; Alenazi et al., 2024); in addition, chitosan influences the expression of several genes, including mitogen-activated protein kinases, geissoschizine synthase, and octadecanoid-derivative responsive AP2-domain genes, which play relevant roles in salt stress response and alkaloid accumulation (Hassan et al., 2021). Furthermore, inhibition of pathways such as lipid peroxidation has been proposed as a mechanism by which chitosan alleviates salt stress (Peykani and Sepehr, 2018).
On the other hand, a treatment using Bacillus thuringiensis (seed treatment) and chitosan (foliar application) on sweet pepper plant grown under different salinity regimes led to improve some growth factors such as chlorophyll content, chlorophyll fluorescence parameter, fruit yield, and to reduce lipid peroxidation and electrolyte leakage in stressed plants; similarly, the proline accumulation and enzyme activity was regulated as well as increased the number of fruit plant, fruit fresh weight plant, and total fruit yield of sweet pepper grown under saline conditions (ALKahtani et al., 2020).
3.5.4 Stimulation of root growth
The combined application of PGPR and chitosan has been evaluated in some studies (Mukta et al., 2017; Ortega-García et al., 2021). For instance, studies involving plants of Asparagus officinalis L., bio-fertilized with a combination of the halobacteria-PGPB Bacillus amyloliquefaciens and chitosan, showed a remarkable increase in the size and weight of the roby approximately 69% and 25%, respectively, compared to non-treated control and individual treatments of B. amyloliquefaciens and chitosan This demonstrates that the synergy between B. amyloliquefaciens and chitosan positively influenced vegetative development. Part of these outcomes are attributed to the capability of B. amyloliquefaciens to produce growth-promoting substances (Ortega-García et al., 2021), particularly hormones such as gibberellic acid, auxin, and IAA, as well as volatile organic compounds like 2,3-butanediol and acetoin, associated whit the modulation of some processes such as cell division and expansion, as well as promoting lateral root development (Chen et al., 2007; Luo et al., 2022). In turn, chitosan, also induces an augmentation in the length of the primary root, along with increases in fresh weight and dry weight of the root, and, additionally, it has been reported to regulate the root architecture system by increasing the diameter of the root tip and root forks (Jiao et al., 2024). These structural changes imply that chitosan accelerates the development of the root system, thereby improving the absorption rate and transport capacity of water and nutrients necessary to sustain normal plant growth. It is also important to note that chitosan can induce the production of some hormones as IAA and GA in the roots. Moreover, the induced accumulation of IAA may modulate the expression of other genes involved in tryptophan biosynthesis (Mukarram et al., 2023), an amino acid known to promote the development of adventitious roots (Bergonci and Paponov, 2022). Thus, taking everything together, it seems clear that PGPB and chitosan act synergistically to promote the development of a more extensive root system, which is also more efficient in the absorption of nutrients, thus facilitating plant growth.
4 Trends in the use of PGPBs and chitosan to increase plant growth and tolerance to diverse types of stress
There is currently a strong trend toward the study of biostimulants to promote plant growth, which includes PGPBs and chitosan due to their potential to enhance plant growth under biotic and abiotic stress conditions. Figure 4 depicts this pattern for the last ten years, showing a significant increase in the number of annual papers retrieved from the Google Scholar database when “chitosan abiotic stress” and “PGPB PGPR abiotic stress” were used as search words. Due to the potential of both to improve the resistance of plants growing under stress conditions, their interactions to improve the response of plants to such conditions researchers have begun to attract attention (Riseh et al., 2021). Furthermore, there is interest in designing green systems for the encapsulation of microbial inoculants using chitosan and other polysaccharide matrices (Perez et al., 2018; Vassilev et al., 2020).
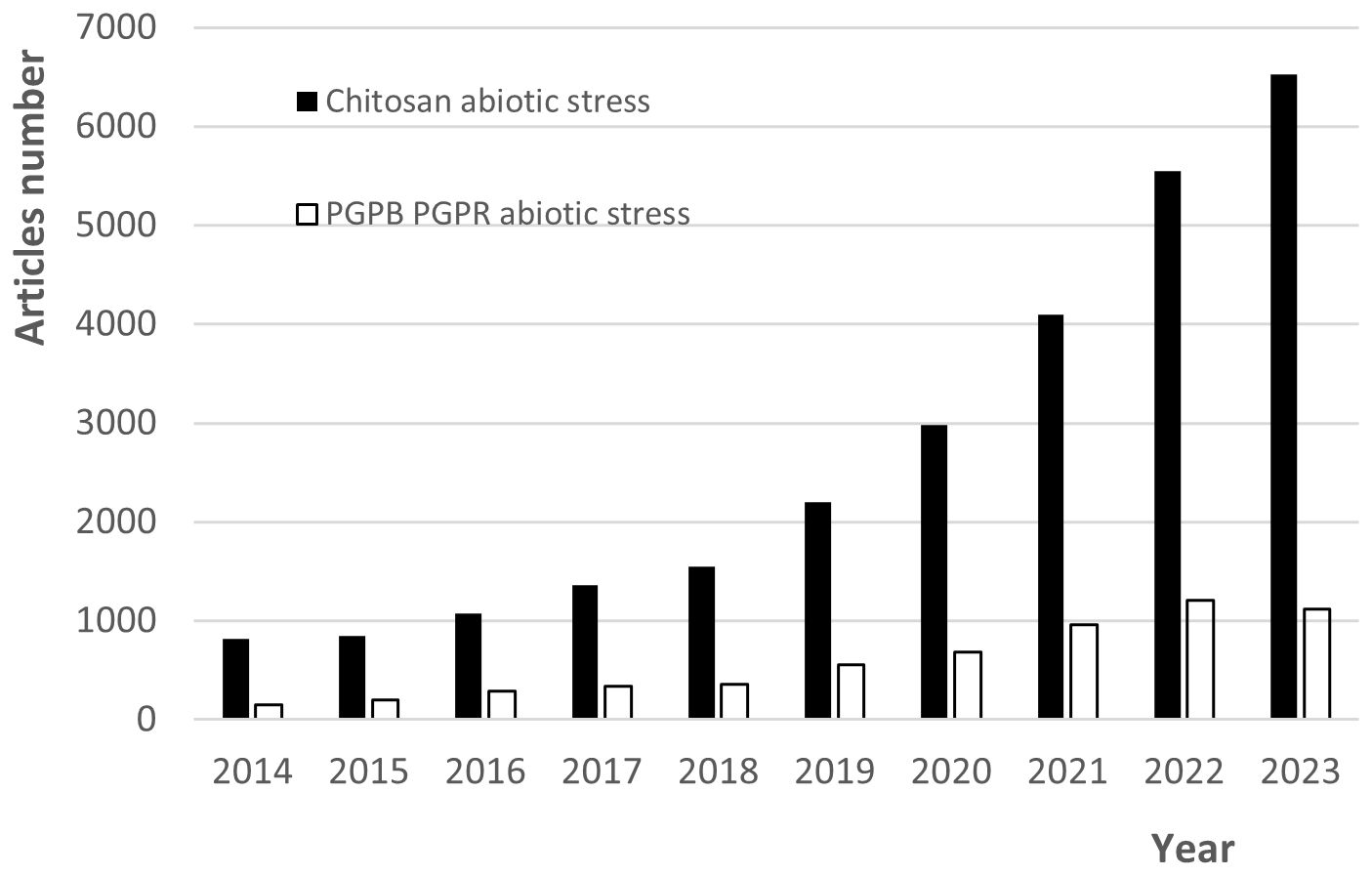
Figure 4. Trends over 10 years for the number of annual papers found in the database “Google Scholar” when “chitosan abiotic stress” and “PGPB PGPR abiotic stress” were used as search words (updated 22 April 2024).
On the other hand, although there are only a few reported studies on the combined applications of PGPB and chitosan as plant growth promoters, their positive results permit to augur a promising near future for these combinations. In this regard, the wide range of possibilities that chitinous biomaterials offer for the development of innovative formulations (in addition to allowing different application methods) as well as the growing expansion of studies related to the impacts on plants of microorganisms obtained from various environments, known, or recently discovered, make this a very exciting field of research.
5 Concluding remarks
The above considerations allow infer that the combined treatments of chitosan with PGPBs, and other microorganisms, may enable new strategies to promote plant growth, especially since there is a wide range of possibilities for such combinations, encompassing chitin and chitosan, and including many of its derivatives, as well as different PGPBs. For the application of chitin and chitosan, variations of their traditional parameters could be considered, such as their origin, methods of preparation and chemical modification, molecular weights, degrees of acetylation or substitution, distribution of the N-acetyl groups along chain length, acids used for its dissolution, application methods (powder, solutions, spray, hydrogels, encapsulation matrix for microorganisms, etc.). Its triple activity as an antimicrobial agent, elicitor and film former should also be considered because it guarantees a good initial level of effectiveness.
Regarding PGPBs, the studies related to its effects on plant growth seem to be expanding, especially due to ongoing research to test on plants the impacts of microorganisms derived from different environments, whether known or recently discovered. Importantly, some studies have also shown that PGPB populations can also be significantly reduced in soils under adverse conditions, prompting new strategies to protect them. Among these strategies, the encapsulation of PGPBs using biomaterials enabling them to retain their viability and stability during the production, storage, and application processes in agricultural settings, has emerged as one of the most efficient approaches to protect PGPBs after inoculation into the soil. In this context, the encapsulation of microorganisms useful in agricultural applications, i.e., by using polysaccharides such as chitosan and its derivatives (Rojas-Pirela et al., 2021; Meyer-Déru et al., 2022), emerges as one of the most active areas of research in the foreseeable future, given the importance of the topic and, especially, because its role could be crucial for food production in extreme conditions, a scenario with increasing possibilities shortly according to the projections associated with climate change.
Author contributions
MR: Data curation, Formal analysis, Investigation, Methodology, Writing – original draft. PC: Conceptualization, Writing – review & editing. CL-V: Conceptualization, Project administration, Resources, Supervision, Writing – original draft, Writing – review & editing. GR: Conceptualization, Funding acquisition, Project administration, Supervision, Writing – review & editing.
Funding
The author(s) declare that no financial support was received for the research, authorship, and/or publication of this article.
Acknowledgments
The authors CL-V and GR acknowledge the COST CA22134 FoodWaStop Action for promoting networking and interaction.
Conflict of interest
The authors declare that the research was conducted in the absence of any commercial or financial relationships that could be construed as a potential conflict of interest.
The reviewer AI declared a past co-authorship with the author GR to the handling editor.
The author(s) declared that they were an editorial board member of Frontiers, at the time of submission. This had no impact on the peer review process and the final decision.
Publisher’s note
All claims expressed in this article are solely those of the authors and do not necessarily represent those of their affiliated organizations, or those of the publisher, the editors and the reviewers. Any product that may be evaluated in this article, or claim that may be made by its manufacturer, is not guaranteed or endorsed by the publisher.
References
Abbas, T., Zahir, Z. A., Naveed, M. (2017). Bioherbicidal activity of allelopathic bacteria against weeds associated with wheat and their effects on growth of wheat under axenic conditions. BioControl 62, 719–730. doi: 10.1007/s10526-017-9836-6
Abd El-Aziz, M. H., Khalil, M. S. (2020). Antiviral and antinematodal potentials of chitosan: review. J. Plant Sci. Phytopathol. 4, 55–59. doi: 10.29328/journal.jpsp.1001051
Abdellatef, M. A. E., Elagamey, E., Kamel, S. M., Abdellatef, M. A. E., Elagamey, E., Kamel, S. M. (2022). “Chitosan is the ideal resource for plant disease management under sustainable agriculture,” in Chitin and Chitosan - Isolation, Properties, and Applications. Eds. Kumar, B., Surguchov, A. (Amsterdam, Netherlands: IntechOpen). doi: 10.5772/intechopen.107958
Acemi, A., Polat, E. G., Çakir, M., Demiryürek, E., Yavuz, B., Fazıl, Ö. (2021). Molecular weight and concentration of chitosan affect plant development and phenolic substance pattern in arugula. Notulae Botanicae Horti Agrobot. Cluj-Napoca 49, 12296. doi: 10.15835/nbha49212296
Adamczuk, A., Jozefaciuk, G. (2022). Impact of chitosan on the mechanical stability of soils. Molecules 27, 2273. doi: 10.3390/molecules27072273
Adeyeye, S. A. (2012). Fungal mycotoxins in foods: A review. Cogent Food & Agriculture. 2, 1213127. doi: 10.1080/23311932.2016.1213127
Agbodjato, N. A., Noumavo, P. A., Adjanohoun, A., Agbessi, L., Baba-Moussa, L. (2016). Synergistic effects of plant growth promoting rhizobacteria and chitosan on in vitro seeds germination, greenhouse growth, and nutrient uptake of maize (Zea mays L.). Biotechnol. Res. Int., 783018. doi: 10.1155/2016/7830182
Ahmed, K. B. M., Khan, M. M. A., Siddiqui, H., Jahan, A. (2020). Chitosan and its oligosaccharides, a promising option for sustainable crop production- a review. Carbohydr. Polym. 227, 115331. doi: 10.1016/j.carbpol.2019.115331
Ajijah, N., Fiodor, A., Pandey, A. K., Rana, A., Pranaw, K. (2023). Plant Growth-Promoting Bacteria (PGPB) with biofilm-forming ability: a multifaceted agent for sustainable agriculture. Diversity 15, 112. doi: 10.3390/d15010112
Alenazi, M. M., El-Ebidy, A. M., El-Shehaby, O. A., Seleiman, M. F., Aldhuwaib, K. J., Abdel-Aziz, H. M. M. (2024). Chitosan and chitosan nanoparticles differentially alleviate salinity stress in Phaseolus vulgaris L. plants. Plants 13, 398. doi: 10.3390/plants13030398
Ali, A., Zahid, N., Manickam, S., Siddiqui, Y., Alderson, P. G., Maqbool, M. (2014). Induction of lignin and pathogenesis related proteins in dragon fruit plants in response to submicron chitosan dispersions. Crop Prot. 63, 83–88. doi: 10.1016/j.cropro.2014.05.009
ALKahtani, M. D. F., Attia, K. A., Hafez, Y. M., Khan, N., Eid, A. M., Ali, M. A. M., et al. (2020). Chlorophyll fluorescence parameters and antioxidant defense system can display salt tolerance of salt-acclimated sweet pepper plants treated with chitosan and Plant growth-promoting Rhizobacteria. Agronomy 10, 1180. doi: 10.3390/agronomy10081180
Allan, C., Hadwiger, L. (1979). The fungicidal effect of chitosan on fungi of varying cell wall composition. Exp. Mycol. 3, 285–287. doi: 10.1016/S0147-5975(79)80054-7
Almeida, L. G., Silva, E. M., Magalhaes, P. C., Karam, D., Reis, C. O., Gomes Júnior, C. C., et al. (2020). Root system of maize plants cultivated under water deficit conditions with application of chitosan. Rev. Bras. Milho Sorgo 19, e1131. doi: 10.18512/1980-6477/rbms.v19n1p11
Attia, M. S., Osman, M. S., Mohamed, A. S., Mahgoub, H. A., Garada, M. O., Abdelmouty, E. S., et al. (2021). Impact of foliar application of chitosan dissolved in different organic acids on isozymes, protein patterns and physio-biochemical characteristics of tomato grown under salinity stress. Plants 10, 388. doi: 10.3390/plants10020388
Azimi, F., Oraei, M., Gohari, G., Panahirad, S., Farmarzi, A. (2021). Chitosan-selenium nanoparticles (Cs–Se NPs) modulate the photosynthesis parameters, antioxidant enzymes activities and essential oils in Dracocephalum moldavica L. under cadmium toxicity stress. Plant Physiol. Biochem. 167, 257–268. doi: 10.1016/j.plaphy.2021.08.013
Badawy, M. E. I., Rabea, E. I. (2016). “Chitosan and its derivatives as active ingredients against plant pests and diseases,” in Chitosan int the Preservation of Agricultural Commodities. Eds. Bautista-Baños, S., Romanazzi, G., Jimenez-Aparicio, A. (Cambridge, MA, USA: Academic Press) 179–219. doi: 10.1016/b978-0-12-802735-6.00007-0
Bákonyi, N., Bott, S., Gajdos, É., Szabó, A., Jakab, A., Tóth, B., et al. (2013). Using biofertilizer to improve seed germination and early development of maize. Pol. J. Environ. Stud. 22, 1595–1599. Available at: https://www.pjoes.com/pdf-89127-22986?filename=Using%20Biofertilizer%20to.pdf.
Balusamy, S. R., Rahimi, S., Sukweenadhi, J., Sunderraj, S., Shanmugam, R., Thangavelu, L., et al. (2022). Chitosan, chitosan nanoparticles and modified chitosan biomaterials, a potential tool to combat salinity stress in plants. Carbohyd. Polym. 284, 119189. doi: 10.1016/j.carbpol.2022.119189
Baque, M. A., Shiragi, M. H. K., Lee, E.-J., Paek, K.-Y. (2012). Elicitor effect of chitosan and pectin on the biosynthesis of anthraquinones, phenolics and flavonoids in adventitious root suspension cultures of “Morinda citrifolia” (L.). Austral. J. Crop Sci. 6, 1349–1355. doi: 10.3316/informit.732619405669256
Begum, N., Hu, Z., Cai, Q., Lou, L. (2019). Influence of PGPB inoculation on HSP70 and HMA3 gene expression in switchgrass under cadmium stress. Plants 8, 504. doi: 10.3390/plants8110504
Bergonci, T., Paponov, I. A. (2022). Amino acid treatments induce adventitious root formation in two different genotypes of Campanula portenschlagiana. Biol. Life Sci. Forum 16, 8. doi: 10.3390/IECHo2022-12498
Boamah, P. O., Onumah, J., Aduguba, W. O., Santo, K. G. (2023). Application of depolymerized chitosan in crop production: A review. Int. J. Biol. Macromol. 235, 123858. doi: 10.1016/j.ijbiomac.2023.123858
Cartaya-Rubio, O. E., Moreno-Zamora, A. M., Guridi-Izquierdo, F. (2023). Influence of chitosan on sunflower (Helianthus annuus L.) plants grown with high levels of metal ions. Cultivos Tropic. 44, 6. Available at: https://cu-id.com/2050/v44n2e07.
Cesa-Luna, C., Baez, A., Quintero-Hernández, V., de la Cruz-Enríquez, J., Castañeda-Antonio, M. D., Muñoz-Rojas, J. (2020). The importance of antimicrobial compounds produced by beneficial bacteria on the biocontrol of phytopathogens. Acta Biol. Col. 25, 140. doi: 10.15446/abc.v25n1.76867
Chakraborty, M., Hasanuzzaman, M., Rahman, M., Khan, M. A. R., Bhowmik, P., Mahmud, N. U., et al. (2020). Mechanism of plant growth promotion and disease suppression by chitosan biopolymer. Agriculture 10, 624. doi: 10.3390/agriculture10120624
Chandra, S., Askari, K., Kumari, M. (2018). Optimization of indole acetic acid production by isolated bacteria from Stevia rebaudiana rhizosphere and its effects on plant growth. J. Genet. Engin. Biotechnol. 16, 581–586. doi: 10.1016/j.jgeb.2018.09.001
Chandrasekaran, M., Kim, K. D., Chun, S. C. (2020). Antibacterial activity of chitosan nanoparticles: a review. Processes 8, 1173. doi: 10.3390/pr8091173
Chanratana, M., Joe, M. M., Roy Choudhury, A., Anandham, R., Krishnamoorthy, R., Kim, K., et al. (2019). Physiological response of tomato plant to chitosan-immobilized aggregated Methylobacterium oryzae CBMB20 inoculation under salinity stress. 3 Biotech. 9, 397. doi: 10.1007/s13205-019-1923-1
Chen, X. H., Koumoutsi, A., Scholz, R., Eisenreich, A., Schneider, K., Heinemeyer, I., et al. (2007). Comparative analysis of the complete genome sequence of the plant growth–promoting bacterium Bacillus amyloliquefaciens FZB42. Nat. Biotechnol. 25, 1007–1014. doi: 10.1038/nbt1325
Chetverikov, S. P., Loginov, O. N. (2009). New metabolites of Azotobacter vinelandii exhibiting antifungal activity. Microbiology 78, 428–432. doi: 10.1134/S0026261709040055
Chookhongkha, N., Sopondilok, T., Photchanachai, S. (2013). Effect of chitosan and chitosan nanoparticles on fungal growth and chilli seed quality. Acta Hortic. 973, 231–237. doi: 10.17660/ActaHortic.2013.973.32
Ciriello, M., Fusco, G. M., Colla, G., Kyriacou, M. C., Sabatino, L., De Pascale, S., et al. (2024). Adaptation of basil to salt stress: molecular mechanism and physiological regulation. Plant Stress 11, 100431. doi: 10.1016/j.stress.2024.100431
Czékus, Z., Iqbal, N., Pollák, B., Martics, A., Ördög, A., Poór, P. (2021). Role of ethylene and light in chitosan-induced local and systemic defence responses of tomato plants. J. Plant Physiol. 263, 153461. doi: 10.1016/j.jplph.2021.153461
de Oliveira, A. M., de Oliveira Junior, E. N. (2020). “Antifungal and filmogenic properties of micro- and nanostructures of chitosan and its derivatives,” in Nanomycotoxicology: Treating Mycotoxins in the Nano Way, vol. 17. Eds. Rai, M., Abd-Elsalam, K. A. (Cambridge, MA, USA: Academic Press), 397–421. doi: 10.1016/B978-0-12-817998-7.00017-3
Divya, K., Vijayan, S., Nair, S. J., Jisha, M. S. (2019). Optimization of chitosan nanoparticle synthesis and its potential application as germination elicitor of Oryza sativa L. Int. J. Biol. Macromol. 124, 1053–1059. doi: 10.1016/j.ijbiomac.2018.11.185
Do, Q. T. (2022). Antagonistic activities of endophytic bacteria isolated from rice roots against the fungus Mag-naporthe oryzae, a causal of rice blast disease. Egyptian J. Biological Pest Control 32, 69. doi: 10.1186/s41938-022-00571-1
Dradrach, A., Iqbal, M., Lewińska, K., Jędroszka, N., Gull-e-Faran, Rana, M. A. K., et al. (2022). Effects of soil application of chitosan and foliar melatonin on growth, photosynthesis, and heavy metals accumulation in wheat growing on wastewater polluted soil. Sustainability 14, 8293. doi: 10.3390/su14148293
Eilenberg, H., Pnini-Cohen, S., Rahamim, Y., Sionov, E., Segal, E., Carmeli, S., et al. (2010). Induced production of antifungal naphthoquinones in the pitchers of the carnivorous plant Nepenthes khasiana. J. Exp. Bot. 61, 911–922. doi: 10.1093/jxb/erp359.PMid:20018905
Elagamey, E., Abdellatef, M. A., Arafat, M. Y. (2022). Proteomic insights of chitosan mediated inhibition of Fusarium oxysporum f. sp. cucumerinum. J. Proteom. 260, 104560. doi: 10.1016/j.jprot.2022.104560
El-Mohamedy, R. S. R., Abdel-Kader, M. M., Abd-El-Kareem, F. (2013). Inhibitory effect of antagonistic bio-agents and chitosan on the growth of tomato root rot pathogens in vitro. Int. J. Agric. Technol. 9, 1507–1520. Available at: https://www.thaiscience.info/Journals/Article/IJAT/10895654.pdf.
Erman, M., Çiğ, F., Ceritoglu, F., Ceritoglu, M. (2022). Plant growth promoting bacteria enhances photosynthesis, nodulation and root system architecture in lentil under lead toxicity. J. Cent. Eur. Agric. 23, 582–591. doi: 10.5513/JCEA01/23.3.3577
Fang, Y., Xiong, L. (2015). General mechanisms of drought response and their application in drought resistance improvement in plants. Cell. Mol. Life Sci. 72, 673–689. doi: 10.1007/s00018-014-1767-0
Fernández, M., Pagnussat, L. A., Borrajo, M. P., Perez Bravo, J. J., Francois, N. J., Creus, C. M. (2022). Chitosan/starch beads as bioinoculants carrier: Long-term survival of bacteria and plant growth promotion. Appl. Microbiol. Biotechnol. 106, 7963–7972. doi: 10.1007/s00253-022-12220-6
Gamalero, E., Glick, B. R. (2015). Bacterial modulation of plant ethylene levels. Plant Physiol. 169, 13–22. doi: 10.1104/pp.15.00284
Geng, W., Li, Z., Hassan, M. J., Peng, Y. (2020). Chitosan regulates metabolic balance, polyamine accumulation, and Na+ transport contributing to salt tolerance in creeping bentgrass. BMC Plant Biol. 20, 506. doi: 10.1186/s12870-020-02720-w
Ghotbi-Ravandi, A. A., Shahbazi, M., Shariati, M., Mulo, P. (2014). Effects of mild and severe drought stress on photosynthetic efficiency in tolerant and susceptible barley (Hordeum vulgare L.) genotypes. J. Agron. Crop Sci. 200, 403–415. doi: 10.1111/jac.12062
Glick, B. R. (2014). Bacteria with ACC deaminase can promote plant growth and help to feed the world. Microbiol. Res. 169, 30–39. doi: 10.1016/j.micres.2013.09.009
Godínez-Garrido, N. A., Ramírez-Pimentel, J. G., Covarrubias-Prieto, J., Cervantes-Ortiz, F., Pérez-López, A., Aguirre-Mancilla, C. L. (2021). Chitosan coating on bean and maize seeds: release of agrochemical fungicide and post-storage condition. J. Seed Sci. 43, e202143036. doi: 10.1590/2317-1545v43254286
Godínez-Garrido, N. A., Torres-Castillo, J. A., Ramírez-Pimentel, J. G., Covarrubias-Prieto, J., Cervantes-Ortiz, F., Aguirre-Mancilla, C. L. (2022). Effects on germination and plantlet development of sesame (Sesamum indicum L.) and bean (Phaseolus vulgaris L.) seeds with chitosan coatings. Agronomy 12, 666. doi: 10.3390/agronomy12030666
Goudarzian, A., Pirbalouti, A. G., Hossaynzadeh, M. (2020). Menthol, balance of menthol/menthone, and essential oil contents of Mentha × Piperita L. under foliar-applied chitosan and inoculation of arbuscular mycorrhizal fungi. J. Essential Oil-Bearing Plants 23, 1012–1021. doi: 10.1080/0972060X.2020.1828177
Guan, Y., Hu, J., Wang, X., Shao, C. (2009). Seed priming with chitosan improves maize germination and seedling growth in relation to physiological changes under low temperature stress. J. Zhejiang Univ. Sci. B 10, 427–433. doi: 10.1631/jzus.B0820373
Gün Polat, E., Acemi, A., Özen, F. (2022). The effects of chitosan and its acetylation degree on in vitro seed germination and organ development in Ageratum houstonianum Mill. Plant Cell Tiss. Organ Cult. 149, 809–821. doi: 10.1007/s11240-022-02305-5
Guo, J., Muhammad, H., Lv, X., Wei, T., Ren, X., Jia, H., et al. (2020). Prospects and applications of plant growth promoting rhizobacteria to mitigate soil metal contamination: A review. Chemosphere 246, 125823. doi: 10.1016/j.chemosphere.2020.125823
Gutiérrez-Pacheco, M. M., Ortega-Ramírez, L. A., Silva-Espinoza, B. A., Cruz-Valenzuela, M. R., González-Aguilar, G. A., Lizardi-Mendoza, J., et al. (2020). Individual and combined coatings of chitosan and carnauba wax with oregano essential oil to avoid water loss and microbial decay of fresh cucumber. Coatings 10, 614. doi: 10.3390/coatings10070614
Hasan, A., Tabassum, B., Hashim, M., Khan, N. (2024). Role of plant growth promoting rhizobacteria (PGPR) as a plant growth enhancer for sustainable agriculture: A review. Bacteria 3, 59–75. doi: 10.3390/bacteria3020005
Hasanuzzaman, M., Fujita, M. (2022). Plant responses and tolerance to salt stress: physiological and molecular interventions. Int. J. Mol. Sci. 23, 4810. doi: 10.3390/ijms23094810
Hasanuzzaman, M., Raihan, M. R. H., Masud, A. A. C., Rahman, K., Nowroz, F., Rahman, M., et al. (2021). Regulation of reactive oxygen species and antioxidant defense in plants under salinity. Int. J. Mol. Sci. 22, 9326. doi: 10.3390/ijms22179326
Hashem, A., Tabassum, B., Fathi Abd_Allah, E. (2019). Bacillus subtilis: a plant-growth promoting Rhizobacterium that also impacts biotic stress. Saudi J. Biol. Sci. 26, 1291–1297. doi: 10.1016/j.sjbs.2019.05.004
Hassan, F. A. S., Ali, E., Gaber, A., Fetouh, M. I., Mazrou, R. (2021). Chitosan nanoparticles effectively combat salinity stress by enhancing antioxidant activity and alkaloid biosynthesis in Catharanthus roseus (L.) G. Don. Plant Physiol. Biochem. 162, 291–300. doi: 10.1016/j.plaphy.2021.03.004
He, L., Yue, Z., Chen, C., Li, C., Li, J., Sun, Z. (2020). Enhancing iron uptake and alleviating iron toxicity in wheat by plant growth-promoting bacteria: theories and practices. Int. J. Agric. Biol. 23, 190–196. doi: 10.17957/IJAB/15.1276
Heidari, J., Amooaghaie, R., Kiani, S. (2020). Impact of chitosan on nickel bioavailability in soil, the accumulation and tolerance of nickel in Calendula tripterocarpa. Int. J. Phytoremed. 22, 1175–1184. doi: 10.1080/15226514.2020.1748564
Hidangmayum, A., Dwivedi, P., Katiyar, D., Hemantaranjan, A. (2019). Application of chitosan on plant responses with special reference to abiotic stress. Physiol. Mol. Bio.l Plants 25, 313–326. doi: 10.1007/s12298-018-0633-1
Hua, C., Li, Y., Wang, X., Kai, K., Su, M., Shi, W., et al. (2019). The effect of low and high molecular weight chitosan on the control of gray mold (Botrytis cinerea) on kiwifruit and host response. Sci. Hortic. 246, 700–709. doi: 10.1016/j.scienta.2018.11.038
Huang, H., Zhao, Y., Fan, L., Jin, Q., Yang, G., Xu, Z. (2020). Improvement of manganese phytoremediation by Broussonetia papyrifera with two plant growth promoting (PGP) Bacillus species. Chemosphere 260, 127614. doi: 10.1016/j.chemosphere.2020.127614
Iqbal, N., Khan, N. A., Ferrante, A., Trivellini, A., Francini, A., Khan, M. I. R. (2017). Ethylene role in plant growth, development and senescence: interaction with other phytohormones. Front. Plant Sci. 8. doi: 10.3389/fpls.2017.00475
Jian, L., Bai, X., Zhang, H., Song, X., Li, Z. (2019). Promotion of growth and metal accumulation of alfalfa by coinoculation with Sinorhizobium and Agrobacterium under copper and zinc stress. PeerJ 7, e6875. doi: 10.7717/peerj.6875
Jiang, T. D., Ma, R. X., Qi, R. M., Zhang, C. P. (1994). Seed treatment and inhibition of plant pathology of chi-tosan. Journal of Environmental Sciences 6, 112–115. Available at: https://www.jesc.ac.cn/jesc_en/ch/reader/cre-ate_pdf.aspx?file_no=19940114&flag=1.
Jiao, Q., Shen, F., Fan, L., Song, Z., Zhang, J., Song, J., et al. (2024). Chitosan regulates the root architecture system, photosynthetic characteristics and antioxidant system contributing to salt tolerance in maize seedling. Agriculture 14, 304. doi: 10.3390/agriculture14020304
Kadıoglu, G., Koseoglu, M., Özdal, M., Sezen, A., Ozdal, O., Algur, Ö. (2018). Isolation of cold tolerant and ACC deaminase producing plant growth promoting Rhizobacteria from high altitudes. Roman. Biotechnol. Lett. 23, 13479–13486. Available at: http://www.rombio.eu/vol23nr2/13.pdf.
Kang, H., Fan, T., Lin, Z., Shi, Y., Xie, X., Li, L., et al. (2024). Development of chitosan/carrageenan macrobeads for encapsulation of Paenibacillus polymyxa and its biocontrol efficiency against clubroot disease in Brassica crops. Int. J. Biol. Macromol. 264, 130323. doi: 10.1016/j.ijbiomac.2024.130323
Karamchandani, B. M., Chakraborty, S., Dalvi, S. G., Satpute, S. K. (2022). Chitosan and its derivatives: Promising biomaterial in averting fungal diseases of sugarcane and other crops. J. Basic Microbiol. 62, 533–554. doi: 10.1002/jobm.202100613
Katsenios, N., Andreou, V., Sparangis, P., Djordjevic, N., Giannoglou, M., Chanioti, S., et al. (2022). Assessment of plant growth promoting bacteria strains on growth, yield and quality of sweet corn. Sci. Rep. 12, 11598. doi: 10.1038/s41598-022-16044-2
Kayoumu, M., Iqbal, A., Muhammad, N., Li, X., Li, L., Wang, X., et al. (2023). Phosphorus Availability affects the photosynthesis and antioxidant system of contrasting low-P-tolerant cotton genotypes. Antioxidants 12, 466. doi: 10.3390/antiox12020466
Ke, C. L., Deng, F. S., Chuang, C. Y., Lin, C. H. (2021). Antimicrobial actions and applications of chitosan. Polymers 13, 904. doi: 10.3390/polym13060904
Khairy, A. M., Tohamy, M. R., Zayed, M. A., Mahmoud, S. F., El-Tahan, A. M., El-Saadony, M. T., et al. (2022). Eco-friendly application of nano-chitosan for controlling potato and tomato bacterial wilt. Saudi J. Biol. Sci. 29, 2199–2209. doi: 10.1016/j.sjbs.2021.11.041
Khalil, M. S., Badawy, M. E. I. (2012). Nematicidal activity of a biopolymer chitosan at different molecular weights against root-knot nematode, Meloidogyne incognita. Plant Prot. Sci. 48, 170–178. doi: 10.17221/46/2011-PPS
Khan, M. A., Asaf, S., Khan, A. L., Jan, R., Kang, S.-M., Kim, K.-M., et al. (2020). Thermotolerance effect of plant growth-promoting Bacillus cereus SA1 on soybean during heat stress. BMC Microbiol. 20, 175. doi: 10.1186/s12866-020-01822-7
Khaptsev, Z., Lugovitskaya, T., Shipovskaya, A., Shipenok, K. (2021). Biological activity of chitosan aspartate and its effect on germination of test seeds. IOP Conf. Series: Earth Environ. Sci. 723, 22074. doi: 10.1088/1755-1315/723/2/022074
Khatoon, Z., Huang, S., Farooq, M. A., Santoyo, G., Rafique, M., Javed, S., et al. (2022). “Chapter 12 - Role of plant growth-promoting bacteria (PGPB) in abiotic stress management,” in Mitigation of Plant Abiotic Stress by Microorganisms. Eds. Santoyo, G., Kumar, A., Aamir, M., Uthandi, S. (Cambridge, MA, USA: Academic Press), 257–272. doi: 10.1016/B978-0-323-90568-8.00012-2
Kulus, D. (2019). “Application of synthetic seeds in propagation, storage, and preservation of Asteraceae plant species,” in Synthetic seeds: germplasm regeneration, preservation and prospects. Eds. Faisal, M., Alatar, A. (Springer, Switzerland), 155–179. doi: 10.1007/978-3-030-24631-0_6
Kumar, A., Singh, S., Gaurav, A. K., Srivastava, S., Verma, J. P. (2020). Plant growth-promoting bacteria: biological tools for the mitigation of salinity stress in plants. Front. Microbiol. 11. doi: 10.3389/fmicb.2020.01216
Lárez-Velásquez, C. (2023). Chitosan: an overview of its multiple advantages for creating sustainable development poles. Polímeros 33, e20230005. doi: 10.1590/0104-1428.20220103
Lárez-Velásquez, C., Rojas-Avelizapa, L. (2020). A Review on the physicochemical and biological aspects of the chitosan antifungal activity in agricultural applications. J. Res. Updates Polym. Sci. 9, 70–79. doi: 10.6000/1929-5995.2020.09.07
Le Gall, H., Philippe, F., Domon, J.-M., Gillet, F., Pelloux, J., Rayon, C. (2015). Cell wall metabolism in response to abiotic stress. Plants 4, 112–166. doi: 10.3390/plants4010112
Lerouge, P., Roche, P., Faucher, C., Maillet, F., Truchet, G., Promé, J. C., et al. (1990). Symbiotic host-specificity of Rhizobium meliloti is determined by a sulphated and acylated glucosamine oligosaccharide signal. Nature 344, 781–784. doi: 10.1038/344781a0
Li, R., He, J., Xie, H., Wang, W., Bose, S. K., Sun, Y., et al. (2019). Effects of chitosan nanoparticles on seed germination and seedling growth of wheat (Triticum aestivum L.). Int. J. Biol. Macromol. 126, 91–100. doi: 10.1016/j.ijbiomac.2018.12.118
Li, Y., Lin, J., Huang, Y., Yao, Y., Wang, X., Liu, C., et al. (2020b). Bioaugmentation-assisted phytoremediation of manganese and cadmium co-contaminated soil by Polygonaceae plants (Polygonum hydropiper L. and Polygonum lapathifolium L.) and Enterobacter sp. FM-1. Plant Soil 448, 439–453. doi: 10.1007/s11104-020-04447-x
Li, K. C., Zhang, X. Q., Yu, Y., Xing, R. E., Liu, S., Li, P. C. (2020a). Effect of chitin and chitosan hexamers on growth and photosynthetic characteristics of wheat seedlings. Photosynthesis 58, 819–826. doi: 10.32615/ps.2020.021
Limpens, E., van Zeijl, A., Geurts, R. (2015). Lipochitooligosaccharides modulate plant host immunity to enable endosymbioses. Annu. Rev. Phytopathol. 53, 311–334. doi: 10.1146/annurev-phyto-080614-120149
Liu, K., Ding, H., Yu, Y., Chen, B. (2019a). A Cold-adapted chitinase-producing bacterium from Antarctica and its potential in biocontrol of plant pathogenic fungi. Mar. Drugs 17, 695. doi: 10.3390/md17120695
Liu, J., Gai, L., Zong, H. (2021). Foliage application of chitosan alleviates the adverse effects of cadmium stress in wheat seedlings (Triticum aestivum L.). Plant Physiol. Biochem. 164, 115–121. doi: 10.1016/j.plaphy.2021.04.038
Liu, J., Zhang, X., Kennedy, J. F., Jiang, M., Cai, Q., Wu, X. (2019b). Chitosan induces resistance to tuber rot in stored potato caused by Alternaria tenuissima. Int. J. Biol. Macromol. 140, 851–857. doi: 10.1016/j.ijbiomac.2019.08.227
Long, S. R. (1996). Rhizobium symbiosis: nod factors in perspective. Plant Cell 8, 1885–1888. doi: 10.1105/tpc.8.10.1885
Lopez-Moya, F., Escudero, N., Zavala-Gonzalez, E. A., Esteve-Bruna, D., Blázquez, M. A., Alabadí, D., et al. (2017). Induction of auxin biosynthesis and WOX5 repression mediate changes in root development in Arabidopsis exposed to chitosan. Sci. Rep. 7, 16813. doi: 10.1038/s41598-017-16874-5
Lopez-Moya, F., Suarez-Fernandez, M., Lopez-Llorca, L. V. (2019). Molecular mechanisms of chitosan interactions with fungi and plants. Int. J. Mol. Sci. 20, 332. doi: 10.3390/ijms20020332
Luo, L., Zhao, C., Wang, E., Raza, A., Yin, C. (2022). Bacillus amyloliquefaciens as an excellent agent for biofertilizer and biocontrol in agriculture: An overview for its mechanisms. Microbiol. Res. 259, 127016. doi: 10.1016/j.micres.2022.127016
Lustriane, C., Dwivany, F. M., Suendo, V., Reza, M. (2018). Effect of chitosan and chitosan-nanoparticles on postharvest quality of banana fruits. J. Plant Biotechnol. 45, 36–44. doi: 10.5010/JPB.2018.45.1.036
Malerba, M., Cerana, R. (2020). Chitin- and chitosan-based derivatives in plant protection against biotic and abiotic stresses and in recovery of contaminated soil and water. Polysaccharides 1, 21–30. doi: 10.3390/polysaccharides1010003
Matilla, M. Á., Krell, T. (2017). Bacterias rizosféricas como fuente de antibióticos. Alianzas y Tendencias BUAP 2, 14–21. Available at: https://www.aytbuap.mx/publicacionesh.mglzgs108ub3.
Meyer-Déru, L., David, G., Auvergne, R. (2022). Chitosan chemistry review for living organisms encapsulation. Carbohyd. Polym. 295, 119877. doi: 10.1016/j.carbpol.2022.119877
Mia, J. (2017). Isolation and characterization of plant growth promoting endophytic bacteria from Eriocephalus africanus roots (South Africa: University of the Western Cape). Available at: https://etd.uwc.ac.za/xmlui/handle/11394/6260.
Miransari, M., Smith, D. L. (2014). Plant hormones and seed germination. Environ. Exp. Bot. 99, 110–121. doi: 10.1016/j.envexpbot.2013.11.005
Mitchell, R., Alexander, M. (1961). Chitin and the biological control of Fusarium diseases. Plant Dis. Rep. 45, 487–490. doi: 10.5555/19620302965
Mosapour-Yahyaabadi, H., Asgharipour, M. R., Basiri, M. (2016). Role of chitosan in improving salinity resistance through some morphological and physiological characteristics in fenugreek (Trigonella foenum-graecum L.). J. Sci. Technol. Greenhouse Cult. 7, 165–175. doi: 10.18869/acadpub.ejgcst.7.1.165
Moumni, M., Brodal, G., Romanazzi, G. (2023). Recent innovative seed treatment methods in the management of seedborne pathogens. Food Secur. 15, 1365–1382. doi: 10.1007/s12571-023-01384-2
Mukarram, M., Ali, J., Dadkhah-Aghdash, H., Kurjak, D., Kačík, F., Ďurkovič, J. (2023). Chitosan-induced biotic stress tolerance and crosstalk with phytohormones, antioxidants, and other signalling molecules. Front. Plant Sci. 14. doi: 10.3389/fpls.2023.1217822
Mukta, J. A., Rahman, M., Sabir, A. A., Gupta, D. R., Surovy, M. Z., Rahman, M., et al. (2017). Chitosan and plant probiotics application enhance growth and yield of strawberry. Biocatal. Agric. Biotechnol. 11, 9–18. doi: 10.1016/j.bcab.2017.05.005
Mustafa, A., Naveed, M., Abbas, T., Saeed, Q., Hussain, A., Ashraf, M. N., et al. (2019). Growth response of wheat and associated weeds to plant antagonistic rhizobacteria and fungi. Ital. J. Agron. 14, 191–198. doi: 10.4081/ija.2019.1449
Nagasawa, N., Mitomo, H., Ha, P. T. L., Watanabe, S., Ito, T., Takeshita, H., et al. (2001).Suppression of Zn stress on barley by irradiated chitosan. In: Conference JAERI-Conf-2001-005. Available online at: https://www.osti.gov/etdeweb/biblio/20226902 (Accessed April 22, 2024).
Naylor, D., Coleman-Derr, D. (2018). Drought stress and root-associated bacterial communities. Front. Plant Sci. 8. doi: 10.3389/fpls.2017.02223
Ngalimat, M. S., Mohd Hata, E., Zulperi, D., Ismail, S. I., Ismail, M. R., Mohd Zainudin, N. A. I., et al. (2021). Plant growth-promoting bacteria as an emerging tool to manage bacterial rice pathogens. Microorganisms 9, 682. doi: 10.3390/microorganisms9040682
Nichols, E. J., Hadwiger, L. A. (1979). “Enzymes from pea tissue degrade Fusarium solani cell walls; chitosan fragments both induce pisatin and inhibit fungal growth,” Phytopathology, 69, 1040 (Abstracts of papers presented at American Phytopathology Society, Meetings 1979). Available at: https://www.apsnet.org/publications/phytopathology/backissues/Docu-ments/1979Articles/Phyto69n09_1019.pdf.
Odat, N., Tawaha, A. M., Hasan, M., Imran, Amanullah, Al-Tawaha, A. R., et al. (2021). “Seed priming with chitosan alleviates salinity stress by improving germination and early growth parameters in common vetch (Vicia sativa),” in IOP Conf. Ser.: Earth Environ. Sci, (Bristol, UK: IOP Publishing) Vol. 788. 012059. doi: 10.1088/1755-1315/788/1/012059
Oddo, E., Russo, G., Grisafi, F. (2019). Effects of foliar application of glycine betaine and chitosan on Puccinellia distans (Jacq.) Parl. subjected to salt stress. Biol. Futura 70, 47–55. doi: 10.1556/019.70.2019.06
Olanrewaju, O. S., Glick, B. R., Babalola, O. O. (2017). Mechanisms of action of plant growth promoting bacteria. World J. Microbiol. Biotechnol. 33, 197. doi: 10.1007/s11274-017-2364-9
Ortega-García, J., Holguín-Peña, R. J., Preciado-Rangel, P., Guillén-Enríquez, R. R., Zapata-Sifuentes, G., Nava-Santos, J. M., et al. (2021). Bacillus amyloliquefaciens as a halo-PGPB and chitosan effects in nutritional value and yield production of Asparagus officinalis L. under Sonora desert conditions. Notulae Botanicae Horti Agrobot. Cluj-Napoca 49, 12414–12414. doi: 10.15835/nbha49312414
Panichikkal, J., Mohanan, D. P., Koramkulam, S., Krishnankutty, R. E. (2022). Chitosan nanoparticles augmented indole-3-acetic acid production by rhizospheric Pseudomonas monteilii. J. Basic Microbiol. 62, 1467–1474. doi: 10.1002/jobm.202100358
Parijadi, A. A. R., Yamamoto, K., Ikram, M. M. M., Dwivany, F. M., Wikantika, K., Putri, S. P., et al. (2022). Metabolome analysis of banana (Musa acuminata) treated with chitosan coating and low temperature reveals different mechanisms modulating delayed ripening. Front. Sustain. Food Syst. 6. doi: 10.3389/fsufs.2022.835978
Pavlicevic, M., Elmer, W., Zuverza-Mena, N., Abdelraheem, W., Patel, R., Dimkpa, C., et al. (2023). Nanoparticles and biochar with adsorbed plant growth-promoting rhizobacteria alleviate Fusarium wilt damage on tomato and watermelon. Plant Physiol. Biochem. 203, 108052. doi: 10.1016/j.plaphy.2023.108052
Perez, J. J., Francois, N. J., Maroniche, G. A., Borrajo, M. P., Pereyra, M. A., Creus, C. M. (2018). A novel, green, low-cost chitosan-starch hydrogel as potential delivery system for plant growth-promoting bacteria. Carbohydr. Polym. 202, 409–417. doi: 10.1016/j.carbpol.2018.07.084
Peykani, L. S., Sepehr, M. F. (2018). Effect of chitosan on antioxidant enzyme activity, proline, and malondialdehyde content in Triticum aestivum L. and Zea maize L. under salt stress condition. Ir. J. Plant Physiol. 9, 2661–2670. Available at: https://sanad.iau.ir/fa/Journal/ijpp/DownloadFile/1026145.
Pishchik, V. N., Filippova, P. S., Mirskaya, G. V., Khomyakov, Y. V., Vertebny, V. E., Dubovitskaya, V. I., et al. (2021). Epiphytic PGPB Bacillus megaterium AFI1 and Paenibacillus nicotianae AFI2 improve wheat growth and antioxidant status under Ni stress. Plants 10, 2334. doi: 10.3390/plants10112334
Prusky, D., Romanazzi, G. (2023). Induced resistance in fruit and vegetables: A host physiological response limiting postharvest disease development. Annu. Rev. Phytopathol. 61, 279–300. doi: 10.1146/annurev-phyto-021722-035135
Qu, D. Y., Gu, W. R., Zhang, L. G., Li, C. F., Chen, X. C., Li, J., et al. (2019). Role of chitosan in the regulation of the growth, antioxidant system and photosynthetic characteristics of maize seedlings under cadmium stress. Russ. J. Plant Physiol. 66, 140–151. doi: 10.1134/S102144371901014X
Quitadamo, F., De Simone, V., Beleggia, R., Trono, D. (2021). Chitosan-induced activation of the antioxidant defense system counteracts the adverse effects of salinity in durum wheat. Plants 10, 1365. doi: 10.3390/plants10071365
Rabêlo, V. M., Magalhães, P. C., Bressanin, L. A., Carvalho, D. T., dos Reis, C. O., Karam, D., et al. (2019). The foliar application of a mixture of semisynthetic chitosan derivatives induces tolerance to water deficit in maize, improving the antioxidant system and increasing photosynthesis and grain yield. Sci. Rep. 9, 8164. doi: 10.1038/s41598-019-44649-7
Raja, K., Sivasubramaniam, K., Anandham, R. (2019). Seed treatment with liquid microbial consortia for germination and vigour improvement in tomato (Solanum lycopersicum L.). JAH 21, 195–200. doi: 10.37855/jah.2019.v21i03.33
Riseh, R. S., Ebrahimi-Zarandi, M., Gholizadeh Vazvani, M., Skorik, Y. A. (2021). Reducing drought stress in plants by encapsulating plant growth-promoting bacteria with polysaccharides. Int. J. Mol. Sci. 22, 12979. doi: 10.3390/ijms222312979
Riseh, R. S., Hassanisaadi, M., Vatankhah, M., Babaki, S. A., Barka, E. A. (2022). Chitosan as a potential natural compound to manage plant diseases. Int. J. Biol. Macromol. 220, 998–1009. doi: 10.1016/j.ijbiomac.2022.08.109
Rojas-Pirela, M., Rojas-Mendizábal, V., Pérez-Pérez, E. M., Lárez-Velásquez, C. (2021). Cell encapsulation using chitosan: chemical aspects and applications. Avances Química 16, 89–103. Available at: http://erevistas.saber.ula.ve/index.php/avancesenquimica/article/download/17665/21921928894.
Romanazzi, G., Feliziani, E., Sivakumar, D. (2018). Chitosan, a biopolymer with triple action on postharvest decay of fruit and vegetables: eliciting, antimicrobial and film-forming properties. Front. Microbiol. 9. doi: 10.3389/fmicb.2018.02745
Romanazzi, G., Moumni, M. (2022). Chitosan and other edible coatings to extend shelf life, manage postharvest decay, and reduce loss and waste of fresh fruits and vegetables. Curr. Opin. Biotechnol. 78, 102834. doi: 10.1016/j.copbio.2022.102834
Rozier, C., Gerin, F., Czarnes, S., Legendre, L. (2019). Biopriming of maize germination by the plant growth-promoting Rhizobacterium Azospirillum lipoferum CRT1. J. Plant Physiol. 237, 111–119. doi: 10.1016/j.jplph.2019.04.011
Schmidt, W., Thomine, S., Buckhout, T. J. (2020). Editorial: Iron nutrition and interactions in plants. Front. Plant Sci. 10. doi: 10.3389/fpls.2019.01670
Selim, S., Akhtar, N., Hagagy, N., Alanazi, A., Warrad, M., El Azab, E., et al. (2022). Selection of newly identified growth-promoting Archaea Haloferax species with a potential action on cobalt resistance in maize plants. Front. Plant Sci. 13. doi: 10.3389/fpls.2022.872654
Selim, S., Hassan, Y. M., Saleh, A. M., Habeeb, T. H., AbdElgawad, H. (2019). Actinobacterium isolated from a semi-arid environment improves the drought tolerance in maize (Zea mays L.). Plant Physiol. Biochem. 142, 15–21. doi: 10.1016/j.plaphy.2019.06.029
Shah, S., Hashmi, M. S. (2020). Chitosan–aloe vera gel coating delays postharvest decay of mango fruit. Hortic. Environ. Biotechnol. 61, 279–289. doi: 10.1007/s13580-019-00224-7
Shahrajabian, M. H., Chaski, C., Polyzos, N., Tzortzakis, N., Petropoulos, S. A. (2021). Sustainable agriculture systems in vegetable production using chitin and chitosan as plant biostimulants. Biomolecules 11, 819. doi: 10.3390/biom11060819
Shamshina, J. L., Kelly, A., Oldham, T., Rogers, R. D. (2020). Agricultural uses of chitin polymers. Environ. Chem. Lett. 18, 53–60. doi: 10.1007/s10311-019-00934-5
Sharp, R. G. (2013). A review of the applications of chitin and its derivatives in agriculture to modify plant-microbial interactions and improve crop yields. Agronomy 3, 757–793. doi: 10.3390/agronomy3040757
Shirkhani, Z., Chehregani Rad, A., Mohsenzadeh, F. (2021). Improving Cd-phytoremediation ability of Datura stramonium L. by Chitosan and Chitosan nanoparticles. Biologia 76, 2161–2171. doi: 10.1007/s11756-021-00758-1
Singh, A., Gairola, K., Upadhyay, V., Kumar, J. (2018). Chitosan: An elicitor and antimicrobial Bio-resource in plant protection. Agric. Rev. 39, 163–168. doi: 10.18805/ag.R-1723
Souza, R., Ambrosini, A., Passaglia, L. M. P. (2015). Plant growth-promoting bacteria as inoculants in agricultural soils. Genet. Mol. Biol. 38, 401–419. doi: 10.1590/S1415-475738420150053
Sreekumar, S., Wattjes, J., Niehues, A., Mengoni, T., Mendes, A. C., Morris, E. R., et al. (2022). Biotechnologically produced chitosans with nonrandom acetylation patterns differ from conventional chitosans in properties and activities. Nat. Commun. 13, 7125. doi: 10.1038/s41467-022-34483-3
Sun, Y., Luo, K., He, J., Zhu, X., Song, X., Sun, Y., Zhao, Y. (2024). Reactive oxygen species responsive chitooligo-saccharides based nanoplatform for sonodynamic therapy in mammary cancer. Carbohydrate Polymers 342, 122403. doi: 10.1016/j.carbpol.2024.122403
Sumbul, A., Ansari, R. A., Rizvi, R., Mahmood, I. (2020). Azotobacter: A potential bio-fertilizer for soil and plant health management. Saudi Journal of Biological Sciences 27, 3634–3640. doi: 10.1016/j.sjbs.2020.08.004
Tarakanov, R., Ignatyeva, I., Dzhalilov, F. (2023). “Detection of Pseudomonas savastanoi pv. glycinea in soybean seeds using PCR assay,” in AIP Conference Proceedings, vol. 2921. (Melville; USA: AIP Publishing).
Tenhaken, R. (2015). Cell wall remodeling under abiotic stress. Front. Plant Sci. 5. doi: 10.3389/fpls.2014.00771
Tham, L. X., Nagasawa, N., Matsuhashi, S., Ishioka, N. S., Ito, T., Kume, T. (2001). Effect of radiation-degraded chitosan on plants stressed with vanadium. Rad. Phys. Chem. 61, 171–175. doi: 10.1016/S0969-806X(00)00388-1
Tripathi, P., Rabara, R. C., Reese, R. N., Miller, M. A., Rohila, J. S., Subramanian, S., et al. (2016). A toolbox of genes, proteins, metabolites and promoters for improving drought tolerance in soybean includes the metabolite coumestrol and stomatal development genes. BMC Genom. 17, 102. doi: 10.1186/s12864-016-2420-0
Vassilev, N., Vassileva, M., Martos, V., Garcia del Moral, L. F., Kowalska, J., Tylkowski, B., et al. (2020). Formulation of microbial inoculants by encapsulation in natural polysaccharides: focus on beneficial properties of carrier additives and derivatives. Front. Plant Sci. 11. doi: 10.3389/fpls.2020.00270
Velásquez, C. L., Pirela, M. L., Chirinos, A., Avelizapa, L. I. (2019). Nuevos retos en agricultura para los biopolìmeros de quitina y quitosano. Parte 1: efectos beneficiosos para los cultivos. Revista Iberoamericana de Polímeros. 20, 118–136. Available at: https://reviberpol.org/wp-content/uploads/2019/06/2019-20-3-118-136-larez-y-col-1.pdf
Verma, C., Quraishi, M. A. (2021). Chelation capability of chitosan and chitosan derivatives: Recent developments in sustainable corrosion inhibition and metal decontamination applications. Curr. Res. Green Sustain. Chem. 4, 100184. doi: 10.1016/j.crgsc.2021.100184
White, P. J., Brown, P. H. (2010). Plant nutrition for sustainable development and global health. Ann. Bot. 105, 1073–1080. doi: 10.1093/aob/mcq085
Widawati, S., Suliasih (2018). The effect of Plant Growth Promoting Rhizobacteria (PGPR) on germination and seedling growth of Sorghum bicolor L. Moench. IOP Conf. Ser.: Earth Environ. Sci. 166, 12022. doi: 10.1088/1755-1315/166/1/012022
Winkler, A. J., Dominguez-Nuñez, J. A., Aranaz, I., Poza-Carrión, C., Ramonell, K., Somerville, S., et al. (2017). Short-chain chitin oligomers: promoters of plant growth. Mar. Drugs 15, 40. doi: 10.3390/md15020040
Xie, Y., Peng, Q., Ji, Y., Xie, A., Yang, L., Mu, S., et al. (2021). Isolation and identification of antibacterial bioactive compounds from Bacillus megaterium L2. Front. Microbiol. 12. doi: 10.3389/fmicb.2021.645484
Xu, D., Li, H., Lin, L., Liao, M., Deng, Q., Wang, J., et al. (2020). Effects of carboxymethyl chitosan on the growth and nutrient uptake in Prunus davidiana seedlings. Physiol. Mol. Biol. Plants 26, 661–668. doi: 10.1007/s12298-020-00791-5
Keywords: biostimulants, chitosan, induced resistance, plant growth-promoting bacteria, stress 1
Citation: Rojas-Pirela M, Carillo P, Lárez-Velásquez C and Romanazzi G (2024) Effects of chitosan on plant growth under stress conditions: similarities with plant growth promoting bacteria. Front. Plant Sci. 15:1423949. doi: 10.3389/fpls.2024.1423949
Received: 26 April 2024; Accepted: 16 October 2024;
Published: 08 November 2024.
Edited by:
Vicent Arbona, University of Jaume I, SpainReviewed by:
Nitin Kumar, Central Pulp & Paper Research Institute (CPPRI), IndiaAntonio Ippolito, University of Bari Aldo Moro, Italy
George Karaoglanidis, Aristotle University of Thessaloniki, Greece
Kata Ludman-Mihály, University of Debrecen, Hungary
Copyright © 2024 Rojas-Pirela, Carillo, Lárez-Velásquez and Romanazzi. This is an open-access article distributed under the terms of the Creative Commons Attribution License (CC BY). The use, distribution or reproduction in other forums is permitted, provided the original author(s) and the copyright owner(s) are credited and that the original publication in this journal is cited, in accordance with accepted academic practice. No use, distribution or reproduction is permitted which does not comply with these terms.
*Correspondence: Cristóbal Lárez-Velásquez, Y2xhcmV6QHVsYS52ZQ==; Gianfranco Romanazzi, Zy5yb21hbmF6emlAdW5pdnBtLml0