- 1Department of Botany, Stress Physiology Phenomic Centre, University of Karachi, Karachi, Pakistan
- 2Mid-Florida Research and Education Center, Environmental Horticulture Department, Institute of Food and Agricultural Science, University of Florida, Apopka, FL, United States
- 3Institute of Oceanography, College of Geography and Oceanography, Minjiang University, Fuzhou, China
Abiotic stress is one of the major constraints which restrain plant growth and productivity by disrupting physiological processes and stifling defense mechanisms. Hence, the present work aimed to evaluate the sustainability of bio-priming salt tolerant endophytes for improving plant salt tolerance. Paecilomyces lilacinus KUCC-244 and Trichoderma hamatum Th-16 were obtained and cultured on PDA medium containing different concentrations of NaCl. The highest salt (500 mM) tolerant fungal colonies were selected and purified. Paecilomyces at 61.3 × 10-6 conidia/ml and Trichoderma at about 64.9 × 10-3 conidia/ml of colony forming unit (CFU) were used for priming wheat and mung bean seeds. Twenty- days-old primed and unprimed seedlings of wheat and mung bean were subjected to NaCl treatments at 100 and 200 mM. Results indicate that both endophytes sustain salt resistance in crops, however T. hamatum significantly increased the growth (141 to 209%) and chlorophyll content (81 to 189%), over unprimed control under extreme salinity. Moreover, the reduced levels (22 to 58%) of oxidative stress markers (H2O2 and MDA) corresponded with the increased antioxidant enzymes like superoxide dismutase (SOD) and catalase (CAT) activities (141 and 110%). Photochemical attributes like quantum yield (FV/FM) (14 to 32%) and performance index (PI) (73 to 94%) were also enhanced in bio-primed plants in comparison to control under stress. In addition, the energy loss (DIO/RC) was considerably less (31 to 46%), corresponding with lower damage at PS II level in primed plants. Also, the increase in I and P steps of OJIP curve in T. hamatum and P. lilacinus primed plants showed the availability of more active reaction centers (RC) at PS II under salt stress in comparison to unprimed control plants. Infrared thermographic images also showed that bio-primed plants were resistant to salt stress. Hence, it is concluded that the use of bio-priming with salt tolerant endophytes specifically T. hamatum can be an effective approach to mitigate the salt stress cosnequences and develop a potential salt resistance in crop plants.
Introduction
The twin goals of ensuring global agricultural productivity and its execution in a sustainable manner are challenged due to the increased incidence of ecological catastrophes (Ebert and Engels, 2020). As a result, our agriculture system is frequently subjected to both biotic and abiotic stress. In the last few decades, a number of studies have been reported the effect of abiotic and biotic stressors on crops (Chinnusamy et al., 2005; Kwon et al., 2009; Fizza et al., 2021; Ansari et al., 2022), highlighting the alternate means of controlling the negative impacts of such stressors and sustain plant growth in a sub-optimal environment. Moreover, out of many environmental fluctuations, soil salinization has become a fundamental enigma as it has been encountered in all climates. The assault of this salinity stress, which is mainly caused by sodium ions, can be observed in the germination, growth, development, and reproduction of the crop (Mahmood et al., 2021). Hence, soils are rendered hypersaline due to the prevalence of NaCl by natural or anthropogenic means, which decreases crop production by more than 20% (Porcel et al., 2012). In response to salt stress, plants show plasticity in terms of periodic adjustment like osmolyte synthesis due to physiological modifications in their defensive metabolism (Nephali et al., 2021). However, the strategies to adapt salt tolerance in crops have become insufficient to overcome extreme salinity (Augé et al., 2014). Thus, to mitigate the salt stress and sustain the modern agriculture system, various biotechnological approaches have been employed to ensure crop productivity.
Among such approaches, bio-priming has been considered an innovative and sustainable method for alleviating plant salt stress. Seed bio-priming is a strategy of seed treatment (seed priming) for regulating plant growth, managing stress, and improving seed germination (Sarkar et al., 2021). Moreover, seed priming alone (osmo-priming, matrix priming) or in combination with a low dosage of biocontrol agents have been reported to increase the germination rate, uniformity and sustainability of plant growth and development under sub-optimal environment (Johnson and Puthur, 2021). However, Seed priming via conventional and specifically chemical means impaired the soil ecosystem, which creates fluctuations in the food chain. Therefore, seed bio-priming with plant growth-promoting microbes (PGPM) that are naturally colonized around the root zone of the plants has a great potential to increase the plant’s performance in a suboptimal environment (Dimkpa et al., 2009).
In addition, it is currently being recognized that the application of endophytes offers a great potential to reduce the abiotic and biotic stress in plants. Lately, the application of endophytes to reduce the hypersaline stress in plants has also been reported (Sandhya et al., 2009; Yao et al., 2010; Verma et al., 2021). Several studies suggested that the endophytes sustained growth by increasing the uptake of nutrients such as zinc, phosphorus, boron and copper and making other nutrients available to plants in a saline-sodic soil (Sarma et al., 2015; Liu et al., 2017).
Paecilomyces lilacinus and Trichoderma hamatum are endophytic saprophyte fungus that can be found in different soil types and have the ability to grow in a broad range of soil pH having sodium ions. P. lilacinus is effectively used to control nematode growth as it has the ability to penetrate and destroy the embryo. Similarly, T. hamatum is a beneficial endophytic plant symbiont, compared to P. lilacinus which is widely used to control fungal diseases in crop plants (Afzal et al., 2013). Some reports indicate that that Trichoderma enhanced the tolerance to abiotic stress in plants (Shoresh et al., 2010; Estrada et al., 2013). However, the role of P. lilacinus in plants to enhance stress tolerance against abiotic stress has not been reported so far. Hence, the present study aimed to probe the application of P. lilacinus and T. hamatum as an effective bio-priming agent in crop plants against hypersaline environment.
Plant photosynthesis coupled with defense mechanisms are the prime physiological modulations that indicate the health status of the crops. The thorough analysis of the photosynthetic apparatus via non-destructive approach like chlorophyll fluorescence can mimic the real time changes in perturbation and light harvesting efficiency of the photosynthetic membrane. Furthermore, light harvesting complexes and reaction centers of PS II are not only true source of energy production but also plays a crucial role to stress tolerance under abiotic stresses. Therefore, the present study evaluated the sustainable role of isolated endophytes through seed-priming on photo-physiology, light harvesting efficiency, energy fluxes, and subsequent antioxidant system in two important crops, under a suboptimal environment. Also knowing that the energy exploitation in the photosynthetic apparatus of bio-primed plants during salt stress tolerance has not been documented so far. Likewise the application of T. hamatum and P. lilacinus as a bio-priming agent to enhance salt tolerance in plants is yet to be studied In essence, the current research was designed to scrutinize the energy distribution inside the photosynthetic membrane by non-destructive means to explicate the energy source for the induction of salt tolerance in plants due to bio-stimulating natural colonizers i.e., T. hamatum and P. lilacinus.
Materials and methods
Seed source and selection
Seeds of Wheat (Triticum aestivum) and Mung bean (Vigna radiata) were collected from the Stress Physiology Phenomic Centre, Department of Botany, University of Karachi, and surface sterilized into 10% NaClO (sodium hypochlorite) for 3 min to remove the surface fungus and dust. Seeds were then thoroughly washed with distilled water to remove NaClO traces.
Collection and purification of beneficial endophytic fungi
The plant-beneficial fungal endophytic fungi P. lilacinus and T. hamatum were obtained from Karachi University Culture Collection (KUCC) and purified on PDA (Potato Dextrose Agar) with several replicates. Saline medium of PDA was prepared to examine the salt tolerance of P. lilacinus and T. hamatum, having several concentrations of NaCl (100, 200, 300, 400, and 500 mM) in its composition. These sets were kept at room temperature 30-34 ± 2°C for 7 days to select salt-tolerant endophytic strains and later it was used for further study (Figure 1). The Colony-forming unit (CFU) was maintained at 61.3 × 10-6 Conidia/ml of Paecilomyces and about 64.9 × 10-3 Conidia/ml of Trichoderma colony forming units (CFU) per milliliter for liquid as:
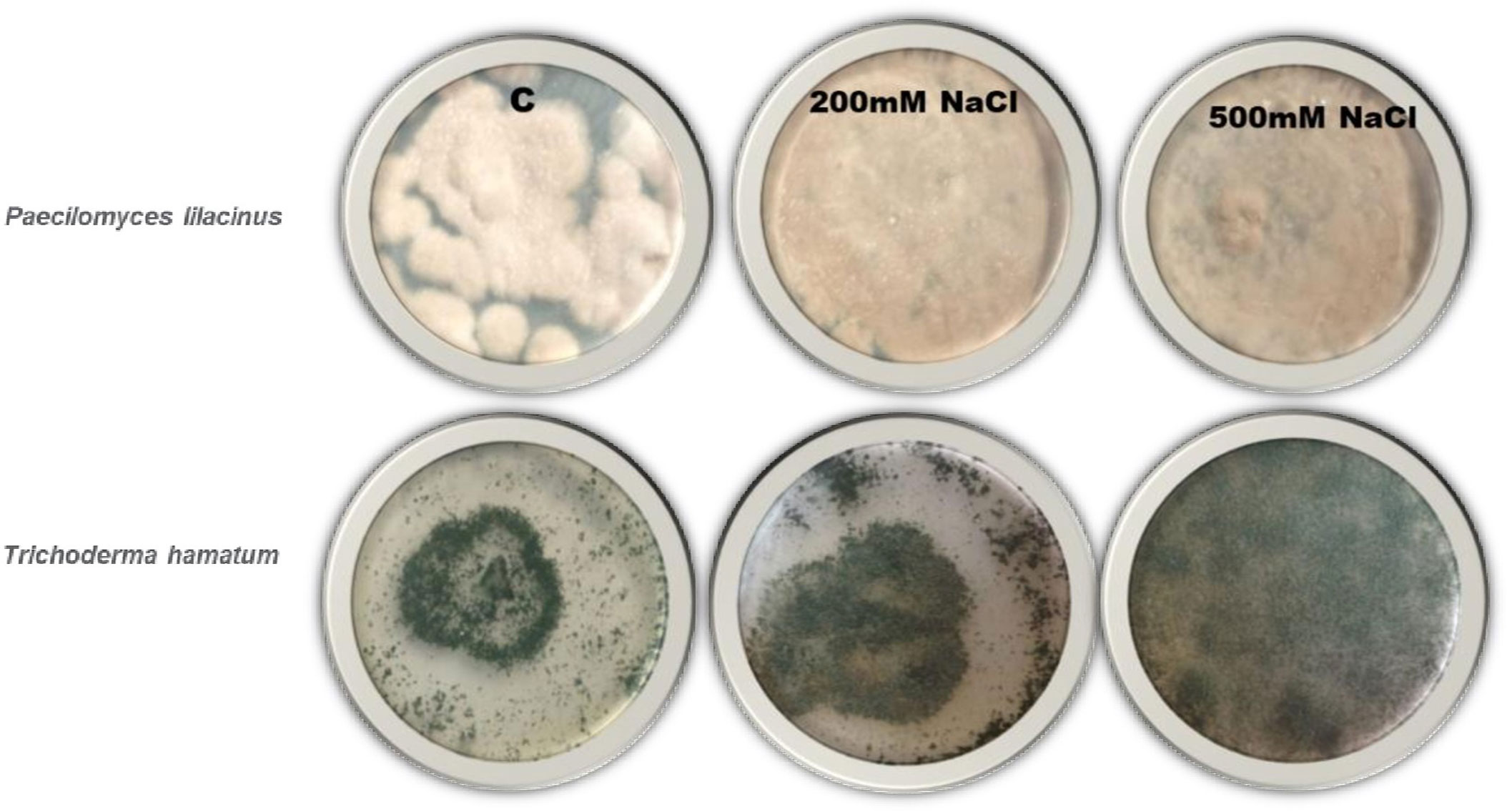
Figure 1 Endophytes culture on high saline medium to use in plant. Highest salt tolerance endophytes culture was used in further study.
Inoculation of fungal endophytes by seed priming technique
The endophytic fungi P. lilacinus and T. hamatum were inoculated in plants by seed bio-priming technique as described by Saeid et al. (2018). Seeds of Wheat and Mung bean were selected for the inoculation of endophytes. The fungal suspension was prepared from pure PDA cultures by adding 10 ml of sterile distilled water into fungal plates. Plates were slightly scratched by a wire loop and fungal suspension was poured into a beaker (the process was repeated twice). The final volume was made up to 100 ml with sterile distilled water to make the stock. From the fungal spore stock, 25 ml was taken and made up the volume up to 100 ml with sterile distilled water to prepare 25% fungal suspension. Later, the surface sterilized and dried seeds of both crops were treated by soaking in the spore suspensions prepared for different time intervals (5, 10, and 15 min). The seeds were dried under a sterile air stream in laminar air flow for 2 h (Singh et al., 2013).
Experimental design and stress application
The experiment was conducted at the Stress Physiology Phenomic Center, Department of Botany, University of Karachi, Pakistan. Under natural environmental conditions, the experiments were organized in a completely randomized design to analyze endophytic symbiosis with the crop plant. Two sets of experiments were conducted, 1) Seeds without inoculation of P. lilacinus and T. hamatum and 2) Seeds with inoculation of P. lilacinus and T. hamatum. Ten treated seeds were sown per pot having 1 Kg of soil and allowed to germinate. The composition of the soil is 80.5% sand particles, 7.1% silt and 8.1% clay, 4.10% organic carbon, 0.83% total nitrogen, pH 7.6, and electrical conductivity was 1.7 dS.m-1. Wheat and Mung bean were allowed to grow at an average day-night temperature of 33 ± 4°C to 22 ± 3°C. Twenty days old inoculated and un-inoculated seedlings were treated with different salt concentrations by gradual increment method to reach 100 and 200 mM NaCl. In this regard, 50 mM for 200 mM and 25 mM for 100 mM was given on alternate days. The moisture level was maintained by adding up water as stated by Umar and Siddiqui (2018). The whole setup of the experiment was repeated with four replicates of treatments and controls. The plants were exposed to saline treatments for 7 days and later plants were harvested.
Relative water content
For the calculation of Relative water content (RWC) Barrs and Weatherley (1962) method was applied with some modifications. Randomly selected leaves of each control and treated samples of an area of 4 × 2 cm2 of wheat and 1.2 cm2 of Mung bean were excised from the mid-veins and the edge section and fresh weight (FW) was recorded. Later, leaves were kept in Petri plates of 90 mm diameter for12 h, which contain distilled water. Afterward, the leaves samples were taken off the Petri plate and turgid weight (TW) were recorded. For the measurement of the dry weight (DW), leaves samples were oven dried at 80°C for 48 hours. RWC was calculated by using the formula:
Stomatal conductance and chlorophyll content index
For the observation of stomatal conductance, young randomly selected leaves of Wheat and Mung bean from each treated and control intact plant was used between 9:00 A.M. and 11:00 A.M. For this investigation, Decagon Leaf Porometer (Model SC-1) was used, and data were recorded from the middle and lower part of the leaf surface. The stomatal conductance of leaf was expressed as mmole m-2s-1. Similarly, the chlorophyll content index (CCI) of young leaves of each treated and control intact plant leaf was recorded between 9:00 A.M. and 11:00 A.M. Chlorophyll Content Meter CCM-200; Opti-Sciences Inc., Hudson, NH, USA was used. The average values of ten leaves of each replicate were used to show in bar graphs.
Photochemical traits of photosystem II
For the photochemical traits of Photosystem II assessment, chlorophyll fluorescence was recorded by using as Opti-Sciences Fluorometer (Model OS-30 p+; Hudson, USA). For the analysis, the youngest and fully expended leaves were selected between 9:00 A.M. and 11:00 A.M. From intact plants, leaves were clipped for 60 min for dark-adapted measurement from each treatment and control plant. Light-adapted quantum yield was recorded under a normal day-light environment. Performance index (PIABS), Original (FO), and maximum (FM), the dark-adapted quantum yield of PS II photochemistry was calculated by the ratio of variable to maximum fluorescence (FV/FM), photochemical quenching (qP), and JIP test data was used to calculate as described by Strasser et al., 2010; Stirbet and Govindjee, 2011 (Supplementary Table 1).
IR thermal images
FLIR-E5 (FLIR Systems, USA) was used before harvesting. IR thermal sensor observed the infra-red thermography from each Wheat and Mung bean treated and control plant. Before the measurement, the system was optimized for 60-90 min, and later on, images were taken. A computerized report was generated using FLIR Software 2.10 after transferring the images into the computer.
H2O2 content
Total hydrogen peroxide (H2O2) content was estimated according to the method described by Velikova et al. (2000). Freshly harvested leaf samples were homogenized in 3 ml of 0.1% (w/v) trichloroacetic acid (TCA) in an ice bath. Afterward, homogenate was centrifuged at 12000 rpm for 15 min. Later on, 0.5 mL of 10 mM phosphate buffer (pH 7.0) and 1 ml of 1 M potassium iodide (KI) were mixed with 0.5 ml of supernatant. Optical density of the supernatant was taken at 390 nm. H2O2 content was estimated with reference to a standard curve and expressed in mmole g-1 FW.
Where,
Ve = Volume used for the estimation, R = Reading from the standard curve, D.F = Dilution factor, Vs = Volume of extract, W =Weight of leaf sample.
Malondialdehyde content
Lipid peroxidation in the leaf tissues was observed by Dhindsa et al. (1981), the amount of malondialdehyde (MDA) produced by the reaction of Thio-barbituric acid (TBA). Freshly harvested leaves samples of 0.25 g were homogenized with 0.1% trichloroacetic acid (TCA) in a pestle and mortar and centrifuged at 10,000 rpm for 5 min. 1mL supernatant was added into 4 ml of 20% TCA containing 0.5% TBA. The mixture was heated for 30 min in a water bath at 95 °C and allowed to cool. Absorbance was recorded at 532 and 600 nm. MDA-TBA extinction co-efficient was recorded at 532 nm.
Antioxidant enzymes activity
Leaf sample of 500 mg in liquid nitrogen (5°C) was homogenized with 10 ml of abstraction buffer (Tris-HCl pH 6.8, 10 ml DDT, 0.1 mM EDTA, 50 mg PVP) for enzymatic antioxidant evaluation. The mixture was centrifuged at 15,000 rpm for 10 mins to estimate total protein by the method described by Bradford (1976). The antioxidant enzymes i.e., Superoxide Dismutase (EC # 1.15.1.1) and catalase (EC # 1.11.1.6) was measured by the method of Beyer and Fridovich (1987) and Patterson et al. (1984), respectively.
Statistical analysis
The data generated from the treated and control groups were subjected to statistical analyses using the software SPSS Version 20 (IBM, United States). The Bonferroni Post- hoc test was applied to differentiate significant differences among the mean values of different treatments and presented as small alphabets on the bar graphs (p< 0.05).
Results
Morphological response of plants against different priming treatments
In the sub-optimal environment, seedling length of wheat and mung bean plants was significantly reduced compared to control (Figure 2). It was evident from the data that the maximum reduction in root and shoot length was observed in wheat (13.83 and 17.4 cm) and mung bean (6.77 and 13.5 cm) plants when exposed to 200 mM salt stress. However, bio-priming with T. hamatum and P. lilacinus alleviates the salt stress and thus increases the seedling length of wheat from 26 to 149% and mung bean from 5 to 216% (Supplementary Table 2). It was observed that bio-priming agents results in a more profound increase in the root length as compared to the shoot length. However, general trend shows that the increase in priming duration such as 5, 10, and 15 minutes had a positive impact on the shoot length in both plants compared to root length. Unlike, the percentage of root length with respect to time duration was slightly increased in bio-primed treated wheat (20 to149%) and substantially increased in mung bean plants (66 to 285%) under 200 mM salt stress (Figure 2, Supplementary Table 2). Among all the treatments, the highest root-to-shoot ratio was observed in mung bean plants when it was primed with T. hamatum (141 to 209%) salt stress, followed by P. lilacinus (57 to 157%) under 200 mM salt stress. However, the root to shoot ratio was comparatively much lower in wheat plants compared to mung bean (Figure 2).
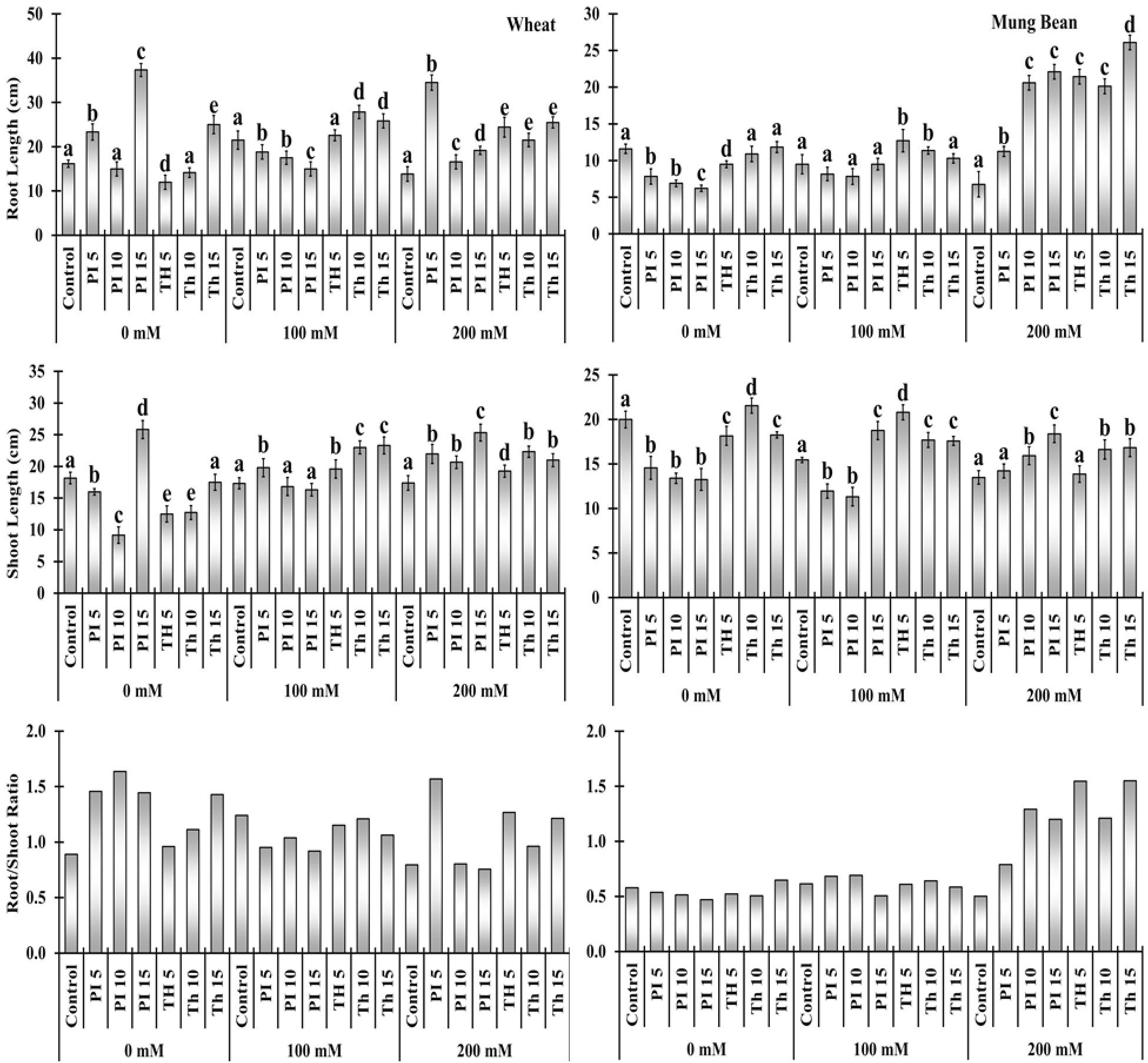
Figure 2 Effects of bio- priming with fungal endophytes on Root Length (RL), Shoot Length (SL) and Root/Shoot ratio of wheat and mung bean grown under saline environment Note: The symbols on the horizontal axis represents: Control: Seeds without priming, Pl = Seed priming with Paecilomyces lilacinus, Th = Seed priming with Trichoderma hamatum 5, 10, 15 = duration of bio-priming in minute, 0, 100 & 200 mM NaCl concentration. On bars, vertical lines represent ± Mean Standard Error (S.E) and similar alphabets represents non-significant difference between the the means of treatment at p<0.05.
Chlorophyll content index and stomatal conductance
Salt stress substantially reduced the chlorophyll content index (CCI) and stomatal conductance of the unprimed plants in comparison to the primed. Bio-priming with T. hamatum significantly increased CCI over control in wheat plants with an increase in priming duration, which was about 141 to 285% under 100 mM and 81 to 189% in 200 mM salinity (Figure 3, Supplementary Table 2). Moreover, P. lilacinus priming had a substantially negative effect on wheat plants at 100 mM salt stress, displaying a decline in CCI percentage over control (-43, -42 and -44%) but substantially increased the CCI content of wheat plants over control under 200 mM salt stress (44, 83, and 362%). P. lilacinus expressed more profound effect on the mung bean plants compared to wheat, had significantly increased the CCI at both 100 and 200 mM salt stress (47 - 170%and 35 - 61%).
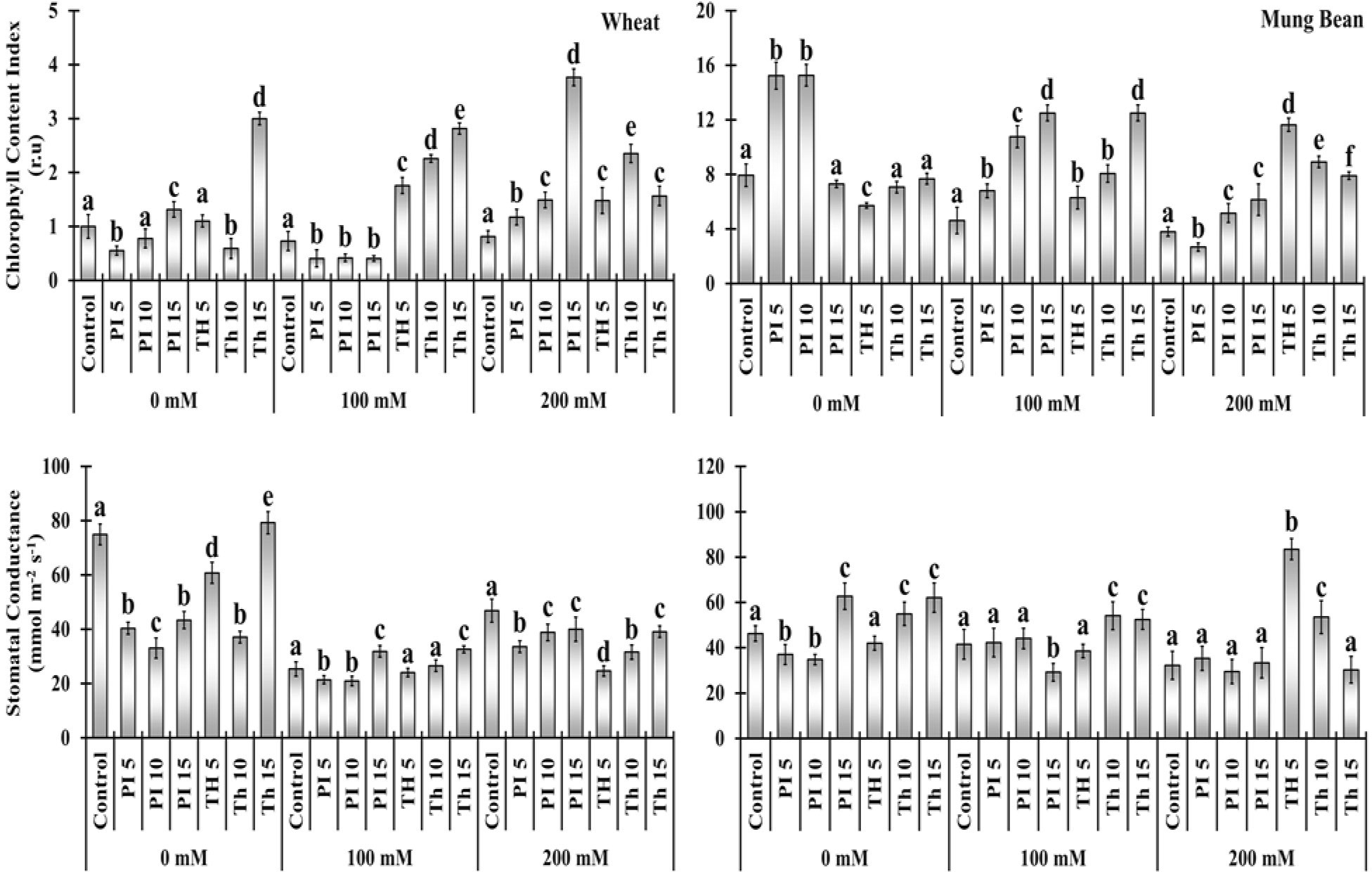
Figure 3 Effects of bio-priming on chlorophyll content index (CCI) and stomatal conductance (gs) of wheat and mung bean plants grown under saline environment. The symbols on the horizontal axis represents: Control: Seeds without priming, Pl = Seed priming with Paecilomyces lilacinus, Th= Seed priming with Trichoderma hamatum 5, 10, 15 = duration of bio-priming in minute, 0, 100 &200 mM NaCl concentration. On bars, vertical lines represent ± Mean Standard Error (S.E) and similar alphabets represents non-significant difference between the means of treatment at p<0.05.
Two types of the consequential stimulated regime by priming agents in wheat and mung bean plants regarding stomatal conductance were observed under extreme salinity (200 mM). Stomatal conductance was significantly decreased in wheat plants over the control when primed with T. hamatum, (-47, -32, and -16%) and with P. lilacinus (-28, -17, and -14%). In contrast, in mung bean plants, both priming agents substantially increased the stomatal conductance over the control (9, -8, 3, 159, 65 and -6%) with some exceptions under 200 mM salt stress respectively (Figure 3, Supplementary Table 2).
Oxidative damage markers
Elevated level of H2O2 and MDA in un-primed plants indicates that salt stress relatively increased the oxidative stress. Bio-priming alleviates the stress in wheat and mung bean plants as the oxidative damage was relatively lower than in control plants. Under 100 mM salt stress, H2O2 was relatively lower in wheat plants primed with T. hamatum (-15, 23, and -22%) and P. lilacinus (-52, -21, and -12%) with some exceptions. (Figure 4, Supplementary Table 2). Moreover, the MDA content among the primed plants was considerably lower in both wheat and mung bean plants in comparison to the control plants. It was evident from the data that MDA content was considerably decreased with the priming of T. hamatum (-47, -39, and 58%) than with P. lilacinus (-29, -32, and 4.98%) in wheat plants under high salinity (200 mM).
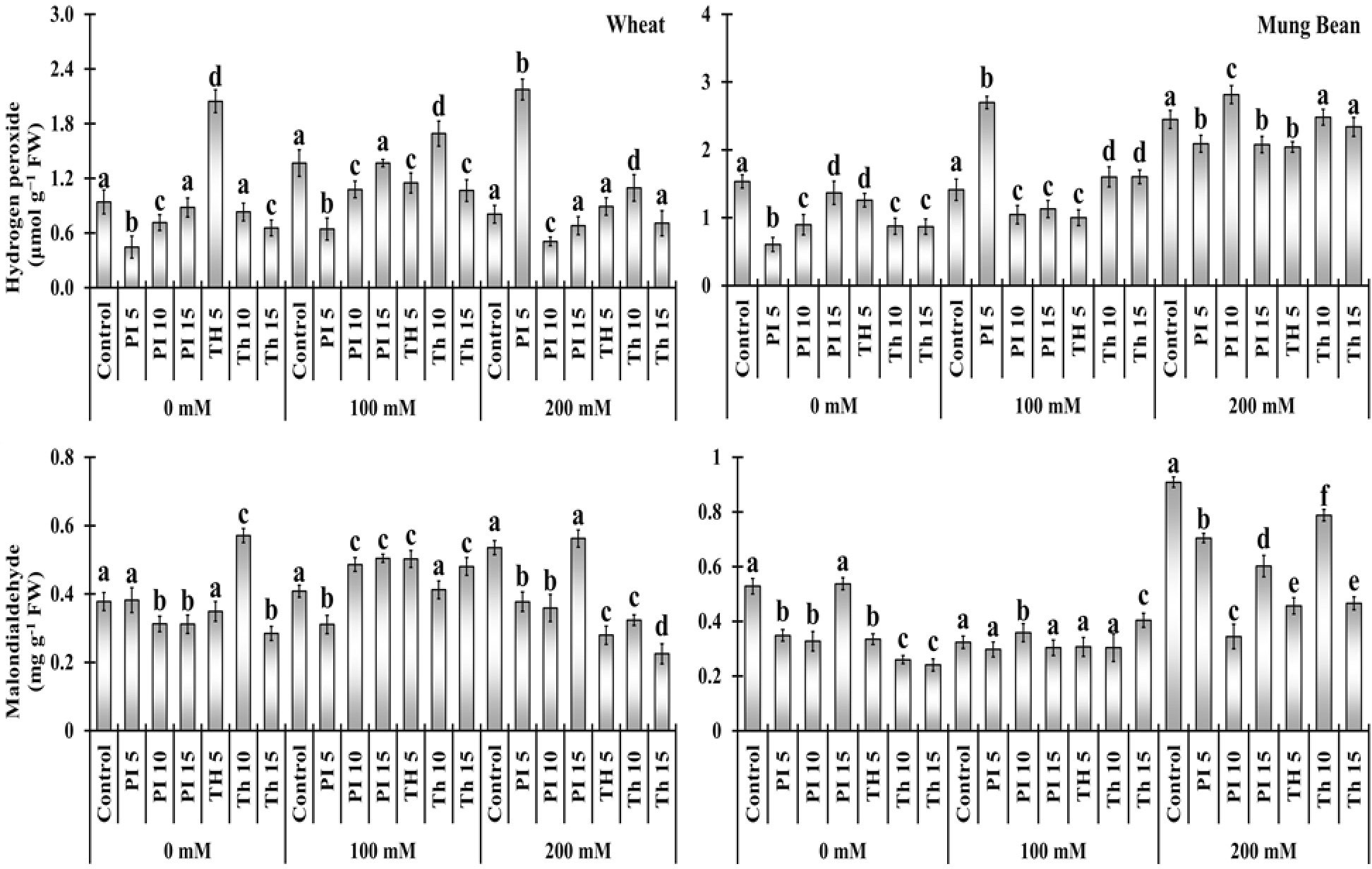
Figure 4 Effects of bio-priming with fungal endophytes on hydrogen peroxide (H2O2) and Malondialdehyde (MDA) contents of wheat and mung bean grown under saline environment. The symbols on the horizontal axis represents: Control: Seeds without priming, Pl = Seed priming with Paecilomyces lilacinus, Th = Seed priming with Trichoderma hamatum 5, 10, 15 = duration of bio-priming in minute, 0, 100 & 200 mM NaCl concentration. On bars, vertical lines represent ± Mean Standard Error (S.E) and similar alphabets represents non-significant difference between the means of treatment at p<0.05.
Photochemical attributes
Salt stress results in a significant decrease in the performance index (PI) and an increase in the dissipation per reaction center (DIO/RC) in wheat and mung bean plants, which was later overcome by bio-priming. Results showed that under 200 mM salt stress, the highest PI was observed in mung bean plants primed with T. hamatum (94%) followed by P. lilacinus (73%) over the control (unprimed plants). Likewise, a similar trend was observed regarding the maximum quantum yield of PS II (FV/FM) in mung bean plants (32 and 26%) in comparison to the un-primed stress plants. In wheat plants, priming of P. lilacinus caused the highest PI and FV/FM (455 and 18%), followed by T. hamatum (357 and 14%) under 200 mM salt stress. However, one way to assess the plant’s performance is to observe the release of absorbed energy, which indicates the performance of the plant under stress conditions. In the present study, we found that dissipation per reaction center (DIO/RC) was significantly decreased due to the priming in both wheat (-31, -42, and -35%) and mung bean (-39, -42, and -46%) under the extreme salinity level (200 mM) (Figure 5, Supplementary Table 2).
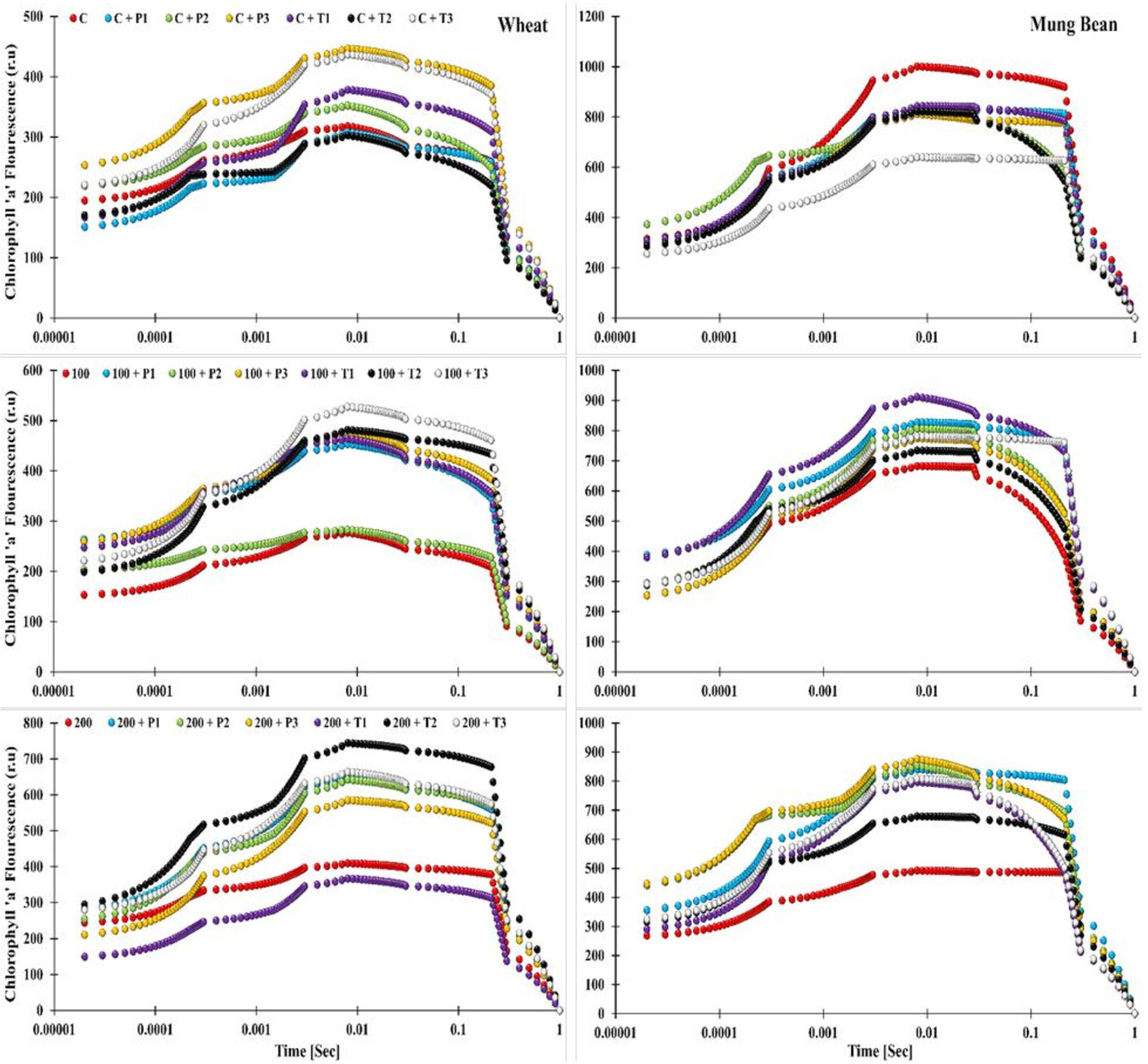
Figure 5 Effects of bio-priming with fungal endophytes on OJIP transient curve of wheat and mung bean grown under saline environment. The symbols on the horizontal axis represents: Control: Seeds without priming, Pl = Seed priming with Paecilomyces lilacinus, Th= Seed priming with Trichoderma hamatum 5, 10, 15 = duration of bio-priming in minute, 0, 100 & 200 mM NaCl concentration.
The OJIP induction curve analysis showed the effect of salt stress as the increase in salinity level (from 0, 100, and 200 mM) caused the decline in the fluorescence intensity (OJIP curve) of the un-primed wheat plants. Highest peaks of the induction transients were observed among the bio-primed plants under both non-stress and stress conditions (T. hamatum and P. lilacinus), while the lowest curve was displayed by the unprimed 200 mM stress plants. However, one striking pattern was observed among the OJIP curve of plants primed with T. hamatum (10 min priming duration) in wheat and mung bean plants. In wheat plants under control (unstressed) conditions, the aforementioned treated plants showed the lowest induction curve, which was moderately increased under 100 mM salt stress and led to the highest peak of all under 200 mM salt stress. In contrast, a complete revert pattern was observed in mung bean plants. T. hamatum (10 mins) primed plants had the highest induction curve values in the control environment, which then decreased to moderate values and then further decreased to a lower curve in the high salinity (200 mM) environment (Figure 6). Moreover, in mung bean plants, the lowest curves were still attributed to the un-primed plants, showing the stress retardation in the photosynthetic machinery of the mung bean plants. The highest curves were exhibited by the plants primed with P. lilacinus under 200 mM stress.
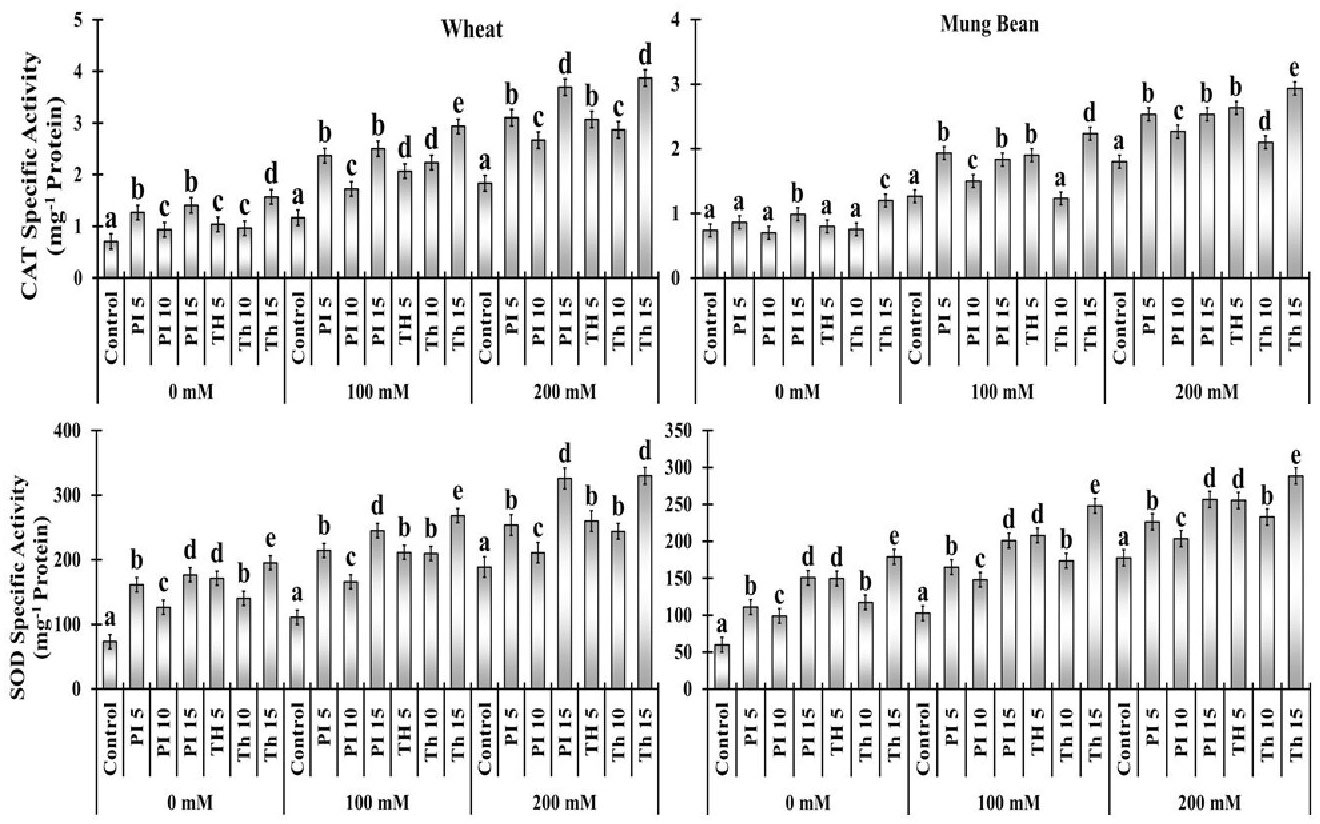
Figure 6 Effects of bio-priming with fungal endophytes on Catalase Specific Activity (CAT) and Superoxide Dismutase Specific Activity (SOD) contents of wheat and mung bean grown under saline environment. The symbols on the horizontal axis represents: Control: Seeds without priming, Pl = Seed priming with Paecilomyces lilacinus, Th= Seed priming with Trichoderma hamatum 5, 10, 15 = duration of bio-priming in minute, 0, 100 & 200 mM NaCl concentration. On bars, vertical lines represent ± Mean Standard Error (S.E) and similar alphabets represents non-significant difference between the means of treatment at p<0.05.
Antioxidant enzymes
Antioxidant enzymes including super oxide dismutase (SOD) and catalase (CAT) activities were measured at different NaCl concentrations with and without endophytes i.e. P. lilacinus and T. hamatum application. In comparison to the control condition, SOD and CAT activities were stimulated by the degree of salinity stress at 100 mM (44 to 141%) and 200 mM (27 to 110%) in both varieties. However, among the two varieties, the increment of SOD and CAT in wheat was greater in comparison to mung beans. Moreover, among the priming treatments, T. haamatum (15 min) prompted the highest SOD (141, 151, 74 and 110%) and CAT (141, 71, 62 and 62%) activity under increasing salt stress over the control, in which the least antioxidant activity was observed. Besides, among different treatments of P. lilacinus the highest increment in SOD (44 to 72%) and CAT (40 to 101%) activities was attributed to the 15 min of priming in both varieties. (Figure 7).
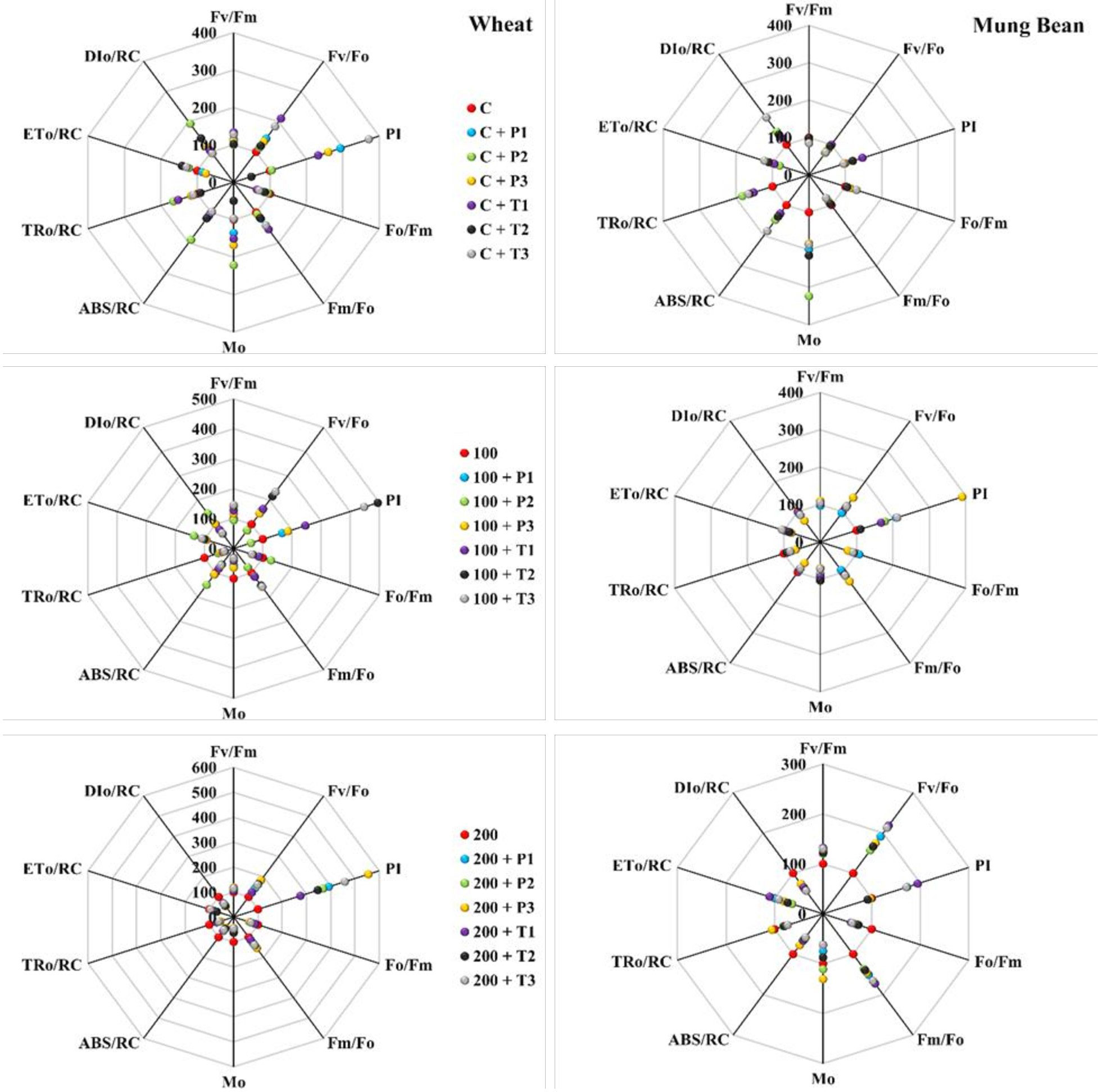
Figure 7 Effects of bio-priming on maximum quantum yield of PSII (FV/FM), activity of water splitting complex on donor site of PSII (FV/FO), performance index (PI), quantum yield of energy dissipation (FO/FM), electron transport rate through PSII (FM/FO), approximated initial slope of fluorescence transient (MO), absorption per reaction centre (ABS/RC), trapping per reaction centre (TRO/RC), electron transport per reaction centre (ETO/RC) and dissipation per reaction centre (DIO/RC) of wheat and Mung bean grown under saline environment. The values of the parameters are expressed as percentage increase or decrease over the control (considered as 100). The symbols on the horizontal axis represents: Control: Seeds without priming, Pl = Seed priming with Paecilomyces lilacinus, Th= Seed priming with Trichoderma hamatum 5, 10, 15 = duration of bio-priming in minute, 0, 100 & 200 mM NaCl concentration. On bars, vertical lines represent ± Mean Standard Error (S.E) and similar alphabets represents non-significant difference between the means of treatment at p<0.05.
Discussion
Due to the changing climate and the increasing assault of abiotic stress, agricultural productivity is heavily curtailed. In the present study two sodium-tolerant biological priming agents, namely T. hamatum and P. lilacinus, with three priming durations (5, 10, and 15 min) were used. Later seeds were allowed to germinate and grow in a salt-stress environment. It was observed that the root and shoot length of both wheat and mung bean plants declined with the elevating salt stress. It is evident from the literature that salt stress inhibited plant growth in a sub-optimal environment (Dey et al., 2004; Azooz et al., 2013; Fizza et al., 2021; Ansari et al., 2022). The decrease in plant growth is attributed to nutrient imbalance, osmotic, and ionic stress (Iqbal and Ashraf, 2013; Rasool et al., 2013; Alqarawi et al., 2014). In the present study, it was observed that the priming with T. hamatum and P. lilacinus increased the root and shoot length of both wheat and mung bean plants in a sub-optimal environment (Figure 2). The highest and most significant amelioration was observed in mung bean plants by virtue of Trichoderma priming. Our findings are in accordance with those of Mastouri et al. (2010) and Rawat et al. (2013), who found that Trichoderma isolates mitigate the negative effects of salt stress in several plants. It was reported that Trichoderma is symbiotically associated with plants and thus enhances plant growth due to hormonal modulation or molecules closely related to GA3 (Iqbal and Ashraf, 2013; Rawat et al., 2013). Thus, Trichoderma association also elongates roots, which aids plants in absorbing nutrients and water from the soil and improves their ability to withstand salt stress (Arora et al., 1992). Likewise, some Paecilomyces spp. has also enhanced plant growth via growth-regulating metabolites like IAA and GA that could work to ameliorate the stress (Bashri and Prasad, 2016; Liu et al., 2019).
Our results, with respect to the decrease in chlorophyll content index (CCI) under salt stress are supported by the findings of Ahmad et al. (2016) for Cicer arietinum, and Alqarawi et al. (2014) for Ephedra alata. The decrease in pigment content is attributed to several factors, including the detrimental effects of salt stress on chloroplast (Zörb et al., 2009), increased activity of chlorophyllase and the consequent reduction in chlorophyll synthesis (Sultana et al., 1999), and instability of the pigment protein complex (Levitt, 1980). The outcomes also demonstrated the potential of T. hamatum and P. lilacinus in curtailing the detrimental effects of NaCl on the CCI and induced a significant rise in chlorophyll content in both salt-treated plants and control plants (Figure 1). P. lilacinus has also been reported to increase the chlorophyll content in carrot plants (Nesha and Siddiqui, 2017). Moreover, Trichoderma spp. has also been linked to improvements in the pigment system and the reduction of harmful effects of NaCl, according to Rawat et al. (2011) and Zhang et al. (2013). Compared to control, plants that are primed with T. hamatum showed improvement in photosynthetic pigments could be attributed by the synthesis of phytohormones such auxin, gibberellins, and cytokinins (Martínez-Medina et al., 2014; Resende et al., 2014).
Salt stress reduced the stomatal conductance of wheat and mung bean plants which is one of the most common responses of plants to prevent excessive water loss and controls the passage of carbon and water between plants and the atmosphere (Brodribb and McAdam, 2011). However, the priming of T. hamatum significantly increased the stomatal conductance over control (un-primed) under extreme salt stress (Figure 1). While in wheat plants, stomatal closure was observed to reduce transpiration and conserve water during salt stress. This closure is regulated through the ABA level as well as extensive signal transduction of guard cells induced by T. hamatum (Efetova et al., 2007; Joshi-Saha et al., 2011). Therefore, two different behavior of T. hamatum priming was observed under high salt stress. In wheat plants, it fosters higher stomatal conductance which could be a strategy to fix more CO2 due to a fast growth strategy before the onset of salt stress consequences compared to mung bean plants.
In salt stress, H2O2 can serve both as a measure of toxicity or that damaged plant cells permanently or it may be a secondary messenger that controls the plant’s antioxidant defense (Gechev et al., 2006). In the current investigation, we discovered that salt stress led to a considerable rise in H2O2 levels. However, in primed wheat plants, the level of H2O2 was significantly lower than in mung bean plants. Moreover, the more decrease in H2O2 level was observed among the plants primed with T. hamatum, therefore, we proposed that priming of T. hamatum promoted lesser oxidative or cellular damage caused by salt stress which is in accordance with the finding of Güler et al. (2001). Likewise, the other damage marker, MDA content was also lower among the wheat plants over the mung beans, hence, the priming was more effective among the wheat plants. As suggested by earlier studies, salt stress may have an impact on altering the composition of membrane lipids since it caused lipid peroxidation (Samadi et al., 2019). The decrease in MDA content suggested that T. hamatum prevented the plant from oxidative damage in comparison to unprimed plants. These findings strongly concur with those of Zhang et al. (2013) who discovered lower levels of lipid peroxidation in cucumber plants under salt stress that had been treated with T. harzianum.
Salt stress adversely affects the photosynthetic apparatus of the plants which can be observed through chlorophyll a fluorescence parameter. Chl fluorescence is frequently employed as a measure of photosystem efficiency because it offers important information about the quantum efficiency of photochemistry and heat dissipation (Lichtenthaler and Burkart, 1999). Quantum yield (FV/FM) and PS II functionality gradually decreased with the increase in exposure time and salt concertation, which negatively affected the membrane stability. This suggests that the PS II reaction center deteriorated under higher stress levels (Lu and Zhang, 2000). However, T. hamatum priming significantly enhanced the FV/FM and PS II efficiency of stressed plants over control and P. lilacinus priming. These outcomes are indicative of T. hamatum efficacy to enhance salt tolerance which is linked with the improved PS II functionality in stressed plants. The increase in energy loss (DIO/RC) among the control plants exhibited stress damage at the PS II level which was quite higher among the control plants while bio-primed plants had considerably very low dissipation hence, lower damage at PS II level.
According to the findings of Ran et al. (2021), the OJIP curve of the present work showed a decline in I and P values with elevated salt stress. However, the increase in I and P steps in T. hamatum and P. lilacinus primed plants showed the availability of more active reaction centers (RC) PS II under salt stress in comparison to control (unprimed plants) (Kalaji et al., 2011). This indicated that bio-primed plants were more tolerant to salt stress as their absorbed energy was more efficiently transferred to reaction centers for photochemistry (Tsimilli-Michael and Strasser, 2008; Stirbet and Govindjee, 2011). The decrease in I and P phase under salt stress control (unprimed) plants was due to a bottleneck in electron transfer at the electron acceptor side of the PSI, the increase in cyclic electron flow (CEF) around the PS I is revealed by the decrease in I-P phase (Kono et al., 2014; Hamdani et al., 2015). This has been alleviated via T. hamatum priming that mitigate the smooth electron flow between PS II and PS I which resulted in high photosynthetic yield of the stressed and unstressed plants (Figure 6).
According to the leaf energy flux model (Figure 8) the highest absorption per reaction center (ABs/RC) and dissipation per reaction center (DIO/RC) were observed among the un-primed plants (wheat and mung bean) which was due to more inactive reaction centers (RC) to active reaction center ratio. Hence, this explains that the controlled plants were able to absorb more photons, but the trapped energy was not used to reduce the plastoquinone pool and absorbed photon was rather dissipated in the form of energy or heat. However, bio-priming enhanced the active to inactive RC ratio among the wheat and mung bean plants which helped to increase the rate of QA reduction by trapped exciton (TRO/RC) under high salt stress (200 mM). This increment led to the enhanced electron transport (ETO/RC) which reflected the increased activity of active RC to reoxidize the reduced QA (Grieco et al., 2015). This combined increased in trapping and transport of exciton displayed the stress tolerance induced by bio-priming agent which reflected in the enhanced photosynthetic yield (PI) and least energy dissipation (DIO/RC) of the primed plants.
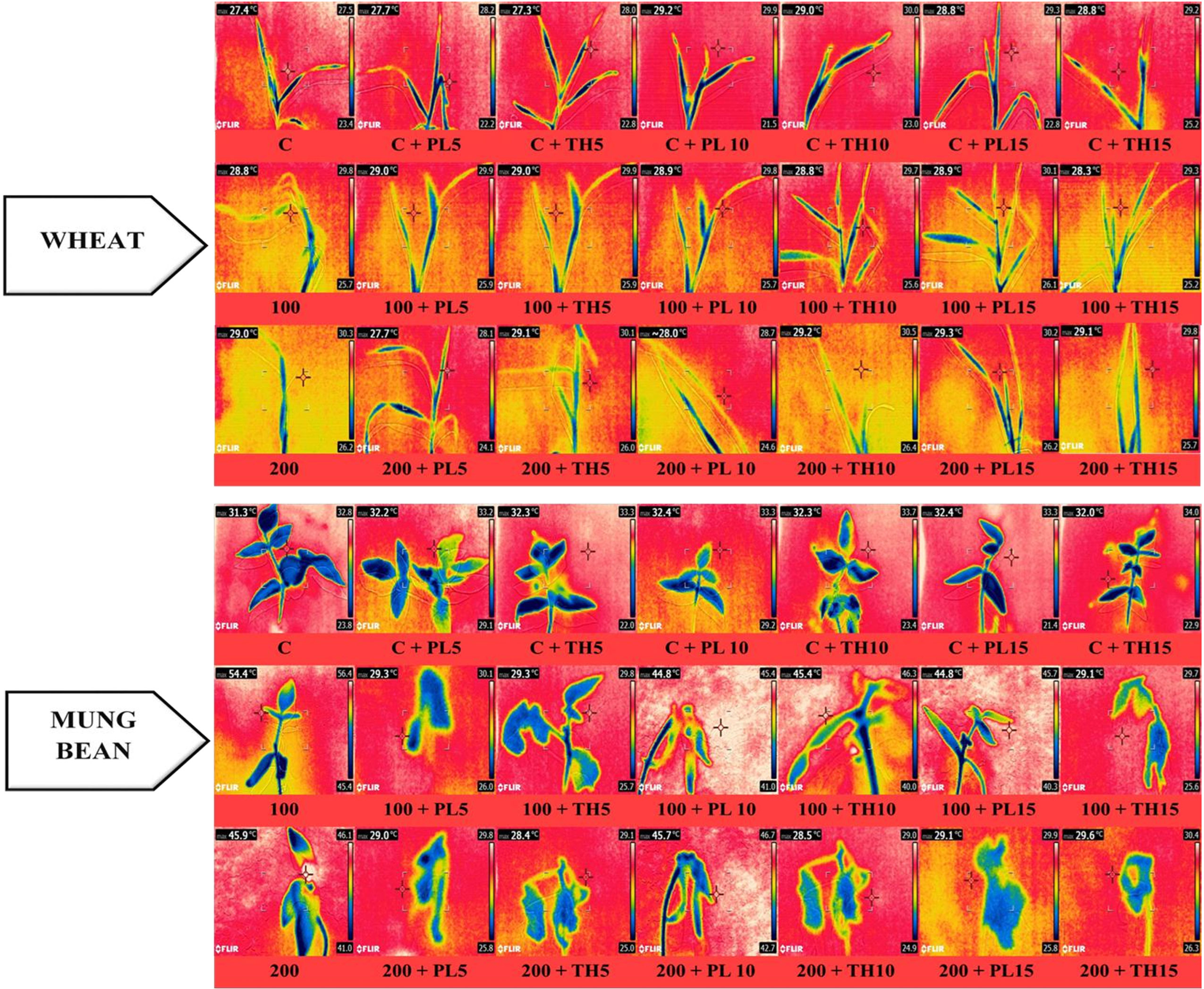
Figure 8 Effects of seed priming with fungal endophytes on infra-red thermal images of wheat and mung bean grown under saline environment. The symbols on the horizontal axis represents: C = Seed without priming, PL5= Seed priming with Paecilomyces lilacinus for 5 mins, PL 10= Seed priming with Paecilomyces lilacinus for 10 mins, PL 15= Seed priming with Paecilomyces lilacinus for 15 mins, TH 5= Seed priming with Trichoderma hamatum for 5 mins, TH 10= Seed priming with Trichoderma hamatum for 10 mins, TH 15= Seed priming with Trichoderma hamatum for 15 mins. 0 (C), 100 and 200 mM represents different salinity (NaCl) levels.
The infra-red thermographic images also evidently supported the results. A significant color change was observed among the leaves of primed and un-primed plants indicating a rise in leaf temperature of the control plants under high salt stress (Figure 9). This rise in temerature reflects the decline in water contents of the leaves. It was evident from the data that bio-primed plants demosntrate lesser increase in leave temperature corresponding with higher water content. Moreover, under the water stress, leaf temperature somewhat mimicked the gas exchange rates and grain output, perhaps due to other changes brought on by this stress factor in plants, like impairments in the rates of photosynthesis and partitioning of energy in plant leaves and canopy structures (resulting in variations in the absorption and/or dissipation of energy) (Casari et al., 2019). Therefore, the results were coherent that the bio-primed plants were more tolerant to varying levels of salt stress (0, 100, and 200 mM) in comparison to the control plants.
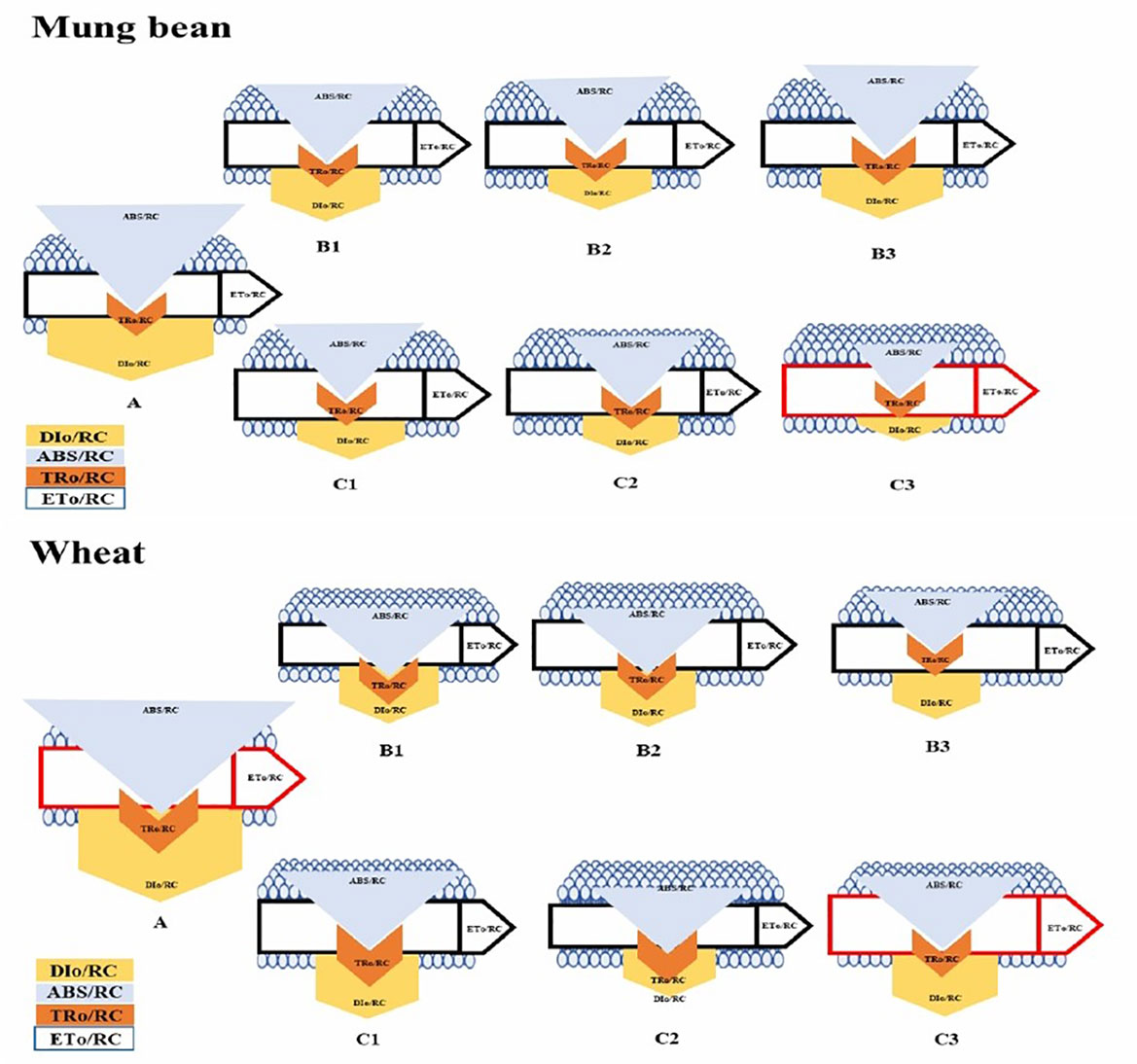
Figure 9 Membrane pipeline model showing the proportion of specific energy fluxes in treated plants. In the membrane, ABS/RC, TRO/RC, ETO/RC, and DIO/RC indicate absorption, maximum trapped exciting flux per active PSII, electron transport, and dissipation flux, respectively. The value of each parameter can be seen in relative changes in the width of each arrow (see the color legend). The diagram exhibits the variation of ABS/RC, TRO/RC, ETO/RC, and DIO/RC, for seven treatments, namely, A=200mM, B1=200mM and Paecilomyces lilacinus strain with 5 minutes time interval, B2=200mM and Paecilomyces lilacinus strain with 10 minutes time interval, B3=200mM and Paecilomyces lilacinus strain with 15 minutes time interval, C1=200mM and Trichoderma harzianum strain with 5 minutes time interval, C2=200mM and Trichoderma harzianum strain with 10 minutes time interval, and C3=200mM and Trichoderma harzianum strain with 15 minutes time interval. The model displays fluxes in different shapes; the size of each shape was developed by the different values of four fluxes in each treatment.
Antioxidant activities are important physiological aspects playing a key role in coping with salt stress (Guo et al., 2018). Abiotic stress causes an increase in ROS production that must be controlled in a homeostatic pool, yet excessive levels of ROS can produce oxidative stress, which can damage plant physiology and cause plant death by causing denaturation of protein structure, lipid peroxidation, and nucleotide disruption (Demidchik, 2015). In this context, an increase in antioxidant activity protects cells against environmental challenges like salinity and drought. P. lilacinus & specifically T. hamatum treated plants showed a remarkable increase in antioxidant enzyme activities like SOD and CAT under high salt stress (200 mM). which significantly reduce the production of ROS like H2O2 that is potent enough to induce lipid peroxidation in cell membrane. Hence, increasing antioxidant activities ultimately brings down the level of MDA in treated plants as compared to control by scavenging ROS (Figure 7).
It is concluded that bio-priming with endophytes produces resistant in crop plants to salt stress through modulation in physiological and photosystem II functionality which was further supported by the infrared thermographic images of the stress and control plants. Endophytes not only sustain better quantum absorption and energy flow in plants but also contribute to sustaining photosystem II performance and lower down the stress markers production and energy loss in a sub-optimal environment. Further our current findings suggest that the use of bio-priming with salt tolerant and bio-stimulating natural colonizers specifically with T. hamatum could be a suitable approach in mitigating salt stress in wheat and mung bean plants.
Data availability statement
The original contributions presented in the study are included in the article/Supplementary Material. Further inquiries can be directed to the corresponding authors.
Author contributions
All authors contributed to the study’s conception and design. Material preparation, search, and collection of relevant articles and reviews were performed by KI, ZS, JC, XW, YR, HA and DW thoroughly checked the first draft and decisively improved the manuscript. All authors contributed to the article and agreed the submitted version. All authors contributed to the article and approved the submitted version.
Conflict of interest
The authors declare that the research was conducted in the absence of any commercial or financial relationships that could be construed as a potential conflict of interest.
Publisher’s note
All claims expressed in this article are solely those of the authors and do not necessarily represent those of their affiliated organizations, or those of the publisher, the editors and the reviewers. Any product that may be evaluated in this article, or claim that may be made by its manufacturer, is not guaranteed or endorsed by the publisher.
Supplementary material
The Supplementary Material for this article can be found online at: https://www.frontiersin.org/articles/10.3389/fpls.2023.1082480/full#supplementary-material
References
Afzal, S. A. I. M. A., Tariq, S., Sultana, V., Ara, J., Ehteshamul-Haque, S. (2013). Managing the root diseases of okra with endo-root plant growth promoting Pseudomonas and Trichoderma viride associated with healthy okra roots. Pak. J. Bot. 45, 1455–1460.
Ahmad, P., Abdel Latef, A. A., AbdAllah, E. F., Hashem, A., Sarwat, M., Anjum, N. A., et al. (2016). Calcium and potassium supplementation enhanced growth, osmolyte secondary metabolite production, and enzymatic antioxidant machinery in cadmium-exposed chickpea (Cicer arietinum l.). Front. Plant Sci. 7, 513. doi: 10.3389/fpls.2016.00513
Alqarawi, A. A., Abd Allah, E. F., Hashem, A. (2014). Alleviation of salt-induced adverse impact via mycorrhizal fungi in ephedra aphylla forssk. J. Plant Interact. 9, 802–810. doi: 10.1080/17429145.2014.949886
Ansari, H. H., Siddiqui, A., Wajid, D., Tabassum, S., Umar, M., Siddiqui, Z. S. (2022). Profiling of energy compartmentalization in photosystem II (PSII), light harvesting complexes and specific energy fluxes of primed maize cultivar (P1429) under salt stress environment. Plant Physiol. Biochem. 170, 296–306. doi: 10.1016/j.plaphy.2021.12.015
Arora, R., Wisniewski, M. E., Scorza, R. (1992). Cold acclimation in genetically related (sibling) deciduous and evergreen peach (Prunus persica [L.] batsch) i. seasonal changes in cold hardiness and polypeptides of bark and xylem tissues. Plant Physiol. 99, 1562–1568. doi: 10.1104/pp.99.4.1562
Augé, R. M., Toler, H. D., Saxton, A. M. (2014). Arbuscular mycorrhizal symbiosis and osmotic adjustment in response to NaCl stress: a meta-analysis. Front. Plant Sci. 5, 562. doi: 10.3389/fpls.2014.00562
Azooz, M. M., Alzahrani, A. M., Youssef, M. M. (2013). The potential role of seed priming with ascorbic acid and nicotinamide and their interactions to enhance salt tolerance in broad bean ('Vicia faba'L.). Aus. J. Crop Sci. 7, 2091–2100. doi: 10.3316/informit.801220319651814
Barrs, H. D., Weatherley, P. E. (1962). A re-examination of the relative turgidity technique for estimating water deficits in leaves. Aus. J. Biol. Sci. 15, 413–428. doi: 10.1071/BI9620413
Bashri, G., Prasad, S. M. (2016). Exogenous IAA differentially affects growth, oxidative stress and antioxidants system in cd stressed trigonella foenum-graecum l. seedlings: Toxicity alleviation by up-regulation of ascorbate-glutathione cycle. Ecotoxic. Environ. Saf. 132, 329–338. doi: 10.1016/j.ecoenv.2016.06.015
Beyer, J. W.F., Fridovich, I. (1987). Assaying for superoxide dismutase activity: some large consequences of minor changes in conditions. Anal. Biochem. 161, 559–566. doi: 10.1016/0003-2697(87)90489-1
Bradford, M. M. (1976). A rapid and sensitive method for the quantitation of microgram quantities of protein utilizing the principle of protein-dye binding. Anal. Biochem. 72, 248–254. doi: 10.1016/0003-2697(76)90527-3
Brodribb, T. J., McAdam, S. A. (2011). Passive origins of stomatal control in vascular plants. Sci 331, 582–585. doi: 10.1126/science.1197985
Casari, R. A., Paiva, D. S., Silva, V. N., Ferreira, T. M., Souza Junior, M. T., Oliveira, N. G., et al. (2019). Using thermography to confirm genotypic variation for drought response in maize. Int. J. Mol. Sci. 20, 2273. doi: 10.3390/ijms20092273
Chinnusamy, V., Jagendorf, A., Zhu, J. K. (2005). Understanding and improving salt tolerance in plants. Crop Sci. 45, 437–448. doi: 10.2135/cropsci2005.0437
Demidchik, V. (2015). Mechanisms of oxidative stress in plants: from classical chemistry to cell biology. Enviro. Exp. Bot. 109, 212–228. doi: 10.1016/j.envexpbot.2014.06.021
Dey, R. K. K. P., Pal, K. K., Bhatt, D. M., Chauhan, S. M. (2004). Growth promotion and yield enhancement of peanut (Arachis hypogaea l.) by application of plant growth-promoting rhizobacteria. Microbiol. Res. 159, 371–394. doi: 10.1016/j.micres.2004.08.004
Dhindsa, R. S., Plumb-Dhindsa, P. A. M. E. L. A., Thorpe, T. A. (1981). Leaf senescence: correlated with increased levels of membrane permeability and lipid peroxidation, and decreased levels of superoxide dismutase and catalase. J. Exp. Bot. 32, 93–101. doi: 10.1093/jxb/32.1.93
Dimkpa, C., Weinand, T., Asch, F. (2009). Plant–rhizobacteria interactions alleviate abiotic stress conditions. Plant Cell Enviro. 32, 1682–1694. doi: 10.1111/j.1365-3040.2009.02028.x
Ebert, A. W., Engels, J. M. (2020). Plant biodiversity and genetic resources matter. Plants 9, 1706. doi: 10.3390/plants9121706
Efetova, M., Zeier, J., Riederer, M., Lee, C. W., Stingl, N., Mueller, M., et al. (2007). A central role of abscisic acid in drought stress protection of agrobacterium-induced tumors on arabidopsis. Plant Physiol. 145, 853–862. doi: 10.1104/pp.107.104851
Estrada, B., Aroca, R., Barea, J. M., Ruiz-Lozano, J. M. (2013). Native arbuscular mycorrhizal fungi isolated from a saline habitat improved maize antioxidant systems and plant tolerance to salinity. Plant Sci. 201, 42–51. doi: 10.1016/j.plantsci.2012.11.009
Fizza, A., Umar, M., Siddiqui, Z. S. (2021). Comparative physiological assessment of some edible oil seed crops under drought stress environment using fluorescence and IR imaging techniques. Pak. J. Bot. 53, 4. doi: 10.30848/PJB2021-4(13)
Gechev, T. S., Van Breusegem, F., Stone, J. M., Denev, I., Laloi, C. (2006). Reactive oxygen species as signals that modulate plant stress responses and programmed cell death. Bioessays 28, 1091–1101. doi: 10.1002/bies.20493
Grieco, M., Suorsa, M., Jajoo, A., Tikkanen, M., Aro, E. M. (2015). Light-harvesting II antenna trimers connect energetically the entire photosynthetic machinery–including both photosystems II and i. biochem. et. biophy. acta. (BBA)-Bioenergetics 1847, 607–619. doi: 10.1016/j.bbabio.2015.03.004
Güler, M., Adak, M. S., Ulukan, H. (2001). Determining relationships among yield and some yield components using path coefficient analysis in chickpea (Cicer arietinum l.). Eur. J. Agro 14, 161–166. doi: 10.1016/S1161-0301(00)00086-1
Guo, R., Wang, Z., Huang, Y., Fan, H., Liu, Z. (2018). Biocontrol potential of saline-or alkaline-tolerant Trichoderma asperellum mutants against three pathogenic fungi under saline or alkaline stress conditions. Braz. J. Microbiol. 49, 236–245. doi: 10.1016/j.bjm.2018.02.008
Hamdani, N., Fdil, R., Tourabi, M., Jama, C., Bentiss, F. (2015). Alkaloids extract of Retama monosperma (L.) boiss. seeds used as novel eco-friendly inhibitor for carbon steel corrosion in 1 m HCl solution: Electrochemical and surface studies. App. Surf. Sci. 357, 1294–1305. doi: 10.1016/j.apsusc.2015.09.159
Iqbal, M., Ashraf, M. (2013). Gibberellic acid mediated induction of salt tolerance in wheat plants: Growth, ionic partitioning, photosynthesis, yield and hormonal homeostasis. Environ. Exp. Bot. 86, 76–85. doi: 10.1016/j.envexpbot.2010.06.002
Johnson, R., Puthur, J. T. (2021). Seed priming as a cost effective technique for developing plants with cross tolerance to salinity stress. Plant Physiol. Biochem. 162, 247–257. doi: 10.1016/j.plaphy.2021.02.034
Joshi-Saha, A., Valon, C., Leung, J. (2011). A brand-new START: abscisic acid perception and transduction in the guard cell. Sci. Signal 4, re4–re4. doi: 10.1126/scisignal.2002164
Kalaji, H. M., Bosa, K., Kościelniak, J., Żuk-Gołaszewska, K. (2011). Effects of salt stress on photosystem II efficiency and CO2 assimilation of two Syrian barley landraces. Environ. Exp. Bot. 73, 64–72. doi: 10.1016/j.envexpbot.2010.10.009
Kono, M., Noguchi, K., Terashima, I. (2014). Roles of the cyclic electron flow around PSI (CEF-PSI) and O2-dependent alternative pathways in regulation of the photosynthetic electron flow in short-term fluctuating light in arabidopsis thaliana. Plant Cell Physiol. 55, 990–1004. doi: 10.1093/pcp/pcu033
Kwon, T. R., Siddiqui, Z. S., Harris, P. J. C. (2009). Effect of supplemental ca++ on ion accumulation, transport and plant growth of salt sensitive brassica rapa landraces. J. Plant Nutri. 32, 644–667. doi: 10.1080/01904160802715455
Levitt, J. (1980). Responses of plants to environmental stress, volume 1: Chilling, freezing, and high temperature stresses (Academic Press). Available at: https://www.cabdirect.org/cabdirect/abstract/19802605739.
Lichtenthaler, H. K., Burkart, S. (1999). Photosynthesis and high light stress. Bulg. J. Plant Physiol. 25, 3–16. Available at: http://www.bio21.bas.bg/ipp/gapbfiles/v-25/99_3-4_03-16.pdf.
Liu, S., Hao, H., Lu, X., Zhao, X., Wang, Y., Zhang, Y., et al. (2017). Transcriptome profiling of genes involved in induced systemic salt tolerance conferred by Bacillus amyloliquefaciens FZB42 in Arabidopsis thaliana. Sci. Rep. 7, 10795. doi: 10.1038/s41598-017-11308-8
Liu, H., Lu, C., Jiang, D., Wang, L., Jiang, Y., Tang, S., et al. (2019). Paecilomyces variotii extracts (ZNC) enhance plant immunity and promote plant growth. Plant Soil 441, 383–397. doi: 10.1007/s11104-019-04130-w
Lu, C. M., Zhang, J. H. (2000). Heat-induced multiple effects on PSII in wheat plants. J. Plant Physiol. 156, 259–265. doi: 10.1016/S0176-1617(00)80315-6
Mahmood, U., Hussain, S., Hussain, S., Ali, B., Ashraf, U., Zamir, S., et al. (2021). Morpho-physio-biochemical and molecular responses of maize hybrids to salinity and waterlogging during stress and recovery phase. Plants 10, 1345. doi: 10.3390/plants10071345
Martínez-Medina, A., Mar, D., Alguacil, M., Pascual, J. A., Van Wees, S. (2014). Phytohormone profiles induced by Trichoderma isolates correspond with their biocontrol and plant growth-promoting activity on melon plants. J. Chem. Eco. 40, 804–815. doi: 10.1007/s10886-014-0478-1
Mastouri, F., Björkman, T., Harman, G. E. (2010). Seed treatment with Trichoderma harzianum alleviates biotic, abiotic, and physiological stresses in germinating seeds and seedlings. Phytopath 100, 1213–1221. doi: 10.1094/PHYTO-03-10-0091
Nephali, L., Moodley, V., Piater, L., Steenkamp, P., Buthelezi, N., Dubery, I., et al. (2021). A metabolomic landscape of maize plants treated with a microbial biostimulant under well-watered and drought conditions. Front. Plant Sci. 12, 676632. doi: 10.3389/fpls.2021.676632
Nesha, R., Siddiqui, Z. A. (2017). Effects of Paecilomyces lilacinus and Aspergillus niger alone and in combination on the growth, chlorophyll contents and soft rot disease complex of carrot. Sci. Horti. 218, 258–264. doi: 10.1016/j.scienta.2016.11.027
Patterson, B. D., MacRae, E. A., Ferguson, I. B. (1984). Estimation of hydrogen peroxide in plant extracts using titanium (IV). Anal. Biochem. 139, 487–492. doi: 10.1016/0003-2697(84)90039-3
Porcel, R., Aroca, R., Ruiz-Lozano, J. M. (2012). Salinity stress alleviation using arbscular mycorrhizal fungi. a review. Agro. Sustain. Dev. 32, 181–200. doi: 10.1007/s13593-011-0029-x
Ran, X., Wang, X., Gao, X., Liang, H., Liu, B., Huang, X. (2021). Effects of salt stress on the photosynthetic physiology and mineral ion absorption and distribution in white willow (Salix alba l.). PloS One 16, e0260086. doi: 10.1371/journal.pone.0260086
Rasool, S., Ahmad, A., Siddiqui, T. O., Ahmad, P. (2013). Changes in growth, lipid peroxidation and some key antioxidant enzymes in chickpea genotypes under salt stress. Acta Physiol. Plant 35, 1039–1050. doi: 10.1007/s11738-012-1142-4
Rawat, I., Kumar, R. ,. R., Mutanda, T., Bux, F. (2011). Dual role of microalgae: phycoremediation of domestic wastewater and biomass production for sustainable biofuels production. App. Energy 88, 3411–3424. doi: 10.1016/j.apenergy.2010.11.025
Rawat, I., Kumar, R. R., Mutanda, T., Bux, F. (2013). Biodiesel from microalgae: a critical evaluation from laboratory to large scale production. App. Energy 103, 444–467. doi: 10.1016/j.apenergy.2012.10.004
Resende, J. A., Diniz, C. G., Silva, V. L., Otenio, M. H., Bonnafous, A., Arcuri, P. B., et al. (2014). Dynamics of antibiotic resistance genes and presence of putative pathogens during ambient temperature anaerobic digestion. J. App. Microbiol. 117, 1689–1699. doi: 10.1111/jam.12653
Saeid, A., Prochownik, E., Dobrowolska-Iwanek, J. (2018). Phosphorus solubilization by bacillus species. Molecules 23, 2897. doi: 10.3390/molecules23112897
Samadi, S., Mehramiz, M., Kelesidis, T., Mobarhan, M. G., Sahebkar, A. H., Esmaily, H., et al. (2019). High-density lipoprotein lipid peroxidation as a molecular signature of the risk for developing cardiovascular disease: Results from MASHAD cohort. J. Cell Physiol. 234, 16168–16177. doi: 10.1002/jcp.28276
Sandhya, V., Ali, S. K. Z., Grover, M., Reddy, G., Venkateswarlu, B. (2009). Alleviation of drought stress effects in sunflower seedlings by exo-polysaccharides producing Pseudomonas putida strain P45. Biol. Fertil. Soil 46, 17–26. doi: 10.1007/s00374-009-0401-z
Sarkar, D., Singh, S., Parihar, M., Rakshit, A. (2021). Seed bio-priming with microbial inoculants: A tailored approach towards improved crop performance, nutritional security, and agricultural sustainability for smallholder farmers. Curr. Res. Env. Sus. 3, 100093. doi: 10.1016/j.crsust.2021.100093
Sarma, B. K., Yadav, S. K., Singh, S., Singh, H. B. (2015). Microbial consortium mediated plant defense against phytopathogens: Readdressing for enhancing efficacy. Soil Biol. Biochem. 87, 25–33. doi: 10.1016/j.soilbio.2015.04.001
Shoresh, M., Harman, G. E., Mastouri, F. (2010). Induced systemic resistance and plant responses to fungal biocontrol agents. Ann. Rev. Phytopath. 48, 21–43. doi: 10.1146/annurev-phyto-073009-114450
Singh, D., Rathod, V., Ninganagouda, S., Herimath, J., Kulkarni, P. (2013). Biosynthesis of silver nanoparticle by endophytic fungi pencillium spp. isolated from Curcuma longa (turmeric) and its antibacterial activity against pathogenic gram negative bacteria. J. Pharm. Res. 7, 448–453. doi: 10.1007/s13205-016-0393-y
Strasser, R. J., Tsimilli-Michael, M., Qiang, S., Goltsev, V. (2010). Simultaneous in vivo recording of prompt and delayed fluorescence and 820-nm reflection changes during drying and after rehydration of the resurrection plant Haberlea rhodopensis. Bio.,Chem. Biophy. Acta Bioener. 1797, 1313–1326. doi: 10.1016/j.bbabio.2010.03.008
Stirbet, A., Govindjee (2011). On the relation between the kautsky effect (chlorophyll a fluorescence induction) and photosystem II: basics and applications of the OJIP fluorescence transient. J. Photochem. Photobiol. 104, 236–257. doi: 10.1016/j.jphotobiol.2010.12.010
Sultana, N., Ikeda, T., Itoh, R. (1999). Effect of NaCl salinity on photosynthesis and dry matter accumulation in developing rice grains. Environ. Exp. Bot. 42, 211–220. doi: 10.1016/S0098-8472(99)00035-0
Tsimilli-Michael, M., Strasser, R. J. (2008). “In vivo assessment of stress impact on plant’s vitality: applications in detecting and evaluating the beneficial role of mycorrhization on host plants,” in Mycorrhiza (Berlin, Heidelberg: Springer), 679–703.
Umar, M., Siddiqui, Z. S. (2018). Physiological performance of sunflower genotypes under combined salt and drought stress environment. Acta Bot. Croa. 77, 36–44. doi: 10.2478/botcro-2018-0002
Velikova, V., Yordanov, I., Edreva, A. (2000). Oxidative stress and some antioxidant systems in acid rain-treated bean plants: protective role of exogenous polyamines. Plant Sci. 151, 59–66. doi: 10.1016/S0168-9452(99)00197-1
Verma, H., Kumar, D., Kumar, V., Kumari, M., Singh, S. K., Sharma, V. K., et al. (2021). The potential application of endophytes in management of stress from drought and salinity in crop plants. Microorganisms 9, 1729. doi: 10.3390/microorganisms9081729
Yao, L., Wu, Z., Zheng, Y., Kaleem, I., Li, C. (2010). Growth promotion and protection against salt stress by Pseudomonas putida rs-198 on cotton. Eur. J. Soil Biol. 46, 49–54. doi: 10.1016/j.ejsobi.2009.11.002
Zhang, F., Yuan, J., Yang, X., Cui, Y., Chen, L., Ran, W., et al. (2013). Putative trichoderma harzianum mutant promotes cucumber growth by enhanced production of indole acetic acid and plant colonization. Plant Soil 368, 433–444. doi: 10.1007/s11104-012-1519-6
Keywords: Chlorophyll ‘a’ fluorescence, bacterial priming, wheat, mung bean, ionic stress, response
Citation: Irshad K, Shaheed Siddiqui Z, Chen J, Rao Y, Hamna Ansari H, Wajid D, Nida K and Wei X (2023) Bio-priming with salt tolerant endophytes improved crop tolerance to salt stress via modulating photosystem II and antioxidant activities in a sub-optimal environment. Front. Plant Sci. 14:1082480. doi: 10.3389/fpls.2023.1082480
Received: 28 October 2022; Accepted: 20 February 2023;
Published: 09 March 2023.
Edited by:
Mamoona Rauf, Abdul Wali Khan University Mardan, PakistanReviewed by:
Amitava Rakshit, Banaras Hindu University, IndiaZahoor Ahmad Sajid, University of the Punjab, Pakistan
Copyright © 2023 Irshad, Shaheed Siddiqui, Chen, Rao, Hamna Ansari, Wajid, Nida and Wei. This is an open-access article distributed under the terms of the Creative Commons Attribution License (CC BY). The use, distribution or reproduction in other forums is permitted, provided the original author(s) and the copyright owner(s) are credited and that the original publication in this journal is cited, in accordance with accepted academic practice. No use, distribution or reproduction is permitted which does not comply with these terms.
*Correspondence: Zamin Shaheed Siddiqui, emFtaW5zc0B1b2suZWR1LnBr; Xiangying Wei, eGlhbmd5aW5nd2VpQG1qdS5lZHUuY24=