- Key Laboratory of Sichuan Province for Bamboo Pests Control and Resource Development, Leshan Normal University, Leshan, Sichuan, China
NAC transcription factors are an important genes that regulate plant growth and development, and can regulate functions such as fruit ripening in plants. Based on genome data of Dendrobium nobile, the NAC gene family was identified and analyzed by bioinformatics methods. In this study, we identified 85 NAC genes in Dendrobium nobile genome, and systematically analyzed the NAC gene family. We found that they were distributed unevenly in the nineteen chromosomes. The amino acid length of D. nobile NAC gene family (DnoNACs) ranged from 80 to 1065, molecular weight ranged from 22.17 to 119.02 kD, and isoelectric point ranged from 4.61~9.26. Its promoter region contains multiple stress responsive elements, including light responsive, gibberellin-responsive, abscisic acid responsiveness, MeJA-responsiveness and drought-inducibility elements. Phylogenetic analysis indicates that the D. nobile NAC gene family is most closely related to Dendrobium catenatum and Dendrobium chrysotoxum. Analysis of SSR loci indicates that the fraction of mononucleotide repeats was the largest, as was the frequency of A/T. Non-coding RNA analysis showed that these 85 NAC genes contain 397 miRNAs. The collinearity analysis shows that 9 collinear locis were found on the chromosomes of D. nobile with Arabidopsis thaliana, and 75 collinear locis with D.chrysotoxum. QRT-PCR experiment under different salt concentration and temperature conditions verified the response mechanism of DnoNAC gene family under stress conditions. Most DnoNAC genes are sensitive to salt stress and temperature stress. The results of this study provide a reference for further understanding the function of NAC gene in D. nobile.
Introduction
Dendrobium nobile, a perennial herb of the genus Dendrobium in the Orchidaceae family, is a traditional herbal medicine found primarily in tropical and subtropical Asia (Li et al., 2020; Li L. et al., 2022). According to research, the chemical constituents of D. nobile are primarily polysaccharides, alkaloids, amino acids, phenanthrenes, and coumarins, with polysaccharides and alkaloids serving as the foundation of its pharmacological effects and being primarily stored in the stem (Xu Q. et al., 2022). D. nobile’s pharmacological effects include antioxidant, antitumor, antihyperglycemic, improving immunity, promoting digestive juice secretion, inhibiting platelet agglutination, and lowering blood lipids (Wang et al., 2010; Li et al., 2011; Liu et al., 2011; Teixeira da Silva and Ng, 2017). Furthermore, the main alkaloid in D. nobile is dendrobine, a sesquiterpene alkaloid with significant pharmacological activity that is considered one of the criteria for evaluating D. nobile quality (Gong et al., 2022).
Transcription factor (TF) is a protein molecule with a specific structure in eukaryotes that has a regulatory influence on gene transcription (Kadonaga, 1998), and transcription factors in plants can be categorized into DOF, WRKY, BZIP, NAC, MYB, ERF, and other families based on DNA structural domains (Tolosa and Zhang, 2020). NAC transcription factors are widespread in terrestrial plants and comprise one of the most extensive families of plant-specific transcription factors involved in plant growth and development, as well as biotic and abiotic stress responses. Its name is derived from three gene fragments, Petunia NAM, Arabidopsis ATAF1/2, and CUC2, and because these three genes encode protein sequences with a highly conserved amino acid sequence at the N-terminus, the initials of these three genes were used to name this structure as the NAC structural domain (Aida, 1997; Olsen et al., 2005a). A typical NAC family member’s N terminus contains a conserved NAC-specific structural domain of about 150 amino acids that not contain the classical helix-turned-helical structure and instead consists of a twisted β-pleated sheet structure surrounded by several helical elements. The functional dimers formed by the NAC domain in this structure serve as a structural template for understanding the function of NAC protein at the molecular level (Ernst et al., 2004). Meanwhile, NAC is made up of three highly conserved sub-structural domains A, B, and C, as well as two less conserved domains D and E. Sub-structural domain C could be engaged in DNA binding, while sub-structural domain E could be involved in control and synergistic sub-structural domain D binding to DNA throughout plant development (Greve et al., 2003; Diao et al., 2020; Ren et al., 2021). The C-terminus of NAC proteins contains a transcriptional regulatory region with highly variable transcriptional activation or repression activity (Jensen et al., 2007), which is typically characterized by the presence of repetitive sequences of simple amino acids like Ser, Thr, Pro, Glu or acidic amino acid residues (Olsen et al., 2005b).
Although members of the NAC family perform some functions similarly, they have distinct roles during various stages and parts of plant growth and development (Liu et al., 2022). For instance, NAC can promote the growth of plant reproductive and nutritive organs, participate in the control of secondary plant cell wall production, signal phytohormones, govern the viability of seeds, and control the flowering and senescence of plant tissues. Additionally, it is also involved in plant defense responses in biotic stresses and responses to abiotic stresses, etc (Puranik et al., 2012; Kim et al., 2016; Guo et al., 2018; Diao et al., 2020). The NAM gene in the wheat NAC transcription factor plays a central role in improving the nutritional value of wheat seeds by regulating the RNA levels of multiple NAM homologs capable of improving protein, zinc and iron content (Uauy et al., 2006). Maize transcription factors ZmNAM1, ZmNAM2, and ZmCUC3 are involved in the formation of interstem meristematic tissues (Zimmermann and Werr, 2005). Ectopic expression of NST1 (NAC secondary wall thinking promoting factor 1) or NST2 in Arabidopsis can cause ectopic thickening of secondary walls of various aboveground tissues (Mitsuda, 2005). PtrWND1B gene expression regulates cell wall thickening in Populus trichocarpa (Zhao et al., 2014). The rose RhNAC100 transcription factor regulates cell expansion (Pei et al., 2013). The tomato SlNAP2 transcription factor regulates leaf senescence and fruit yield (Ma et al., 2018). The rice NAC transcription factor gene OsNAC6 can operate as a transcriptional activator in response to biotic and abiotic stresses, and it can be exploited as a biotechnological tool to improve stress resistance (Nakashima et al., 2007). The ability to express the NtNAC-R1 gene in the tobacco NAC transcription factor impacts lateral root formation and nicotine synthesis (Diao et al., 2020). The wheat TaNAC2-5A transcription factor is important in nitrate signaling, and wheat overexpressing TaNAC2-5A has better yield and nitrogen buildup (He et al., 2015). The kiwifruit NAC transcription factors AaNAC2, AaNAC3, and AaNAC4 genes govern fruit terpene synthesis (Nieuwenhuizen et al., 2015), The barley HvNAC6 transcription factor can increase powdery mildew resistance by modulating ABA (Chen et al., 2013). The norway spruce PaNAC03 transcription factor affects embryonic development and inhibits flavonoid synthesis (Dalman et al., 2017).
Since the first discovery of NAC transcription factors in 1996, researchers have discovered the existence of NAC transcription factors in an increasing number of plants, and have made certain progress in their structure, expression characteristics, and biological functions. At the moment, research on NAC transcription factors is continuing. Some studies have identified and analyzed members of the NAC gene family in plant genomes such as Arabidopsis thaliana (Hisako et al., 2004), Zanthoxylum bungeanum (Hu et al., 2022), potato (Singh et al., 2013), melon (Wei et al., 2016), sunflower (Bengoa Luoni et al., 2021), Asparagus officinalis (Li C. et al., 2022), Brassica juncea var. Tumida (He et al., 2020), Saccharum spontaneum (Shen et al., 2022) and Hibiscus hamabo Sieb (Wang et al., 2022b), but there is no relevant research report on the NAC gene family in D. nobile’s whole genome. In this study, bioinformatics methods were used to conduct a comprehensive analysis of the physicochemical properties, chromosomal positioning, and conserved motifs of the D. nobile NAC gene family (named: DnoNAC), and the resulting DnoNAC proteins were compared in multiple sequences to construct an phylogenetic tree to analyze their molecular evolutionary relationships, with the goal of laying the groundwork for future research on the functions of the D. nobile NAC.
Materials and methods
Data acquisition, plant materials and experimental design
The whole genome sequences, protein sequences and gene annotation files of D. nobile were downloaded from NCBI database (https://www.ncbi.nlm.nih.gov) (Xu Q. et al., 2022). The NAC family protein sequences of Arabidopsis thaliana, Oryza sativa subsp. japonica, Zea mays, Populus euphratica, Populus trichocarpa, Brachypodium distachyon and Brachypodium stacei were downloaded from Plant Transcription Factor Database (http://planttfdb.gao-lab.org/) (Chang and Chow, 2023). The genome sequences, protein sequences and gene annotation files of Dendrobium catenatum and Dendrobium chrysotoxum were downloaded from the NCBI database to identify the NAC gene protein sequences for subsequent bioinformatics analysis. D. nobile seedlings are planted in Key Laboratory of Sichuan Province for Bamboo Pests Control and Resource Development of Leshan Normal University from March 10th to May 10th, 2023. The first experimental treatment is as follows: the treatment group treated D. nobile seedlings with NaCl solutions at concentrations of 1.0, 3.0, 6.0, and 10.0 g/L for 0 h, 24 h, 48 h, and 72 h, while the control group had a concentration of 0 g/L. The second experimental treatment is as follows: the treatment group is cultivating D. nobile seedlings at temperatures of 0 °C, 5 °C, 10 °C, 35 °C, and 40 °C for 0 h, 24 h, 48 h, and 72 h. while the control group had a temperature of 25 °C. The total RNA was extracted from young leaves of the treatment group and the control group. Reverse transcription of purified RNA into cDNA using a reverse transcription kit, and reverse transcribed cDNA was used for qRT-PCR to verify the expression of NAC transcription factor family members in D. nobile. Plant RNA extraction kit and cDNA reverse transcription kit are purchased from TIANGEN Biotech(Beijing)Co.,Ltd. The qRT-PCR experiments in this study were all completed on fluorescence quantitative PCR instrument (qToWer3G) of the Analytick Jena AG. The number of replicates of biological samples in each treatment group and control group is 3, and the number of machine replicates on fluorescence quantitative PCR is 3. All DnoNAC gene primers designed by TBtools Batch q-RT-PCR primer design tool used in the qRT-PCR validation experiment in this study are shown in Supplementary Table 1. The qRT-PCR primers used in this study were synthesized by Sangon Biotech (Shanghai) Co., Ltd on commission.
Analysis of identification, chromosomal localization
Analysis of conserved structural domains was performed using the online software SMART (http://smart.embl-heidelberg.de/) (Li et al., 2021). The family genes were identified by combining SMART online software and Pfam, and the selected sequences were simplified using TBtools (Chen et al., 2020). Chromosomal localization analysis of the NAC gene family was performed using MapChart software based on the GFF3 files of the genes.
Analysis of phylogenetic tree, gene structure, conserved motifs, conserved domains, cis-acting elements
D. nobile NAC protein sequences were aligned using the Clustalx2.1 program, and the NGPhylogeny.fr online tool (https://NGPhylogeny.fr) was used to build a phylogenetic tree of D. nobile NAC proteins, which was subsequently ornamented using the ITOL online website (https://itol.embl.de/) (Puranik et al., 2013; Letunic and Bork, 2021; Wang et al., 2021). The same method was used to construct other phylogenetic trees. The NAC gene structure of D. nobile was mapped using the GSDS2.0 (http://gsds.cbi.pku.edu.cn/) tool to analyse its exon and intron structures (Hu et al., 2015). MEME(http://meme-suite.org/tools/meme) was used to examine the conservative motifs in the D. nobile NAC protein sequences (Bailey et al., 2009). The maximum number of conservative motifs detected was set to ten, and all other parameters were left at their default values (Bailey et al., 2006). Conserved domains in DonNAC genes were identified on NCBI’s Conserved Domain Database(https://www.ncbi.nlm.nih.gov/Structure/bwrpsb/bwrpsb.cgi) (Marchler-Bauer et al., 2015). The upstream 2000 bp sequence of D. nobile NAC CDS sequences was extracted by TBtools v1.108 software, submitted to PlantCare online website (http://bioinformatics.psb.ugent.be/webtools/plantcare/html/) for prediction of cis-acting element types, positions, and numbers, filtered and counted in Excel 2019, and the obtained cis-acting element position information was used for visualization in TBtools v1.108 (Chen et al., 2020; Hou et al., 2022; Luo et al., 2022).
Physicochemical properties and subcellular localization analysis
The ExPASY website’s ProtParam tool (http://web.expasy.org/protparam/) was used to analyze the number, molecular weight(MW), isoelectric point information(pI), total number of positively or negatively charged residues, instability index, grand average of hydropathicity(GEAVY), and aliphatic index (Xu B. et al., 2022) of amino acids in DnoNAC proteins. Predicting the subcellular localization of DnoNAC proteins by the WoLF PSORT online website (https://www.genscript.com/wolf-psort.html) (Paul et al., 2007).
Analysis of transmembrane structural domains, hydrophobicity of amino acids and the secondary and tertiary structures
The transmembrane structure of DnoNAC proteins was analyzed by TMHMMServerv.2.0 software(https://services.healthtech.dtu.dk/services/TMHMM-2.0) (Ding et al., 2019), and the hydrophilicity and hydrophobicity of DnoNAC proteins was predicted by ProtScale (https://web.expasy.org/protscale/) (You et al., 2017). The SOPMA tool (https://npsa-prabi.ibcp.fr/cgi-bin/npsa_automat.pl?page=npsa_sopma.html) was used to predict the secondary structure of DnoNAC proteins (Geourjon and Deléage, 1995). The tertiary structure of this protein family was predicted using SWISS-MODEL online software (https://swissmodel.expasy.org/) (Marco et al., 2014).
Analysis of codon bias
The necessary metrics, such as codon base composition, relative synonymous codon usage (RSCU), codon adaptation index (CAI), codon bias index (CBI) and effective number of codons (ENC) were obtained using the software CodonW1.4.2. The software TBtools v1.108 was used to create a Heatmap clustering of codons RSCU of NAC gene family in D. nobile (Wang et al., 2022a).
Collinearity analysis
The TBtools software Fasta Stats tool was used to process the genome sequence to obtain chromosome length files, the Gene Density Profile tool was used to process the gene structure annotation information to obtain gene density files, and the One Step MCScanX-Super Fast tool was used to compare the D. nobile proteins themselves to obtain blast results. The GFF3 Gene Position(Info.) Parse tool was used to acquire all genes’ positions, and the Advanced Circos tool was used to visualize the data (Krzywinski et al., 2009). The similar procedure was used to create D. nobile and Arabidopsis Circos maps. The simple Ka/Ks Calculator(NG) in Tbtools v1.120 was used to compute the nonsynonymous substitution rate(Ka), synonymous substitution rate(Ks) and selective strength(Ka/Ks) values.
Prediction of miRNAs targeting DnoNAC genes
Potential miRNAs targeting DnoNAC genes were predicted using online software psRNATarget (https://www.zhaolab.org/psRNATarget/) with default parameters (Dai and Zhao, 2011).
Analysis of SSR loci
The SSR locis contained in DnoNAC genes were analysed using MISA-web online software (https://webblast.ipk-gatersleben.de/misa/) with default parameters (Beier et al., 2017).
Results
Chromosome mapping analysis
85 NAC genes were identified in D. nobile genome by Pfam model and SMART search, which were called DnoNAC01~DnoNAC85 based on the gene descriptions. DnoNAC genes were not found on chromosome 8 and were dispersed irregularly on the other chromosomes, according to the findings of chromosomal localization analysis using Mapchart 2.32. Chromosome 19 has the most DnoNAC genes, with 11 genes, named DnoNAC01 to DnoNAC11. Chr2, Chr7 and Chr11 have the fewest DnoNAC genes, with only two family members (Supplementary Figure 1).
Phylogenetic analysis
85 members of the family was divided into 6 subfamilies based on significant amino acid sequence similarity and evolutionary closeness(designated Group1 to Group6). The DnoNACs in the 6 subfamilies vary greatly, with Group3 having the most family members, accounting for 49.41% of the total number of family members, and Groups 1 and 2 having the fewest, accounting for 1.18% of the total number of family members. Group1 and Group2 are the most primitive of the entire family, while Group6 is the fastest evolving (Figure 1A).
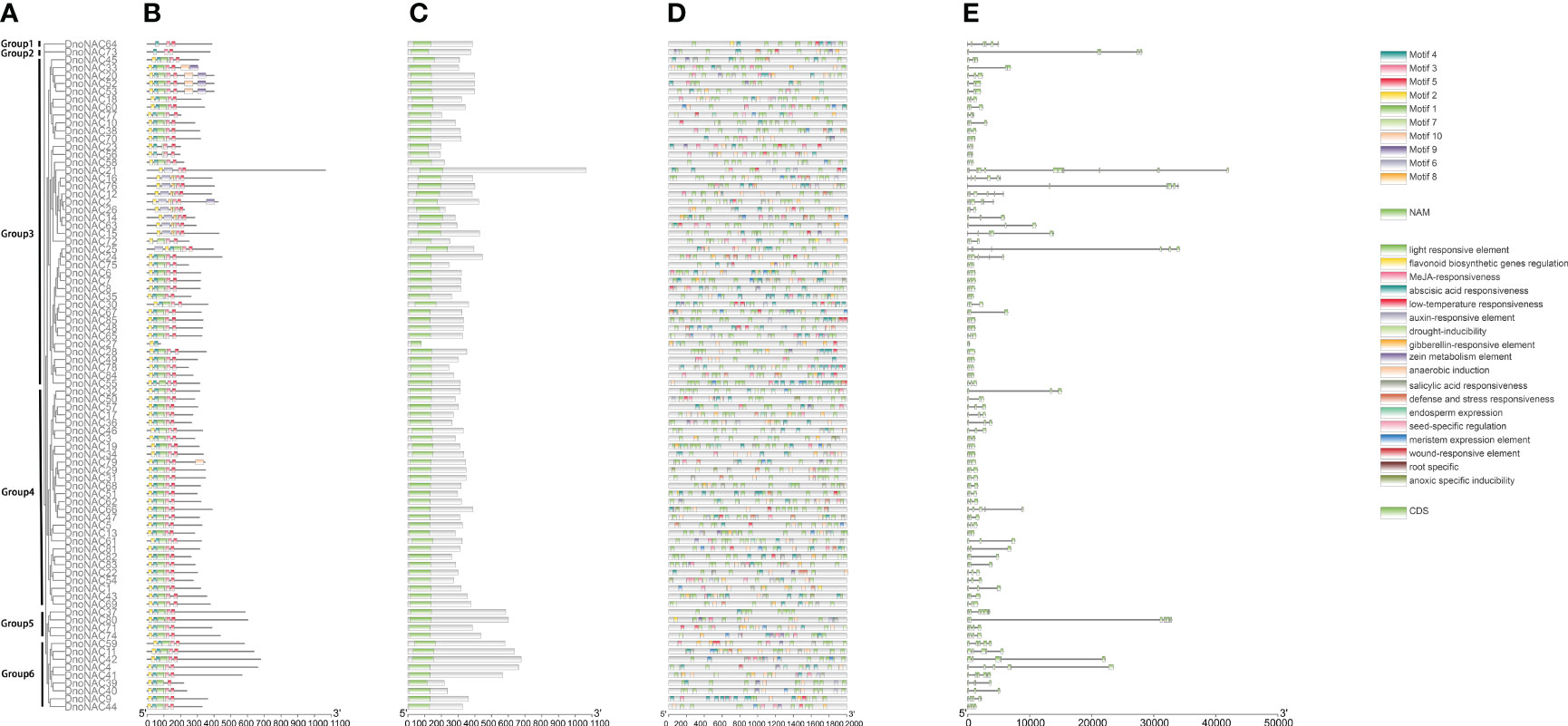
Figure 1 Phylogenetic tree, conserved motif, conserved domain, cis-acting elements and gene structure of NAC family in Dendrobium nobile. (A) phylogenetic tree of DnoNAC genes. (B) conserved motifs of DnoNAC proteins. (C) conserved domains of DnoNAC proteins. (D) cis-acting elements of DnoNAC genes’ promoter. (E) gene structure of DnoNAC genes.
The phylogenetic tree of NAC gene family in D. nobile and Arabidopsis thaliana were separated into 15 subclades (designated Group1 to Group15), with 85 DnoNAC genes dispersed on 14 subclades excluding Group4. Group15 contains the most members at 14 subclades, followed by Group5 with 11 and Group9 with 10 DnoNAC members, Group6 contains 3 DnoNAC members, Group3 and Group13 contain the least number of members at 2. In general, Group 1 members are the most primitive, while Group 15 members are the most rapidly developing (Figure 2).
The phylogenetic tree of NAC gene family in D. nobile and Dendrobium catenatum were separated into 12 subclades (designated Group1 to Group12), each containing 2 to 15 members. Group6 contains the most members at 15, followed by Group9 with 14 and Group2 with 11 DnoNAC members, Group5 contains 5 DnoNAC members, Group7 and Group11 contain the least number of members at 2. In general, Group 1 members are the most primitive, while Group 12 members are the most rapidly developing (Figure 3).
The phylogenetic tree of NAC gene family in D. nobile and Dendrobium chrysotoxum were separated into 9 subclades (designated Group1 to Group9), each containing 1 to 23 members. Group4 contains the most members at 23, followed by Group1 with 15 and Group9 with 13 DnoNAC members, Group2 contains 2 DnoNAC members, and Group7 contains the least number of members at 1. In general, Group 1 members are the most primitive, while Group 9 members are the most rapidly developing (Figure 4).
The phylogenetic tree of NAC gene family in D. nobile, Arabidopsis thaliana, Dendrobium catenatum, Dendrobium chrysotoxum, Oryza sativa subsp. Japonica, Zea mays, Populus euphratica, Populus trichocarpa, Brachypodium distachyon and Brachypodium stacei were separated into 10 subclades (designated Group1 to Group10), with 85 DnoNAC genes dispersed on 10 subclades excluding Group1 and Group7. Group6 and Group8 contain the most members at 20, followed by Group3 with 10 and Group10 with 9 DnoNAC members. Combined with the distribution of these genes on the phylogenetic tree, it is known that the D. nobile NAC gene family is most closely related to Dendrobium catenatum and Dendrobium chrysotoxum, followed by Brachypodium distachyon, and Zea mays is the most distant (Figure 5).
Gene structure analysis
The gene structural map of the D. nobile revealed that all 85 DnoNAC genes comprised introns and exons. The number of exons ranged from 2 to 13, with 60 genes having 3 exons, accounting for 70.59% of the total. The family member with the fewest exons was DnoNAC27, and the family member with the most exons was DnoNAC21 (Figure 1E).
Conservative motifs and conserved domain analysis
The conservative motifs of the 85 NAC protein sequences of D. nobile were analysed by using the online tool MEME. According to the findings, the lengths of the ten motifs ranged from 11 to 50 amino acids, 85 members included motifs in varied numbers, ranging from 2 to 8, with 83 members containing motif 2, showing that this motif 2 is more conserved in D. nobile NAC proteins (Supplementary Figure 2). Most DnoNACs’ motifs are placed in the following order, motif2, motif4, motif1, motif7, motif3, motif5 were found in most subfamilies, with the highest frequency, and are associated with the NAM structural domain, which corresponds to the five conserved substructures A to E of the N-end part of the DnoNAC transcription factor. Furthermore, there are a few DnoNACs with varying numbers and types of motifs, presumably due to mutations during evolution, which may be related to the various functions performed in the organism. (Figures 1B, C).
Cis-acting elements analysis
The screening of 18 cis-acting elements was accomplished by analyzing 2000 bp regions upstream of the promoters of DnoNAC gene family members (Figure 1D). Light responsive elements were found in 85 DnoNAC family members, with the promoter region of DnoNAC55 having the highest (31 light responsive elements)elements. Abscisic acid responsiveness element was found in 71 family members, with the promoter region of DnoNAC78 having the highest (13 light responsive elements). MeJA-responsiveness element was found in 67 family members, with the promoter region of DnoNAC54 having the highest (18 light responsive elements). Anaerobic induction element was found in 64 family members, with the promoter region of DnoNAC11 having the highest (5 light responsive elements). Gibberellin-responsive element was found in 43 family members, with the promoter region of DnoNAC17 and DnoNAC27 having the highest (3 light responsive elements). Drought-inducibility element was found in 40 family members, with the promoter region of DnoNAC15 having the highest (3 light responsive elements). Salicylic acid responsiveness element was found in 40 family members, with the promoter region of DnoNAC7 and DnoNAC68 having the highest (3 light responsive elements). Auxin-responsive element was found in 36 family members, with the promoter region of DnoNAC13, DnoNAC21, DnoNAC30, DnoNAC47, DnoNAC54 and DnoNAC65 having the highest (2 light responsive elements). Zein metabolism element was found in 36 family members, with the promoter region of DnoNAC73 having the highest (3 light responsive elements). Root specific element was only found in 1 family members, with the promoter region of DnoNAC10. Seed-specific regulation element was found in 36 family members, with the promoter region of DnoNAC18 having the highest (3 light responsive elements). Low-temperature responsiveness element was found in 31 family members, with the promoter region of DnoNAC30 having the highest (4 light responsive elements). Meristem expression element was found in 30 family members, with the promoter region of DnoNAC42 having the highest (3 light responsive elements). Defense and stress responsiveness element was found in 27 family members, with the promoter region of DnoNAC22 and DnoNAC47 having the highest (3 light responsive elements). Endosperm expression element was found in 23 family members, with the promoter region of DnoNAC19 having the highest (4 light responsive elements). Anoxic specific inducibility element was found in 9 family members, with the promoter region of DnoNAC29, DnoNAC50, DnoNAC54 and DnoNAC55 having the highest (2 light responsive elements). Flavonoid biosynthetic genes regulation element was found in 6 family members, with the promoter region of DnoNAC1, DnoNAC4, DnoNAC51, DnoNAC64, DnoNAC72 and DnoNAC80 having the highest (1 light responsive elements). Wound-responsive element was found in 5 family members, with the promoter region of DnoNAC4, DnoNAC15, DnoNAC66, DnoNAC74 and DnoNAC77 having the highest (1 light responsive elements). Members of the DnoNAC family contain a variety of cis-elements, and it is anticipated that these family members serve critical roles in D. nobile’s response to environmental stress and hormone control (Figure 6).
The analysis of transcription factor binding sites revealed that all DnoNACs exhibited a dense distribution of TFBSs in the promoter region. Based on the number of binding sites, four fundamental TFBSs were chosen for demonstration, with ERF having the most at 12,231 (Supplementary Figure 3), followed by BBR-BPC at 4,231 (Supplementary Figure 4), MYB at 3,290 (Supplementary Figure 5), and NAC having the fewest at 3,288 (Supplementary Figure 6). Three DnoNACs(DnoNAC14, DnoNAC49, and DnoNAC71) were expected to have the highest number of TFBSs. The prediction of TFBSs provides a basis for further identification and validation of target genes.
Analysis of the physicochemical properties and subcellular localization
The D. nobile NAC protein sequences were submitted to the ProtParam online program to calculate its length, Mw, pI, and other properties. The results showed that the total number of amino acids of D. nobile NAC protein family members ranged from 80 aa to 1065 aa, with DnoNAC21 having the highest number of amino acids (1065 aa) and DnoNAC27 having the lowest number of amino acids (80 aa). The average length of amino acid is 349, and the molecular weight ranges from 22.17 to 119.02 kD. Its pI ranges from 4.61 to 9.26, covering a wide range, with 29 members of its family having a theoretical isoelectric point greater than 7 and the remaining family members having a theoretical isoelectric point less than 7. As a result, the majority of members are acidic proteins. The majority of the 85 family members have instability coefficients above 40%, with only 16 members having instability index between 27.53% and 39.88%, the range of Aliphatic index of the family members between 51.78 and 86.85 indicates the wide variation in the thermal stability of the family proteins. The grand average of hydropathicity of the family members were all negative, with the greatest being -0.352 for member DnoNAC44 and the lowest being -0.918 for member DnoNAC14. 54 members have a greater number of negatively charged residues than positively charged residues, indicating a negative charge, 8 members have an equal number of negatively charged residues as positively charged residues, indicating electric neutrality, and the remaining 23 members have a greater number of positively charged residues than negatively charged residues, indicating a positive charge.
The subcellular localization results of the DnoNAC protein family shows that 70 DnoNAC members were found in the nucleus, six members are found in chloroplast (DnoNAC11, DnoNAC12, DnoNAC23, DnoNAC30, and DnoNAC69, DnoNAC80), three members were found in cytoplasm (DnoNAC26, DnoNAC74, DnoNAC78). three members were found in the mitochondria, including DnoNAC55, DnoNAC76 and DnoNAC83, three members were found in the peroxisome, including DnoNAC16, DnoNAC17 and DnoNAC43 (Table 1).
Analysis of transmembrane structure prediction and hydrophobicity of amino acids
The analysis of transmembrane structural domains of the protein members encoded by the DnoNAC protein family revealed that only DnoNAC11, DnoNAC37, DnoNAC42, DnoNAC59, DnoNAC74, and DnoNAC80 of the 85 DnoNAC family members have transmembrane structural domains, implying that the family proteins are transmembrane proteins(Table 2, Supplementary Figure 7).
The greatest hydrophobicity value of the DnoNAC protein members varied from 0.833 to 3.600, whereas the maximum hydrophilicity value ranged from -1.911 to -3.856. Based on the rule that the lower the amino acid value, the more hydrophilic, and the higher the amino acid value, the more hydrophobic. According to the trend of lower amino acid values being more hydrophilic and higher amino acid values being more hydrophobic, alanine at position 586 of DnoNAC80 has the most hydrophobic value while glutamic acid at position 160 of DnoNAC17 has the greatest hydrophilic value. The hydrophilic peaks are more frequent and dense than the hydrophobic peaks. As a result, the DnoNAC protein is relatively hydrophilic (Supplementary Table 2, Supplementary Figure 8).
Analysis of the secondary and tertiary structures
The secondary structure of the DnoNAC proteins were examined using SOPMA online program, and the results revealed that the NAC gene family’s secondary structure is mostly made of α-helix, extended strand, β-turn and random coil. Among them, the highest proportion of random coil is 38.59% (DnoNAC21) to 73.62% (DnoNAC20), the proportion of alpha helix is 12.84% (DnoNAC34) to 33.90% (DnoNAC21), the proportion of extended strand structure is 6.28% (DnoNAC20) to 21.59 (DnoNAC36), and the lowest proportion of beta turn is 1.25% (DnoNAC27) ~ 8.74% (DnoNAC24). Except for DnoNAC14, DnoNAC26, DnoNAC27, DnoNAC34, DnoNAC36, DnoNAC40, DnoNAC46, and DnoNAC54, whose secondary structure ratios were random coil > extended strand ≥ alpha helix > beta turn, the remaining 74 members’ secondary structure ratios were random coil > alpha helix > extended strand > beta turn, indicating that side chain interactions have a significant impact on DnoNAC proteins(Supplementary Table 3, Supplementary Figure 9).
The SWISS-MODEL online software was used to predict the tertiary structure of the protein members encoded by the D. nobile NAC gene family, and the family was divided into six groups based on the similarity of the projected outcomes, marked as A, B, C, D, E and F. Group A has 63 members, followed by Group C, which has 13 members, Group B has 6 family members(DnoNAC3, DnoNAC5, DnoNAC44, DnoNAC51, DnoNAC61, and DnoNAC79). Groups D, E, and F each have one family member(DnoNAC14, DnoNAC21, and DnoNAC27). In conclusion, the D. nobile NAC family members have a higher fraction of random coil, whereas other structures are scattered across the protein structure, which is consistent with the secondary structure predictions (Supplementary Figure 10).
Analysis of codon usage bias
The average content of the third base of the codon was T3s > A3s > C3s > G3s. The average GC content(GC) of the codon was 0.29-0.58, with a mean value of 0.40. GC of silent 3rd codon posit(GC3s) was 0.27-0.58, with a mean value of 0.38. According to an analysis of codon-related parameters of the D. nobile NAC gene family. Both the GC content and the GC3 mean values are less than 50%, showing that AU is utilized more frequently than GC in the codon of this family member’s coding sequence. The codon adaptiation index(CAI) varied from 0.11 to 0.22, with a mean value of 0.17, showing that the D. nobile NAC gene family has a low preference for codon selection. The frequency of optimal codons(Fop) varied from 0.31 to 0.50, with a mean value of 0.37. The codon bias index(CBI) ranged from 0.16 to 0.15, with a mean value of -0.07. The effective number of codons(ENc) varied from 45.56 to 59.70, with a mean value of 53.32, indicating that family members are more varied from one another, have relatively modest levels of expression, and show a low preference for codons when encoding amino acids. The number of synonymous codons (L_sym) ranged from 97 to 12736, with a mean of 1616.54. Total number of amino acids (L_aa) ranged from 100 to 13179, with a mean of 1674.25, and the aromaticity of protein (Aromo) ranged from 0.06 to 0.19, with a mean of 0.12 (Supplementary Table 4).
Analysis of relative synonymous codon usage
There are 27 high-use codons(RSCU>1), 13 codons of which end in U, 11 end in A, 2 end in G, and 1 end in C.(with the exception of the termination codons UAA, UGA and UAG, as well as the initiation codons AUG and UGG). In 32 low-usage codons, 16 end in C, 10 end in G, 3 end in A and 3 end in U. This indicates that the preference for high-use codons ends in U and the preference for low-use codons ends in C. In addition, the RSCU value for AGA is greater than 2, revealing that D. nobile NAC family members have a strong preference for this codon (Table 3)
The heat map clustering of codons RSCU of NAC gene family in D. nobile revealed that DnoNAC67 and DnoNAC85 were clustered separately, DnoNAC30 and DnoNAC35 were clustered together, and the remaining genes were clustered together. Members of the same clade show similar codon usage biases, and codons in the same clade have essentially identical RSCU value sizes among different members (Figure 7).
Collinearity analysis
Gene duplication can occur in a variety of ways, and gene families have been amplified primarily through segmental duplication, tandem duplication, and whole genome duplication(WGD) during organism evolution, with duplicated genes providing control over the physiological and morphological evolution of plants (Kong et al., 2010; Landis et al., 2018). The link between the gene family members was investigated further by comparing the D. nobile NAC protein sequences, which revealed 30 pairs of fragment copy genes among the 85 D. nobile NAC gene family members(connected by red lines in Figure 8). Among the 19 chromosomes, chr6 has the most fragment replication genes, with 8 pairs. Chr4, chr10, and chr17 each has one pair of fragment replication genes. There are no DnoNAC fragment replication genes in chr2, chr8, or chr11, pairs of genes within chromosomes are only present in chr13. D. nobile NAC family genes are hypothesized to have undergone some scale of fragment duplication events during evolutionary development (Figure 8).
Ka/Ks is the ratio of the non-synonymous substitution rate (Ka) to the synonymous substitution rate (Ks) of two protein-coding genes, and it may be used to evaluate if this protein-coding gene is under selection pressure. The Ka/Ks ratios were all less than 1, so the genes were subject to purifying selection in evolution (Table 4).
To further investigate the evolutionary history of NAC genes in various species, collinearity analysis of D. nobile and Arabidopsis NAC gene family was performed. The results revealing that 8 members of the D. nobile NAC gene family have a source relationship with Arabidopsis (DnoNAC27, DnoNAC33, DnoNAC34, DnoNAC35, DnoNAC46, DnoNAC54, DnoNAC58, DnoNAC72), and the most homologous gene pairs exist in chr14(connected by red lines in Figure 9). It is speculated that these gene pairs came from the same ancestor.
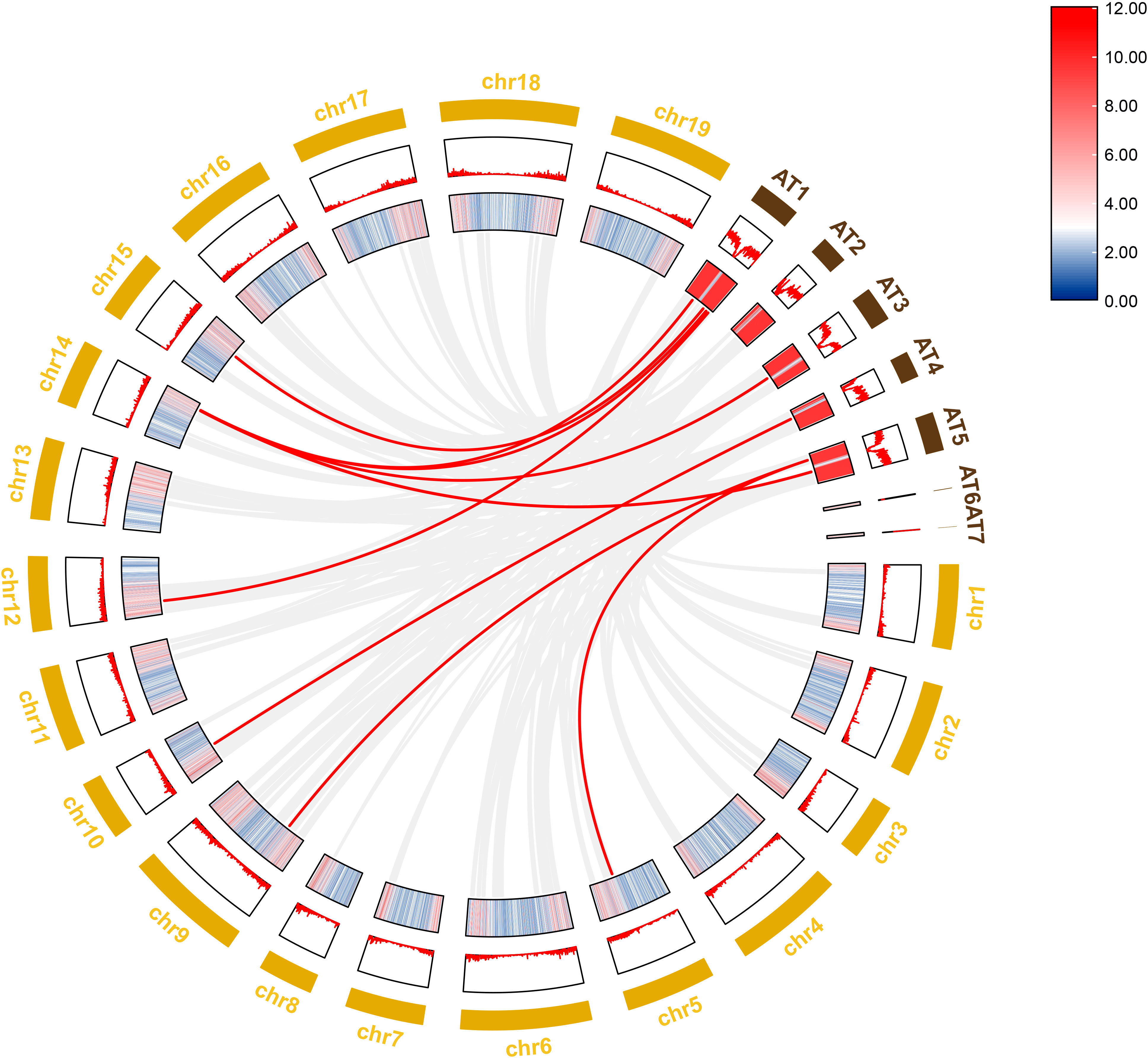
Figure 9 Collinearity analysis of NAC gene family between Dendrobium nobile (chr1–chr19) and Arabidopsis thaliana (AT1–AT7).
To further investigate the evolutionary history of NAC genes in various species, collinearity analysis of D. nobile and Dendrobium chrysotoxum NAC gene family was performed. The results revealing that 75 members of the D. nobile NAC gene family have a source relationship with Dendrobium chrysotoxum. There are no homologous gene pair in chr8, two homologous gene pairs in chr2, chr7, and chr11, three homologous gene pairs in chr1, chr3, chr4, chr5, chr15 and chr17, four homologous gene pairs in chr10, chr14 and chr18, five homologous gene pairs in chr12, six homologous gene pairs in chr6, chr9 and chr16, eight homologous gene pairs in chr13 and chr19. There are no DnoNAC fragment replication genes in chr8, pairs of genes within chromosomes are only present in chr13(connected by red lines in Figure 10). Collinearity analysis of D. nobile and Dendrobium catenatum NAC gene family reveals that 9 members of the D. nobile NAC gene family have a source relationship with Dendrobium catenatum. There is one homologous gene pair in chr3, chr5, chr7, chr9, chr10, chr16 and chr19, and two homologous gene pairs in chr12(connected by red lines in Figure 11). It is speculated that these gene pairs came from the same ancestor.
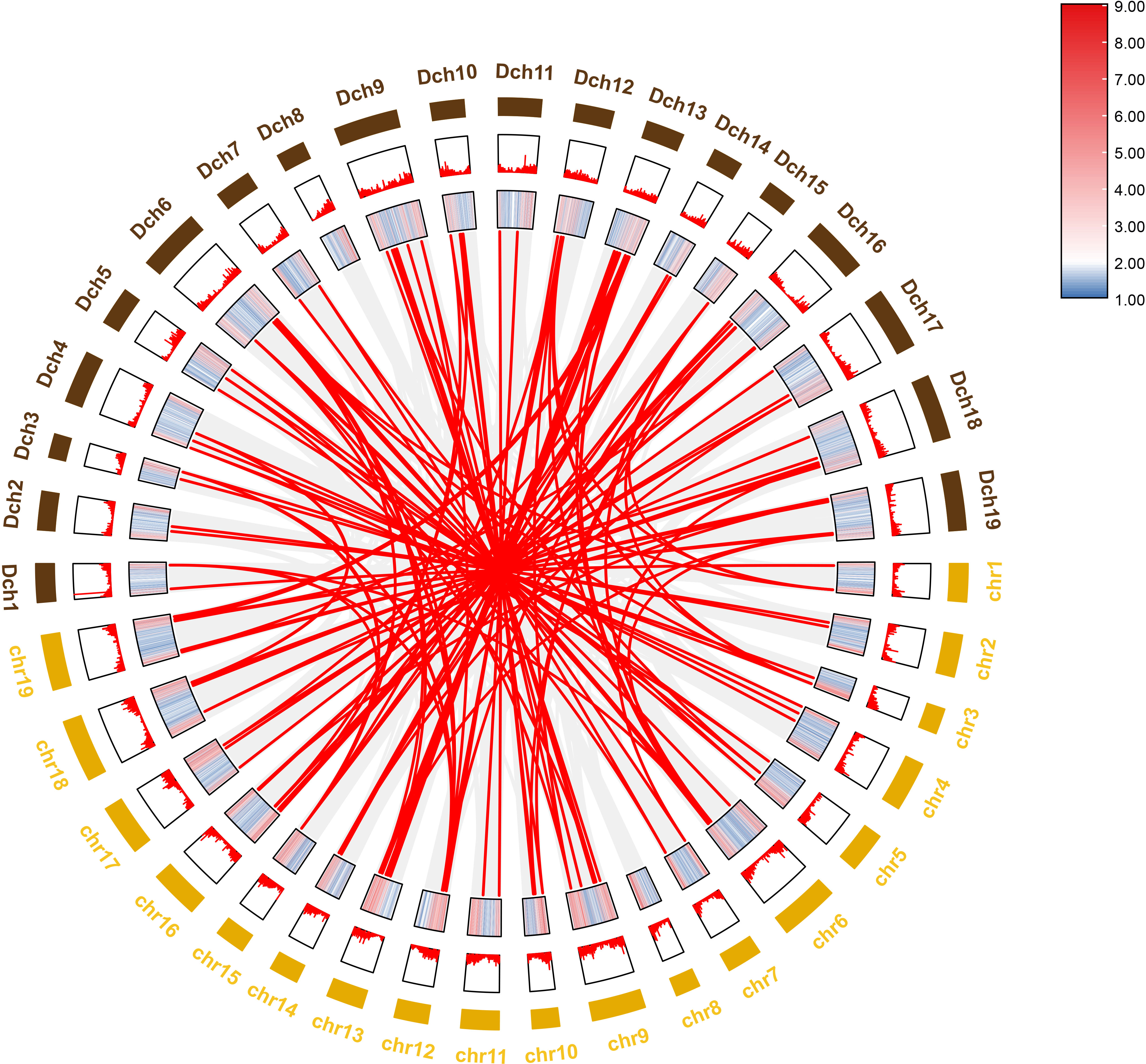
Figure 10 Collinearity analysis of NAC gene family between Dendrobium nobile (chr1–chr19) and Dendrobium chrysotoxum (Dch1–Dch19).
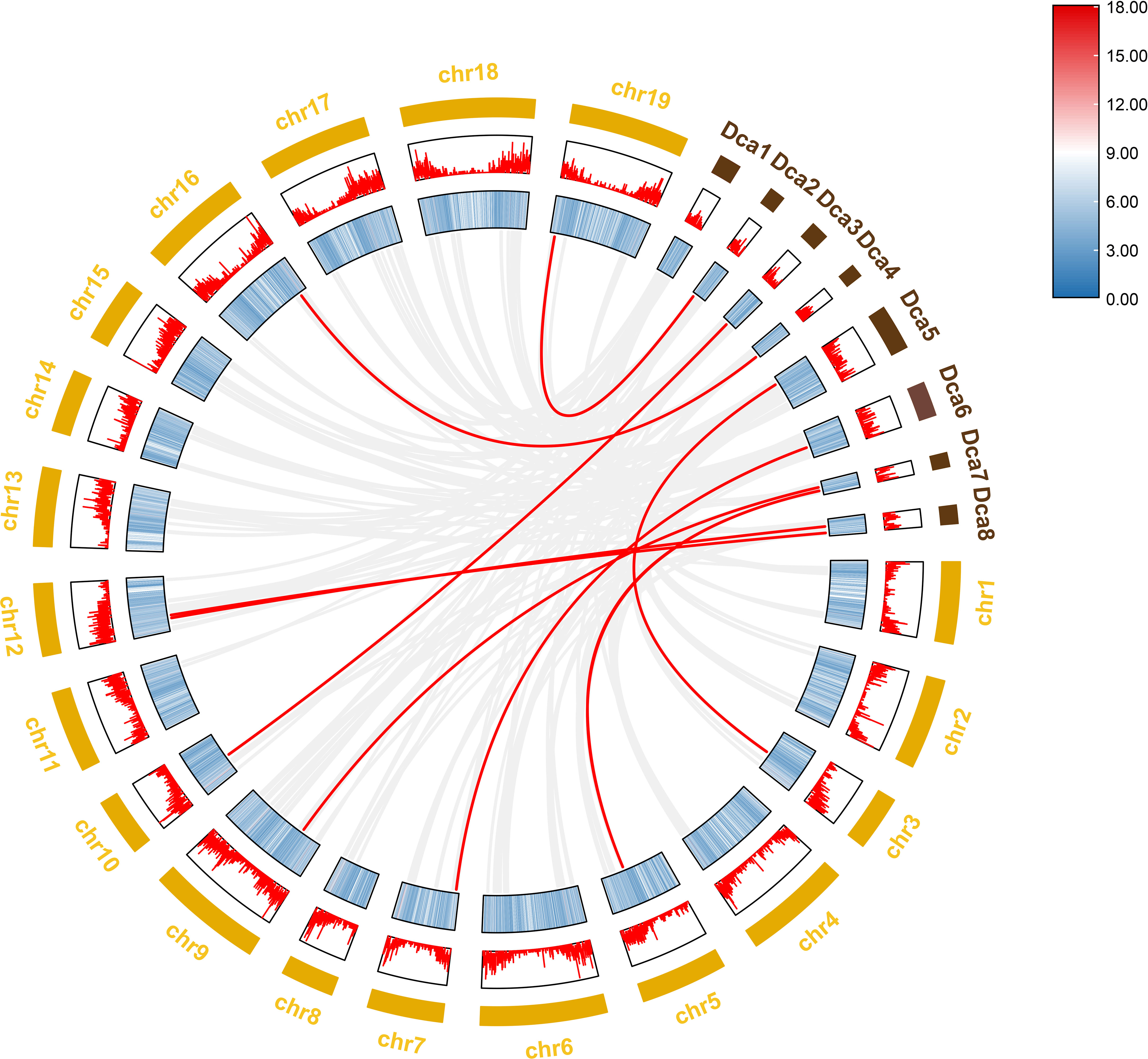
Figure 11 Collinearity analysis of NAC gene family between Dendrobium nobile (chr1–chr19) and Dendrobium catenatum (Dca1–Dca8).
Collinearity analysis was performed on D. nobile, Arabidopsis thaliana and Dendrobium chrysotoxum to explore the conservation and variability of NAC gene family members over species evolution. During the evolution of NAC family gene members in D. nobile, the conservation between D. nobile and Dendrobium chrysotoxum was greater, while the variability between D. nobile and Arabidopsis thaliana was greater. The collinear loci of NAC gene were not evenly distributed on the chromosomes. 9 collinear loci were found on the chromosomes of D. nobile with Arabidopsis thaliana, and 75 collinear loci with Dendrobium chrysotoxum (Figure 12). Collinearity analysis of D. nobile, Arabidopsis thaliana, and Dendrobium catenatum revealed that there were a few direct homologous gene pairings and high diversity within the three families (Figure 13).
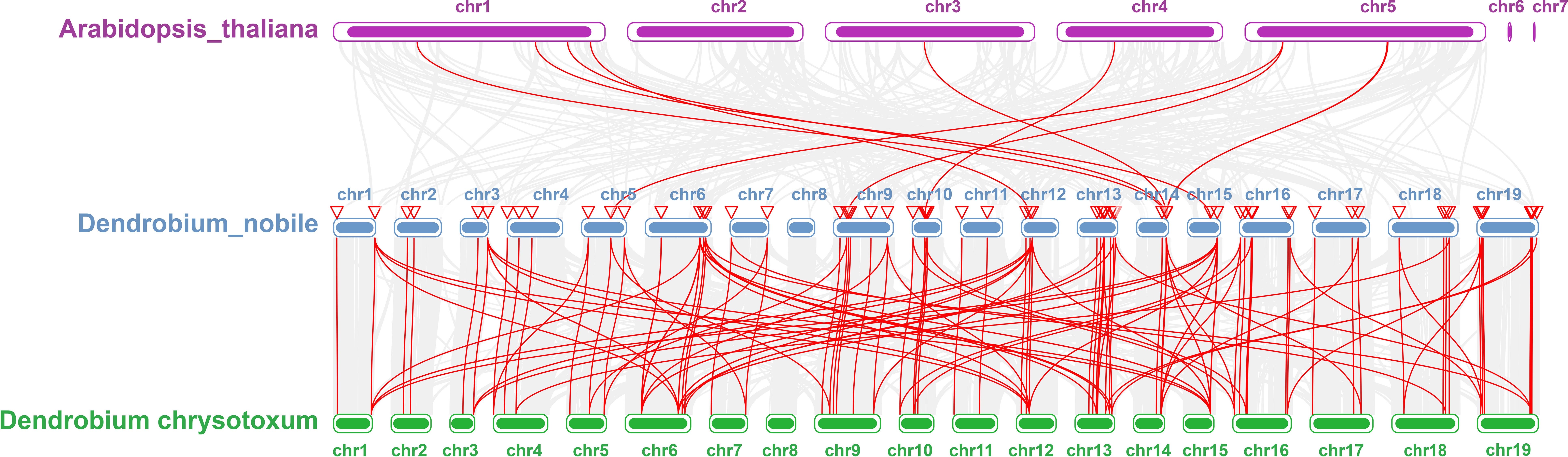
Figure 12 Collinearity analysis of NAC gene family between Dendrobium nobile (chr1–chr19), Arabidopsis thaliana (AT1–AT7) and Dendrobium chrysotoxum (Dch1–Dch19).
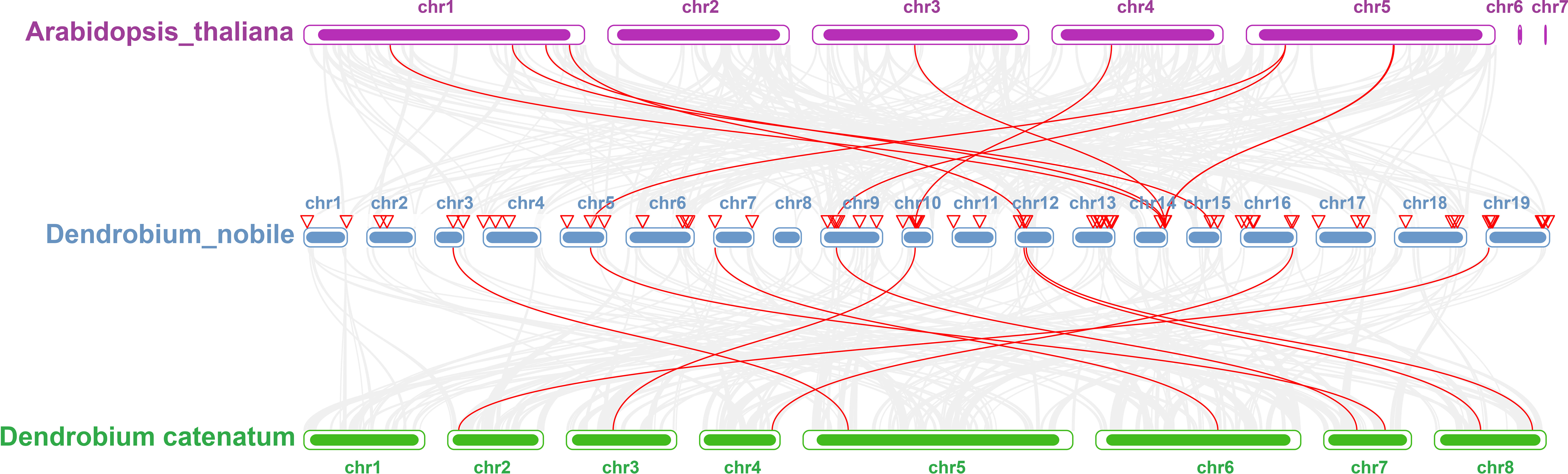
Figure 13 Collinearity analysis of NAC gene family between Dendrobium nobile (chr1–chr19), Arabidopsis thaliana (chr1–chr7) and Dendrobium catenatum (chr1–chr8).
Prediction of miRNAs targeting in DnoNAC genes
397 miRNAs were predicted to target 85 DnoNAC genes. The number of target genes of these miRNAs varied greatly, with ath-miR5021 having up to 40 target genes, ath-miR5658 having up to 39 target genes, ath-miR5640 having up to 33 target genes, but 65 miRNAs having only one (ath-miR158a-3p, ath-miR158b, ath-miR161.1, ath-miR162a-3p etc.). The majority of miRNA mature sequences (5’ – 3’) were 21 bp in length, accounting for 76.20% of all sequences. The miRNA mature sequences (5’ – 3’) were 19 bp and 26 bp in length each accounting for 0.03% of all sequences (Supplementary Table 5).
Analysis of SSR loci in DnoNAC genes
The study’s findings revealed that D. nobile SSR locis were rich in repeat types such as mononucleotide, dinucleotide, trinucleotide, hexanucleotide, and compound nucleotide. The number of each repeat type varied substantially, but mononucleotide repeats dominated, accounting for 58.91% of all SSRs with a total length of 1324 bp. The distribution of mononucleotide ssr loci displayed a clear preference, with the ssr loci number of motif A/T is 102, while the motif C/G is just 7. Dinucleotide repeats accounting for 14.59% of all SSRs with a total length of 820 bp and the dominant motif type is AT/TA. Trinucleotide repeats accounting for 11.89% of all SSRs with a total length of 417 bp. Hexanucleotide repeats accounting for 0.05% of all SSRs with a total length of 30 bp. Compound repeats accounting for 13.51% of all SSRs with a total length of 2050 bp. Overall, The majority of SSR loci in D. nobile were less than 50 bp long, accounting for 85.94% of all SSRs (Supplementary Table 6).
Expression of DnoNAC genes under salt stress and different temperature conditions
The gene expression of 85 DnoNAC genes in leaves of D. nobile seedlings under different temperature stress treatments was analyzed by qRT-PCR, and the results showed that changes in gene expression level were rather small after 48 hours of the low temperature stress treatment. After 72 hours of low temperature treatment, the expression level of DnoNAC3, DnoNAC7, DnoNAC14, DnoNAC62, DnoNAC63, DnoNAC65, DnoNAC76 and DnoNAC78 genes showed a decreasing trend, and a total of 20 DnoNAC genes with DnoNAC5, DnoNAC9, DnoNAC10, DnoNAC15, DnoNAC19, DnoNAC24, DnoNAC28, DnoNAC31, DnoNAC37, DnoNAC46, DnoNAC53, DnoNAC54, DnoNAC55, DnoNAC57, DnoNAC58, DnoNAC59, DnoNAC68, DnoNAC79, DnoNAC84 and DnoNAC85 showed an increasing trend in expression, in which the expression of DnoNAC37, DnoNAC57 and DnoNAC68 was significantly increased. The expression level of DnoNAC9, DnoNAC10, DnoNAC11, DnoNAC18, DnoNAC34, DnoNAC40, DnoNAC56, DnoNAC60, DnoNAC64 and DnoNAC72 genes increased significantly after 24 hours of high-temperature stress treatment. After 48 hours of high temperature stress treatment, the gene expression of DnoNAC16, DnoNAC21, DnoNAC42, DnoNAC59, and DnoNAC80 rose considerably. the gene expression of DnoNAC7, DnoNAC13, DnoNAC32, DnoNAC33, DnoNAC36, DnoNAC38, DnoNAC39 and DnoNAC67 showed an increasing trend after 72 hours of high-temperature stress treatment (Figure 14). In short, the NAC transcription factors may play an important part in cold and high temperature resistance in D. nobile and must be highly expressed following a period of stress.
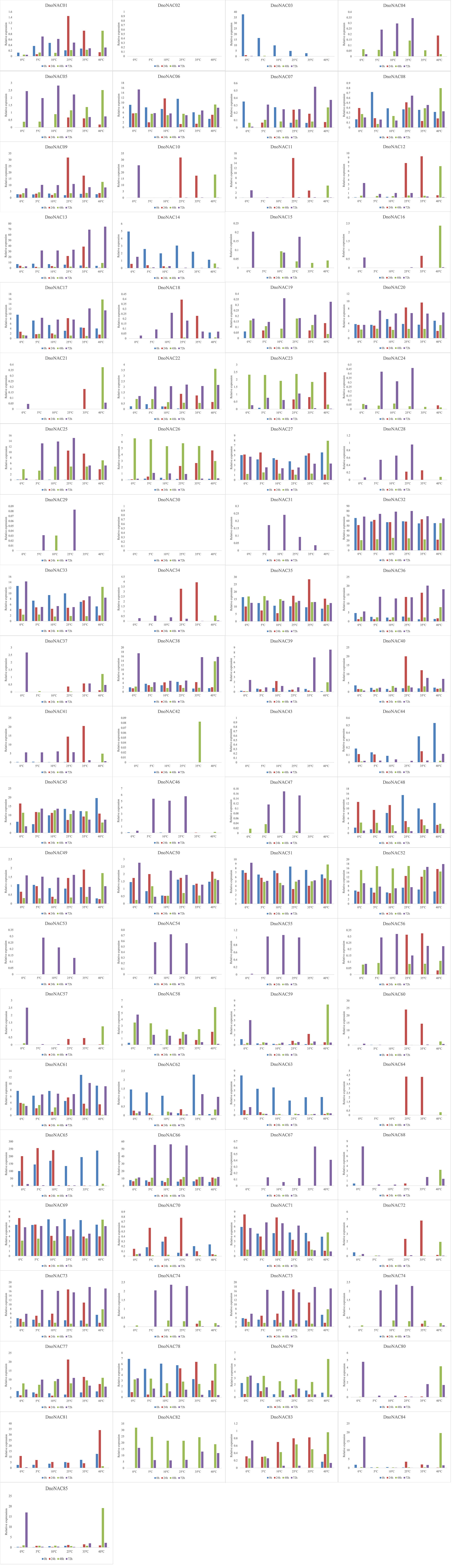
Figure 14 Expression level of DnoNAC genes at 0h, 24h, 48h and 72h under diffenrent temperature stress.
The gene expression of 85 DnoNAC genes in leaves of D. nobile seedlings under different salt concentration stress treatments was analyzed by qRT-PCR. After 24 hours of salt stress treatment, the gene expression of DnoNAC25, DnoNAC34, DnoNAC35, DnoNAC36, DnoNAC37, DnoNAC43, DnoNAC45, DnoNAC49, DnoNAC51, DnoNAC55, DnoNAC56, DnoNAC60, DnoNAC78 and DnoNAC80 had a tendency to decline with an increase in salt concentration. The gene expression of DnoNAC10, DnoNAC15, DnoNAC16, DnoNAC19, DnoNAC44, DnoNAC47, DnoNAC57, DnoNAC64 and DnoNAC70 showed a declining trend under low salt concentration stress after 48 hours of salt stress treatment. After 72 hours of salt stress treatment, there was an increasing trend in the expression of DnoNAC3, DnoNAC14 and DnoNAC50(Figure 15). The findings imply that salt stress regulates the expression of D. nobile NAC family genes, which may be crucial in response to salt stress.
The above results indicate that most members of the DnoNAC gene family in leaves of D. nobile seedlings have sensitive response mechanisms to salt stress, low temperature and high temperature stress, indicating that most members of the DnoNAC genes have molecular functions to regulate salt stress, low temperature and high temperature stress during the growth and development of D. nobile.
Discussion
Gene expression regulation is a way of regulating plant stress in response to adversity and plays a crucial role in plant growth and development, with the fundamental regulatory elements being transcription factors. Plant transcription factors have long been a focus of functional genomics research. As a trans-acting factor, it interacts with cis-elements of particular target genes to regulate various signaling pathways (Kumar et al., 2021). To date, thousands of transcription factors have been found in higher plants, with a considerable fraction being linked to resistance to or eradication of drought, high salinity, low and high temperatures (Liu and Zou, 2010) The NAC gene family is a group of transcription factors found in green plants. According to reports, there are 117 NAC genes in Arabidopsis (Ooka, 2003; Nuruzzaman et al., 2010), 151 in Rice (Yuan et al., 2019), 110 in Potato (Singh et al., 2013), 152 in Tobacco (Rushton et al., 2008), and 152 in Soybean (Le et al., 2011). In the present study, 85 NAC genes were identified in D. nobile, the number of which was lower than that of arabidopsis, rice and soybean. This discrepancy in number might be attributed to the fact that D. nobile’s genome developed without polyploidization events, which is compatible with the findings of academic investigations on the tomato NAC gene family (Jin et al., 2020).
The physicochemical properties, subcellular localization, conserved motifs, protein structure, codon bias, collinearity, phylogenetic tree construction and cis-elements of 85 D. nobile NAC transcription factor were analyzed in this study using relevant databases and software tools. The interrelationships among different D. nobile NAC gene family members were obtained.
The bioinformatics analysis of D. nobile NAC gene family members revealed that the isoelectric points of D. nobile NAC gene family members span a wide range, indicating that the encoded proteins can adapt to different acidic and alkaline environments. The total average hydrophilicity was negative, indicating that D. nobile NAC proteins are relatively hydrophilic proteins. The D. nobile NAC gene family contains 54 negatively charged residues, 8 electrically neutral residues and 23 positively charged residues, presumably the D. nobile NAC protein is negatively charged.
The highest proportion of secondary structures in this gene family is random coiled, followed by the alpha-helix and extended strand, and the least proportion of beta-turn. The overall proportions are relatively consistent and it can be assumed that D. nobile NAC family are relatively conservative in structure. The spatial conformation of the protein folding in the tertiary structure is highly conserved, but the tertiary structures of DnoNAC14, DnoNAC21, and DnoNAC27 are more distinct from the other family members, as are the corresponding conserved motifs. The tertiary structure of DnoNAC27 is the simplest, lacking a large number of conserved motifs and comprising just motif2 and motif4. The roles of DnoNAC14, DnoNAC21, and DnoNAC27 are thought to be slightly different from those of other family members. This is consistent with the findings of scientific investigations on the sesame NAC transcription factor family (Zhang et al., 2018).
According to the projected subcellular localization data, the places where D. nobile NAC transcription factors work are somewhat distributed, but the majority of members are located in the nucleus. This implies that D. nobile NAC transcription factors may behave differently in distinct subcellular locations, with the nucleus serving as the primary site of action. The precise location of each family member requires futuer investigation. This is consistent with the findings of scientific investigations on the Passiflora edulis NAC transcription factor family (Yang et al., 2022).
The analysis of codon use bias revealed that 85 family members exhibited slight preference for codon selection. Only the RSCU of AGA is greater than 2, indicating that AGA is strongly preferred by family members. The average GC3s and GC content was less than 50%, and the high-use codon preference ended in A/U(T), suggesting that A/U(T) was utilized more frequently than G/C in the coding sequence codon.
From the phylogenetic tree of NAC gene family in D. nobile, it can be seen that the D. nobile NAC gene family can be divided into 11 subfamilies and the family members are unevenly distributed in each subfamily. Combining the results of collinearity analysis and the phylogenetic tree of NAC gene family in D. nobile and Arabidopsis NAC gene family, it was discovered that there are eight pairings of genes with a high degree of similarity(DnoNAC27 and AT1G61110.1, DnoNAC33 and AT1G26870.1, DnoNAC34 and AT3G29035.1, DnoNAC35 and AT1G69490.1, DnoNAC46 and AT1G76420.1, DnoNAC54 and AT4G10350.1, DnoNAC54 and AT4G10350.1 DnoNAC58 and AT5G13180.1, DnoNAC72 and AT5G13180.1). Except for DnoNAC46 and AT1G76420.1, which are found in neighboring subclades, the remaining seven pairs of genes are found on the same subclade and are quite near together, presumably their biological functions are also similar. Based on the phylogenetic tree of D. nobile and other typical species, it is known that the D. nobile NAC gene family is most closely related to Dendrobium catenatum and Dendrobium chrysotoxum.
The cis-acting element of the promoter region regulates the exact commencement of gene transcription and transcriptional efficiency by binding to transcription factors, it can determine the core area of transcriptional activation (Wittkopp and Kalay, 2011; Ding et al., 2021; Liu et al., 2023; Rui et al., 2023). The NAC family has a substantial amount of cis-acting elements associated to light sensitivity, hormone response, biotic and abiotic stress response that are speculated to have a role in D. nobile growth and development, stress tolerance, and hormone signaling. This is consistent with the findings of Saidi et al. on the NAC gene family in other plants (Ling et al., 2017; Saidi et al., 2017; Liu et al., 2018; Wang et al., 2023).
MiRNAs regulate many genes and so act in multiple biological processes, demonstrating the complexity of miRNA control of target genes. The study identified ath-miR5021 with a high number of target genes and the majority of miRNA mature sequences(5’ – 3’) were 21 bp in length. Analysis of SSR loci through MISA-web software indicates that the fraction of mononucleotide repeats was the largest, as was the frequency of A/T. AT/GC is the prominent motif for dinucleotides. Excluding compound nucleotides, The SSR motif type grow with the number of motif, while the number of SSR loci decreases with the number of motif.
In this study, 85 DnoNAC genes in D. nobile genome were identified, with its amino acid length ranged from 80 to 1065. Its promoter region contains multiple stress responsive elements, including light responsive, gibberellin-responsive, abscisic acid responsiveness, MeJA-responsiveness and drought-inducibility elements. NAC gene family in D. nobile is most closely related to that of Dendrobium catenatum and Dendrobium chrysotoxum. The fraction of mononucleotide repeats in its SSRs was the largest, as was the frequency of A/T. These 85 DnoNAC genes contain 397 miRNAs. The collinearity analysis shows that 9 collinear locis were found on the chromosomes of D. nobile with Arabidopsis thaliana, and 75 collinear locis with D.chrysotoxum. The response mechanism of DnoNAC gene family in leaves of D. nobile seedlings to salt stress, low temperature and high temperature stress was verified by qRT-PCR experiment. These results provide a reference for further understanding the function of NAC gene family in D. nobile.
Data availability statement
The datasets presented in this study can be found in online repositories. The names of the repository/repositories and accession number(s) can be found in the article/Supplementary Material.
Author contributions
CF: Experimental design, Resources, Funding acquisition, Writing-original draft,Writing-review & editing. MYL: Investigation, Experimental operations, Formal analysis, Visualization, Writing-original draft. All authors contributed to the article and approved the submitted version.
Funding
This work was supported by Science and Technology Project of Leshan Normal University (LZD010, XJR17005, 2022SSDJS005, KYPY2023-0006).
Conflict of interest
The authors declare that the research was conducted in the absence of any commercial or financial relationships that could be construed as a potential conflict of interest.
Publisher’s note
All claims expressed in this article are solely those of the authors and do not necessarily represent those of their affiliated organizations, or those of the publisher, the editors and the reviewers. Any product that may be evaluated in this article, or claim that may be made by its manufacturer, is not guaranteed or endorsed by the publisher.
Supplementary material
The Supplementary Material for this article can be found online at: https://www.frontiersin.org/articles/10.3389/fpls.2023.1232804/full#supplementary-material
References
Aida, M. (1997). Genes involved in organ separation in Arabidopsis: an analysis of the cup-shaped cotyledon mutant. Plant Cell 9 (6), 841–857. doi: 10.1105/tpc.9.6.841
Bailey, T. L., Boden, M., Buske, F. A., Frith, M., Grant, C. E., Clementi, L., et al. (2009). MEME SUITE: tools for motif discovery and searching. Nucleic Acids Res. 37 (Web Server issue), W202–W208. doi: 10.1093/nar/gkp335
Bailey, T. L., Nadya, W., Chris, M., Li, W. W. (2006). MEME: discovering and analyzing DNA and protein sequence motifs. Nucleic Acids Res. 34 (Web Server), W369–W373. doi: 10.1093/nar/gkl198
Beier, S., Thiel, T., Münch, T., Scholz, U., Mascher, M. (2017). MISA-web: a web server for microsatellite prediction. Bioinformatics 33 (16), 2583–2585. doi: 10.1093/bioinformatics/btx198
Bengoa Luoni, S. A., Cenci, A., Moschen, S., Nicosia, S., Radonic, L. M., Sabio Y García, J. V., et al. (2021). Genome-wide and comparative phylogenetic analysis of senescence-associated NAC transcription factors in sunflower (Helianthus annuus). BMC Genomics 22 (1), 893. doi: 10.1186/s12864-021-08199-5
Chang, W. C., Chow, C. N. (2023). Database for plant transcription factor binding sites. Methods Mol. Biol. 2594, 173–183. doi: 10.1007/978-1-0716-2815-7_13
Chen, C., Chen, H., Zhang, Y., Thomas, H. R., Frank, M. H., He, Y. H., et al. (2020). TBtools: An integrative toolkit developed for interactive analyses of big biological data. Mol. Plant 13 (8), 1194–1202. doi: 10.1016/j.molp.2020.06.009
Chen, Y. J., Perera, V., Michael, W. (2013). The barley HvNAC6 transcription factor affects ABA accumulation and promotes basal resistance against powdery mildew. Plant Mol. Biol. 83 (6), 577–590. doi: 10.1007/s11103-013-0109-1
Dai, X., Zhao, P. X. (2011). psRNATarget: a plant small RNA target analysis server. Nucleic Acids Res. 39 (Web Server issue), W155–W159. doi: 10.1093/nar/gkr319
Dalman, K., Wind, J. J., Nemesio-Gorriz, M., Hammerbacher, A., Lundén, K., Ezcurra, I., et al. (2017). Overexpression of PaNAC03, a stress induced NAC gene family transcription factor in Norway spruce leads to reduced flavonol biosynthesis and aberrant embryo development. BMC Plant Biol. 17 (1), 6. doi: 10.1186/s12870-016-0952-8
Diao, P., Chen, C., Zhang, Y., Meng, Q., Lv, W., Ma, N. (2020). The role of NAC transcription factor in plant cold response. Plant Signal Behav. 15 (9), 1785668. doi: 10.1080/15592324.2020.1785668
Ding, S., Feng, X., Du, H., Wang, H. (2019). Genome-wide analysis of maize OSCA family members and their involvement in drought stress. PeerJ 7, e6765. doi: 10.7717/peerj.6765
Ding, Y., Zhu, J., Zhao, D., Liu, Q., Yang, Q., Zhang, T. (2021). Targeting cis-regulatory elements for rice grain quality improvement. Front. Plant Sci. 12, 705834. doi: 10.3389/fpls.2021.705834
Ernst, H. A., Nina Olsen, A., Skriver, K., Larsen, S., Leggio, L. L. (2004). Structure of the conserved domain of ANAC, a member of the NAC family of transcription factors. EMBO Rep. 5 (3), 297–303. doi: 10.1038/sj.embor.7400093
Geourjon, C., Deléage, G. (1995). SOPMA: significant improvements in protein secondary structure prediction by consensus prediction from multiple alignments. Cabios 11 (6), 681–684. doi: 10.1093/bioinformatics/11.6.681
Gong, D., Wu, B., Qin, H., Fu, D., Guo, S., Wang, B., et al. (2022). Functional characterization of a farnesyl diphosphate synthase from D. nobile Lindl. Amb Express 12 (1), 129. doi: 10.1186/s13568-022-01470-2
Greve, K., Cour, T. L., Jensen, M. K., Poulsen, F. M., Skriver, K. (2003). Interactions between plant RING-H2 and plant-specific NAC (NAM/ATAF1/2/CUC2) proteins: RING-H2 molecular specificity and cellular localization[J]. Biochem. J. 371 (1), 97–108. doi: 10.1042/bj20021123
Guo, S., Dai, S., Singh, P. K., Wang, H., Wang, Y., Tan, J. L. H., et al. (2018). A membrane-bound NAC-like transcription factor osNTL5 represses the flowering in oryza sativa. Front. Plant Sci. 9, 555. doi: 10.3389/fpls.2018.00555
He, J., He, X., Chang, P., Jiang, H. Z., Gong, D. P., Sun, Q. (2020). Genome-wide identification and characterization of TCP family genes in Brassica juncea var. Tumida. PeerJ 8 (3), e9130. doi: 10.7717/peerj.9130
He, X., Qu, B., Li, W., Zhao, X. Q., Teng, W., Ma, W.Y., et al. (2015). The nitrate-inducible NAC transcription factor taNAC2-5A controls nitrate response and increases wheat yield. Plant Physiol. 169 (3), 1991–2005. doi: 10.1104/pp.15.00568
Hisako, O., Kouji, S., Koji, D., Nagata, T., Otomo, Y., Murakami, K., et al. (2004). Comprehensive Analysis of NAC Family Genes in Oryza sativa and Arabidopsis thaliana. DNA Res. 6), 239–247. doi: 10.1093/dnares/10.6.239
Hou, S., Zhang, Q., Chen, J., Meng, J., Wang, C., Du, J., et al. (2022). Genome-wide identification and analysis of the GRAS transcription factor gene family in theobroma cacao. Genes-Basel 14 (1), 57. doi: 10.3390/genes14010057
Hu, B., Jin, J., Guo, A. Y., Zhang, H., Luo, J., Gao, G. (2015). GSDS 2.0: an upgraded gene feature visualization server. Bioinformatics 31 (8), 1296–1297. doi: 10.1093/bioinformatics/btu817
Hu, H., Ma, L., Chen, X., Fei, X., He, B., Luo, Y., et al. (2022). Genome-wide identification of the NAC gene family in zanthoxylum bungeanum and their transcriptional responses to drought stress. Int. J. Mol. Sci. 23 (9), 4769. doi: 10.3390/ijms23094769
Jensen, M. K., Rung, J. H., Gregersen, P. L., Gjetting, T., Fuglsang, A.T., Hansen, M., et al. (2007). The Hv NAC6 transcription factor: a positive regulator of penetration resistance in barley and Arabidopsis[J]. Plant Mol. Biol. 65 (1), 137–150. doi: 10.1007/s11103-007-9204-5
Jin, J. F., Wang, Z. Q., He, Q. Y., Wang, J. Y., Li, P. F., Xu, J. M., et al. (2020). Genome-wide identification and expression analysis of the NAC transcription factor family in tomato (Solanum lycopersicum) during aluminum stress. BMC Genomics 21 (1), 288. doi: 10.1186/s12864-020-6689-7
Kadonaga, J. T. (1998). Eukaryotic transcription: an interlaced network of transcription factors and chromatin-modifying machines. Cell 92 (3), 307–313. doi: 10.1016/S0092-8674(00)80924-1
Kim, H. J., Nam, H. G., Lim, P. O. (2016). Regulatory network of NAC transcription factors in leaf senescence. Curr. Opin. Plant Biol. 33, 48–56. doi: 10.1016/j.pbi.2016.06.002
Kong, H., Landherr, L. L., Frohlich, M. W., Mack, J. L., Ma, H., dePamphilis, C. W. (2010). Patterns of gene duplication in the plant SKP1 gene family in angiosperms: evidence for multiple mechanisms of rapid gene birth. Plant J. 50 (5), 873–885.doi: 10.1111/j.1365-313X.2007.03097.x
Krzywinski, M., Schein, J., Birol, I., Connors, J., Gascoyne, R., Horsman, D., et al. (2009). Circos: an information aesthetic for comparative genomics. Genome Res. 19 (9), 1639–1645. doi: 10.1101/gr.092759.109
Kumar, R., Das, S., Mishra, M., Choudhury, D. R., Sharma, K., Kumari, A., et al. (2021). Emerging roles of NAC transcription factor in medicinal plants: progress and prospects. 3 Biotech. 11 (10), 425. doi: 10.1007/s13205-021-02970-x
Landis, J. B., Soltis, D. E., Marx, H. E., Barker, M. S., Tank, D. C., et al. (2018). Impact of whole-genome duplication events on diversification rates in angiosperms. Am. J. Bot. 105 (3), 348–363. doi: 10.1002/ajb2.1060
Le, D. T., Nishiyama, R., Watanabe, Y., Mochida, K., Yamaguchi-Shinozaki, K., Shinozaki, K., et al. (2011). Genome-wide survey and expression analysis of the plant-specific NAC transcription factor family in soybean during development and dehydration stress. DNA Res. 18 (4), 263–276. doi: 10.1093/dnares/dsr015
Letunic, I., Bork, P. (2021). Interactive Tree Of Life (iTOL) v5: an online tool for phylogenetic tree display and annotation. Nucleic Acids Res. 49 (W1), W293–W296. doi: 10.1093/nar/gkab301
Li, M., Hou, L., Liu, S., Zhang, C. X., Yang, W. C., Pang, X. M., et al. (2021). Genome-wide identification and expression analysis of NAC transcription factors in Ziziphus jujuba Mill. reveal their putative regulatory effects on tissue senescence and abiotic stress responses. Ind. Crop Prod 173, 114093. doi: 10.1016/j.indcrop.2021.114093
Li, Y., Li, F., Gong, Q., Wu, Q., Shi, J. (2011). Inhibitory effects of Dendrobium alkaloids on memory impairment induced by lipopolysaccharide in rats. Planta Med. 77 (2), 117–121. doi: 10.1055/s-0030-1250235
Li, L., Liu, C., Wen, W., Li, Q., Pan, T., Li, Z., et al. (2022). Dendrobine biosynthesis in D. nobile in four different habitats is affected by the variations in the endophytic fungal community. Front. Microbiol. 13, 981070. doi: 10.3389/fmicb.2022.981070
Li, Z., Xiang, J., Hu, D., Song, B. (2020). Naturally potential antiviral agent polysaccharide from D. nobile Lindl. Pestic Biochem. Phys. 167, 104598. doi: 10.1016/j.pestbp.2020.104598
Li, C., Zhang, J., Zhang, Q., Dong, A., Wu, Q., Zhu, X., et al. (2022). Genome-wide identification and analysis of the NAC transcription factor gene family in garden asparagus (Asparagus officinalis). Genes-Basel 13 (6), 976. doi: 10.3390/genes13060976
Ling, L., Song, L., Wang, Y., Guo, C. H. (2017). Genome-wide analysis and expression patterns of the NAC transcription factor family in Medicago truncatula. Physiol. Mol. Biol. Pla 23 (2), 343–356. doi: 10.1007/s12298-017-0421-3
Liu, H., Chen, S., Wu, X., Li, J., Xu, C., Huang, M., et al. (2023). Identification of the NAC Transcription Factor Family during Early Seed Development in Akebia trifoliata (Thunb.) Koidz. Plants (Basel) 12 (7), 1518. doi: 10.3390/plants12071518
Liu, G. S., Li, H. L., Grierson, D., Fu, D. Q. (2022). NAC transcription factor family regulation of fruit ripening and quality: A review. Cells 11 (3), 525. doi: 10.3390/cells11030525
Liu, J., Chen, T. Y., Yuan, Y., Zhou, J. H., Zhao, Y. Y., Huang, L. Q. (2018). Bioinformatics and expression analysis of NAC transcription factor genes in scutellaria baicalensis. World J. Tradit Chin. Med. 4 (02), 5–10. doi: 10.4103/wjtcm.wjtcm_7_18
Liu, X. F., Zhu, J., Ge, S. Y., Xia, L. J., Yang, H. Y., Qian, Y. T., et al. (2011). Orally administered Dendrobium officinale and its polysaccharides enhance immune functions in BALB/c mice. Nat. Prod Commun. 6 (6), 867–870. doi: 10.1177/1934578X1100600627
Liu, C., Zou (2010). Advances in DREB transcription factors and plant abiotic stress tolerance. Biol. Bull-Us 20 (10), 26–27. doi: 10.1017/S0004972710001772
Luo, T., Song, Y., Gao, H., Wang, M., Cui, H., Ji, C., et al. (2022). Genome-wide identification and functional analysis of Dof transcription factor family in Camelina sativa. BMC Genomics 23 (1), 812. doi: 10.1186/s12864-022-09056-9
Ma, X., Zhang, Y., Tureková, V., Xue, G. P., Fernie, A. R., Roeber, B. M., et al. (2018). The NAC transcription factor slNAP2 regulates leaf senescence and fruit yield in tomato. Plant Physiol. 177 (3), 00292.2018. doi: 10.1104/pp.18.00292
Marchler-Bauer, A., Derbyshire, M. K., Gonzales, N. R., Lu, S., Chitsaz, F., Geer, L. Y., et al. (2015). CDD: NCBI's conserved domain database. Nucleic Acids Res. 43 (Database issue), D222–D226. doi: 10.1093/nar/gku1221
Marco, B., Stefan, B., Andrew, W., Konstantin, A., Gabriel, S., Tobias, S., et al. (2014). SWISS-MODEL: modelling protein tertiary and quaternary structure using evolutionary information. Nucleic Acids Res. 42 (W1), W252–258. doi: 10.1093/nar/gku340
Mitsuda, N. (2005). The NAC transcription factors NST1 and NST2 of arabidopsis regulate secondary wall thickenings and are required for anther dehiscence. Plant Cell 17 (11), 2993–3006. doi: 10.1105/tpc.105.036004
Nakashima, K., Tran, L. P., Nguyen, D.V., Fujita, M., Maruyama, K., Todaka, D., et al. (2007). Functional analysis of a NAC-type transcription factor OsNAC6 involved in abiotic and biotic stress-responsive gene expression in rice. Plant Cell Physiol. 51 (4), 617–630. doi: 10.1111/j.1365-313X.2007.03168.x
Nieuwenhuizen, N. J., Chen, X., Wang, M. Y., Matich, A. J., Perez, R. L., Allan, A. C., et al. (2015). Natural variation in monoterpene synthesis in kiwifruit: transcriptional regulation of terpene synthases by NAC and ETHYLENE-INSENSITIVE3-like transcription factors. Plant Physiol. 167 (4), 1243. doi: 10.1104/pp.114.254367
Nuruzzaman, M., Manimekalai, R., Sharoni, A. M., Satoh, K., Kondoh, H., Ooka, H., et al. (2010). Genome-wide analysis of NAC transcription factor family in rice. Gene 465 (1-2), 30–44. doi: 10.1016/j.gene.2010.06.008
Olsen, A. N., Ernst, H. A., Leggio, L. L., Skriver, K. (2005a). DNA-binding specificity and molecular functions of NAC transcription factors. Plant Sci. 169 (4), 785–797. doi: 10.1016/j.plantsci.2005.05.035
Olsen, A. N., Ernst, H. A., Leggio, L. L., Skriver, K. (2005b). NAC transcription factors: structurally distinct, functionally diverse. Trends Plant Sci. 10 (2), 79–87. doi: 10.1016/j.tplants.2004.12.010
Ooka, H. (2003). Comprehensive analysis of NAC family genes in Oryza sativa and Arabidopsis thaliana. DNA Res. 10 (6), 239–247. doi: 10.1093/dnares/10.6.239
Paul, H., Keun-Joon, P., Takeshi, O., Fujita, N., Harada, H., Adams-Collier, C. J., et al. (2007). WoLF PSORT: protein localization predictor. Nucleic Acids Res. 35 (Web Server issue), 585–587. doi: 10.1093/nar/gkm259
Pei, H. X., Ma, N., Tian, J, Luo, J., Chen, J. W., Li, J., et al. (2013). An NAC transcription factor controls ethylene-regulated cell expansion in flower petals. Plant Physiol. 163 (2), 775–791. doi: 10.1104/pp.113.223388
Puranik, S., Sahu, P. P., Mandal, S. N., VS, B., Parida, S. K., Prasad, M. (2013). Comprehensive genome-wide survey, genomic constitution and expression profiling of the NAC transcription factor family in foxtail millet (Setaria italica L.). PloS One 8 (5), e64594. doi: 10.1371/journal.pone.0064594
Puranik, S., Sahu, P. P., Srivastava, P. S., Prasad, M. (2012). NAC proteins: regulation and role in stress tolerance. Trends Plant Sci. 17 (6), 369–381. doi: 10.1016/j.tplants.2012.02.004
Ren, Y., Huang, Z., Jiang, H., Wang, Z., Wu, F., Xiong, Y., et al. (2021). A heat stress responsive NAC transcription factor heterodimer plays key roles in rice grain filling. J. Exp. Bot. 72 (8), 2947–2964. doi: 10.1093/jxb/erab027
Rui, Z., Pan, W., Zhao, Q., Hu, H., Li, X., Xing, L., et al. (2023). Genome-wide identification, evolution and expression analysis of NAC gene family under salt stress in wild emmer wheat (Triticum dicoccoides. L). Int. J. Biol. Macromol 230, 123376. doi: 10.1016/j.ijbiomac.2023.123376
Rushton, P. J., Bokowiec, M. T., Han, S., Zhang, H., Brannock, J. F., Chen, X., et al. (2008). Tobacco transcription factors: novel insights into transcriptional regulation in the Solanaceae. Plant Physiol. 147 (1), 280–295. doi: 10.1104/pp.107.114041
Saidi, M. N., Mergby, D., Brini, F. (2017). Identification and expression analysis of the NAC transcription factor family in durum wheat (Triticum turgidum L. ssp. durum). Plant Physiol. Bioch 112, 117–128. doi: 10.1016/j.plaphy.2016.12.028
Shen, Q., Qian, Z., Wang, T., Zhao, X., Gu, S., Rao, X., et al. (2022). Genome-wide identification and expression analysis of the NAC transcription factor family in Saccharum spontaneum under different stresses. Plant Signal Behav. 17 (1), 2088665. doi: 10.1080/15592324.2022.2088665
Singh, A. K., Sharma, V., Pal, A. K., Acharya, V., Ahuja, P. S. (2013). Genome-wide organization and expression profiling of the NAC transcription factor family in potato (Solanum tuberosum L.). DNA Res. 20 (4), 403–423. doi: 10.1093/dnares/dst019
Teixeira da Silva, J. A., Ng, T. B. (2017). The medicinal and pharmaceutical importance of Dendrobium species. Appl. Microbiol. Biot 101 (6), 2227–2239. doi: 10.1007/s00253-017-8169-9
Tolosa, L. N., Zhang, Z. (2020). The role of major transcription factors in solanaceous food crops under different stress conditions: Current and future perspectives. Plants-Basel 9 (1), 56. doi: 10.3390/plants9010056
Uauy, C., Distelfeld, A., Fahima, T., Blechl, A., Dubcovsky, J. (2006). A NAC gene regulating senescence improves grain protein, zinc, and iron content in wheat[J]. Science 314 (5803), 1298–1301. doi: 10.1126/science.1133649
Wang, Z., Cai, Q., Wang, Y., Li, M., Wang, C., Wang, Z., et al. (2022a). Comparative analysis of codon bias in the chloroplast genomes of theaceae species. Front. Genet. 13, 824610. doi: 10.3389/fgene.2022.824610
Wang, T., Gao, X., Chen, S., Li, D., Chen, S., Xie, M., et al. (2021). Genome-wide identification and expression analysis of ethylene responsive factor family transcription factors in Juglans regia. PeerJ 9, e12429. doi: 10.7717/peerj.12429
Wang, Z., Ni, L., Liu, D., Fu, Z., Hua, J., Lu, Z., et al. (2022b). Genome-Wide Identification and Characterization of NAC Family in Hibiscus hamabo Sieb. et Zucc. under Various Abiotic Stresses. Int. J. Mol. Sci. 23 (6), 3055. doi: 10.3390/ijms23063055
Wang, J. H., Zha, X. Q., Luo, J. P., Yang, X. F. (2010). An acetylated galactOmannoglucan from the stems of D. nobile Lindl. Carbohyd Res. 345 (8), 1023–1027. doi: 10.1016/j.carres.2010.03.005
Wang, Z., Zhang, Z., Wang, P., Qin, C., He, L., Kong, L., et al. (2023). Genome-wide identification of the NAC transcription factors family and regulation of metabolites under salt stress in Isatis indigotica. Int. J. Biol. Macromol 240, 124436. doi: 10.1016/j.ijbiomac.2023.124436
Wei, S., Gao, L., Zhang, Y., Zhang, F. R., Yang, X., Huang, D. F. (2016). Genome-wide investigation of the NAC transcription factor family in melon (Cucumis melo L.) and their expression analysis under salt stress. Plant Cell Rep. 35 (9), 1827–1839. doi: 10.1007/s00299-016-1997-8
Wittkopp, P. J., Kalay, G. (2011). Cis-regulatory elements: molecular mechanisms and evolutionary processes underlying divergence. Nat. Rev. Genet. 13 (1), 59–69. doi: 10.1038/nrg3095
Xu, B., Chen, B., Qi, X., Liu, S., Zhao, Y., Tang, C., et al. (2022). Genome-wide identification and expression analysis of rcMYB genes in rhodiola crenulata. Front. Genet. 13, 831611. doi: 10.3389/fgene.2022.831611
Xu, Q., Niu, S. C., Li, K. L., Zheng, P. J., Zhang, X. J., Jia, Y., et al. (2022). Chromosome-scale assembly of the Dendrobium nobile genome provides insights into the molecular mechanism of the biosynthesis of the medicinal active ingredient of dendrobium. Front. Genet. 13, 844622. doi: 10.3389/fgene.2022.844622
Yang, Q., Li, B., Rizwan, H. M., Sun, K., Zeng, J., Shi, M., et al. (2022). Genome-wide identification and comprehensive analyses of NAC transcription factor gene family and expression analysis under Fusarium kyushuense and drought stress conditions in Passiflora edulis. Front. Plant Sci. 13, 972734. doi: 10.3389/fpls.2022.972734
You, H., Yuan, Y., Li, C., Zhang, L. (2017). Cloning and expression analysis of MYB homologous gene pwMYB20 from picea wilsonii. Scientia Silvae Sinicae 53 (5), 23–32. doi: 10.11707/j.1001-7488.20170504
Yuan, X., Wang, H., Cai, J., Bi, Y., Li, D., Song, F. (2019). Rice NAC transcription factor ONAC066 functions as a positive regulator of drought and oxidative stress response. BMC Plant Biol. 19 (1), 278. doi: 10.1186/s12870-019-1883-y
Zhang, Y., Li, D., Wang, Y., Zhou, R., Wang, L., Zhang, Y., et al. (2018). Genome-wide identification and comprehensive analysis of the NAC transcription factor family in Sesamum indicum. PloS One 13 (6), e0199262. doi: 10.1371/journal.pone.0199262
Zhao, Y., Sun, J., Xu, P., Zhang, R., Li, L. G. (2014). Intron-mediated alternative splicing of wood-associated nac transcription factor1b regulates cell wall thickening during fiber development in populus species. Plant Physiol. 164 (2), 765–776. doi: 10.1104/pp.113.231134
Keywords: Dendrobium nobile, NAC gene family, genome-wide identification, molecular evolution, collinearity analysis
Citation: Fu C and Liu M (2023) Genome-wide identification and molecular evolution of NAC gene family in Dendrobium nobile. Front. Plant Sci. 14:1232804. doi: 10.3389/fpls.2023.1232804
Received: 16 June 2023; Accepted: 31 July 2023;
Published: 21 August 2023.
Edited by:
Raju Datla, Global Institute for Food Security (GIFS), CanadaReviewed by:
Cheng Qin, Zunyi Vocational and Technical College, ChinaJie Liu, Zunyi Medical University, China
Copyright © 2023 Fu and Liu. This is an open-access article distributed under the terms of the Creative Commons Attribution License (CC BY). The use, distribution or reproduction in other forums is permitted, provided the original author(s) and the copyright owner(s) are credited and that the original publication in this journal is cited, in accordance with accepted academic practice. No use, distribution or reproduction is permitted which does not comply with these terms.
*Correspondence: Chun Fu, NTEzMzI1ODUwQHFxLmNvbQ==
†These authors have contributed equally to this work