- Department of Plant Biology, Michigan State University, East Lansing, MI, United States
Acyl editing refers to a deacylation and reacylation cycle on a lipid, which allows for fatty acid desaturation and modification prior to being removed and incorporated into other pools. Acyl editing is an important determinant of glycerolipid synthesis and has been well-characterized in land plants, thus this review begins with an overview of acyl editing in plants. Much less is known about acyl editing in algae, including the extent to which acyl editing impacts lipid synthesis and on which lipid substrate(s) it occurs. This review compares what is known about acyl editing on its major hub phosphatidylcholine (PC) in land plants with the evidence for acyl editing of betaine lipids such as diacylglyceryltrimethylhomoserine (DGTS), the structural analog that replaces PC in several species of microalgae. In land plants, PC is also known to be a major source of fatty acids and diacylglycerol (DAG) for synthesis of the neutral lipid triacylglycerol (TAG). We review the evidence that DGTS contributes substantially to TAG accumulation in algae as a source of fatty acids, but not as a precursor to DAG. We conclude with evidence of acyl editing on other membrane lipid substrates in plants and algae apart from PC or DGTS, and discuss future analyses to elucidate the role of DGTS and other betaine lipids in acyl editing in microalgae.
Introduction
Research in algal lipid metabolism has undergone a resurgence in recent years (Merchant et al., 2012; Du and Benning, 2016; Goncalves et al., 2016), particularly due to interest in the neutral lipid triacylglycerol (TAG), which is a source of nutritionally valuable fatty acids and a key feedstock for biodiesel fuel production. Both the quantity and composition of TAG are important and are governed by fluxes through intermediate glycerolipids pools such as membrane lipids (Goncalves et al., 2013; Légeret et al., 2016; Young and Shachar-Hill, 2021). The process of acyl editing, which is used to mean the addition and removal of an acyl group on a lipid with the potential for tailoring and modification and subsequent incorporation into another lipid pool, plays a central role in fluxes between membrane lipids and TAG. Much of what is currently known about acyl editing has been determined in land plants, which utilize the extraplastidial lipid phosphatidylcholine (PC) as the main hub of acyl editing and fatty acid modification, and which also contributes to TAG synthesis. However, a range of algae lack PC and rather contain ether-linked betaine lipids (Kato et al., 1996; Künzler and Eichenberger, 1997), thus raising the question: To what extent do betaine lipids replace the functions of PC in algae? A better understanding of acyl editing is crucial to efforts in algal lipid engineering; improving the yield and composition of algal TAG without this knowledge is likely to remain a hit-or-miss affair.
PC serves as the major acyl hub in plants, where it is the predominant lipid outside of the chloroplast in most plant cells. PC plays a central role in several aspects of plant lipid metabolism by acting as the major substrate for extraplastidial acyl modifications, in lipid trafficking between subcellular compartments, and in conducting fluxes toward TAG synthesis (Miquel and Browse, 1992; Bates et al., 2012; Chen et al., 2015). In many microalgal species, betaine lipids are present in inverse proportion to PC levels, replacing it completely in some cases and partially in others. Due to this inverse relationship and the structural similarity of betaine lipids and PC (Figure 1), it has been hypothesized that betaine lipids play analogous roles to PC in algae. The betaine lipid diacylglyceryl-N,N,N-trimethylhomoserine (DGTS) is widely distributed in nonflowering groups such as green algae, lichens, mosses, and ferns and is absent in seed plants and flowering plants (Künzler and Eichenberger, 1997), suggesting that the capacity to synthesize DGTS was lost in the spermatophyte ancestor to seed plants. Some bacteria are capable of synthesizing DGTS in response to phosphate starvation (Geiger et al., 1999), and DGTS is present in some fungi as well (Künzler and Eichenberger, 1997). It is believed that a green algae ancestor was capable of producing DGTS, thus creating a “DGTS branch.” However, some algae in this branch lack DGTS, indicating that some organisms either reduced or lost the capacity for betaine lipid synthesis. The replacement of PC with DGTS may have evolved as a stress acclimation response to low phosphorus or low temperature environments.
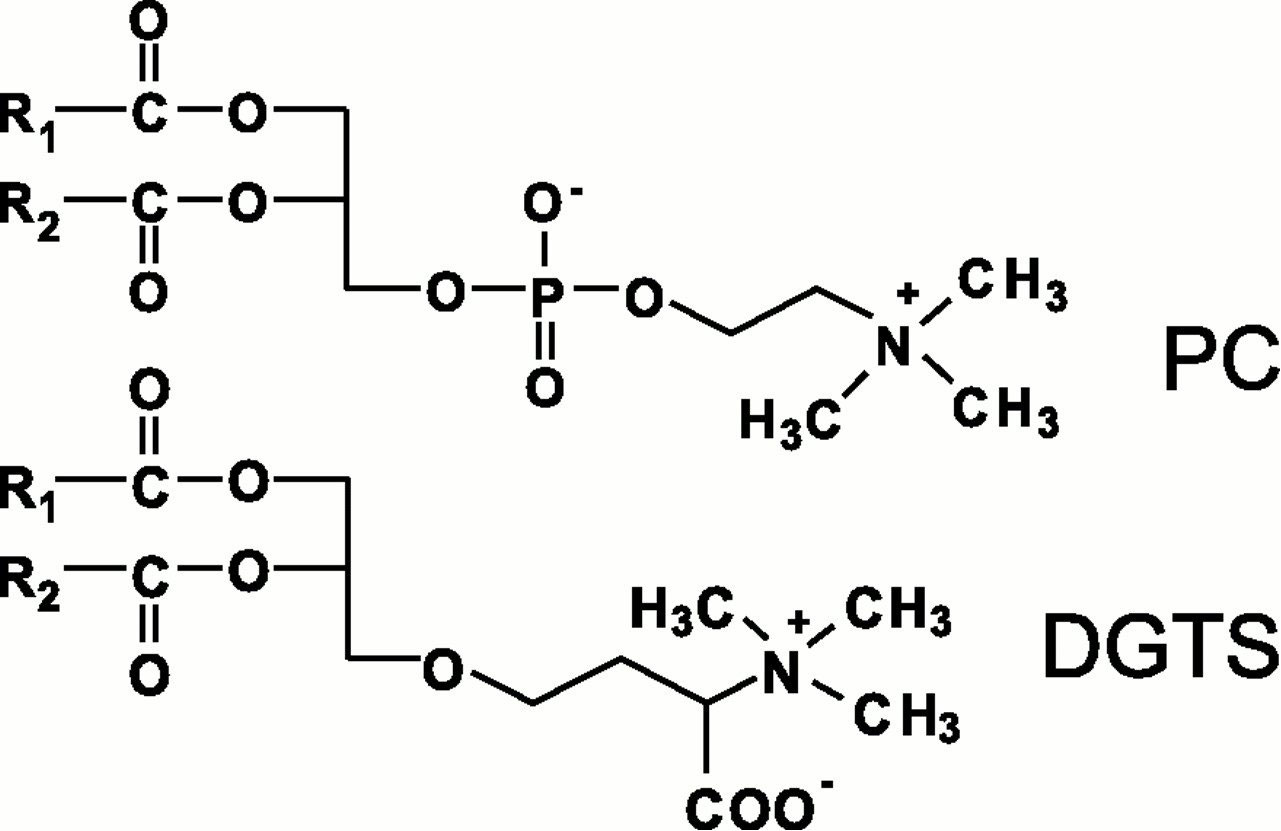
Figure 1 Structures of PC and DGTS. Figure reproduced with permission from Klug and Benning, 2001. Copyright (2001) National Academy of Sciences, U.S.A. DGTS, diacylglyceryl-N,N,N-trimethylhomoserine; PC, phosphatidylcholine.
In this review, we compare the roles of PC in plant lipid metabolism with what is known about the role of betaine lipids, particularly DGTS, in algae. The first sections below describe the metabolic roles of PC in membrane synthesis and oil accumulation in plants. The subsequent sections present evidence in favor and against DGTS replacing the different functions of PC in algae and discuss alternative acyl editing substrates apart from PC or DGTS. The evidence outlined for PC’s role in plants serves as a pointer to methods that have or can be used in the future to address these questions in algae. Rational engineering of algal lipid metabolism such as TAG accumulation and composition will require knowledge of their acyl hubs, as evidenced by prior attempts to engineer the accumulation of particular fatty acids in oilseeds, in which flux through PC represented a major bottleneck (Bates and Browse, 2011).
Overview of acyl editing in plants
The process of acyl editing is an important determinant of eukaryotic membrane lipid synthesis as well as TAG accumulation and has been well-characterized in land plants. Therefore, we begin this review with an overview of acyl editing and related functions in plants. The existence of a deacylation and reacylation cycle on PC, the most abundant membrane lipid in animals and the major extraplastidic one in plants, was originally inferred from 14C-tracer experiments. Supplying rat lung tissue with 14C-acetate and 14C-glycerol resulted in a ratio of radioactivity in fatty acids compared to glycerol that was much higher in phospholipids than in TAG, implying that turnover of fatty acids occurred on PC (Lands, 1958). It was hypothesized that this occurred via the action of a phospholipase generating lysophosphatidylcholine (lyso-PC, PC with one fatty acid removed), which was then reacylated to form PC (Lands, 1960). In microsomes of developing safflower cotyledons, 14C-labeling revealed rapid exchange of fatty acids between diacylglycerol (DAG) and PC (Stobart and Stymne, 1985), and evidence was found that this acyl exchange was catalyzed by the forward and reverse reactions of acyl-CoA:lysophosphatidylcholine acyltransferase (LPCAT) (Stymne and Stobart, 1984). Thus, acyl exchange on PC, termed “the Lands’ cycle,” could proceed via two possible mechanisms: the action of a phospholipase followed by reacylation by LPCAT, or by both forward and reverse reactions catalyzed by LPCAT (Figure 2).
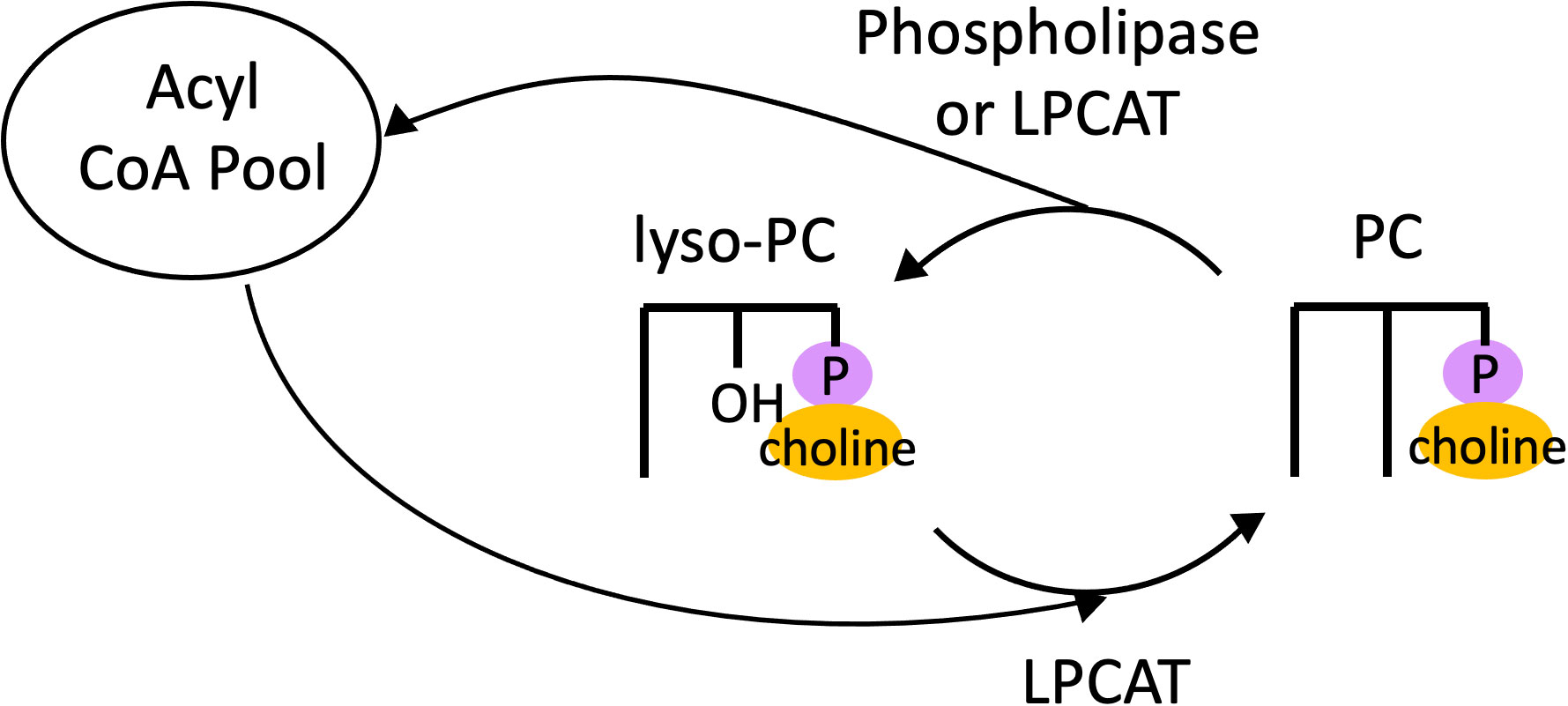
Figure 2 The Lands Cycle. Cycle of deacylation of PC via either a phospholipase or LPCAT to produce lyso-PC, which is then reacylated by LPCAT to regenerate PC. LPCAT, acyl-CoA:lysophosphatidylcholine acyltransferase; PC, phosphatidylcholine.
A study utilizing 14C-acetate and 14C-glycerol labeling in pea leaves found that the majority of newly made fatty acids were rapidly incorporated into PC rather than proceeding via the de novo synthesis pathway (Bates et al., 2007). Here, “de novo synthesis” refers to the Kennedy pathway in which glycerol-3-phosphate (G3P) is acylated to form lyso-phosphatidic acid, which is then acylated to form phosphatidic acid (PA), whose phosphate group is removed to form DAG. PC is then synthesized from DAG via cytidine-5′-diphosphocholine:diacylglycerol cholinephosphotransferase (CPT). This labeling study in pea leaves found that over 90% of 14C-labeled PC molecules contained one 14C-labeled fatty acid and one unlabeled fatty acid, and 62% of the label was found at the sn-2 position and 38% at the sn-1 position (Bates et al., 2007). PC acyl editing was found to be the major route of newly made fatty acid flux in plant leaves (Bates et al., 2007), a process that allows polyunsaturated fatty acids (PUFAs) to be produced on PC and then distributed to other lipids.
Labeling with 14C-acetate and 14C-glycerol during TAG synthesis in developing soybean embryos also revealed the direct incorporation of newly made fatty acids into PC similar to that observed in pea leaves (Bates et al., 2009). In addition, 86% of newly synthesized fatty acids in PC were found at the sn-2 position, suggesting that acyl editing occurs primarily at this location (Bates et al., 2009). It was concluded that the major flux of newly made fatty acids proceeded through PC via acyl editing in this oilseed, highlighting the importance of acyl editing in lipid synthesis in TAG accumulation as well as membrane synthesis.
Phosphatidylcholine serves as the substrate of fatty acid modification and key intermediate in TAG synthesis in plants
In plants, PC is the major site of extraplastidial desaturation to produce PUFAs, as studies have demonstrated that oleic acid (18:1Δ9) is desaturated to form linoleic acid (18:2Δ9,12) in a lipid-linked manner on PC (Sperling and Heinz, 1993; Sperling et al., 1993; Figure 3). This work found that desaturation could occur at the sn-1 or sn-2 position of PC based on extraction of PC from in vivo cell cultures followed by treatment with a stereospecific lipase (Sperling et al., 1993), but given that in PC polyunsaturated C18-fatty acids are more abundant at the sn-2 position, this is the major site of desaturation on PC in planta. Desaturation of 18:1 to 18:2 on PC is catalyzed by the desaturase Fatty Acid Desaturase 2 (FAD2), while desaturation of 18:2 to 18:3 on PC is catalyzed by FAD3 (Figure 3). These desaturase genes were discovered by isolating Arabidopsis mutants deficient in the fatty acid resulting from their activity (Miquel and Browse, 1992; Browse et al., 1993), and these genes were cloned and demonstrated to complement the mutant phenotype (Arondel et al., 1992; Okuley et al., 1994). Thus, it is believed that PC is synthesized from 16:0/18:1 or 18:1/18:1 DAG, with 16:0 primarily at the sn-1 position while 18:1 can occur at both the sn-1 and sn-2 positions, after which 18:1 is desaturated to form 18:2 followed by 18:3 (Figure 3; Browse and Somerville, 1991). Given that there is a lack of evidence for DAG or other lipids in the endoplasmic reticulum (ER) serving as substrates for desaturation, PC is believed to be the major site of fatty acid desaturation in this membrane system.
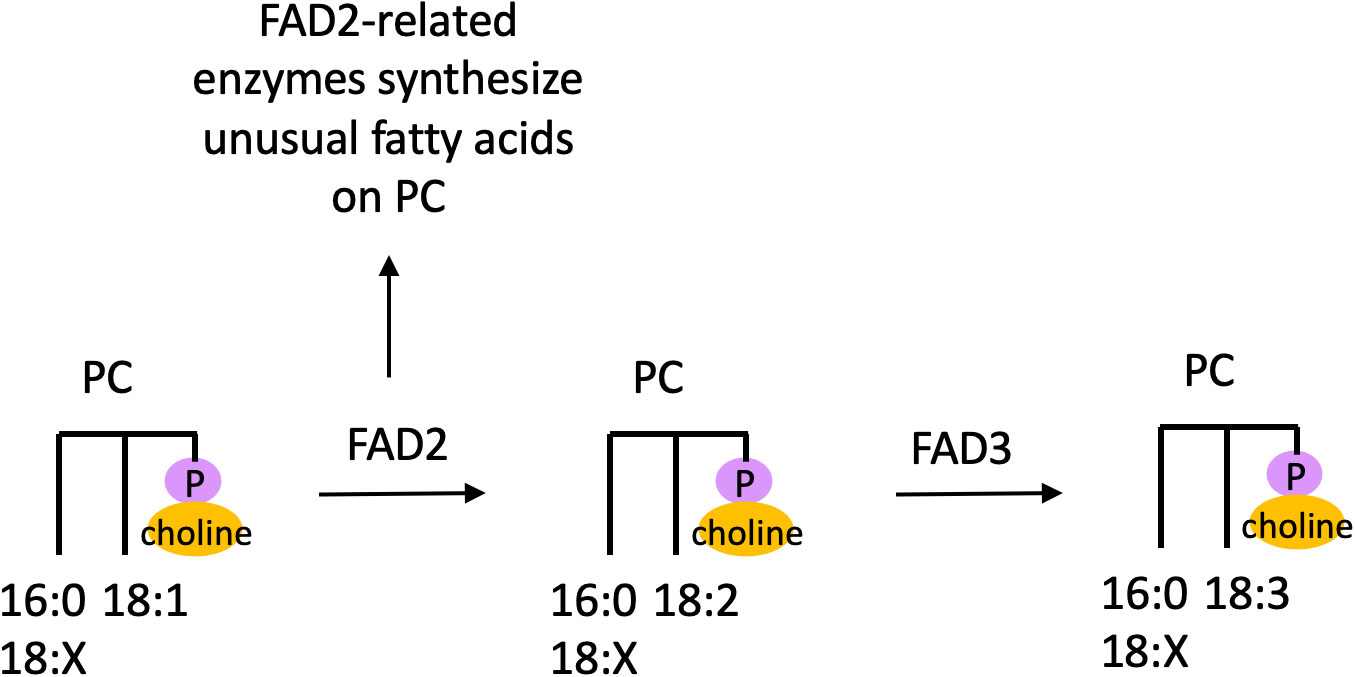
Figure 3 Desaturation and production of unusual fatty acids on PC. Desaturation and unusual fatty acid synthesis occurs on the sn-2 position of PC. Here ‘18:X’ refers to a fatty acid with 18 carbons and an unspecified number of double bonds. FAD, Fatty Acid Desaturase; PC, phosphatidylcholine.
Thus, PC is the site of extraplastidial desaturation, and acyl flux through PC is a major route for PUFA incorporation into TAG. The fatty acids of PC can reach TAG by three major routes as shown in Figure 4: 1) Fatty acids may be removed from PC by a phospholipase or acyltransferase and enter the acyl-CoA pool, where they are then available for glycerolipid synthesis. 2) PC may be converted into DAG, the precursor for TAG synthesis, by the reverse action of cytidine-5′-diphosphocholine:diacylglycerol cholinephosphotransferase (CPT), by phosphatidylcholine:diacylglycerol cholinephosphotransferase (PDCT), or by the action of phospholipase C or phospholipase D followed by phosphatidic acid phosphatase to remove the phosphate headgroup. 3) A fatty acid may be directly transferred from PC onto the sn-3 position of DAG to produce TAG by the action of phospholipid:diacylglycerol acyltransferase (PDAT) (Dahlqvist et al., 2000; Stahl et al., 2004). Each of these routes of acyl flux from PC can contribute to increase the PUFA content in TAG.
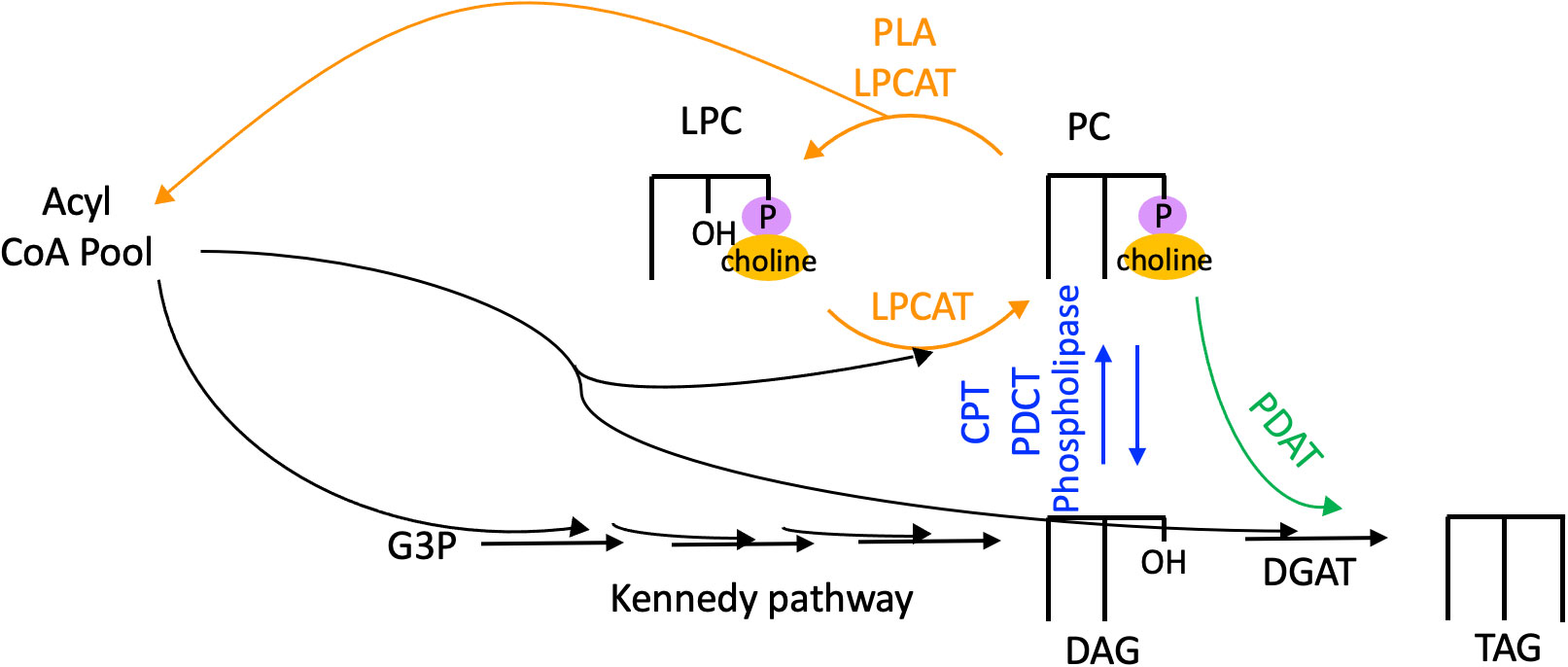
Figure 4 Routes of acyl flux through PC into TAG. Orange arrows, PC acyl editing reactions. Blue arrows, headgroup exchange for PC-DAG conversion. Green arrows, transfer of an acyl group from PC onto TAG via the acyltransferase PDAT. CPT, cytidine-5′-diphosphocholine:diacylglycerol cholinephosphotransferase; DAG, diacylglycerol; DGAT, diacylglycerol acyltransferase; G3P, glycerol 3-phosphate; LPC, lyso-phosphatidylcholine; LPCAT, acyl-CoA:lysophosphatidylcholine acyltransferase; PC, phosphatidylcholine; PDAT, phospholipid:diacylglycerol acyltransferase; PDCT, phosphatidylcholine:diacylglycerol cholinephosphotransferase; PLA, phospholipase; TAG, triacylglycerol.
In addition to desaturation, PC also acts as the substrate for several unusual fatty acid modifications (Figure 3). Mutations in plant fatty acid desaturases can give rise to alternate enzymatic activities such as hydroxylation, epoxygenation, and triple-bond formation. For example, many variants of the FAD2 desaturase, which desaturates oleic acid (18:1) to linoleic acid (18:2) on PC, have been discovered. In castor bean, a hydroxylase acts on the Δ12 position of oleic acid on the sn-2 position of PC to produce ricinoleic acid, and this hydroxylase is a homolog of FAD2 (Bafor et al., 1991; Van De Loo et al., 1995). An acetylenase in Crepis alpina is a variant of FAD2 that introduces a triple bond at the Δ12 position of linoleic acid on PC to form crepenynic acid, and this unusual fatty acid then accumulates in TAG (Banas et al., 1997). In addition, a Δ12-epoxygenase from Crepis palaestina catalyzes the formation of vernolic acid from linoleic acid, presumably on PC (Lee et al., 1998). Thus, PC is the key substrate for synthesizing PUFAs as well as uncommon fatty acids.
Although PC is the site of synthesis of unusual fatty acids, some plants contain high amounts of unusual fatty acids in TAG while PC contains low levels of these fatty acids. For example, in developing endosperms of castor bean, the hydroxylated fatty acid ricinoleic acid accumulates to only 5% in PC while it accumulates to ~85% in TAG despite its synthesis on PC (Stahl et al., 1995). Thus, acyl flux through the sn-2 position of PC must occur with high efficiency and selectivity. However, when the castor bean hydroxylase is transgenically expressed in Arabidopsis, hydroxy-fatty acids only accumulate to ~17% in seed TAG (Broun and Somerville, 1997). Isotopic labeling analyses using 14C-glycerol revealed a ~50% reduction in label incorporation in total lipids, primarily due to a reduction in the use of de novo DAG for PC synthesis (Bates and Browse, 2011). Thus, flux through PC can represent a bottleneck for the accumulation of particular fatty acids in TAG (Bates and Browse, 2011).
Another example of PC acting as a control point for fluxes of unusual fatty acids is the unusual fatty acid petroselinic acid (18:lcisΔ6), which is present in low levels in PC (15-20%) and high levels in TAG (70-75%) in both carrot and coriander seed endosperm (Cahoon and Ohlrogge, 1994). However, 14C-acetate time course labeling experiments revealed that at early time points, radiolabel was the most concentrated in PC and entered it at the highest rates, while at later time points radiolabel accumulated most strongly in TAG, and primarily (80-85%) in petroselinic acid (Cahoon and Ohlrogge, 1994). These results suggest that there is significant flux of petroselinic acid from PC into TAG. Similar results were found in Thunbergia alata, whose unusual monoenoic fatty acid 16:1Δ6 comprises >80% of TAG fatty acids and which appears first in PC (Schultz and Ohlrogge, 2000). Therefore, in order to engineer high levels of select fatty acids in transgenic oilseeds or algae, the major fluxes of TAG synthesis, including the sites of modification and distribution, must be elucidated.
Mechanisms of acyl editing and acyl flux through PC into TAG
PC conversion into DAG for TAG synthesis has been shown to be an important biochemical pathway in oilseeds. For instance, 14C-acetate and 14C-glycerol labeling of developing soybean embryos demonstrated that over 95% of TAG synthesis was found to be derived from DAG generated from PC, or “PC-derived DAG” (Bates et al., 2009; Figure 5). This TAG synthesis pathway was also found to be significant in Arabidopsis seeds, with 14C-glycerol labeling revealing that PC-derived DAG is utilized for as much as 93% of TAG synthesis (Bates and Browse, 2011). Thus, in several oilseeds de novo-synthesized DAG is converted to PC, which is converted back to DAG, which produces TAG (Figure 5). However, developing castor-bean endosperm appears to utilize de novo-synthesized DAG to produce TAG (Bafor et al., 1991), so this pathway is not ubiquitously utilized by all plants.
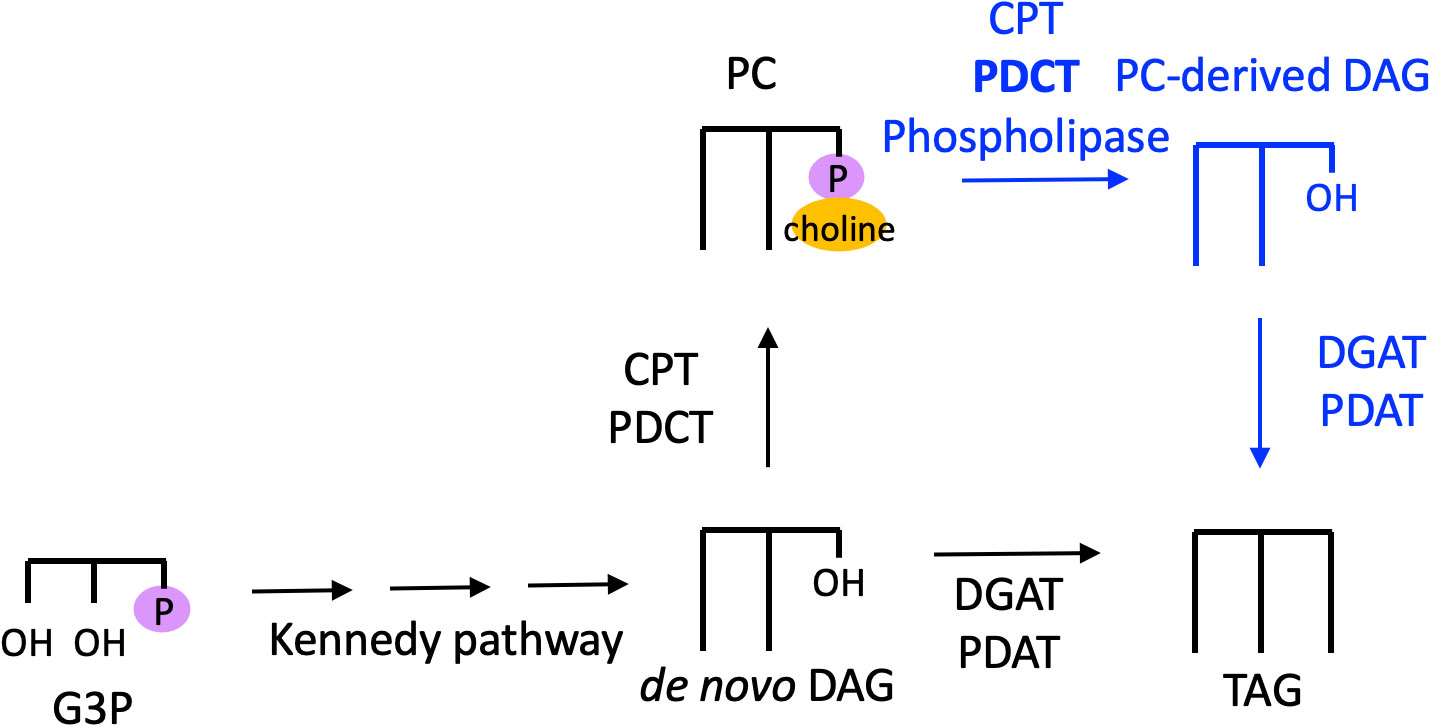
Figure 5 PC-derived DAG pathway to TAG synthesis. Black text and arrows represent the de novo synthesis pathway to PC and TAG, blue text and arrows represent the PC-derived DAG pathway to produce TAG. The enzyme PDCT is shown in bold because it is believed to catalyze the major PC-DAG conversion. CPT, cytidine-5′-diphosphocholine:diacylglycerol cholinephosphotransferase; DAG, diacylglycerol; DGAT, diacylglycerol acyltransferase; G3P, glycerol 3-phosphate; PC, phosphatidylcholine; PDAT, phospholipid:diacylglycerol acyltransferase; PDCT, phosphatidylcholine:diacylglycerol cholinephosphotransferase; TAG, triacylglycerol.
PC-derived DAG can be synthesized by the reverse action of CPT, which produces PC (Slack et al., 1983). PC-DAG interconversion can also be catalyzed by PDCT, which reversibly exchanges the phosphocholine headgroup between PC and DAG. DAG may also be produced from PC via the action of phospholipase C, or via phospholipase D followed by phosphatidic acid phosphatase. However, characterization of the Arabidopsis rod1 mutant, which encodes PDCT, revealed that at least 40% of the PUFAs in TAG are derived from PC-derived DAG produced by this enzyme (Lu et al., 2009). Thus, PDCT catalyzes the major PC-DAG interconversion in developing Arabidopsis seeds (Figure 5).
In addition to headgroup removal, PC can also contribute to TAG synthesis via the well-known PDAT enzyme, which has been characterized in yeast, oilseeds, and other plant tissues (Dahlqvist et al., 2000; Stahl et al., 2004). PDAT catalyzes an acyl-CoA independent pathway of TAG formation by transferring an acyl group from the sn-2 position of PC onto DAG (Figure 4). PDAT is known to conduct significant flux into TAG in yeast and oilseeds, although knockout of PDAT in Arabidopsis resulted in no statistically significant difference in seed lipid content, likely due to compensation by diacylglycerol acyltransferase (DGAT) activity (Mhaske et al., 2005). In microalgae, pdat insertional mutants appeared to accumulate 25% less TAG during nitrogen deprivation compared to the parental strain (Boyle et al., 2012), suggesting that it may be a determinant of TAG accumulation in algae. However, artificial miRNA-silenced PDAT knockdowns in Chlamydomonas reinhardtii did not show significant reduction of TAG under N-deprivation (Yoon et al., 2012), and further characterization of C. reinhardtii pdat knockout mutants suggested that PDAT primarily regulates TAG biosynthesis under favorable conditions rather than persistent stress conditions (Lee et al., 2022). Thus, PDAT may play a diminished role in TAG synthesis in algae compared to yeast and plants.
Two studies probed the enzymes underlying the acyl editing mechanism in Arabidopsis seeds by generating double mutants in both LPCAT genes of Arabidopsis (lpcat1/lpcat2) (Bates et al., 2012; Wang et al., 2012), which deacylate and reacylate lyso-PC and PC in the acyl editing cycle (Figure 2). Both studies observed a reduction of PUFA content in seeds by ~10% in the lpcat1/lpcat2 double mutant (Bates et al., 2012; Wang et al., 2012). In addition, both studies used 14C-labeling to confirm that in the double mutants, nascent fatty acids were incorporated in the de novo synthesis route of DAG followed by PC rather than by direct incorporation into PC characteristic of acyl editing (Bates et al., 2012; Wang et al., 2012). Both studies also found that the lpcat1/lpcat2 double mutants accumulated lyso-PC, indicating they were impaired in their ability to reacylate lyso-PC to PC. Thus, these studies provided strong evidence that the LPCAT genes are crucial components of the acyl editing cycle in Arabidopsis. In addition, Bates et al. generated a triple mutant lpcat1/lpcat2/rod1 that was also deficient in PDCT, which interconverts DAG and PC. The triple mutant’s PUFA content in seed TAG was reduced by about two-thirds (Bates et al., 2012), suggesting that PDCT and the LPCAT genes are responsible for the majority of fatty acid flux in and out of PC, via PC-DAG interconversion by PDCT and acyl editing by LPCAT.
Early evidence in microsomes of developing safflower cotyledons suggested that acyl exchange on PC was catalyzed by the forward and reverse reactions of LPCAT (Stymne and Stobart, 1984), and this was later confirmed by expressing Arabidopsis LPCAT2 in yeast and measuring LPCAT activity by the rate of incorporation of 14C-18:1 fatty acid into PC (Lager et al., 2013). These assays revealed that LPCAT1 and LPCAT2 can catalyze both the acylation and deacylation of PC (Lager et al., 2013). While both LPCATs can acylate and deacylate at the sn-1 position, the sn-2 position is strongly preferred (Lager et al., 2013). Both LPCAT enzymes showed low activity toward 16:0 fatty acid, and thus are probably not involved in acylating or deacylating 16:0 on PC (Lager et al., 2013). Thus, LPCAT is reversible in vitro, although it is still possible that acyl editing could proceed via the action of a phospholipase followed by the forward reaction of LPCAT to regenerate PC.
In addition to LPCAT reversibility, Lager et al. used microsomes of developing safflower seeds to characterize other reactions involved in PC acyl editing. By performing isotopic labeling experiments with [14C]18:1-lyso-PC and [14C]choline, they demonstrated that lyso-PC:lyso-PC transacylation occurred, and named this activity lysphosphatidylcholine transacylase (LPCT) (Lager et al., 2015). Thus, LPCT activity utilizes two lyso-PC molecules and produces PC and glycerophosphocholine (GPC; Figure 6A). By incubating microsomal preparations of various developing oilseeds in [14C]GPC and 18:1-CoA and measuring the radioactive PC product, acyl-CoA:glycerophosphocholine acyltransferase (GPCAT) activity was also demonstrated (Lager et al., 2015), which catalyzes the acylation of GPC to lyso-PC (Figure 6B). The enzyme responsible for LPCT activity has yet to be identified, but the GPCAT gene has been identified and cloned in Arabidopsis, and GPCAT homologs have been found across other eukaryotic clades including green algae (Głąb et al., 2016).
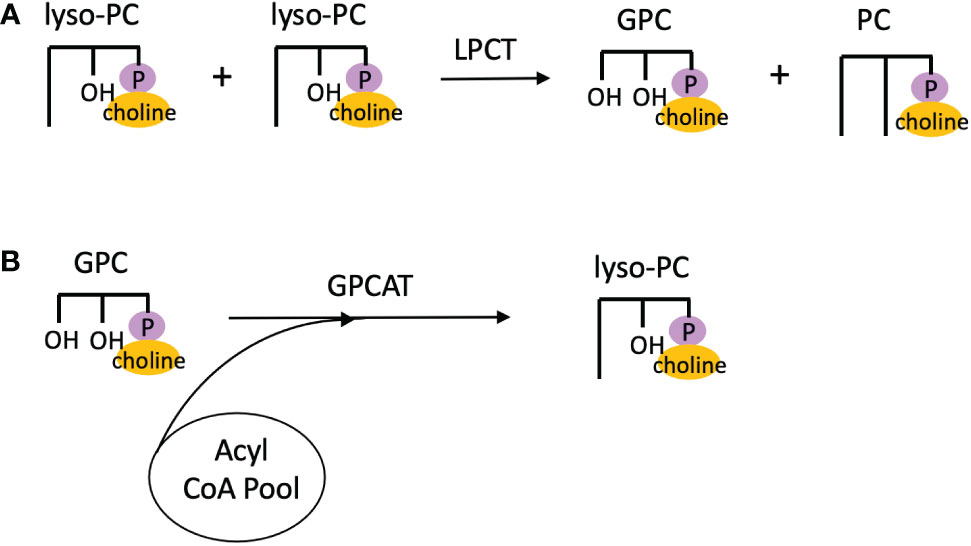
Figure 6 Additional PC acyl editing reactions. (A) Lyso-PC:lyso-PC transacylation via LPCT activity. (B) Acylation of GPC by GPCAT activity. GPC, glycerophosphocholine; GPCAT, acyl-CoA:glycerophosphocholine acyltransferase; LPCT, lysphosphatidylcholine transacylase; PC, phosphatidylcholine.
Many studies on developing seeds are performed in vitro, and one study sought to compare in vitro versus in vivo growth on acyl lipid metabolism in Camelina sativa leaves (Klińska et al., 2021). Significant differences were observed between the two growth conditions, with in vivo leaves containing over twice the total fatty acid content of in vitro leaves, in vivo conditions resulting in a higher degree of desaturation in fatty acids compared to in vitro, and the dominant lipid in in vitro conditions being PC rather than monogalactosyldiacylglycerol (MGDG) (Klińska et al., 2021). Interestingly, higher activity of acyl-CoA:lysophospholipid acyltransferases (LPLATs) was observed in vitro, as well as an increased rate of phospholipid remodeling (Klińska et al., 2021). Collectively, these results indicate that acyl lipid metabolism and acyl editing differ substantially between in vitro and in vivo conditions, and results from one condition should not be extrapolated to the other.
Acyl editing is also known to occur in response to stress, such as temperature stress, salt stress, and nutrient deprivation. For instance, lipidomic profiling during chilling stress in barley roots revealed that as particular molecular species of PC decreased, those molecular species increased in PA (Vilchez et al., 2021). Transcriptional analysis of genes involved in acyl editing revealed that the barley LPCAT gene was upregulated within five hours of cold recovery, and phospholipase D activity rose during cold treatment and after five hours of cold recovery (Vilchez et al., 2021). Thus, it is likely that PC is hydrolyzed to produce PA during cold stress and recovery, and that acyl editing of PC plays an important role in chilling stress and recovery.
Betaine lipids may replace PC as a fatty acid editing hub in algae
In flowering plants, PC is the major extraplastidial membrane lipid, but some algae and nonflowering plants contain DGTS and/or other betaine lipids in place of PC as the major extraplastidial lipid. DGTS is a betaine lipid that is structurally highly similar to PC, but it lacks phosphate in its headgroup and rather contains an ether bond linking the headgroup to the glycerol backbone (Figure 1). Due to its structural similarity to PC, DGTS is widely believed to replace the function of PC in extrachloroplastic membranes, and several studies in algae and diatoms containing DGTS have found evidence that DGTS may substitute for several of the functions of PC.
The green microalga C. reinhardtii is the most studied alga in lipid and other areas of metabolism. C. reinhardtii lacks PC and rather contains DGTS, and an inverse relationship between the quantities of these two lipids occurs in many species of microalgae (Dembitsky, 1996). When 14C-oleic acid is supplied exogenously to C. reinhardtii cells, label is rapidly incorporated into DGTS and it contains the majority of the radiolabel, similar to that which is observed in PC in flowering plants (Schlapfer and Eichenberger, 1983; Giroud and Eichenberger, 1989). The golden-brown microalga Ochromonas danica contains the betaine lipids DGTS and diacylglycerylhydroxymethyltrimethyl-β-alanine (DGTA), and small amounts of PC. When this alga was incubated with 14C-oleic acid, the majority of the radiolabel was incorporated into DGTS, suggesting that DGTS is the primary acceptor of exogenous oleic acid (Vogel and Eichenberger, 1992). Similar findings were observed in the brown algae Fucus vesiculosus and Ascophyllum nodosum, which contain DGTA and minor quantities of PC. In these algae, labeling with 14C-acetate revealed DGTA to be the lipid with the highest incorporation of radioactivity (Jones and Harwood, 1993). Thus, in algae containing ether-linked betaine lipids in place of PC, supplying exogenous labeled fatty acids or acetate results in the early and strong incorporation of label into betaine lipid, analogous to the rapid incorporation of radiolabel into PC observed in land plants.
In addition to being the first major lipid into which exogenous radiolabel is incorporated, DGTS also appears to be the site of extraplastidial desaturation of C18 fatty acids akin to PC. When 14C-oleic acid was supplied exogenously to C. reinhardtii, radiolabel first appeared in molecular species of DGTS containing 18:1, and then shifted to species containing 18:2, followed by species containing 18:3Δ5,9,12, suggesting that C18 fatty acid desaturation occurs on DGTS (Schlapfer and Eichenberger, 1983; Giroud and Eichenberger, 1989). Similar results were obtained in a pulse-chase labeling experiment in the golden-brown microalga O. danica, in which labeling with 14C-oleic acid resulted in radiolabel being primarily concentrated in DGTS in its 18:1 and 18:2 fatty acids (Vogel and Eichenberger, 1992). During the chase, radiolabel decreased very rapidly in 18:1 while it decreased more slowly in 18:2 fatty acids, and radiolabel increased strongly in 18:3 and 18:4 fatty acids (Vogel and Eichenberger, 1992). This suggested that 18:1 is desaturated on DGTS to produce 18:3 and 18:4 fatty acids. Thus, in algae containing DGTS rather than PC, DGTS appears to take on PC’s role as a substrate of extraplastidial fatty acid desaturation.
Labeling experiments in oilseed plants using 14C-acetate have demonstrated that radiolabeled fatty acids are rapidly incorporated into PC prior to their incorporation into DAG and TAG (Bates et al., 2009). Pulse-chase experiments in C. reinhardtii cells supplied with 14C-radiolabeled fatty acids in nutrient replete medium and then transferred into unlabeled, nitrogen-deprived medium to induce TAG accumulation revealed that radiolabel was rapidly lost from DGTS while it increased in TAG during the chase period (Xu et al., 2016). Similarly, when the brown alga F. vesiculosus was incubated with 14C-acetate, radiolabel was initially most concentrated in the betaine lipid DGTA, and over time decreased in DGTA while increasing strongly in neutral lipids, primarily TAG (Jones and Harwood, 1993). Thus, pulse-chase experiments with radiolabeled substrates indicate that fatty acids pass through betaine lipids into TAG in algae in a similar manner as PC in land plants.
In addition to pulse-chase experiments, several lipidomics-based analyses point to fatty acid flux through betaine lipids into TAG. One study utilizing subcellular lipidomics to investigate the origins of TAG formation in nitrogen-deprived C. reinhardtii indicated that the membrane lipids MGDG, digalactosyldiacylglycerol (DGDG), and DGTS all contribute acyl chains to TAG accumulation (Yang et al., 2018). In particular, they noted that as certain molecular species of DGTS accumulated, there was a corresponding increase in the levels of those acyl chains in TAG (Yang et al., 2018). In support of this finding, a glycerolipidomics study of the BAFJ5 starchless mutant in C. reinhardtii subjected to high light and nitrogen deprivation concluded that 18:3Δ9,12,15/16:0 from DGDG and 16:0/18:3Δ5,9,12 from DGTS were major contributors to 18:3 accumulation in TAG (Yang et al., 2020). The diatom Phaeodactylum strain Pt4 (UTEX 646) contains DGTA as the major extraplastidial lipid and minor amounts of PC (Abida et al., 2015), and analysis of its lipid molecular species composition during nitrogen deprivation indicated that the betaine lipid was the largest membrane lipid contributor of fatty acids to TAG accumulation (Popko et al., 2016). The major TAG species that accumulated were matched to a reduction in those corresponding species in MGDG and the betaine lipid, but only the decrease in the latter was large enough to account for the increase observed in TAG (Popko et al., 2016). Thus, several lines of evidence indicate that betaine lipids serve as a major source of fatty acids for TAG synthesis.
The relationship between DGTS composition and levels and TAG accumulation was further evident in knockdown transformants in the betaine lipid synthase gene (BTA1) in C. reinhardtii. Artificial microRNA knockdown of the BTA1 gene expression level led to a significant decrease in DGTS and MGDG contents while TAG increased 2-3 fold (Lee et al., 2017). The authors postulate that the observed decrease in MGDG was due to the indirect effect of ER stress due to the substantial decrease of DGTS. Their results indicated that the synthesis of DGTS was inhibited by the reduced expression of BTA1, thus resulting in an increased amount of DAG that could not be converted into DGTS, which was then available for TAG synthesis (Lee et al., 2017). This was evidenced by a 40% reduction in DGTS content in the BTA1 gene knockdown lines compared to wild type and empty vector controls, and a 2-3 fold increase in the amounts of DAG and TAG (Lee et al., 2017). On the other hand, the reduction in MGDG was interpreted to be due to induced breakdown due to ER stress rather than inhibition of its synthesis, thus releasing fatty acids which could then contribute to TAG synthesis (Lee et al., 2017). Therefore, the effects of downregulating BTA1 expression suggested that DGTS plays a significant role in the accumulation of TAG in C. reinhardtii.
Due to structural differences between DGTS and PC, the mechanism by which DGTS contributes to TAG accumulation likely differs from that of PC. DGTS contains an ether bond linking its headgroup to the glycerol backbone (Figure 1), making it unlikely for DGTS’s headgroup to be chemically or enzymatically removed to form DAG as occurs in PC. Enzymes capable of breaking this ether bond have not yet been identified, but are presumed to exist in order for cells to catabolize DGTS. Moreover, the vast majority of molecular species of DGTS contain a C18 fatty acid on the sn-2 position (Giroud et al., 1988) while ~90% of TAG molecular species contain a C16 fatty acid on the sn-2 position (Fan et al., 2011), thus supporting the idea that DGTS is not a direct precursor to DAG for TAG assembly. Therefore, fatty acid flux from DGTS into TAG probably occurs via the action of lipases or acyltransferases. In support of this hypothesis, Vogel and Eichenberger, 1992 observed turnover of radiolabel in the 18:2 fatty acid of DGTS that was substantially faster than the turnover of its polar headgroup. Similarly, 13C-labeling of C. reinhardtii lipids followed by an unlabeled chase into nitrogen deprivation revealed very little turnover of DGTS’s glycerol backbone and homoserine betaine headgroup compared to its fatty acids (Young et al., 2022). In addition, several studies have found that the quantity of DGTS stays constant in C. reinhardtii cells during nitrogen deprivation (Fan et al., 2011; Yang et al., 2018), despite high rates of TAG accumulation and the occurrence of fatty acid flux from DGTS into TAG. Therefore, it is probable that fatty acids from DGTS reach TAG by deacylation rather than removal of the betaine headgroup to form a DAG molecule that is then converted into TAG.
On the other hand, there is evidence that MGDG rather than DGTS is converted into DAG for TAG synthesis in C. reinhardtii. Lipidomic analysis of heat-stressed C. reinhardtii revealed a strong decrease in the major MGDG molecular species and an accumulation of this species in DAG and TAG (Légeret et al., 2016). This finding was corroborated by their use of a crfad7 mutant, which altered the major MGDG molecular species and confirmed its decrease under heat stress and accumulation in DAG and TAG (Légeret et al., 2016). In accordance with this, Young et al., 2022 found evidence of MGDG recycling into DAG and TAG in C. reinhardtii during nitrogen deprivation, as isotopic label decreased in the major MGDG species and increased in the corresponding species of DAG and TAG. In addition, the stereochemical positions of the characteristic fatty acids of the major MGDG species were matched to the identical positions in DAG and TAG (Young et al., 2022). Data mining of the C. reinhardtii lipid body proteome (Moellering and Benning, 2010) led to the identification of a putative galactosyl hydrolase gene (CrGH), and insertional mutants in this gene have reduced TAG content (Gu et al., 2021). Thus, the route of acyl flux through PC via headgroup removal appears to be replaced in microalgae by removal of the galactosyl headgroup of MGDG rather than headgroup removal of DGTS.
DGTS appears to play a role in phosphorus-sparing in microalgae. Under optimal growth conditions, the green alga Chlorella kessleri does not contain DGTS, and contains PC as the dominant extraplastidic lipid. Within 48 hours of transfer to phosphorus-deficient conditions, this alga almost completely replaces the phospholipids PC and phosphatidylethanolamine (PE) with an equivalent amount of DGTS (Oishi et al., 2022). Interestingly, the fatty acid composition of DGTS was nearly identical to that of PC and PE in phosphorus-replete conditions (Oishi et al., 2022). C. kessleri was found to contain a homolog of the C. reinhardtii BTA1 gene, and the expression of this gene appears to be induced under phosphorus-deprived conditions in order to lower the alga’s phosphorus demand (Oishi et al., 2022). Similar findings were reported in the heterokont Nannochloropsis oceanica, which contains both DGTS and PC under nutrient replete growth conditions. During phosphorus deprivation, PC is reduced by 95% in N. oceanica, while DGTS levels strongly increased, becoming the most abundant membrane lipid in the cells (Meng et al., 2019). Upon resupply of phosphorus to the cells, PC levels increased and DGTS fell to pre-deprivation levels (Meng et al., 2019). This study also found that DGTS appeared to replace PC as the substrate for C18 fatty acid desaturation and acyl remodeling under phosphorus deprivation (Meng et al., 2019). Thus, levels of DGTS and PC are inversely correlated, and DGTS appears to substitute for PC under phosphorus-deprived conditions due to it being a non phosphorus-containing lipid.
DGTS may also aid microalgae in adaptation to lower temperatures. When the haptophyte microalga Pavlova lutheri was subjected to a low temperature, an increase in the relative amount of PUFAs and betaine lipid was observed (Tatsuzawa and Takizawa, 1995). At 15°C, the relative percentage of betaine lipid in P. lutheri increased four-fold compared to cells grown at 25°C (Tatsuzawa and Takizawa, 1995). In the heterokont N. oceanica, mutants lacking either DGTS synthesis (bta1) or PC synthesis were generated in order to determine which lipid plays a role in adaptation to lower temperatures (Murakami et al., 2018). Only the bta1 mutant which lacked DGTS synthesis displayed significantly impaired cell growth at low temperatures, indicating that DGTS is required for maintaining optimal growth at low temperatures (Murakami et al., 2018). In both P. lutheri and N. oceanica, DGTS contains a high a proportion of 20:5 fatty acid, therefore it is postulated that enhanced DGTS under low temperatures aids in maintaining membrane fluidity by having a high PUFA content (Tatsuzawa and Takizawa, 1995; Murakami et al., 2018).
Evidence for acyl editing substrates in plants and microalgae apart from PC and DGTS
Indications of an MGDG acyl editing cycle in C. reinhardtii began with a study characterizing a galactoglycerolipid lipase gene named PLASTID GALACTOGLYCEROLIPID DEGRADATION1 (PGD1) (Li et al., 2012). This gene was discovered in an insertional mutant screen for lowered TAG content during nitrogen deprivation, and the insertion was found to be in a putative lipase-encoding gene. In terms of acyl composition, the pgd1 mutant contained a lower amount of oleic acid (18:1) in TAG (Li et al., 2012). In vitro lipase assays revealed MGDG to be the substrate of the PGD1 lipase, and PGD1 preferentially hydrolyzes newly synthesized MGDG (18:1Δ9/16:0) at the sn-1 position (Li et al., 2012). Pulse-chase analyses using 14C-acetate revealed that the proportion of label remained higher in MGDG and DGDG in the pgd1 mutant during nitrogen deprivation, whereas in the wild type the label decreased in membrane lipids as it increased in TAG (Li et al., 2012). Thus, PGD1 is a lipase that preferentially releases 18:1Δ9 from newly-made MGDG, and the released fatty acid joins the acyl-CoA pool where it is available to contribute to TAG synthesis (Figure 7).
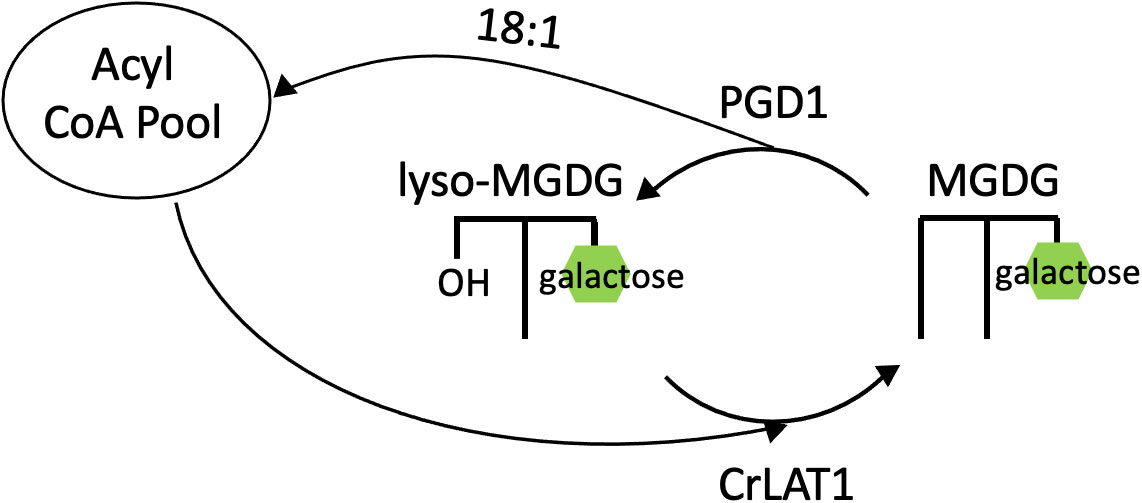
Figure 7 Acyl editing cycle of MGDG in C. reinhardtii. Removal of 18:1 from newly made MGDG at the sn-1 position by PGD1, and reacylation of lyso-MGDG catalyzed by CrLAT1. CrLAT1, C. reinhardtii Lysolipid Acyltransferase 1; MGDG, monogalactosyldiacylglycerol; PGD1, Plastid Galactoglycerolipid Degradation 1.
A follow up study further explored the role of PGD1 in membrane lipid turnover and remodeling in C. reinhardtii in response to unfavorable environmental conditions (Du et al., 2018). In this study, sensitivity of lipid analyses was increased by isolating chloroplasts, and an increased level of MGDG in pgd1 mutants compared to wild type was observed (Du et al., 2018). In terms of MGDG acyl composition, a lower quantity of 16:3 and 18:2 was observed but a higher amount of 16:4 and 18:3 in pgd1 cells compared to wild type (Du et al., 2018). This supports the model of PGD1-mediated turnover of MGDG 18:1Δ9 into TAG, as pgd1 mutants are impaired in the flux of 18:1 from MGDG into TAG, thereby allowing oleate to reside in MGDG longer and thus become desaturated. Thus, these studies point to an acyl editing cycle on MGDG during nitrogen-deprived conditions.
Recently, an enzyme that appears to catalyze the reacylation of lyso-MGDG (MGDG with one fatty acid removed) has been characterized, and this activity would complete an acyl editing cycle of MGDG. A homolog of the Arabidopsis thaliana LPCAT1 gene was discovered in C. reinhardtii (CrLAT1), and this gene contained a conserved membrane-bound O-acyl transferase (MBOAT) domain (Iwai et al., 2021). Knockdown CrLAT1 mutants contained an increased proportion of lyso-MGDG and decreased TAG accumulation compared to wild type cells (Iwai et al., 2021). In contrast, overexpression CrLAT1 mutants contained a lower proportion of lyso-MGDG compared to wild type cells (Iwai et al., 2021). The most abundant fatty acid in lyso-MGDG was 16:4 with a minor proportion of other C16 fatty acids, supporting a model in which CrLAT1 acylates lyso-MGDG with a C18 fatty acid. This acyl editing mechanism in C. reinhardtii consists of PGD1 hydrolyzing newly-made 18:1Δ9/16:0 MGDG, releasing 18:1Δ9 to the cytosolic acyl-CoA pool where it is available for TAG synthesis, and CrLAT1 may catalyze the reacylation of lyso-MGDG at the sn-1 position with a C18 fatty acid (Figure 7).
Evidence of acyl editing on lipid substrates other than PC or DGTS has also been observed in land plants. In one study, a Δ6 desaturase from Physcomitrella patens was introduced into Arabidopsis, which lacks a Δ6 desaturase. The Δ6 desaturase is ER-localized with a preference for the sn-2 position of PC, and indeed in the transformant ~90% of the Δ6 fatty acids in PC were located in the sn-2 position (Hurlock et al., 2018). Δ6 acyl groups are synthesized in the ER and must be imported into the chloroplast, and they would presumably be located at the sn-2 position of chloroplastic lipids if acyl editing does not occur. However, in each of the chloroplastic lipids [MGDG, DGDG, sulfoquinovosyldiacylglycerol (SQDG), and phosphatidylglycerol (PG)], the Δ6 acyl groups were approximately equally distributed between the sn-1 and sn-2 positions, indicating that acyl editing of plastidial lipids does occur (Hurlock et al., 2018). Interestingly, PA isolated from whole leaf tissues displayed the same stereochemical distribution of Δ6 fatty acids as PC, while PA isolated from chloroplasts displayed the reverse stereochemistry with the majority of the Δ6 fatty acids located at the sn-1 position (Hurlock et al., 2018). Thus, some portion of PA is likely subject to acyl editing as well. Furthermore, through liquid chromatography mass spectrometry (LC-MS) a 34:7 MGDG molecular species was identified that contained one ER-derived fatty acid and one chloroplast-derived fatty acid, as it contained one 18:4 fatty acid with a Δ6 desaturation synthesized in the ER and one 16:3 fatty acid that is synthesized exclusively in chloroplast via the FAD5 desaturase (Hurlock et al., 2018). This supports the idea that acyl editing occurs on MGDG, as one exclusively ER-derived fatty acid and one exclusively chloroplast-derived fatty acid were found on the same molecule. However, the transgenic lines appeared to have higher lipid turnover compared to wild type (Hurlock et al., 2018), therefore it is possible that increased acyl editing may have been triggered in the transgenic line.
One study characterizing the function of a phospholipase A1, PLASTID LIPASE1 (PLIP1), in Arabidopsis found evidence of acyl editing on the plastidic phospholipid PG. PLIP1 contains a conserved Lipase 3 domain, similar to the PGD1 lipase of C. reinhardtii (Wang et al., 2017). In vitro assays of PLIP1 revealed that its preferred substrate is 18:3/16:1Δ3t PG, and that PLIP1 acts on the sn-1 position to release the 18:3 fatty acid (Wang et al., 2018). A pulse-chase experiment using 14C-acetate in leaves of PLIP1 overexpressor lines in Arabidopsis revealed rapid incorporation of label in PG compared to wild type, with PG containing ~70% of the total label by the end of the pulse phase (Wang et al., 2018). During the chase phase, PG rapidly lost most of its label while PC rapidly accumulated label (Wang et al., 2017). A 14C-acetate pulse-chase experiment in developing Arabidopsis seeds also demonstrated higher incorporation of label into PG compared to wild type seeds and increased turnover of PG during the chase period, while label concomitantly turned over in PC and accumulated heavily in TAG (Wang et al., 2018). Furthermore, plip1 insertional mutants displayed ~10% lowered seed fatty acid content (the majority of which is in TAG) compared to wild type, while PLIP1 overexpressors had a 40-50% increase in seed fatty acid content (Wang et al., 2018). Taken together, these results indicate that PLIP1 transfers a polyunsaturated fatty acid from PG into PC, and this fatty acid fluxes through PC and contributes to TAG synthesis (Figure 8). Thus, PLIP1 appears to be part of an acyl editing cycle that acts on PG and contributes to the flux of PUFAs through PC into TAG.
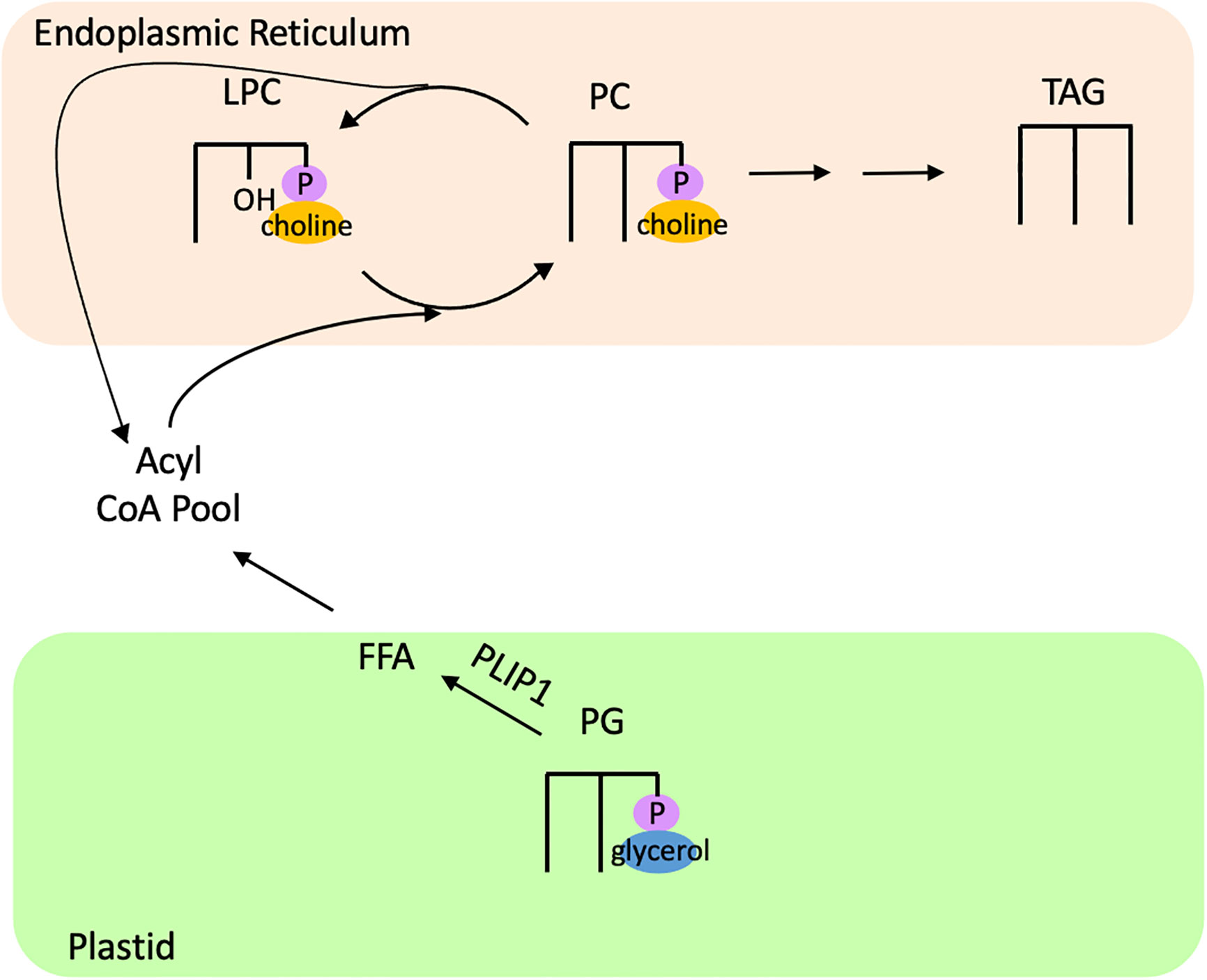
Figure 8 Acyl editing of PG in Arabidopsis contributes to TAG synthesis. The lipase PLIP1 releases 18:3 from 18:3/16:1Δ3t PG, which is channeled into PC and eventually incorporated into TAG. FFA, free fatty acid; LPC, lysophosphatidylcholine; PC, phosphatidylcholine; PG, phosphatidylglycerol; PLIP1, Plastid Lipase 1; TAG, triacylglycerol.
In addition, the lipase HEAT INDUCIBLE LIPASE1 (HIL1) in Arabidopsis was characterized via in vitro lipase activity assays and found to have a substrate preference for MGDG (Higashi et al., 2018). Insertional hil1 mutants are impaired in the remodeling of 18:3 into TAG during heat stress (Higashi et al., 2018), thus indicating that HIL1 plays a role in the turnover of PUFAs from MGDG to TAG.
Previous studies demonstrated that LPCAT can catalyze both the acylation and deacylation of PC via the forward and reverse reactions, respectively (Lager et al., 2013). In vitro assays investigated the capability of Arabidopsis LPCAT2 and Arabidopsis lysophosphatidylethanolamine acyltransferase (LPEAT2) to catalyze the forward and reverse reactions in the phospholipids PE and PA (Jasieniecka-Gazarkiewicz et al., 2016). This study found that although both AtLPCAT2 and AtLPEAT2 could catalyze the reverse reaction (deacylating an intact phospholipid), the activity of the reverse reaction varied greatly between phospholipids and was the lowest for PA (Jasieniecka-Gazarkiewicz et al., 2016). However, these enzymatic assays established the possibility of acyl remodeling of PE and PA. The substrate specificity and forward and reverse activity of LPAAT and LPEAT were then assayed in microsomal fractions of Camelina sativa seeds (Klińska et al., 2020). In terms of substrate specificity in the forward reaction (i.e. acylating a lyso-phospholipid to form a phospholipid), 16:0 and unsaturated C18 fatty acids were the preferred substrates of LPAAT, while unsaturated C18 fatty acids were the preferred acyl donors in the LPEAT-catalyzed reaction (Klińska et al., 2020). The activity of the reverse reaction (deacylating a phospholipid) was assayed by incubating 14C-acyl-CoAs with PA or PE, and it was found that both LPAAT and LPEAT could catalyze the backward reaction, although the acyl donor used affected the degree of activity observed (Klińska et al., 2020). Although the back-reaction could be performed by LPEAT, PE displayed a very slow remodeling rate and only contributed about 2% to the fatty acids in mature C. sativa seeds (in which TAG comprises ~93% of total lipids), despite PE constituting ~13-20% of all polar lipids (Pollard et al., 2015; Klińska et al., 2020). However, PE may donate a fatty acid to TAG synthesis via the action of PDAT, and the resulting lyso-PE could be reacylated with acyl-CoA by LPEAT. Interestingly, although PA only constitutes a minor fraction of polar lipids in C. sativa seeds (~2-4%), it appears ~5% of fatty acids in mature C. sativa seeds are first esterified to PA, before being transferred to the acyl-CoA pool via LPAATs (Klińska et al., 2020). This degree of acyl editing on PA is surprising given that desaturation is not known to occur on PA, although PA constitutes a relatively small proportion of membrane lipids and ultimately is not a substantial fatty acid contributor to seed oil content. Thus, PA and PE are potential substrates of acyl remodeling in plants, although neither seems to contribute flux as significant as PC.
Discussion
Acyl flux through PC serves several important functions in plants, including acting as a major site of extraplastidial desaturation, as a distributor of PUFAs into other lipids via acyl editing, and as an important hub of acyl flux into the neutral lipid TAG. Interestingly, the model green microalga C. reinhardtii completely lacks PC, and many species of microalgae contain highly reduced PC content, thus raising the question of if and how the functions of PC are replaced in microalgae. Given their inverse relationship and the structural similarity of DGTS and other betaine lipids to PC, it seems reasonable to postulate that betaine lipids substitute for the functions of PC in microalgae. Consistent with such roles is the evidence described above of rapid incorporation of exogenous fatty acids into DGTS, extraplastidial desaturation on DGTS, and substantial fatty acid flux through DGTS into TAG. However, the structure of DGTS makes it highly improbable that an analogous biochemical reaction of PC headgroup removal could occur on DGTS, and studies have found evidence of slower turnover of the DGTS backbone and headgroup compared to its fatty acids (Vogel and Eichenberger, 1992; Young et al., 2022). Additionally, knockout and characterization of the C. reinhardtii homolog of PDAT have not shown that DGTS replaces PC as the substrate of this enzyme in algae (Boyle et al., 2012; Yoon et al., 2012). In fact, phylogenetic analysis of the PDAT protein revealed the divergent evolution of PDAT in plants and algae and suggested that PDAT has a diminished and/or different role in green algae (Falarz et al., 2020). Similarly, knockdown of the C. reinhardtii homolog of Arabidopsis LPCAT revealed altered turnover of MGDG rather than DGTS (Iwai et al., 2021). Taken together, it seems likely that DGTS takes on some, but not all, of the roles in algae that PC has in plants. This is illustrated in Figure 9, which highlights known and potential pathways of fatty acid editing in algae.
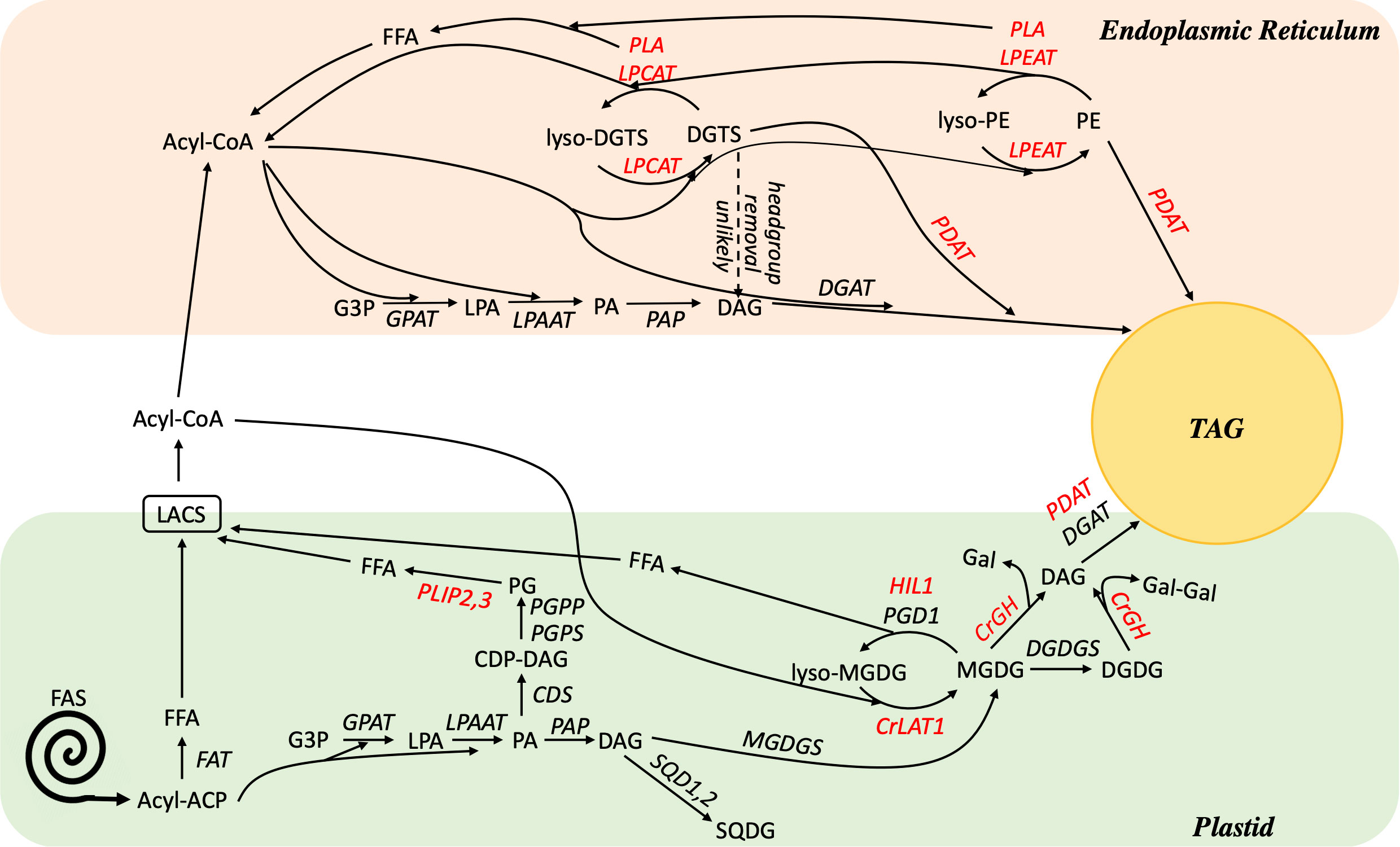
Figure 9 Working model of lipid metabolism in C. reinhardtii during TAG accumulation. Enzymes are shown in italics, for those shown in red further evidence is needed to test their role(s) and/or substrates in vivo. A dashed arrow indicates that for DGTS, headgroup removal or exchange is unlikely. Biosynthesis reactions of extraplastidial lipids DGTS, PE, and PI are omitted for simplicity. ACP, acyl carrier protein; CDP-DAG, cytidine diphosphate diacylglycerol; CDS, CDP-DAG synthase, CrGH, C. reinhardtii galactosyl hydrolase; CrLAT1, C. reinhardtii Lysolipid Acyltransferase 1; DAG, diacylglycerol; DGAT, diacylglycerol acyltransferase; DGDGS, digalactoslydiacylglycerol synthase; DGTS, diacylglyceryl-N,N,N-trimethylhomoserine; FAS, fatty acid synthase; FAT, fatty acyl-ACP thioesterase; FFA, free fatty acid; G3P, glycerol 3-phosphate; Gal, galactose; GPAT, glycerol-3-phosphate acyltransferase; HIL1, heat inducible lipase 1; LACS, long chain acyl-CoA synthetase; LPA, lysophosphatidic acid; LPAAT, lysophosphatidic acid acyltransferase; LPCAT, acyl-CoA:lysophosphatidylcholine acyltransferase; LPEAT, lysophosphatidylethanolamine acyltransferase; MGDG, monogalactosyldiacylglycerol; MGDGS, monogalactosyldiacylglycerol synthase; PA, phosphatidic acid; PAP, phosphatidic acid phosphatase; PDAT, phospholipid:diacylglycerol acyltransferase; PE, phosphatidylethanolamine; PG, phosphatidylglycerol; PGD1, Plastid Galactoglycerolipid Degradation 1; PGPP, phosphatidylglycerol phosphate phosphatase; PGPS, phosphatidylglycerolphosphate synthase; PLA, phospholipase; PLIP2, 3, plastid lipase 2, 3; SQD1, 2, sulfoquinovosyldiacylglycerol synthase; SQDG, sulfoquinovosyldiacylglycerol; TAG, triacylglycerol.
In addition to the functions described above, PC may also play a role in lipid trafficking. The eukaryotic pathway of galactolipid synthesis requires transport of some lipid precursor from the ER to the plastid. The Arabidopsis trigalactosyldiacylglycerol (TGD) transmembrane protein complex of the chloroplast has been shown to conduct ER-to-plastid lipid trafficking (Xu et al., 2003; Xu et al., 2010; Fan et al., 2015), although its transported lipid substrate remains unknown. Potential candidates for the transported lipid substrate include PC, lyso-PC, DAG, or PA, but regardless of its identity the transported lipid is believed to be derived from PC. Interestingly, recent studies suggest that the PC involved in transport to the chloroplast for galactolipid synthesis is metabolically distinct from the PC pool utilized for acyl editing (Karki et al., 2019). Intriguingly, despite its lack of PC C. reinhardtii contains an ortholog of the plant TGD transporter called CrTGD2, and isotopic labeling experiments indicated that Chlamydomonas can import lipid precursors from the ER (Warakanont et al., 2015). It is postulated that PA may be the lipid species transported from the ER to the chloroplast in C. reinhardtii. It remains to be determined what role DGTS may have in lipid trafficking in algae.
Thus, the extent to which betaine or other lipids replace the roles of PC in algae is an area ripe for future study. While radiolabeling has been instrumental in confirming some of the functions of DGTS, more detailed 13C isotopic labeling time courses should be performed in order to trace the fatty acid fluxes of DGTS in algae. The relative amount of fatty acid modification and export from DGTS into other lipids such as TAG should be estimated by 13C and/or other isotopic labeling to determine its potential contribution as an acyl hub. Prior 13C-labeling studies have demonstrated that DGTS contributes substantial fatty acid flux toward TAG synthesis (Young and Shachar-Hill, 2021), and future studies examining the turnover of individual lipid components on a finer timescale can be expected to illuminate the mechanism of DGTS’s contribution to the synthesis of TAG and other glycerolipids. In addition, we believe that more detailed analysis of algal homologs of plant enzymes known to conduct acyl flux through PC including LPCAT, PDAT, and phospholipase A2 would be valuable. Such analyses should include in vitro enzymatic assays to ascertain whether DGTS may replace PC as the preferred substrate of these enzymes. Finally, the use of [13C2 18O2]acetate labeling may shed light onto the role of DGTS and other lipids as acyl editing hubs, as 18O fatty acid labeling can help determine the number of hydrolysis reactions that have taken place, thus informing the history of a fatty acid and possible routes of its trafficking (Pollard and Ohlrogge, 1999). Given the numerous critical functions of PC in plant lipid metabolism, determining the extent to which betaine or other lipids replace the function of PC in algae is important for understanding and engineering algal lipid metabolism.
Author contributions
DH and YS-H discussed and conceived of the ideas in this paper. DH researched, wrote the article, and prepared the figures. YS-H contributed to the final form of the manuscript. All authors contributed to the article and approved the submitted version.
Funding
This work was supported by the National Institute of General Medical Sciences of the National Institutes of Health predoctoral training award from grant no. T32-GM110523 to DH. Its contents are solely the responsibility of the authors and do not necessarily represent the official views of the NIGMS or NIH. This work was also made possible by a University Distinguished Fellowship awarded to DH by the Graduate School of Michigan State University. This research was supported by the Office of Science (BER), U.S. Department of Energy, Grant no DE-SC0018269.
Conflict of interest
The authors declare that the research was conducted in the absence of any commercial or financial relationships that could be construed as a potential conflict of interest.
Publisher’s note
All claims expressed in this article are solely those of the authors and do not necessarily represent those of their affiliated organizations, or those of the publisher, the editors and the reviewers. Any product that may be evaluated in this article, or claim that may be made by its manufacturer, is not guaranteed or endorsed by the publisher.
Glossary
Abbreviations
BTA1, Betaine Lipid Synthase 1; CPT, CDP-choline:1,2-Diacylglycerol Cholinephosphotransferase; CrLAT1, C. reinhardtii Lysolipid Acyltransferase 1; DAG, Diacylglycerol; DGAT, Diacylglycerol Acyltransferase; DGDG, Digalactosyldiacylglycerol; DGTA, Diacylglycerylhydroxymethyltrimethyl-β-Alanine; DGTS, Diacylglyceryl-N,N,N-trimethylhomoserine; ER, Endoplasmic Reticulum; FA, Fatty Acid; FFA, Free Fatty Acid; FAD, Fatty Acid Desaturase; G3P, Glycerol 3-Phosphate; GPAT, Glycerol-3-Phosphate Acyltransferase; GPC, Glycerolphosphocholine; GPCAT, Glycerolphosphocholine Acyltransferase; LC-MS, Liquid Chromatography Mass Spectrometry; LPAAT, Lysophosphatidic Acid Acyltransferase; LPCAT, Lysophosphatidylcholine Acyltransferase; LPCT, Lysophosphatidylcholine Transacylase; LPEAT, Lysophosphatidylethanolamine Acyltransferase; LPLAT, Lysophospholipid Acyltransferase; MGDG, Monogalactosyldiacylglycerol; PA, Phosphatidic Acid; PC, Phosphatidylcholine; PDAT, Phospholipid : Diacylglycerol Acyltransferase; PDCT, Phosphatidylcholine : Diacylglycerol Cholinephosphotransferase; PE, Phosphatidylethanolamine; PG, Phosphatidylglycerol; PGD1, Plastid Galactoglycerolipid Degradation 1; PLIP1, Plastid Lipase 1; PUFA, Polyunsaturated Fatty Acid; SQDG, Sulfoquinovosyldiacylglycerol; TAG, Triacylglycerol; TGD, Trigalactosyldiacylglycerol.
References
Abida, H., Dolch, L. J., Meï, C., Villanova, V., Conte, M., Block, M. A., et al. (2015). Membrane glycerolipid remodeling triggered by nitrogen and phosphorus starvation in phaeodactylum tricornutum. Plant Physiol. 167, 118–136. doi: 10.1104/pp.114.252395
Arondel, V., Lemieux, B., Hwang, I., Gibson, S., Goodman, H. M., Somerville, C. R. (1992). Map-based cloning of a gene controlling omega-3 fatty acid desaturation in Arabidopsis. Science 258, 1353–1355. doi: 10.1126/science.1455229
Bafor, M., Smith, M. A., Jonsson, L., Stobart, K., Stymne, S. (1991). Ricinoleic acid biosynthesis and triacylglycerol assembly in microsomal preparations from developing castor-bean (Ricinus communis) endosperm. Biochem. J. 280, 507–514. doi: 10.1042/bj2800507
Banas, A., Bafor, M., Wiberg, E., Lenman, M., Ståhl, U., Stymne, S. (1997). “Biosynthesis of an acetylenic fatty acid in microsomal preparations from developing seeds of crepis alpina,” in Physiology, biochemistry and molecular biology of plant lipids (Dordrecht: Springer), 57–59.
Bates, P. D., Browse, J. (2011). The pathway of triacylglycerol synthesis through phosphatidylcholine in arabidopsis produces a bottleneck for the accumulation of unusual fatty acids in transgenic seeds. Plant J. 68, 387–399. doi: 10.1111/j.1365-313X.2011.04693.x
Bates, P. D., Durrett, T. P., Ohlrogge, J. B., Pollard, M. (2009). Analysis of acyl fluxes through multiple pathways of triacylglycerol synthesis in developing soybean embryos. Plant Physiol. 150, 55–72. doi: 10.1104/pp.109.137737
Bates, P. D., Fatihi, A., Snapp, A. R., Carlsson, A. S., Browse, J., Lu, C. (2012). Acyl editing and headgroup exchange are the major mechanisms that direct polyunsaturated fatty acid flux into triacylglycerols. Plant Physiol. 160, 1530–1539. doi: 10.1104/pp.112.204438
Bates, P. D., Ohlrogge, J. B., Pollard, M. (2007). Incorporation of newly synthesized fatty acids into cytosolic glycerolipids in pea leaves occurs via acyl editing. J. Biol. Chem. 282, 31206–31216. doi: 10.1074/jbc.M705447200
Boyle, N. R., Page, M. D., Liu, B., Blaby, I. K., Casero, D., Kropat, J., et al. (2012). Three acyltransferases and nitrogen-responsive regulator are implicated in nitrogen starvation-induced triacylglycerol accumulation in chlamydomonas. J. Biol. Chem. 287, 15811–15825. doi: 10.1074/jbc.M111.334052
Broun, P., Somerville, C. (1997). Accumulation of ricinoleic, lesquerolic, and densipolic acids in seeds of transgenic Arabidopsis plants that express a fatty acyl hydroxylase cDNA from castor bean. Plant Physiol. 113, 933–942. doi: 10.1104/pp.113.3.933
Browse, J., McConn, M., James, D., Miquel, M. (1993). Mutants of Arabidopsis deficient in the synthesis of alpha-linolenate. biochemical and genetic characterization of the endoplasmic reticulum linoleoyl desaturase. J. Biol. Chem. 268, 16345–16351. doi: 10.1016/S0021-9258(19)85427-3
Browse, J., Somerville, C. (1991). Glycerolipid synthesis: biochemistry and regulation. Annu. Rev. Plant Biol. 42, 467–506. doi: 10.1146/annurev.pp.42.060191.002343
Cahoon, E. B., Ohlrogge, J. B. (1994). Apparent role of phosphatidylcholine in the metabolism of petroselinic acid in developing umbelliferae endosperm. Plant Physiol. 104, 845–855. doi: 10.1104/pp.104.3.845
Chen, G., Woodfield, H. K., Pan, X., Harwood, J. L., Weselake, R. J. (2015). Acyl-trafficking during plant oil accumulation. Lipids 50, 1057–1068. doi: 10.1007/s11745-015-4069-x
Dahlqvist, A., Ståhl, U., Lenman, M., Banas, A., Lee, M., Sandager, L., et al. (2000). Phospholipid: diacylglycerol acyltransferase: an enzyme that catalyzes the acyl-CoA-independent formation of triacylglycerol in yeast and plants. Proc. Natl. Acad. Sci. U.S.A. 97, 6487–6492. doi: 10.1073/pnas.120067297
Dembitsky, V. M. (1996). Betaine ether-linked glycerolipids: chemistry and biology. Prog. Lipid Res. 35, 1–51. doi: 10.1016/0163-7827(95)00009-7
Du, Z. Y., Benning, C. (2016). Triacylglycerol accumulation in photosynthetic cells in plants and algae. Subcell. Biochem. 86, 179–205. doi: 10.1007/978-3-319-25979-6_8
Du, Z. Y., Lucker, B. F., Zienkiewicz, K., Miller, T. E., Zienkiewicz, A., Sears, B. B., et al. (2018). Galactoglycerolipid lipase PGD1 is involved in thylakoid membrane remodeling in response to adverse environmental conditions in chlamydomonas. Plant Cell. 30, 447–465. doi: 10.1105/tpc.17.00446
Falarz, L. J., Xu, Y., Caldo, K. M. P., Garroway, C. J., Singer, S. D., Chen, G. (2020). Characterization of the diversification of phospholipid: diacylglycerol acyltransferases in the green lineage. Plant J. 103, 2025–2038. doi: 10.1111/tpj.14880
Fan, J., Andre, C., Xu, C. (2011). A chloroplast pathway for the de novo biosynthesis of triacylglycerol in chlamydomonas reinhardtii. FEBS Lett. 585, 1985–1991. doi: 10.1016/j.febslet.2011.05.018
Fan, J., Zhai, Z., Yan, C., Xu, C. (2015). Arabidopsis TRIGALACTOSYLDIACYLGLYCEROL5 interacts with TGD1, TGD2, and TGD4 to facilitate lipid transfer from the endoplasmic reticulum to plastids. Plant Cell. 27, 2941–2955. doi: 10.1105/tpc.15.00394
Geiger, O., Röhrs, V., Weissenmayer, B., Finan, T. M., Thomas-Oates, J. E. (1999). The regulator gene phoB mediates phosphate stress-controlled synthesis of the membrane lipid diacylglyceryl-n, n, n-trimethylhomoserine in rhizobium (Sinorhizobium) meliloti. Mol. Microbiol. 32, 63–73. doi: 10.1046/j.1365-2958.1999.01325.x
Giroud, C., Eichenberger, W. (1989). Lipids of chlamydomonas reinhardtii. incorporation of [14C] acetate,[14C] palmitate and [14C] oleate into different lipids and evidence for lipid-linked desaturation of fatty acids. Plant Cell Physiol. 30, 121–128. doi: 10.1093/oxfordjournals.pcp.a077705
Giroud, C., Gerber, A., Eichenberger, W. (1988). Lipids of chlamydomonas reinhardtii. analysis of molecular species and intracellular site (s) of biosynthesis. Plant Cell Physiol. 29, 587–595. doi: 10.1093/oxfordjournals.pcp.a077533
Głąb, B., Beganovic, M., Anaokar, S., Hao, M. S., Rasmusson, A. G., Patton-Vogt, J., et al. (2016). Cloning of glycerophosphocholine acyltransferase (GPCAT) from fungi and plants: a novel enzyme in phosphatidylcholine synthesis. J. Biol. Chem. 291, 25066–25076. doi: 10.1074/jbc.M116.743062
Goncalves, E. C., Johnson, J. V., Rathinasabapathi, B. (2013). Conversion of membrane lipid acyl groups to triacylglycerol and formation of lipid bodies upon nitrogen starvation in biofuel green algae chlorella UTEX29. Planta 238, 895–906. doi: 10.1007/s00425-013-1946-5
Goncalves, E. C., Wilkie, A. C., Kirst, M., Rathinasabapathi, B. (2016). Metabolic regulation of triacylglycerol accumulation in the green algae: identification of potential targets for engineering to improve oil yield. Plant Biotechnol. J. 14, 1649–1660. doi: 10.1111/pbi.12523
Gu, X., Cao, L., Wu, X., Li, Y., Hu, Q., Han, D. (2021). A lipid bodies-associated galactosyl hydrolase is involved in triacylglycerol biosynthesis and galactolipid turnover in the unicellular green alga chlamydomonas reinhardtii. Plants 10, 675. doi: 10.3390/plants10040675
Higashi, Y., Okazaki, Y., Takano, K., Myouga, F., Shinozaki, K., Knoch, E., et al. (2018). HEAT INDUCIBLE LIPASE1 remodels chloroplastic monogalactosyldiacylglycerol by liberating α-linolenic acid in arabidopsis leaves under heat stress. Plant Cell. 30, 1887–1905. doi: 10.1105/tpc.18.00347
Hurlock, A. K., Wang, K., Takeuchi, T., Horn, P. J., Benning, C. (2018). In vivo lipid ‘tag and track’approach shows acyl editing of plastid lipids and chloroplast import of phosphatidylglycerol precursors in Arabidopsis thaliana. Plant J. 95, 1129–1139. doi: 10.1111/tpj.13999
Iwai, M., Yamada-Oshima, Y., Asami, K., Kanamori, T., Yuasa, H., Shimojima, M., et al. (2021). Recycling of the major thylakoid lipid MGDG and its role in lipid homeostasis in chlamydomonas reinhardtii. Plant Physiol. 187, 1341–1356. doi: 10.1093/plphys/kiab340
Jasieniecka-Gazarkiewicz, K., Demski, K., Lager, I., Stymne, S., Banaś, A. (2016). Possible role of different yeast and plant lysophospholipid: acyl-CoA acyltransferases (LPLATs) in acyl remodelling of phospholipids. Lipids 51, 15–23. doi: 10.1007/s11745-015-4102-0
Jones, A. L., Harwood, J. L. (1993). Lipid metabolism in the brown marine algae fucus vesiculosus and ascophyllum nodosum. J. Exp. Bot. 44, 1203–1210. doi: 10.1093/jxb/44.7.1203
Karki, N., Johnson, B. S., Bates, P. D. (2019). Metabolically distinct pools of phosphatidylcholine are involved in trafficking of fatty acids out of and into the chloroplast for membrane production. Plant Cell. 31, 2768–2788. doi: 10.1105/tpc.19.00121
Kato, M., Sakai, M., Adachi, K., Ikemoto, H., Sano, H. (1996). Distribution of betaine lipids in marine algae. Phytochemistry 42, 1341–1345. doi: 10.1016/0031-9422(96)00115-X
Klińska, S., Jasieniecka-Gazarkiewicz, K., Demski, K., Banaś, A. (2020). Editing of phosphatidic acid and phosphatidylethanolamine by acyl-CoA: Lysophospholipid acyltransferases in developing camelina sativa seeds. Planta 252, 1–17. doi: 10.1007/s00425-020-03408-z
Klińska, S., Kędzierska, S., Jasieniecka-Gazarkiewicz, K., Banaś, A. (2021). In vitro growth conditions boost plant lipid remodelling and influence their composition. Cells 10, 2326. doi: 10.3390/cells10092326
Klug, R. M., Benning, C. (2001). Two enzymes of diacylglyceryl-O-4′-(N, n, n,-trimethyl) homoserine biosynthesis are encoded by btaA and btaB in the purple bacterium rhodobacter sphaeroides. Proc. Natl. Acad. Sci. U.S.A. 98, 5910–5915. doi: 10.1073/pnas.101037998
Künzler, K., Eichenberger, W. (1997). Betaine lipids and zwitterionic phospholipids in plants and fungi. Phytochemistry 46, 883–892. doi: 10.1016/S0031-9422(97)81274-5
Lager, I., Glab, B., Eriksson, L., Chen, G., Banas, A., Stymne, S. (2015). Novel reactions in acyl editing of phosphatidylcholine by lysophosphatidylcholine transacylase (LPCT) and acyl-CoA: glycerophosphocholine acyltransferase (GPCAT) activities in microsomal preparations of plant tissues. Planta 241, 347–358. doi: 10.1007/s00425-014-2184-1
Lager, I., Yilmaz, J. L., Zhou, X. R., Jasieniecka, K., Kazachkov, M., Wang, P., et al. (2013). Plant acyl-CoA: lysophosphatidylcholine acyltransferases (LPCATs) have different specificities in their forward and reverse reactions. J. Biol. Chem. 288, 36902–36914. doi: 10.1074/jbc.M113.521815
Lands, W. E. (1958). Metabolism of glycerolipides: a comparison of lecithin and triglyceride synthesis. J. Biol. Chem. 231, 883–888. doi: 10.1016/S0021-9258(18)70453-5
Lands, W. E. (1960). Metabolism of glycerolipids: II. the enzymatic acylation of lysolecithin. J. Biol. Chem. 235, 2233–2237. doi: 10.1016/S0021-9258(18)64604-6
Lee, M., Lenman, M., Banaś, A., Bafor, M., Singh, S., Schweizer, M., et al. (1998). Identification of non-heme diiron proteins that catalyze triple bond and epoxy group formation. Science 280, 915–918. doi: 10.1126/science.280.5365.915
Lee, Y. Y., Park, R., Miller, S. M., Li, Y. (2022). Genetic compensation of triacylglycerol biosynthesis in the green microalga chlamydomonas reinhardtii. Plant J. 111, 1069–1080. doi: 10.1111/tpj.15874
Lee, J. W., Shin, S. Y., Kim, H. S., Jin, E., Lee, H. G., Oh, H. M. (2017). Lipid turnover between membrane lipids and neutral lipids via inhibition of diacylglyceryl n, n, n-trimethylhomoserine synthesis in Chlamydomonas reinhardtii. Algal Res. 27, 162–169. doi: 10.1016/j.algal.2017.09.001
Légeret, B., Schulz-Raffelt, M., Nguyen, H. M., Auroy, P., Beisson, F., Peltier, G., et al. (2016). Lipidomic and transcriptomic analyses of chlamydomonas reinhardtii under heat stress unveil a direct route for the conversion of membrane lipids into storage lipids. Plant Cell Environ. 39, 834–847. doi: 10.1111/pce.12656
Li, X., Moellering, E. R., Liu, B., Johnny, C., Fedewa, M., Sears, B. B., et al. (2012). A galactoglycerolipid lipase is required for triacylglycerol accumulation and survival following nitrogen deprivation in chlamydomonas reinhardtii. Plant Cell. 24, 4670–4686. doi: 10.1105/tpc.112.105106
Lu, C., Xin, Z., Ren, Z., Miquel, M. (2009). An enzyme regulating triacylglycerol composition is encoded by the ROD1 gene of Arabidopsis. Proc. Natl. Acad. Sci. U.S.A. 106, 18837–18842. doi: 10.1073/pnas.0908848106
Meng, Y., Cao, X., Yang, M., Liu, J., Yao, C., Xue, S. (2019). Glycerolipid remodeling triggered by phosphorous starvation and recovery in nannochloropsis oceanica. Algal Res. 39, 101451. doi: 10.1016/j.algal.2019.101451
Merchant, S. S., Kropat, J., Liu, B., Shaw, J., Warakanont, J. (2012). TAG, you’re it! chlamydomonas as a reference organism for understanding algal triacylglycerol accumulation. Curr. Opin. Biotechnol. 23, 352–363. doi: 10.1016/j.copbio.2011.12.001
Mhaske, V., Beldjilali, K., Ohlrogge, J., Pollard, M. (2005). Isolation and characterization of an arabidopsis thaliana knockout line for phospholipid: diacylglycerol transacylase gene (At5g13640). Plant Physiol. Biochem. 43, 413–417. doi: 10.1016/j.plaphy.2005.01.013
Miquel, M., Browse, J. (1992). Arabidopsis mutants deficient in polyunsaturated fatty acid synthesis. biochemical and genetic characterization of a plant oleoyl-phosphatidylcholine desaturase. J. Biol. Chem. 267, 1502–1509. doi: 10.1016/S0021-9258(18)45974-1
Moellering, E. R., Benning, C. (2010). RNA Interference silencing of a major lipid droplet protein affects lipid droplet size in chlamydomonas reinhardtii. Eukaryot. Cell. 9, 97–106. doi: 10.1128/EC.00203-09
Murakami, H., Nobusawa, T., Hori, K., Shimojima, M., Ohta, H. (2018). Betaine lipid is crucial for adapting to low temperature and phosphate deficiency in nannochloropsis. Plant Physiol. 177, 181–193. doi: 10.1104/pp.17.01573
Oishi, Y., Otaki, R., Iijima, Y., Kumagai, E., Aoki, M., Tsuzuki, M., et al. (2022). Diacylglyceryl-n, n, n-trimethylhomoserine-dependent lipid remodeling in a green alga, chlorella kessleri. Commun. Biol. 5, 1–13. doi: 10.1038/s42003-021-02927-z
Okuley, J., Lightner, J., Feldmann, K., Yadav, N., Lark, E., Browse, J. (1994). Arabidopsis FAD2 gene encodes the enzyme that is essential for polyunsaturated lipid synthesis. Plant Cell. 6, 147–158. doi: 10.1105/tpc.6.1.147
Pollard, M., Martin, T. M., Shachar-Hill, Y. (2015). Lipid analysis of developing camelina sativa seeds and cultured embryos. Phytochemistry 118, 23–32. doi: 10.1016/j.phytochem.2015.07.022
Pollard, M., Ohlrogge, J. (1999). Testing models of fatty acid transfer and lipid synthesis in spinach leaf using in vivo oxygen-18 labeling. Plant Physiol. 121, 1217–1226. doi: 10.1104/pp.121.4.1217
Popko, J., Herrfurth, C., Feussner, K., Ischebeck, T., Iven, T., Haslam, R., et al. (2016). Metabolome analysis reveals betaine lipids as major source for triglyceride formation, and the accumulation of sedoheptulose during nitrogen-starvation of phaeodactylum tricornutum. PloS One 11, e0164673. doi: 10.1371/journal.pone.0164673
Schlapfer, P., Eichenberger, W. (1983). Evidence for the involvement of diacylglyceryl (N, n, n,-trimethy homoserine in the desaturation of oleic and linoleic acids in Chlamydomonas reinhardi (chlorophyceae. Plant Sci. Lett. 32, 243–252. doi: 10.1016/0304-4211(83)90121-9
Schultz, D. J., Ohlrogge, J. B. (2000). Biosynthesis of triacylglycerol in thunbergia alata: additional evidence for involvement of phosphatidylcholine in unusual monoenoic oil production. Plant Physiol. Biochem. 38, 169–175. doi: 10.1016/S0981-9428(00)00739-7
Slack, C. R., Campbell, L. C., Browse, J. A., Roughan, P. G. (1983). Some evidence for the reversibility of the cholinephosphotransferasecatalysed reaction in developing linseed cotyledons in vivo. Biochim. Biophys. Acta Lipids Lipid Metab. 754, 10–20. doi: 10.1016/0005-2760(83)90076-0
Sperling, P., Heinz, E. (1993). Isomeric sn-1-octadecenyl and sn-2-octadecenyl analogues of lysophosphatidylcholine as substrates for acylation and desaturation by plant microsomal membranes. Eur. J. Biochem. 213, 965–971. doi: 10.1111/j.1432-1033.1993.tb17841.x
Sperling, P., Linscheid, M., Stöcker, S., Mühlbach, H. P., Heinz, E. (1993). In vivo desaturation of cis-delta 9-monounsaturated to cis-delta 9, 12-diunsaturated alkenylether glycerolipids. J. Biol. Chem. 268, 26935–26940. doi: 10.1016/S0021-9258(19)74200-8
Stahl, U., Banas, A., Stymne, S. (1995). Plant microsomal phospholipid acyl hydrolases have selectivities for uncommon fatty acids. Plant Physiol. 107, 953–962. doi: 10.1104/pp.107.3.953
Stahl, U., Carlsson, A. S., Lenman, M., Dahlqvist, A., Huang, B., Banasí, W., et al. (2004). Cloning and functional characterization of a phospholipid: diacylglycerol acyltransferase from arabidopsis. Plant Physiol. 135, 1324–1335. doi: 10.1104/pp.104.044354
Stobart, A. K., Stymne, S. (1985). The interconversion of diacylglycerol and phosphatidylcholine during triacylglycerol production in microsomal preparations of developing cotyledons of safflower (Carthamus tinctorius l.). Biochem. J. 232, 217–221. doi: 10.1042/bj2320217
Stymne, S., Stobart, A. K. (1984). Evidence for the reversibility of the acyl-CoA: lysophosphatidylcholine acyltransferase in microsomal preparations from developing safflower (Carthamus tinctorius l.) cotyledons and rat liver. Biochem. J. 223, 305–314. doi: 10.1042/bj2230305
Tatsuzawa, H., Takizawa, E. (1995). Changes in lipid and fatty acid composition of pavlova lutheri. Phytochemistry 40, 397–400. doi: 10.1016/0031-9422(95)00327-4
Van De Loo, F. J., Broun, P., Turner, S., Somerville, C. (1995). An oleate 12-hydroxylase from ricinus communis l. is a fatty acyl desaturase homolog. Proc. Natl. Acad. Sci. U.S.A. 92, 6743–6747. doi: 10.1073/pnas.92.15.6743
Vilchez, A. C., Margutti, M. P., Reyna, M., Wilke, N., Villasuso, A. L. (2021). Recovery from chilling modulates the acyl-editing of phosphatidic acid molecular species in barley roots (Hordeum vulgare l.). Plant Physiol. Biochem. 167, 862–873. doi: 10.1016/j.plaphy.2021.09.005
Vogel, G., Eichenberger, W. (1992). Betaine lipids in lower plants. biosynthesis of DGTS and DGTA in ochromonas danica (Chrysophyceae) and the possible role of DGTS in lipid metabolism. Plant Cell Physiol. 33, 427–436. doi: 10.1093/oxfordjournals.pcp.a078271
Wang, K., Froehlich, J. E., Zienkiewicz, A., Hersh, H. L., Benning, C. (2017). A plastid phosphatidylglycerol lipase contributes to the export of acyl groups from plastids for seed oil biosynthesis. Plant Cell. 29, 1678–1696. doi: 10.1105/tpc.17.00397
Wang, L., Shen, W., Kazachkov, M., Chen, G., Chen, Q., Carlsson, A. S., et al. (2012). Metabolic interactions between the lands cycle and the Kennedy pathway of glycerolipid synthesis in Arabidopsis developing seeds. Plant Cell. 24, 4652–4669. doi: 10.1105/tpc.112.104604
Warakanont, J., Tsai, C. H., Michel, E. J., Murphy, G. R., III, Hsueh, P. Y., Roston, R. L., et al. (2015). Chloroplast lipid transfer processes in chlamydomonas reinhardtii involving a TRIGALACTOSYLDIACYLGLYCEROL 2 (TGD 2) orthologue. Plant J. 84, 1005–1020. doi: 10.1111/tpj.13060
Xu, C., Andre, C., Fan, J., Shanklin, J. (2016). Cellular organization of triacylglycerol biosynthesis in microalgae. Subcell. Biochem. 86, 207–221. doi: 10.1007/978-3-319-25979-6_9
Xu, C., Fan, J., Riekhof, W., Froehlich, J. E., Benning, C. (2003). A permease-like protein involved in ER to thylakoid lipid transfer in arabidopsis. EMBO J. 22, 2370–2379. doi: 10.1093/emboj/cdg234
Xu, C., Moellering, E. R., Muthan, B., Fan, J., Benning, C. (2010). Lipid transport mediated by arabidopsis TGD proteins is unidirectional from the endoplasmic reticulum to the plastid. Plant Cell Physiol. 51, 1019–1028. doi: 10.1093/pcp/pcq053
Yang, M., Kong, F., Xie, X., Wu, P., Chu, Y., Cao, X., et al. (2020). Galactolipid DGDG and betaine lipid DGTS direct De novo synthesized linolenate into triacylglycerol in a stress-induced starchless mutant of Chlamydomonas reinhardtii. Plant Cell Physiol. 61, 851–862. doi: 10.1093/pcp/pcaa012
Yang, M., Meng, Y., Chu, Y., Fan, Y., Cao, X., Xue, S., et al. (2018). Triacylglycerol accumulates exclusively outside the chloroplast in short-term nitrogen-deprived Chlamydomonas reinhardtii. Biochim. Biophys. Acta Mol. Cell Biol. Lipids. 1863, 1478–1487. doi: 10.1016/j.bbalip.2018.09.009
Yoon, K., Han, D., Li, Y., Sommerfeld, M., Hu, Q. (2012). Phospholipid: diacylglycerol acyltransferase is a multifunctional enzyme involved in membrane lipid turnover and degradation while synthesizing triacylglycerol in the unicellular green microalga chlamydomonas reinhardtii. Plant Cell. 24, 3708–3724. doi: 10.1105/tpc.112.100701
Young, D. Y., Pang, N., Shachar-Hill, Y. (2022). 13C-labeling reveals how membrane lipid components contribute to triacylglycerol accumulation in chlamydomonas. Plant Physiol. 189, 1326–1344. doi: 10.1093/plphys/kiac154
Keywords: microalgae, lipid, acyl editing, phosphatidylcholine, triacylglyceride (TAG)
Citation: Hoffmann DY and Shachar-Hill Y (2023) Do betaine lipids replace phosphatidylcholine as fatty acid editing hubs in microalgae? Front. Plant Sci. 14:1077347. doi: 10.3389/fpls.2023.1077347
Received: 22 October 2022; Accepted: 04 January 2023;
Published: 19 January 2023.
Edited by:
Ben Lucker, Prosel Biosciences, United StatesReviewed by:
Agnieszka Zienkiewicz, Nicolaus Copernicus University in Toruń, PolandJuliette Jouhet, UMR5168 Laboratoire de Physiologie Cellulaire Vegetale (LPCV), France
Copyright © 2023 Hoffmann and Shachar-Hill. This is an open-access article distributed under the terms of the Creative Commons Attribution License (CC BY). The use, distribution or reproduction in other forums is permitted, provided the original author(s) and the copyright owner(s) are credited and that the original publication in this journal is cited, in accordance with accepted academic practice. No use, distribution or reproduction is permitted which does not comply with these terms.
*Correspondence: Danielle Yvonne Hoffmann, eW91bmdkMzRAbXN1LmVkdQ==
†ORCID: Danielle Yvonne Hoffmann, orcid.org/0000-0002-1881-7380
Yair Shachar-Hill, orcid.org/0000-0001-8793-5084