- 1College of Agronomy, Northwest A&F University, Xianyang, China
- 2Academy of Agricultural Sciences, Key Laboratory of Agro-Ecological Protection & Exploitation and Utilization of Animal and Plant Resources in Eastern Inner Mongolia, Chifeng University, Chifeng, China
Drought and salt stress can strongly affect the growth and development of wheat. Wheat adapts to drought and salt stress through osmotic regulation. Betaine aldehyde dehydrogenase (BADH) is a key enzyme in the synthesis of betaine, an osmotic regulator. We cloned a region of the TaBADH-A1 promoter and genomic DNA that included the introns and exons, from four Chinese wheat cultivars. Following the analysis of TaBADH-A1 genomic DNA and promoter sequence polymorphisms of 4 cloned and 15 cultivars from the database, 7 haplotypes of TaBADH-A1 gene were identified. We divided the 7 haplotypes with a 254 bp insertion or deletion (indel) into two main alleles, BADH-A1a and BADH-A1b. Meanwhile, a molecular marker was developed based on the 254 bp indel of the third intron of TaBADH-A1 gene. Expression levels of BADH-A1b were found to be significantly higher than those of BADH-A1a under drought and salt stress conditions. Betaine accumulation was significantly higher in wheat containing BADH-A1b compared to BADH-A1a under drought and salt stress. We also identified that the average relative germination and survival rates of wheat with the BADH-A1b allele were significantly higher than wheat with the BADH-A1a allele. The results reveal that wheat containing BADH-A1b has stronger drought and salt tolerance than wheat with BADH-A1a. Meanwhile, the geographic distribution and frequency of the TaBADH-A1 locus alleles indicate that BADH-A1a has been preferred in Chinese wheat breeding programs, while BADH-A1b, associated with favorable stress tolerance, has been neglected. The results of this study provide evidence for an excellent candidate allele for marker-assisted selection of new wheat cultivars with increased salt tolerance and drought resistance.
Introduction
It is well known that wheat is one of the most important and widely cultivated cereals, supporting the daily caloric and protein requirements of 60% of the world’s population (Tadesse et al., 2017). Drought has become a serious environmental problem due to global climate change, and a reduction of water by up to 40% causes a reduction in wheat yield of up to 21% (Younis et al., 2020). At the same time, salinization is also an important factor in reduced crop productivity. Salt issues affect about 20% of the total global land area and almost 40% of irrigated lands; this phenomenon shows an increasing trend, and 50% of total cultivated land worldwide is projected to be salinized by 2050 (Urbanavičiūtė et al., 2021). Among all abiotic stresses, stress due to drought and salt is the main challenge for plant growth and development, causing great damage to food production worldwide (Ma et al., 2020). Therefore, improving the drought and salt tolerance is an urgent problem to be solved in wheat breeding. One effective way to improve drought and salt resistance in wheat is to explore and utilize excellent alleles of genes related to drought resistance and salt tolerance.
Both drought and salt stress trigger osmotic regulation in plants, which is the main component of the physiological mechanism determining the drought resistance and salt tolerance of plants (Khoshro et al., 2013; Zhu, 2016). Under drought and salt stress, there is an increased accumulation of substances involved in osmotic regulation, such as betaine, proline, soluble sugar, and inorganic ions, which decreases the osmotic potential and increases the osmotic pressure of cells, resulting in buffering of the redox potential and the maintenance of protein structure, and photosynthetic organs are protected from stress damage (Gadallah, 1999; Mäkelä et al., 2000; Cha-Um and Kirdmanee, 2010; Wang et al., 2018). Betaine, an important osmotic regulatory substance, also has multiple functions in reducing cell osmotic potential and increasing cell osmotic pressure to protect plants from abiotic stress (Zhang et al., 2020). Betaine aldehyde dehydrogenase (BADH) is an important enzyme that catalyzes the final step of betaine production (Fujiwara et al., 2008). The BADH gene was first cloned in Spinacia oleracea (Weretilnyk and Hanson, 1990) and has, to date been isolated in a large number of plants, such as Sesuvium portulacastrum, Amaranthus hypochondriacus L., Arabidopsis thaliana, and Triticum aestivum L. (Legaria et al., 1998; Missihoun et al., 2011; Yang et al., 2015; Sun et al., 2019). Previous studies have shown that BADH overexpression in plants can increase their tolerance to various abiotic stresses (Fan et al., 2012; Yang et al., 2015; Sun et al., 2019). For example, overexpression of SoBADH (S. oleracea) in transgenic sweet potato improved tolerance to salt and oxidative stress and low temperature by increasing betaine accumulation (Fan et al., 2012). Overexpression of SpBADH (S. portulacastrum) in Arabidopsis resulted in higher betaine content and increased tolerance to drought/osmotic stress (Yang et al., 2015). Overexpression of TaBADH (T. aestivum L.) in Arabidopsis was found to enhance betaine accumulation and salt tolerance (Sun et al., 2019). The betaine content and TaBADH expression level of different wheat cultivars were found to be significantly different under abiotic stresses such as drought and salt (Jing et al., 1999; Zhao et al., 2001; Li et al., 2005). We speculate that there may be differences in the transcriptional regulatory sequences of the TaBADH that lead to the differing betaine contents of the various wheat cultivars.
In the process of evolution, domestication, and breeding, wheat has undergone two doublings, via crossing, in reaching its present polyploid form. Since the A genome is the basic genome of wheat evolution, it plays a central role in the evolution of polyploid wheat, contributing to its rich genetic diversity. In this study, we analyzed the TaBADH-A1 gene sequence polymorphisms in 19 wheat cultivars, evaluated the drought resistance and salt tolerance of TaBADH-A1 locus alleles, and explored the molecular and physiological mechanisms, and this information was used in the development of a functional molecular marker, 6 AM. We also explored the correlation between TaBADH-A1 locus alleles and agronomic traits, the distribution of alleles in Chinese wheat zones, and the occurrence frequency of TaBADH-A1 locus alleles in wheat breeding years. As a result of our study, we present an excellent candidate allele for the molecular breeding of wheat with improved drought and salt tolerance.
Materials and methods
Plant materials
Four Chinese wheat cultivars (Taishan 1, Zhongmai 9, Bima 4, and Lantian 23) with different levels of adaptability were selected for cloning TaBADH-A1. Taishan 1 has the excellent characteristic of strong resistance to stress; Zhongmai 9 withstood the rare occurrence of freezing damage in 1993–1994 and exhibits strong resistance to abiotic stresses; Bima 4 is one of the backbone parents of wheat in China with a large cultivation area, and it is planted in regions with sufficient water and fertilizer; and Lantian 23 is a high-yielding cultivar adapted to abundant water and fertile conditions. A set of nulli-tetrasomic lines of Chinese Spring (N6AT6B, N6BT6D, and N6DT6A) was used as PCR template, agarose gel electrophoresis results as a reference for the chromosome localization of primers. A total of 121 wheat cultivars (lines; population 1) in different ecological regions covering 10 wheat-producing areas in China were selected for the identification of drought resistance at the germination stage to evaluate the drought resistance of different alleles. Using Chinese Spring and Yanfu 188 as parents, a population of F6 recombinant inbred lines (population 2) was constructed to verify the drought and salt resistance of TaBADH-A1 locus different alleles. Similarly, another population of F6 recombinant inbred lines (population 3) was created using Chinese Spring and Ning 9940; two F6 populations were obtained using the single-seed descent approach. The relationship between TaBADH-A1 locus alleles and agronomic traits was validated in both populations. A wheat mini-core collection (population 4, 262 cultivars (lines; landraces), provided by Chenyang Hao at the Chinese Academy of Agricultural Sciences), which is characterized by wide geographic distribution, was used for geographic distribution analysis of the TaBADH-A1 locus alleles. In addition, 121 cultivars (lines; population 1) also were selected to analyze the geographic distribution and frequency of TaBADH-A1 locus alleles in breeding years.
DNA extraction, TaBADH-A1 gene cloning, and sequence analysis
Genomic DNA of the 4 wheat cultivars (Taishan1, Bima 4, Zhongmai 9, and Lantian 23) and 4 populations was extracted using the CTAB method (Stein et al., 2010). TaBADH-A1-specific primers were designed (Supplementary Table S1) according to the assembled TaBADH-A1 sequences (TraesCS6A02G371100) using Oligo 7.0 software. Subsequently, a region of the promoter and genomic DNA sequence that includes the introns and exons of TaBADH-A1 was cloned from each of the 4 cultivars. The PCR system details are as follows: 50 μl reactions contained 2 μl each of forward and reverse primers (10 μM) and 2 μl DNA (300 ng·μL−1), 25 μl of 2 × Phanta Max Buffer, 1 μl dNTP Mix, 1 μl Phanta Max Super-Fidelity DNA Polymerase, and 17 μl ddH2O. The PCR program comprised a cycle of 95°C for 3 min followed by 34 cycles of 95°C for 15 s, 62°C for 15 s, and 72°C for 8 min, followed by 95°C for 15 s, 62°C for 15 s, and 72°C for 8 min, and finally 72°C for 5 min (Phanta Max Super-Fidelity DNA Polymerase, Vazyme).
Sequences for the TaBADH-A1 promoter and genomic region including introns and exon of 15 wheat cultivars (ArinaLrFor, Cadenza, Claire, Jagger, Julius, Lancer, Paragon, SY Mattis, Robigus, Weebill, Stanley, Landmark, Norin 61, Mace, and Chinese Spring) were downloaded from the Ensembl Plants database1. The TaBADH-A1 gene sequence polymorphisms were analyzed in Taishan 1, Bima 4, Zhongmai 9, Lantian 23, and the 15 wheat cultivars. To analyze the TaBADH-A1 sequences of genomic (include intron and exon) and promoter regions, the Giri2 and PlantCare3 databases were used for the prediction of transposons and cis-acting elements. DNAMAN 9.0 software was used for sequence alignment.
Development of a molecular marker
After analyzing the TaBADH-A1 gene sequence polymorphism in Taishan 1, Bima 4, Zhongmai 9, Lantian 23, and 15 wheat cultivars based on sequences from the database, the molecular marker 6 AM was developed based on the insertion/deletion (indel) of 254 bp in the third intron region. Primers for the molecular marker 6 AM were designed as 5′-CGCAAGTCAATGGGCTGTGTAT-3′ and 5′-CAAGACAGGAGGGGAATGGGAAAT-3′. The PCR system components were as follows: 10 μl of 2× Rapid Taq Master Mix, 7 μl ddH2O, 1 μl 6 AM-F (10 μM), 1 μl 6 AM-R (10 μM), and1 μL DNA (300 ng·μL−1; Rapid Taq Master Mix, Vazyme). The PCR program for 6 AM comprised a cycle at 95°C for 3 min, 95°C for 15 s, 62°C for 15 s, and 72°C for 15 s, followed by 34 additional cycles of 95°C for 15 s, 62°C for 15 s, and 72°C for 15 s, and finally 72°C for 5 min. The PCR products were separated on 2% agarose gel. The primer pairs were located by PCR amplification using the nulli-tetrasomic lines of Chinese Spring as a template. The validity of the 6 AM primers was verified using PCR-based amplified fragment length polymorphism. Genotyping was performed in population 1, 2, 3, and 4 using the 6 AM molecular marker to characterize the function of alleles.
RNA extraction and qRT-PCR analysis
The 5 randomly selected recombination inbred lines (population 2), Yanfu 188, and Bima 4 with the BADH-A1a allele and the 5 randomly selected lines of population 2, Taishan 1, and Chinese Spring with the BADH-A1b allele were planted in half-strength Hoagland nutrient solution in a light incubator at 22°C under controlled conditions (60% relative humidity, 16/8 h light/dark cycle). At 4-leaf stage seedlings, Yanfu 188, Bima 4, Taishan 1, and Chinese Spring leaf samples were collected at untreated 0, 1, 6, 12, and 24 h. Subsequently, all wheat at 4-leaf stage seedlings were treated with half-strength Hoagland nutrient solution containing 200 mM NaCl or 20% PEG 6000. Leaf samples were collected at treated 0, 1, 6, 12, and 24 h. All samples were immediately stored at −80°C for further RNA extraction.
Total RNA was extracted using TRIzol (Qingke, Beijing), and cDNA was then synthesized using EasyScript One-Step gDNA Removal and cDNA Synthesis SuperMix (TransGen Biotech, Beijing). Quantitative real-time PCR (qRT-PCR) was performed using Perfect Start™ Green qPCR Supermix (TransGen Biotech, Beijing). TaActin was used as an internal reference gene for wheat. All qRT-PCR analyses were conducted in this study based on 3 technical replicates and 3 biological replicates. Relative gene expression levels were calculated using the 2−ΔΔCt method (Ma et al., 2020).
Betaine content determination
The materials used for expression analysis, Ning 9940 with BADH-A1a allele and Longfumai 18 with BADH-A1b allele, were grown and treated in the same way as above (RNA extraction and qRT-PCR analysis section). Leaf samples were collected from Longfumai 18, Taishan 1, Chinese Spring, Bima 4, Yanfu 188, and Ning 9940 to determine betaine content after plants had undergone stress treatments for 0, 1, 6, 12, 24, and 48 h with half-strength Hoagland nutrient solution containing 200 mM NaCl or 20% PEG 6000. Similarly, population 2 leaf samples were collected after stress treatment for 48 h with half-strength Hoagland nutrient solution containing 200 mM NaCl or 20% PEG 6000. All the samples were dried, each 0.2 g dry weight sample was thoroughly mixed with 1 mL of 80% methanol at 60°C for 30 min and centrifuged at 10,000 rpm for 15 min, and the supernatant from the sample that had absorbed methanol was completely volatilized to a constant volume of 1 ml as the sample solution. Betaine solutions at 0.1, 0.2, 0.3, 0.4, 0.5, 0.6, 0.7, 0.8, 0.9, and 1.0 mg·L−1 were prepared as standard solutions, with ddH2O as the blank or negative control. Then, 0.1 mL each of sample solution, standard solution, and ddH2O was thoroughly and separately mixed with 1 ml of saturated Reinecke salt solution (pH = 1) and incubated at 4°C for 2 h, centrifuged at 8000 rpm for 15 min, then the supernatant was removed and mixed with ether, followed by centrifuging at 8000 rpm for 15 min, and the supernatant from this step was then mixed with 70% acetone. The absorbance of this mixture was then measured at 525 nm. A standard curve was drawn using the absorbance values of the standard solutions. The experimental method and the formula for calculation of betaine content were used as indicated in the instructions of the Solarbio betaine content detection kit (BC3130). Three biological and technical replicates were analyzed for each condition.
Identification of drought resistance and salt tolerance in wheat
The drought resistance of 121 cultivars (lines; population 1) at the germination stage was characterized. A total of 100 seeds were placed into a 12 × 12 × 5 cm3 germinating box, and single-layer filter paper was used as the sprout bed. Then, 12 mL of 20% PEG 6000 was added to the treatment group and 12 mL of ddH2O was added to the control group. A further 100 seeds were used as replicates, and the experiments for the treatment and control groups were repeated 4 times each. The standard for wheat seed germination was defined as the point at which the length of the wheat radicle was equal to the length of the seed and the length of the germ reached half the length of the seed. The number of germinated seeds was investigated, and the relative germination rate of seeds was calculated after 168 h of culture at 20°C in the dark as a ratio of the germination rate of the treatment group divided by that of the control group.
Five lines with BADH-A1a and five lines with BADH-A1b from the recombination inbred lines (population 2) were planted in pots with nutritive soil (matrix of soil: vermiculite = 3:1) in a light incubator. The flowerpot dimensions were 10 × 10 cm2 at the top, 7.5 × 7.5 cm2 at the bottom, and 7.5 cm high, and each pot was filled with 165 g of nutritious soil and planted with 4 wheat seedlings. Drought and salinity stress treatments were imposed at the 4-leaf stage. Under drought treatment, wheat plants were deprived of water for 15 days and then re-watered for 5 days. Under salt stress treatment, wheat plants were irrigated with H2O solution containing 700 mM NaCl for 25 days. The ultimate number of surviving plants and survival rates were determined. Each experiment (with each line including 36 wheat plants) was repeated 3 times.
Field management and investigation of agronomic traits
In 2020, a total of 194 lines from population 2 and 182 lines from population 3 were planted in Yangling (34°16’ N, 108°4′ E; Shaanxi Province, China). Each line was planted in one 0.5 m row, with about 20–30 plants in each row, spaced 25 cm apart in each row. Compound (20% nitrogen, 18% phosphorus, 5% potassium; 300 kg/ha) and nitrogen (46% nitrogen; 300 kg/ha) fertilizers were applied to the wheat before planting. The field was irrigated in December 2020. Data on agronomic traits, including grain number per spike (GN), effective spikelet number per spike (ESN), thousand kernel weight (TKW), flag leaf area per plant (FLA), spike length (SL), length of last internode (LLI), and plant height (PH), were collected from populations 2 and 3. Three plants from each strain were randomly selected to measure GN, ESN, FLA, SL, LLI, and PH. The average values of the measurements were taken as the value for each agronomic trait. Spikelets containing grains were defined as effective spikelets. FLA was calculated as 0.7 × leaf length × leaf width. SL, LLI, and PH were measured from the bottom to the top of the spike, from the last internode to the bottom of the spike, and from ground level to the tip of the spike, respectively.
Association analysis
Genotyping of populations (1, 2, 3, and 4) was performed using the molecular marker 6 AM. Recombinant inbred lines (populations 2 and 3) were used to analyze the correlations between alleles and agronomic traits. The wheat mini-core collection (population 4) and 121 cultivars (lines) (population 1) were used to analyze the geographic distribution of different alleles, and the frequency of different alleles in wheat releasing years was analyzed using population 1.
Statistical analysis
The statistically significant differences in TaBADH-A1 expression level, betaine content, and phenotype between BADH-A1a and BADH-A1b were evaluated in SPSS 20 software.
Results
Sequence polymorphism and molecular marker development of TaBADH-A1 in wheat
A single pair of TaBADH-A1-specific primers were designed (Supplementary Table S1) to clone the region of promoter and genomic DNA containing introns and exons of TaBADH-A1 from the wheat cultivars Taishan 1, Bima 4, Zhongmai 9, and Lantian 23. Based on comparisons with the coding sequence (TraesCS6A02G371100), the sequence of TaBADH-A1 was found to consist of 15 exons and 14 introns (Figure 1). The length of promoter and genomic DNA that includes introns and exons of TaBADH-A1 in Taishan 1, Bima 4, Zhongmai 9, and Lantian 23 was 7,675, 7,919, 7,919, and 7,919 bp, respectively. The TaBADH-A1 gene sequence polymorphisms were analyzed in the 4 cloned cultivars and 15 cultivars from the database; we found 42 single nucleotide polymorphisms (SNPs) and four 1 bp and two 2 bp indels in the promoter region of TaBADH-A1 (Supplementary Table S2), and 28 SNPs, a 254 bp, two 1 bp, and a 2 bp indels in the genomic DNA. The TaBADH-A1 gene variations were grouped into 7 haplotypes (Supplementary Table S2). Haplotypes 3–7 had 2 additional ABREs, 1 additional I-box, 1 additional O2-site, 1 additional G-box, 1 additional STRE, 1 additional TCA-element, 1 additional W-box, 1 additional AT-TATA-box, and 1 less TGA element than haplotypes 1 and 2 in their promoter region. In the third intron region, the 254 bp fragment of insert, which was a DNA miniature inverted-repeat transposable element (MITE), contained 1 Box-4, 1 MRX, 2 W-box, 2 CAAT-box, and 11 TATA-box elements. The SNPs in exons do not lead to amino acid mutations. As DNA MITE can have a greater impact on gene function, we divided the 7 haplotypes with 254 bp indel fragments into 2 main alleles, BADH-A1a and BADH-A1b (Figure 1; Supplementary Table S2).
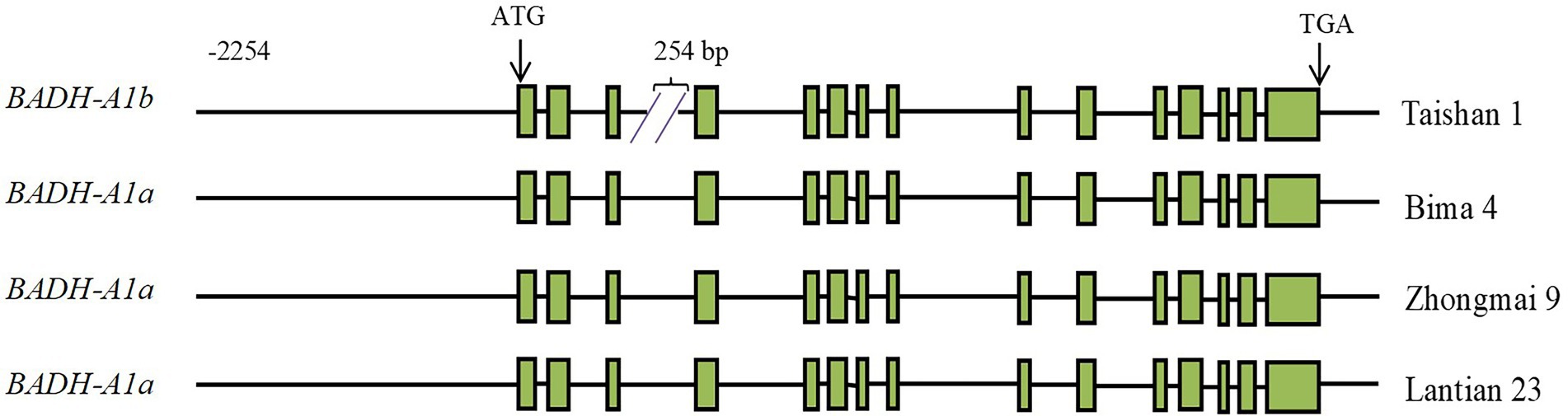
Figure 1. Schematic diagram of TaBADH-A1 gene structure. ATG start codon is designated as position 1 bp. Green rectangles are exons; black line before ATG is promoter region; black lines after ATG and before TGA are intron regions; black line after TGA is 3′ untranslated region.
Subsequently, specific primers were designed according to sequences at both ends of the 254 bp indel of the TaBADH-A1 third intron (Supplementary Table S1). DNA of Chinese Spring, N6AT6B, N6BT6D, and N6DT6A and ddH2O was used as templates for PCR using molecular marker primers. The PCR products using N6AT6B DNA and ddH2O as a template did not contain the target gene, while the other PCR products all contained the target gene (Supplementary Figure S1). The 6 AM marker primers amplify a region located in wheat chromosome 6A. Genotyping of all populations using a molecular marker showed the presence of amplified fragment length polymorphisms in PCR products, indicating the effectiveness of 6 AM as a molecular marker (Supplementary Figure S2). The amplified fragments of 6 AM were 828 and 574 bp, which correspond to BADH-A1a and BADH-A1b, respectively (Supplementary Figure S2). The functional molecular marker 6 AM was developed based on the 254 bp indel in TaBADH-A1 (Supplementary Table S1).
Expression analysis of TaBADH-A1 locus two alleles in wheat under drought and salt stress
DNA MITE insertion in the third intron might affect gene expression (Dumesic et al., 2013; Kim Guisbert and Sontheimer, 2013). At the 4-leaf stage, Yanfu 188 and Bima 4 with BADH-A1a and Taishan 1 and Chinese Spring with BADH-A1b were selected to investigate the expression of the two alleles. Under normal conditions, the expression pattern of TaBADH-A1 gene in the 4 cultivars was consistent, which showed no change in expression level at 0, 1, 6, 12, and 24 h (Supplementary Figure S3). Subsequently, the expression level of the TaBADH-A1 gene at 0 h of treated can be representative of the expression level of 1, 6, 12, and 24 h of untreated. Under stress treatment for 0 h, the expression level of BADH-A1b gene was higher than that of BADH-A1a. The expression pattern of TaBADH-A1 gene in the 4 cultivars was consistent, first increasing and then decreasing with the extension of the drought and salt stress time (Figures 2A,B). Notably, we found that expression levels of BADH-A1b were all significantly higher than those of BADH-A1a before and after stress (Figures 2A,B). Considering the differences in the genetic background among wheat cultivars, the population of F6 recombinant inbred lines (population 2) were constructed using Chinese Spring and Yanfu 188 as parents to further verify the above result. Lines 1–5 of population 2 with BADH-A1a and lines 6–10 of population 2 with BADH-A1b were selected for further study. Under drought and salt stress, the expression of TaBADH-A1 gene was found to first increase and then decrease (Figures 2C,D). Meanwhile, expression levels of BADH-A1b were significantly higher than those of BADH-A1a before and after stress treatment (Figures 2C,D). Therefore, the deletion of DNA MITE can enhance TaBADH-A1 gene expression.
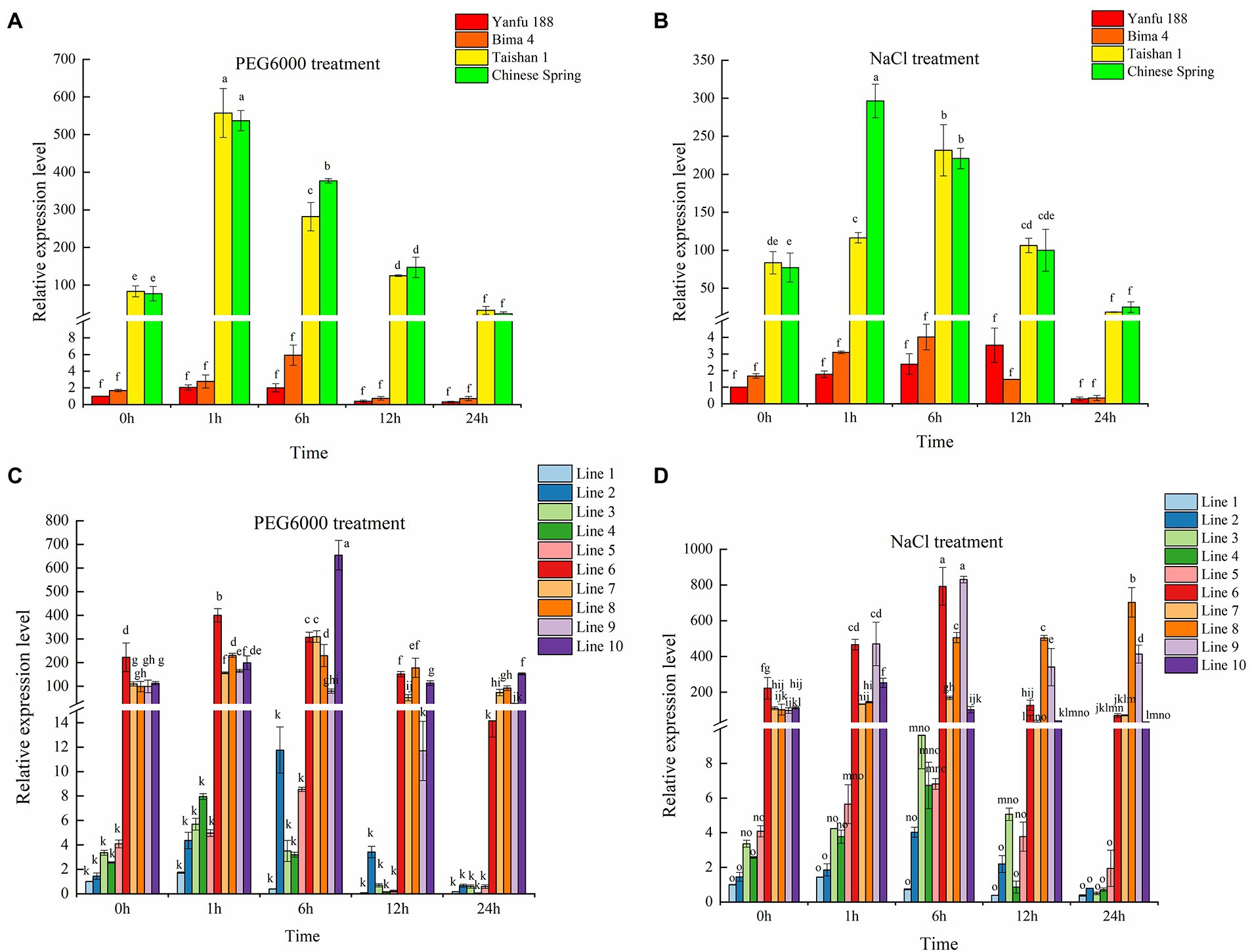
Figure 2. Expression patterns of TaBADH-A1 locus alleles in salt (200 mM NaCl) and drought (20% PEG 6000). Wheat was subjected to drought and salt stress at 4-leaf stage; leaves were used as samples for RNA extraction. (A,B) TaBADH-A1 expression of Yanfu 188 under control treatment was used as standard. Yanfu 188 and Bima 4 containing BADH-A1a; Taishan 1 and Chinese Spring containing BADH-A1b. (C,D) TaBADH-A1 expression of line 1 under control treatment was used as standard; lines 1–5 containing BADH-A1a; lines 6–10 containing BADH-A1b. The 2−ΔΔCT method was used to calculate relative gene expression. Statistically significant differences are indicated with different letters (LSD, p < 0.05).
Analysis of betaine content in wheat containing different alleles under drought and salt stress
Through genotyping of the 6 AM molecular marker, wheat cultivars with BADH-A1b (Longfumai 18, Taishan 1, Chinese Spring) and wheat cultivars with BADH-A1a (Bima 4, Yanfu 188, Ning 9940) were selected to identify betaine content under drought and salt stress at the 4-leaf stage. Under drought stress, we found that the betaine content in leaves of wheat cultivars containing BADH-A1b decreased slightly at 1 h and then increased gradually, while the content in cultivars containing BADH-A1a decreased slightly at 1 h, then increased and peaked at 12 h, and then began to decline (Figure 3A). Under salt stress, betaine content in the leaves of wheat cultivars containing BADH-A1a and BADH-A1b decreased slightly at 1 h, increased and peaked at 6 h, and then began to decline. However, the betaine content of wheat cultivars containing BADH-A1b increased again at 48 h (Figure 3B). It is noteworthy that the betaine content in leaves of wheat cultivars containing BADH-A1b was always higher compared to cultivars containing BADH-A1a regardless of drought or salt stress, and the largest difference was at 48 h of stress. This might be due to the fact that the expression level of BADH-A1b gene was always higher than that of BADH-A1a. Furthermore, we used recombinant inbred lines (population 2) to identify the betaine content of lines containing different alleles after 48 h of drought and salt stress. Under drought stress 48 h, the betaine content of lines 6–10, containing BADH-A1b, was 24, 23, 23, 25, and 24 μmol·g−1 (dry weight, DW), respectively, and the betaine content of lines 1–5, containing BADH-A1a, was 17, 16, 16, 16, and 16 μmol·g−1 (DW), respectively (Figure 3C). Under salt stress 48 h, the betaine content of lines 6–10 was 23, 22, 24, 25, and 23 μmol·g−1 (DW), respectively, and the content of lines 1–5 was 17, 16, 16, 16, and 15 μmol·g−1 (DW), respectively (Figure 3D). Therefore, wheat containing BADH-A1b had significantly higher betaine content than wheat with BADH-A1a under drought and salt stress.
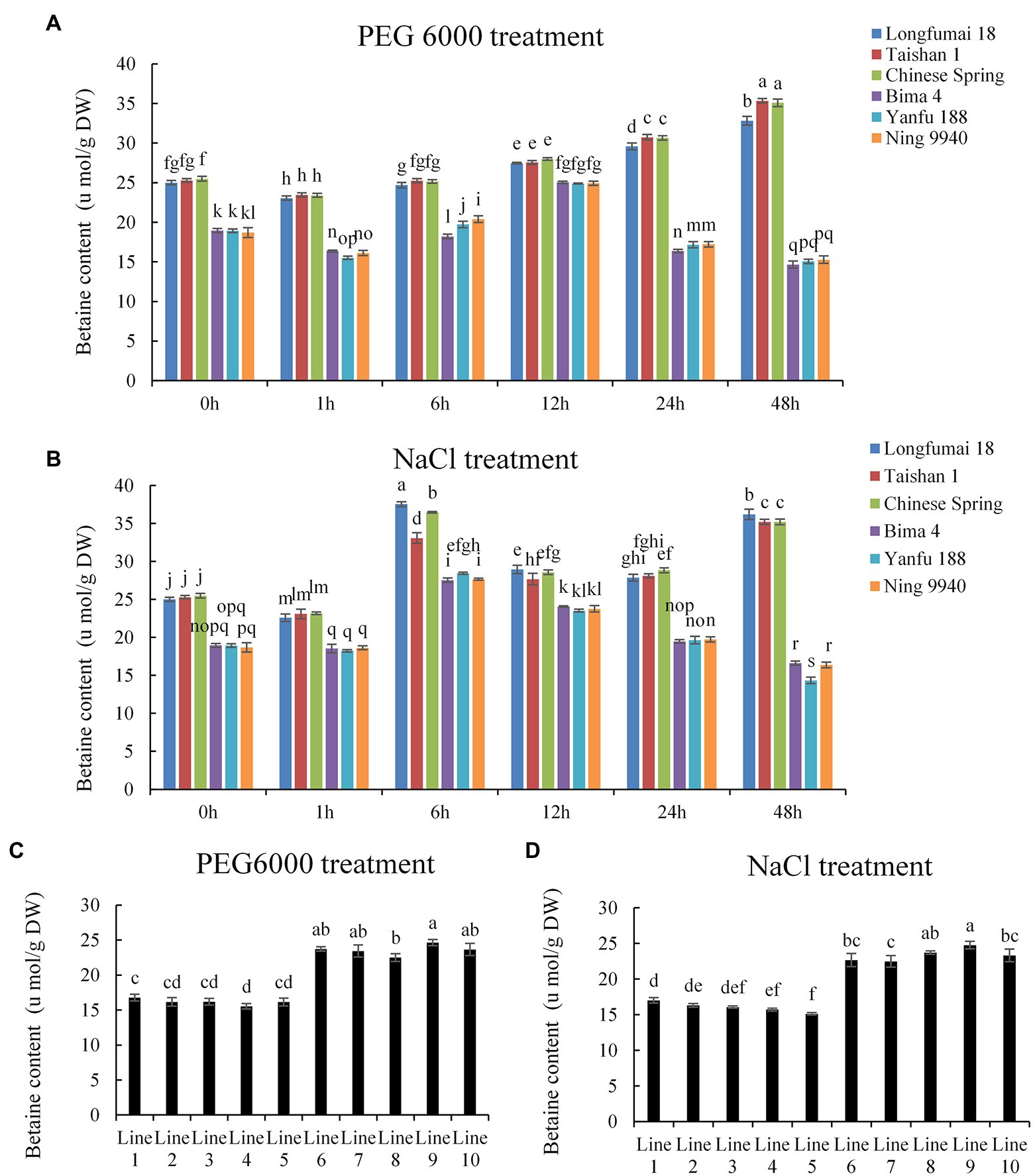
Figure 3. Betaine content of wheat containing different alleles under drought (20% PEG 6000) and salt stress (200 mM NaCl) at 4-leaf stage. DW, dry weight. (A,B) Bima 4, Yanfu 188, and Ning 9940 contain BADH-A1a; Longfumai 18, Taishan 1, and Chinese Spring containing BADH-A1b. (C,D) Betaine content of wheat under stress for 48 h; lines 1–5 containing BADH-A1a; lines 6–10 containing BADH-A1b. Statistically significant differences are indicated with different letters (LSD, p < 0.05).
Analysis of drought and salt resistance of wheat with different alleles
We identified the relative germination rate of 121 cultivars (lines; population 1). The average relative germination rate of wheat containing BADH-A1a was 51.2%, while that of BADH-A1b was 67.3% (Table 1). The wheat cultivars (lines) containing BADH-A1b had a significantly higher average relative germination rate than those with BADH-A1a, which shows that BADH-A1b can increase the drought resistance of wheat during the germination period.
The recombinant inbred lines (population 2) containing different alleles were treated with drought and salt in the 4-leaf stage. Under drought stress, the effects on lines 6–10, containing BADH-A1b, were relatively more mild (grew well with green leaves) than lines 1–5, containing BADH-A1a (Figure 4A). After 5 days of recovery water treatment, the survival rates of lines 6–10 were 49.3, 61.7, 52.3, 59.0, and 51.0%, respectively, while the survival rates of lines 1–5 were 38.7, 37.7, 36.7, 35.7, and 32.0%, respectively (Figure 4A). The results indicate that BADH-A1b could significantly increase the drought resistance of wheat at the 4-leaf stage. After 25 days of salt stress, leaves of lines 1–5, containing BADH-A1a, were more withered than those of lines 6–10, with BADH-A1b; the survival rates of lines 1–5 were 56.0, 71.3, 59.0, 66.7, and 72.0%, respectively, while the survival rates of lines 6–10 were 91.7, 85.0, 89.7, 86.7, and 88.0%, respectively (Figure 4B). The results show that BADH-A1b can significantly increase the salt tolerance of wheat at the 4-leaf stage.
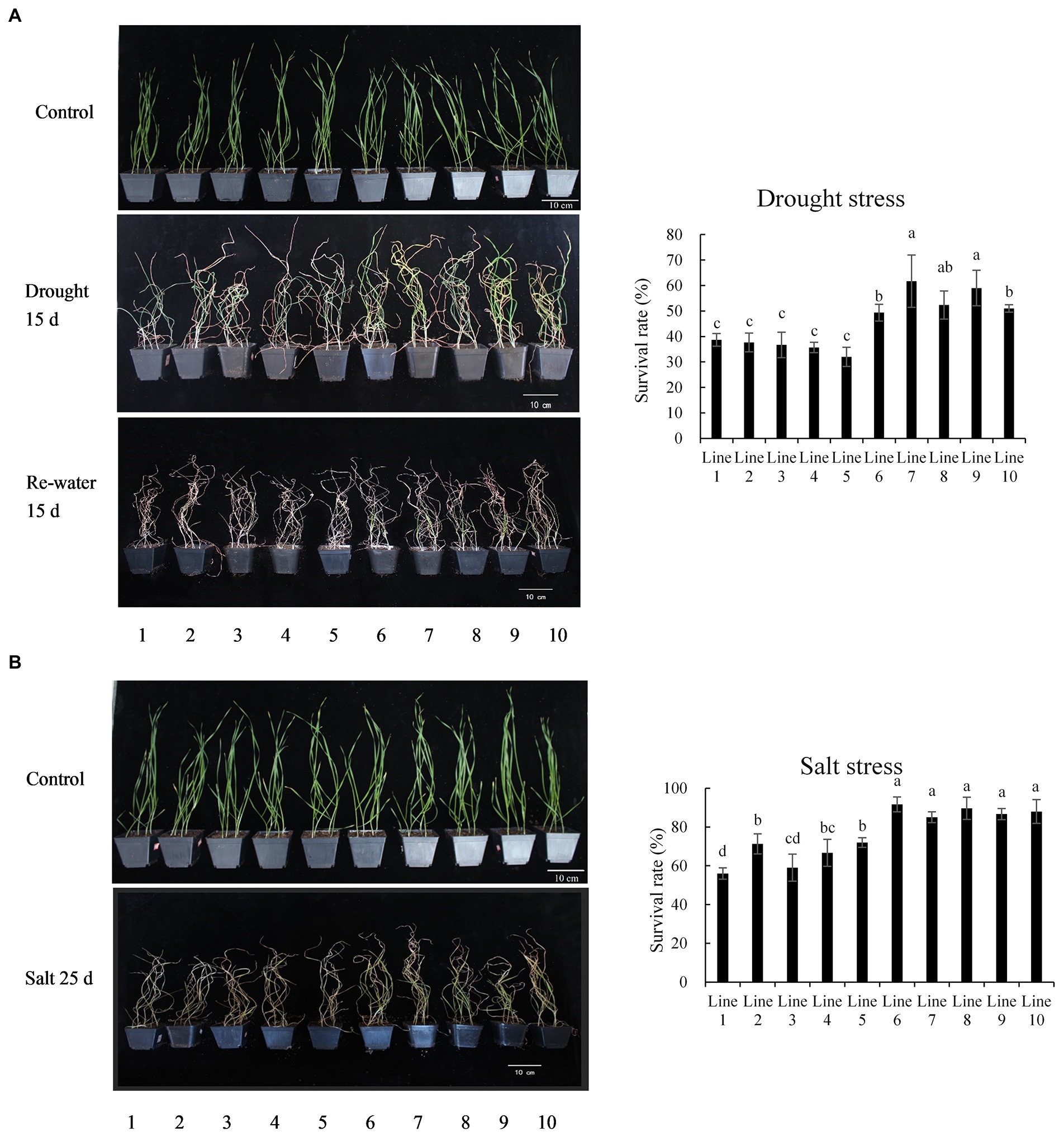
Figure 4. Phenotype analysis of recombinant inbred lines of TaBADH-A1 locus alleles under drought and salt stress. Lines 1–5 containing BADH-A1a; lines 6–10 containing BADH-A1b. (A) Survival rates calculated after withholding water for 15 days and water recovery for 5 days. (B) Survival rates were calculated under salt solution (700 mM NaCl) irrigation for 25 days. Statistically significant differences are indicated with different letters (LSD, p < 0.05).
Association of TaBADH-A1 locus alleles with agronomic traits
The recombinant inbred line populations (2 and 3) were genotyped according to PCR amplified fragment length polymorphisms using the 6 AM molecular marker. Subsequently, relationships between TaBADH-A1 locus alleles and agronomic traits were analyzed, including grain number per spike (GN), effective spikelet number per spike (ESN), thousand kernel weight (TKW), flag leaf area per plant (FLA), spike length (SL), length of the last internode (LLI), and plant height (PH). We found no significant differences in GN, ESN, TKW, FLA, SL, LLI, and PH between plants carrying either BADH-A1a or BADH-A1b (Supplementary Table S3).
Geographic distribution of BADH-A1a and BADH-A1b in Chinese zones
The wheat production area in China is divided into 10 major agro-ecological production zones based on cropping season, variety type, and ecological conditions (Li et al., 2016). The mini-core collection and 121 cultivars (lines; populations 1 and 4) covering all 10 zones were used to evaluate the geographic distribution of TaBADH-A1 locus alleles. Overall, BADH-A1a and BADH-A1b were distributed in all zones (Figure 5A). BADH-A1a was present in 59% of the mini-core collection (population 4), while BADH-A1b was present in 41% (Figure 5A). However, not all wheat zones had more plants with BADH-A1a than BADH-A1b in population 4, only the Northeastern Spring Wheat Zone, Southern Autumn-Sown Spring Wheat Zone, Qinghai-Tibetan Plateau Spring–Winter Wheat Zone, Southwestern Autumn-Sown Spring Wheat Zone, Middle and Lower Yangtze Valley Autumn-Sown Spring Wheat Zone, and more overseas introduction plants had BADH-A1a than BADH-A1b. In population 1, BADH-A1a accounted for the vast majority at 90%. The coverage of BADH-A1b was only slightly higher than BADH-A1a in the Northern Winter Wheat Zone, Northeastern Spring Wheat Zone, and Xinjiang Winter–Spring Wheat Zone (Figure 5B). The results show a weak selection of the favorable allele, BADH-A1b, in all zones.
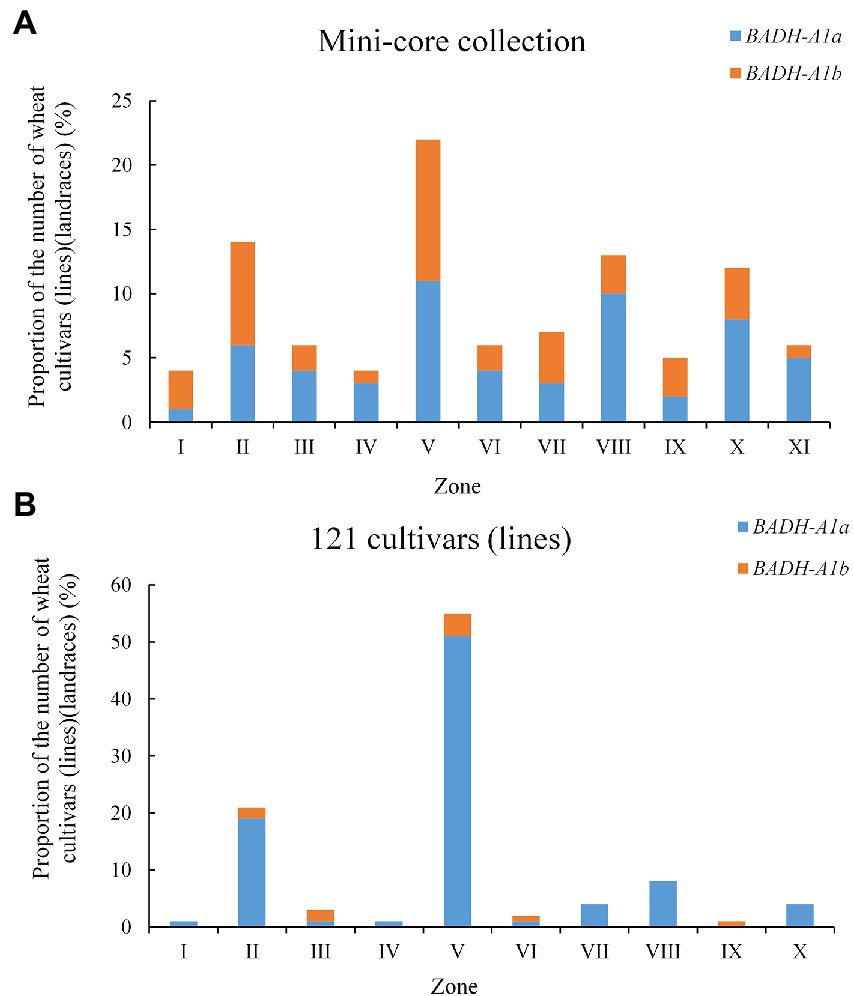
Figure 5. Geographic distribution of BADH-A1a and BADH-A1b in Chinese wheat production zones. (A) In mini-core collection population; (B) In 121 cultivars (lines) population. I, Northern Spring Wheat Zone; II, Northern Winter Wheat Zone; III, Northeastern Spring Wheat Zone; IV, Southern Autumn-Sown Spring Wheat Zone; V, Yellow and Huai River Valley Facultative Wheat Zone; VI, Qinghai-Tibetan Plateau Spring–Winter Wheat Zone; VII, Northwestern Spring Wheat Zone; VIII, Southwestern Autumn-Sown Spring Wheat Zone; IX, Xinjiang Winter–Spring Wheat Zone; X, Middle and Lower Yangtze Valley Autumn-Sown Spring Wheat Zone; XI, Overseas introduction.
Frequency of TaBADH-A1 locus alleles in wheat breeding history
To assess TaBADH-A1 locus allele frequency in different breeding years, the 121 cultivars (lines) were divided into 6 subgroups according to whether their release dates were in the 1950s, 1960s, 1970s, 1980s, or 1990s, or post-2000. BADH-A1a was present in 90% of the 121 cultivars (lines) and all 6 subgroups (Table 2). BADH-A1b, which first appeared in the 1980s and reached its peak in the post-2000s, accounted for only 10%, which indicates the allele BADH-A1b was selected by breeders to resist progressively deteriorating climatic conditions.
Discussion
Analysis of the mechanism of allele BADH-A1b in improving drought and salt tolerance in wheat
Osmotic adjustment is the main component of the physiological machinery of drought and salt tolerance in wheat (Khoshro et al., 2013). Betaine, a small, nontoxic organic metabolite, functions as an osmotic regulator under drought and salt stress (Yang et al., 2015). Betaine aldehyde dehydrogenase (BADH) is an important enzyme that catalyzes the last step of betaine synthesis (Fujiwara et al., 2008). In this study, the TaBADH-A1 gene, which encodes BADH, was cloned in Taishan 1, Bima 4, Zhongmai 9, and Lantian 23. Based on sequence polymorphism analysis, we found 7 haplotypes of the TaBADH-A1 gene in the 4 cloned wheat cultivars and 15 wheat cultivars from the database. We divided the seven haplotypes with 254 bp indel fragments into two main alleles, BADH-A1a and BADH-A1b, and the molecular marker 6 AM was developed based on these fragments.
First, the 254 bp fragment inserted into the third intron of BADH-A1a contains a TATA-box and W-box. A TATA-box is the core promoter element that initiates gene transcription and participates in the formation of the transcription initiation complex (Bernard et al., 2010). The WRKY transcription factors can bind to W-box, while most of the WRKY transcription factors of known function are negative regulators, with only a few of those characterized as having a positive regulatory role (Jiang et al., 2017). The WRKY transcription factors may recruit other proteins to bind to the W-box of the fragment inserted into the third intron of BADH-A1a to jointly inhibit BADH-A1a expression. Introns were previously considered useless gene sequences, but they have since been shown to regulate gene expression. For example, the adaptor protein MINI ZINC FINGER 2 binds to the WUSCHEL (WUS) intron and recruits KNUCKLES, TOPLESS, and HISTONE DEACETYLASE 19 to form a transcriptional repressor complex that represses WUS expression in A. thaliana (Bollier et al., 2018).
Second, pre-mRNAs containing transposons are subject to inefficient splicing (Dumesic et al., 2013). The introns that contain transposons exhibited a greater dwell time on spliceosomes, and pre-mRNAs in the stalled spliceosomes became substrates for the synthesis of siRNAs used to dampen expression of the corresponding gene (Kim Guisbert and Sontheimer, 2013). The 254 bp fragment inserted into the third intron of BADH-A1a contain DNA miniature inverted-repeat transposable elements, which might reduce splicing efficiency and thus suppress BADH-A1a expression. The above two processes may be responsible for the higher expression level of BADH-A1b than BADH-A1a before and after stress.
BADH-A1b was always found to be expressed at a high level, which results in increased betaine content. After 48 h of stress, leaves of wheat containing BADH-A1a had decreased betaine content, while leaves of wheat containing BADH-A1b maintained a higher betaine content. We also identified that the average relative germination and survival rates of wheat with BADH-A1b allele under drought and salt stress were significantly higher compared to wheat with BADH-A1a allele. BADH-A1b which can increase the content of betaine may decrease the osmotic potential and increase the osmotic pressure of cells for over a longer period of stress time more markedly than BADH-A1a, further enhancing the drought resistance and salt tolerance of wheat (Table 1 and Figure 4). Meanwhile, in wheat growing under normal water supply, BADH-A1b had no effect on the agronomic traits, which indicated excellent growth performance (Supplementary Table S3). However, BADH-A1b may confer improvements measurable in some agronomic traits in wheat subject to drought and salt conditions, though further research is required to determine whether this is the case.
Distribution of TaBADH-A1 locus alleles in Chinese wheat
In Chinese wheat, the mini-core collection (population 4) accounts for 70% of the genetic representation. The two alleles of TaBADH-A1 locus were almost equally represented in the wheat mini-core collection (population 4; Figure 5A). The genetic resources of the two alleles were present in all wheat regions of China. This might be due to the fact that wheat zones have not been dry for many years. Wheat breeding in China has experienced three stages since the 1950s: disease resistance and stable yield, dwarfing and high yield, and high yield and excellent quality (He et al., 2011). Therefore, improving the adaptability of wheat has been neglected. This has resulted in an extremely uneven distribution of the two studied alleles in 121 cultivars (lines; population 1): BADH-A1a accounts for 90% while BADH-A1b accounts for only 10% (Figure 5B). Improving wheat stress resistance and paying attention to water saving have been proposed since the 1980s (He et al., 2018). This purpose might well explain the absence of BADH-A1b prior to the 1980s (Table 2). Since 2011, research efforts focusing on the lasting resistance of wheat have increased, considering such aspects as drought resistance, heat resistance, and adaptability to other factors (He et al., 2011). Therefore, the favorable allele BADH-A1b should be continuously selected for wheat growth under future unpredictable climate deterioration.
In summary, we found seven haplotypes of the TaBADH-A1 gene in wheat, the seven haplotypes divided with 254 bp indel into two alleles. The expression level of BADH-A1b was significantly higher than that of BADH-A1a under drought and salt stress. BADH-A1b can increase the betaine content of wheat and further enhance its drought resistance and salt tolerance. BADH-A1b had no effect on the agronomic traits of wheat grown under normal conditions. Notably, the favorable allele BADH-A1b has been neglected in the selection, but it should be continuously selected in the face of future climate deterioration. Our study provides evidence indicating that BADH-A1b is an excellent candidate allele for breeding to improve the drought resistance and salt tolerance of wheat.
Data availability statement
The original contributions presented in the study are included in the article/Supplementary Material, further inquiries can be directed to the corresponding authors.
Author contributions
XZ and JX conceived and designed the experiments. MY and YY performed the experiments, analyzed the data, and wrote the manuscript. YY, MZ, SG, NL, FW, SZ, and HZ revised the manuscript. All authors contributed to the article and approved the submitted version.
Funding
This study was funded by the National Natural Science Foundation of China (32171992, 31671693 and 31260324), Key Research and Development Project of Shaanxi Province (2019ZDLNY04-05), Central Guidance on Local Science and Technology Development Fund of Mongolia Autonomous Region (ZY20200089), Program for Young Talents of Science and Technology in Universities of Inner Mongolia Autonomous Region (NJYT-19-A25), and Research Program of Science and Technology at Universities of Inner Mongolia Autonomous Region (NJZZ22132).
Acknowledgments
We thank funding for our support and teacher Haochen Yang, for providing the mini-core collection.
Conflict of interest
The authors declare that the research was conducted in the absence of any commercial or financial relationships that could be construed as a potential conflict of interest.
Publisher’s note
All claims expressed in this article are solely those of the authors and do not necessarily represent those of their affiliated organizations, or those of the publisher, the editors and the reviewers. Any product that may be evaluated in this article, or claim that may be made by its manufacturer, is not guaranteed or endorsed by the publisher.
Supplementary material
The Supplementary Material for this article can be found online at: https://www.frontiersin.org/articles/10.3389/fpls.2022.942359/full#supplementary-material
Footnotes
1. ^https://plants.ensembl.org/index.html
3. ^https://bioinformatics.psb.ugent.be/webtools/plantcare/html/
References
Bernard, V., Brunaud, V., and Lecharny, A. (2010). TC-motifs at the TATA-box expected position in plant genes: a novel class of motifs involved in the transcription regulation. BMC Genomics 11:166. doi: 10.1186/1471-2164-11-166
Bollier, N., Sicard, A., Leblond, J., Latrasse, D., Gonzalez, N., and Gévaudant, F. (2018). At-mini zinc finger 2 and sl-inhibitor of meristem activity, a conserved missing link in the regulation of floral meristem termination in Arabidopsis and tomato. Plant Cell 30, 83–100. doi: 10.1105/tpc.17.00653
Cha-Um, S., and Kirdmanee, C. (2010). Effect of glycinebetaine on proline, water use, and photosynthetic efficiencies, and growth of rice seedlings under salt stress. Turk. J. Agric. For. 34, 517–527. doi: 10.3906/tar-0906-34
Dumesic, P. A., Natarajan, P., Chen, C., Drinnenberg, I. A., Schiller, B. J., and Thompson, J. (2013). Stalled spliceosomes are a signal for RNAi-mediated genome defense. Cell 152, 957–968. doi: 10.1016/j.cell.2013.01.046
Fan, W. J., Zhang, M., Zhang, H. X., and Zhang, P. (2012). Improved tolerance to various abiotic stresses in transgenic sweet potato (Ipomoea batatas) expressing spinach betaine aldehyde dehydrogenase. PLoS One 7:e37344. doi: 10.1371/journal.pone.0037344
Fujiwara, T., Hori, K., Ozaki, K., Yokota, Y., Mitsuya, S., Ichiyanagi, T., et al. (2008). Enzymatic characterization of peroxisomal and cytosolic betaine aldehyde dehydrogenases in barley. Physiol. Plant. 134, 22–30. doi: 10.1111/j.1399-3054.2008.01122.x
Gadallah, M. A. A. (1999). Effects of proline and glycinebetaine on Viciafaba responses to salt stress. Biol. Plant. 42, 249–257. doi: 10.1023/A:1002164719609
He, Z. H., Xia, X. C., Chen, X. M., and Zhuang, Q. S. (2011). Progress of wheat breeding in China and the future perspective. Acta Agron. Sin. 37, 202–215. doi: 10.3724/SP.J.1006.2011.00202
He, Z. H., Zhuang, Q. S., Cheng, S. H., Yu, Z. W., Zhao, Z. D., and Liu, X. (2018). Wheat production and technology improvement in China. J. Agric. 8, 99–106. doi: 10.11923/j.issn.2095-4050.cjas2018-1-107
Jiang, J., Ma, S., Ye, N., Jiang, M., Cao, J., and Zhang, J. (2017). WRKY transcription factors in plant responses to stresses. J. Integr. Plant Biol. 59, 86–101. doi: 10.1111/jipb.12513
Jing, R. L., Chang, X. P., Hu, H. R., and Dong, Y. C. (1999). A relationship between betaine metabolism and drought resistance of winter wheat seedling under changed watering. Acta Agron. Sin. 25, 494–498.
Khoshro, H. H., Taleei, A., Bihamta, M. R., Shahbazi, M., and Abbasi, A. (2013). Expression analysis of the genes involved in osmotic adjustment in bread wheat (Triticum aestivum L.) cultivars under terminal drought stress conditions. J. Crop Sci. Biotechol. 16, 173–181. doi: 10.1007/s12892-013-0040-7
Kim Guisbert, K. S., and Sontheimer, E. J. (2013). Quit stalling or you’ll be silenced. Cell 152, 938–939. doi: 10.1016/j.cell.2013.02.025
Legaria, J., Rajsbaum, R., Muñoz-Clares, R. A., Villegas-Sepúlveda, N., Simpson, J., and Iturriaga, G. (1998). Molecular characterization of two genes encoding betaine aldehyde dehydrogenase from amaranth. Expression in leaves under short-term exposure to osmotic stress or abscisic acid. Gene 218, 69–76. doi: 10.1016/s0378-1119(98)00381-3
Li, B., Li, Q. R., Mao, X. G., Li, A., Wang, J. Y., Chang, X. P., et al. (2016). Two novel AP2/EREBP transcription factor genes TaPARG gave pleiotropic functions on plant architecture and yield-related traits in common wheat. Front. Plant Sci. 7:1191. doi: 10.3389/fpls.2016.01191
Li, Y. H., Wang, W., Yang, X. H., and Zou, Q. (2005). Expression of BADH and betaine content in wheat cultivars with different drought resistance under drought stress. Acta Agron. Sin. 31, 425–430.
Ma, X. J., Fu, J. D., Tang, Y. M., Yu, T. F., Yin, Z. G., Chen, J., et al. (2020). GmNFYA13 improves salt and drought tolerance in transgenic soybean plants. Front. Plant Sci. 11:587244. doi: 10.3389/fpls.2020.587244
Mäkelä, P., Karkkainen, J., and Somersalo, S. (2000). Effect of glycinebetaine on chloroplast ultrastructure, chlorophyll and protein content, and RuBPCO activities in tomato grown under drought or salinity. Biol. Plant. 43, 471–475. doi: 10.1023/A:1026712426180
Missihoun, T. D., Schmitz, J., Klug, R., Kirch, H. H., and Bartels, D. (2011). Betaine aldehyde dehydrogenase genes from Arabidopsis with different sub-cellular localization affect stress responses. Planta 233, 369–382. doi: 10.1007/s00425-010-1297-4
Stein, N., Herren, G., and Keller, B. (2010). A new DNA extraction method for high-throughput marker analysis in a large-genome species such as Triticum aestivum. Plant Breed. 120, 354–356. doi: 10.1046/j.1439-0523.2001.00615.x
Sun, Y. L., Liu, X., Fu, L. S., Qin, P., Li, T., Ma, X. F., et al. (2019). Overexpression of TaBADHincreases the salt tolerance in Arabidopsis. Can. J. Plant Sci. 99, 546–555. doi: 10.1139/cjps-2018-0190
Tadesse, W., Halila, H., Jamal, M., El-hanafi, S., Assefa, S., Oweis, T., et al. (2017). Role of sustainable wheat production to ensure food security in the CWANA region. J. Exp. Biol. Agricult. Sci. 5, 15–32. doi: 10.18006/2017.5(Spl-1-SAFSAW)
Urbanavičiūtė, I., Bonfiglioli, L., and Pagnotta, M. A. (2021). One hundred candidate genes and their roles in drought and salt tolerance in wheat. Int. J. Mol. Sci. 22:6378. doi: 10.3390/ijms22126378
Wang, X. L., Zhang, X. X., Chen, J., Wang, X., Cai, J., Zhou, Q., et al. (2018). Parental drought-priming enhances tolerance to post-anthesis drought in offspring of wheat. Front. Plant Sci. 9:261. doi: 10.3389/fpls.2018.00261
Weretilnyk, E. A., and Hanson, A. D. (1990). Molecular cloning of a plant betaine-aldehyde dehydrogenase, an enzyme implicated in adaptation to salinity and drought. Proc. Natl. Acad. Sci. U. S. A. 87, 2745–2749. doi: 10.1073/pnas.87.7.2745
Yang, C. L., Zhou, Y., Fan, J., Fu, Y. H., Shen, L. B., Yao, Y., et al. (2015). SpBADH of the halophyte Sesuvium portulacastrum strongly confers drought tolerance through ROS scavenging in transgenic Arabidopsis. Plant Physiol. Biochem. 96, 377–387. doi: 10.1016/j.plaphy.2015.08.010
Younis, A., Ramzan, F., Ramzan, Y., Zulfiqar, F., Ahsan, M., and Lim, K. B. (2020). Molecular markers improve abiotic stress tolerance in crops: a review. Plan. Theory 9:1374. doi: 10.3390/plants9101374
Zhang, T. P., Li, Z. M., Li, D. X., Li, C. Y., Wei, D. D., Li, S. F., et al. (2020). Comparative effects of glycinebetaine on the thermotolerance in coda- and BADH-transgenic tomato plants under high temperature stress. Plant Cell Rep. 39, 1525–1538. doi: 10.1007/s00299-020-02581-5
Zhao, S. Q., Lin, C., Yang, X. H., and Wei-Hua, W. U. (2001). Relationship between drought resistance and betaine aldehyde dehydrogenase in the shoots of different genotypic wheat and sorghum. Acta Bot. Sin. 43, 108–110.
Keywords: wheat, drought, salt, TaBADH-A1, allele
Citation: Yu M, Yu Y, Guo S, Zhang M, Li N, Zhang S, Zhou H, Wei F, Song T, Cheng J, Fan Q, Shi C, Feng W, Wang Y, Xiang J and Zhang X (2022) Identification of TaBADH-A1 allele for improving drought resistance and salt tolerance in wheat (Triticum aestivum L.). Front. Plant Sci. 13:942359. doi: 10.3389/fpls.2022.942359
Edited by:
Bhaskar Gupta, Government General Degree College, Singur, IndiaReviewed by:
Jesus Alfredo Rosas Rodríguez, University of Sonora, MexicoCinzia Montemurro, University of Bari Aldo Moro, Italy
Copyright © 2022 Yu, Yu, Guo, Zhang, Li, Zhang, Zhou, Wei, Song, Cheng, Fan, Shi, Feng, Wang, Xiang and Zhang. This is an open-access article distributed under the terms of the Creative Commons Attribution License (CC BY). The use, distribution or reproduction in other forums is permitted, provided the original author(s) and the copyright owner(s) are credited and that the original publication in this journal is cited, in accordance with accepted academic practice. No use, distribution or reproduction is permitted which does not comply with these terms.
*Correspondence: Jishan Xiang, eGlhbmdqc2hAMTI2LmNvbQ==; Xiaoke Zhang, emhhbmd4aWFva2U2NkAxMjYuY29t