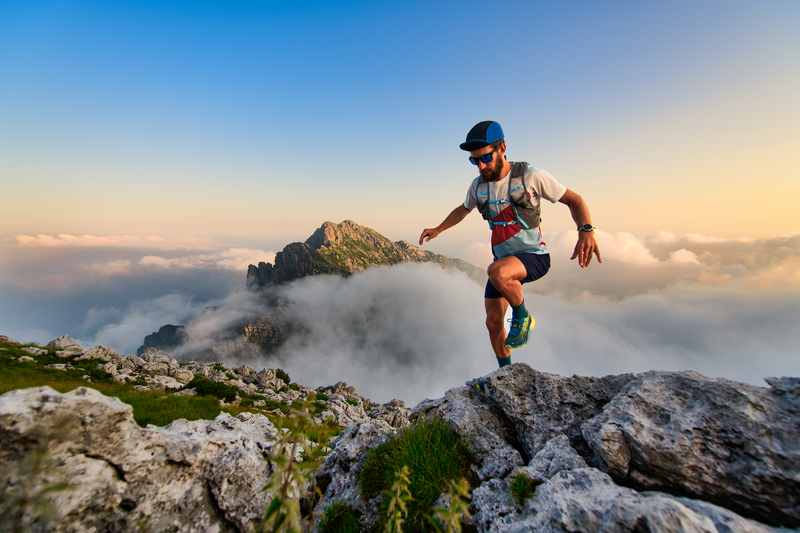
95% of researchers rate our articles as excellent or good
Learn more about the work of our research integrity team to safeguard the quality of each article we publish.
Find out more
ORIGINAL RESEARCH article
Front. Plant Sci. , 06 July 2022
Sec. Plant Pathogen Interactions
Volume 13 - 2022 | https://doi.org/10.3389/fpls.2022.932762
Winter wheat cultivar Pindong 34 has both adult-plant resistance (APR) and all-stage resistance (ASR) to stripe rust, which is caused by Puccinia striiformis f. sp. tritici (Pst). To map the quantitative trait loci (QTL) for stripe rust resistance, an F6−10 recombinant inbred line (RIL) population from a cross of Mingxian 169 × Pingdong 34 was phenotyped for stripe rust response over multiple years in fields under natural infection conditions and with selected Pst races under controlled greenhouse conditions, and genotyping was performed with a 90K single nucleotide polymorphism (SNP) array chip. Inclusive composite interval mapping (ICIM) identified 12 APR resistance QTLs and 3 ASR resistance QTLs. Among the 12 APR resistance QTLs, QYrpd.swust-1BL (explaining 9.24–13.33% of the phenotypic variation), QYrpd.swust-3AL.1 (11.41–14.80%), QYrpd.swust-3AL.2 (11.55–16.10%), QYrpd.swust-6BL (9.39–12.78%), QYrpd.swust-6DL (9.52–16.36%), QYrpd.swust-7AL (9.09–17.0%), and QYrpd.swust-7DL (8.87–11.38%) were more abundant than in the five tested environments and QYrpd.swust-1AS (11.05–12.72%), QYrpd.swust-1DL (9.81–13.05%), QYrpd.swust-2BL.1 (9.69–10.57%), QYrpd.swust-2BL.2 (10.36–12.97%), and QYrpd.swust-2BL.3 (9.54–13.15%) were significant in some of the tests. The three ASR resistance QTLs QYrpd.swust-2AS (9.69–13.58%), QYrpd.swust-2BL.4 (9.49–12.07%), and QYrpd.swust-7AS (16.16%) were detected based on the reactions in the seedlings tested with the CYR34 Pst race. Among the 15 QTLs detected in Pindong 34, the ASR resistance gene QYrpd.swust-7AS mapped on the short arm of chromosome 7A was likely similar to the previously reported QTL Yr61 in the region. The QTLs identified in the present study and their closely linked molecular markers could be useful for developing wheat cultivars with durable resistance to stripe rust.
Stripe rust, which is caused by Puccinia striiformis f. sp. tritici, is one of the most destructive fungal diseases of wheat in many cool and temperate regions of the world (Wellings, 2011), with China representing one of the most seriously affected areas (Wan et al., 2004, 2007). The pathogen of stripe rust is capable of long-distance dissemination and produces large-scale epidemics in a relatively short time, particularly under disease-favorable environmental conditions (Chen, 2020).
Compared to control measures, such as chemicals and cultivation methods, growing resistant cultivars is the most economical, environmentally friendly, and effective method for mitigating the disease (Chen, 2013). However, wheat breeding for stripe rust resistance has faced severe obstacles, such as the high frequency of variation in stripe rust races, which results in the continuous appearance of new physiological races, and many main wheat varieties have a single source of resistance, resulting in the frequent loss of resistance in agricultural production (Chen, 2013, 2020; Zhao et al., 2016). Therefore, exploring new effective disease resistance genes and identifying molecular markers closely linked to resistance genes are very important prerequisites for future breeding programs.
To date, more than 83 Yr genes for resistance to stripe rust have been identified and officially named, and dozens of temporarily named Yr genes or quantitative trait loci (QTL) have been reported (Maccaferri et al., 2015; McIntosh et al., 2017). Wheat stripe rust resistance genes can be divided into different types according to different classification criteria. One of the main classification methods divides the genes into two categories: all-stage resistance (also called seedling resistance) and adult-plant resistance (Chen, 2005, 2013). All-stage resistance can be detected at the seedling stage and is expressed throughout all the growth stages. This type of resistance is generally controlled by a single or several major genes, and at present, most of the resistance genes identified belong to all-stage resistance. This type of resistance is specialized to physiological races; that is, the variation and evolution of physiological races often lead to the loss of disease resistance in a short period of time, meaning that the persistence and stability of this type of resistance cannot be ensured (Chen, 2013). In contrast, adult-plant resistance shows a disease susceptibility response in the seedling stage, and as the plant grows older and the weather becomes warmer, the resistance usually shows a low infection type, low severity, and slow disease development rate in the adult stage. Adult resistance is usually not specific to pathogen races, thus reducing the selection pressure of wheat varieties on stripe pathogen races, and it corresponds to lasting and stable disease resistance. According to previous research results, adult resistance is generally controlled by several micro-effect genes (Chen, 2013) and could also be controlled by major effect genes, such as Yr52 (Ren et al., 2012) and Yr59 (Zhou et al., 2014). Due to these characteristics of adult resistance, many researchers worldwide have utilized resistance in combination with all-stage resistance as the main direction of adult-plant breeding wheat varieties exhibiting disease resistance.
Pindong 34 is a winter wheat cultivar bred in 1990 by the Institute of Crop Variety Resources, Chinese Academy of Agricultural Sciences, and it exhibits a high level of resistance to stripe rust and most other wheat diseases and insect pests. In 2014, Zhou et al. (2014) mapped the seedling resistance gene Yr61 using a population of 208 F2 plants and 128 derived F2:3 lines in a cross between Mingxian 169 and Pindong 34. To effectively utilize the high level of resistance against stripe rust in Pindong 34, it is necessary to determine the mode of inheritance and identify the molecular markers linked to stripe rust resistance. The objectives of the present study were to characterize the resistance in Pindong 34, map and identify genes for all-stage resistance and adult-plant resistance, and develop molecular markers linked to the two types of resistance genes.
Pindong 34 was selected from the cross [(Yan 7578/81/128)//176(15)9-26/Dongda 2]. Although it has the characteristics of low plant height, high 1,000-grain weight, and large grains, Pindong 34 had not been widely used in production. The most important characteristic of Pindong 34 is that it possessed resistance to various stripe rust races based on seedling tests and adult tests conducted by previous researchers under both controlled greenhouse and field conditions (unpublished data). Pindong 34 was crossed as a male parent with Mingxian 169 (MX169), which has a genotype susceptible to stripe rust. An F6:10 recombinant inbred line (RIL) population consisting of 119 lines was developed from a single F1 plant, and it was used for genetic analysis and molecular mapping.
A total of 119 F6:10 RIL populations derived from a cross between Pindong 34 and Mingxian 169 were included in each test. Seedlings were grown for 15 days in a rust-free growth chamber at a temperature cycle of 20 °C during daytime and 16 °C at night, with a photoperiod of 16 h. At the two-leaf stage, seedlings were uniformly inoculated with fresh urediniospores of race CYR34 with talc at a ratio of 1:20. Inoculated plants were kept in a dark dew chamber at 10 °C and over 100% relative humidity for 24 h for urediniospores to germinate and infect the plants, which were then moved to a growth chamber set to a diurnal cycle, with a gradual change in the temperature from 12°C to 16°C and a 16-h photoperiod, to enhance stripe rust development. The infection type was recorded 15 days after inoculation using the 0–9 scale (Line and Qayoum, 1992). Seedlings of MX169 were included as the susceptibility check.
The 119 RIL families and parents were grown in natural environments of Mianyang (31°33′ N, 104°55′ E) of Sichuan province and Yangling (34°17′ N, 108°04′ E) of Shaanxi province in China from 2016 to 2020. The RILs were planted in a randomized block design with two replicates. The plots were 1 m long and 25 cm apart. Twenty seeds were planted in each row. Every 20 lines, the highly susceptible stripe rust wheat variety Mingxian 169 was planted, and in each environment around the plot, Mingxian 169 was also planted as an inducer. The soil conditions in the experimental fields were favorable for crop growth and development and grain filling.
Mianyang is located in southwestern China at an average altitude of 485 m above sea level, and is characterized by a mean annual rainfall of 823.3 mm (36 years average) and a mean annual temperature of 16.0 °C (36 years average). Mianyang is a natural over-wintering and over-summering region for stripe rust in China and nurseries regularly become infected without artificial inoculation. Yangling is located in northwestern China at an average altitude of 519 m above sea level, and is characterized by a mean annual rainfall of 635.1 mm per year and a mean annual temperature of 12.9 °C. Trials at Yangling were inoculated with a mixture of Pst races CYR32 and CYR34 suspended in liquid paraffin (1:300) and sprayed onto Mingxian 169 and Avocet S at flag leaf emergence. When the susceptible variety reached 90%−100%, the response type severity was recorded for both the Mianyang and Yangling district populations and their parents according to criteria from grades 0–9 as described by Line and Qayoum (1992).
The DNA from the parents (Pindong 34 and Mingxian 169) and 119 RIL populations was isolated from young leaves after freezing in liquid nitrogen and grinding using a modified cetyltrimethylammonium bromide (CTAB) method (Li et al., 2013). After removing RNA with RNase and centrifugation, DNA was dissolved in Tris-EDTA buffer (10 mM Tris-HCl and 1 mM EDTA, pH 8.0). The DNA quality and concentrations were determined by electrophoresis and spectrophotometry (NanoDrop ND-1000, Thermo Scientific, Wilmington, DE, USA). The stock DNA solutions were diluted with sterilized ddH2O to different concentrations depending on the requirements of the individual experiment for further molecular analysis.
Two STS markers were developed from the RGAP markers flanking the resistance gene Yr61 reported in 2014 and screened for polymorphisms between the parents of the RIL population. The polymorphic markers were used to genotype the RILs. The distributions of the markers on wheat chromosome 7AS were determined based on Zhou et al. (2014). The STS markers STS5467 (forward primer: 5′-GTAGGTTCTCGACTCCACACAC-3′ and reverse primers: 5′-GTTGTAGGAGCAGCGGAAGAAC-3′) and STS5765b (forward primer: 5′-ATCATCTCATTAGCGTTTAG-3′ and reverse primer: 5′-CTCTGCCTGTGCTTTCGG-3′) were used to detect the products of polymerase chain reaction (PCR) through direct labeling. The PCR mixture, amplification conditions, and product detection for STS markers included initial denaturation at 94°C for 4 min and 35 cycles of 94°C for 35 s, 55°C for 35 s, and 72°C for 35 s (Zhou et al., 2014).
Genotype data from the DNA of the 119 populations was scanned by the 90K chip assay to perform the preliminary genotyping of missing and unexamined data. Marker deletion was performed using the “Bin” function in ICI Mapping v4.1 software, and genetic mapping was performed using ICI Mapping v4.1 software with the Kosambi mapping function (Kosambi, 2016).
The infection type (IT) and disease severity (DS) data of both the seedling stage and adult-plant stage in each year and location were used for QTL analysis and mapping, and QTL mapping was performed with ICI Mapping v4.1 software to detect possible QTLs. When the LOD value was >2.5, a QTL was identified, and the contribution rate and additive effect value of each QTL were calculated.
The phenotypic data were analyzed based on a variance analysis (ANOVA) in ICI Mapping v4.1 software for variance analysis and generalized heritability calculation. Broad-sense heritability (h2) of stripe rust resistance was estimated as /( + /r + /re) (Wang, 2009; Meng et al., 2015), where genotypic (line), genotype × environment interaction, residual error variances, e=number of environments, and r=replicates per environment.
To determine the additive effects and combinations of QTLs, boxplots were plotted for the mean IT and mean DS values of the 119 RILs sharing the same number of beneficial alleles.
The locations of the QTLs identified in this study were compared with those of previously published resistance genes or QTLs to stripe rust to obtain the physical positions, reference sequences, and marker alignments of polymorphic SNP markers, and the SNP probes were aligned with the Chinese Spring sequence through a BLAST search (IWGSC Ref Seq v1.0; https://plants.ensembl.org/Triticum_aestivum/Info/Index).
A test of the 119 RIL populations from the Mingxian169 × Pingdong 34 cross at the seedling stage with the race CYR34 showed that Pindong 34 was highly resistant (IT 0) and Minxian 169 was susceptible (IT 9). The 119 F6:10 RIL population showed two-stage differentiation segregation in terms of IT, and the lines were classified into resistant (IT 0–2) and susceptible (IT 9) groups (Figures 1, 2). The ratio of resistant and susceptible families was 15:104 (expected ratio of 17:102), suggesting that three genes (two dominant and one recessive, χ2 = 0.21, P = 0.65) are responsible for resistance to race CYR34 in Pindong 34 at the seedling stage. In the greenhouse test, all the RILs were highly resistant (IT 0–2) or highly susceptible (IT 9), indicating that the race-specific all-stage resistance was mainly qualitative. Thus, the results showed that some genes conferred race-specific all-stage resistance in Pindong 34.
Figure 1. Frequency distributions of seedling infection type (IT) value in the Mingxian 169 (MX) × Pindong 34 (PD) derived recombinant inbred line (RIL) population tested with races CYR34 in greenhouse. Arrows indicate the values of the parental lines.
Figure 2. Stripe rust reactions on the leaves of resistant parent Pindong 34 (PD), susceptible parent Mingxian 169 (MX), and representative stripe rust reactions of some of the recombinant inbred lines (RILs).
Adult-plant resistance was evaluated in fields in Mianyang and Yangling from 2016 to 2020 under natural infection conditions. The susceptible parent Minxian 169 was susceptible (IT 9) at both seedling and adult-plant growth stages. The infection type of Pindong 34 was consistently 0 when tested at the adult-plant stage under field conditions, whereas Minxian 169 displayed 90–100% severity with IT=9 in all environments (Figure 3), indicating that stripe rust developed to levels adequate for collecting high-quality phenotypic data. The IT and DS values of the 119 individual RILs were continuously distributed from 0 to 9 and 0 to 100%, indicating that APR resistance in Pindong 34 was quantitatively inherited (Figure 3). In general, the ITs in Yangling were higher for most of the lines than the ITs in Mianyang (Figure 3). The correlation coefficients (r) between IT and DS were not very high between some of the 19 environments (r = 0.68–0.99, Table 1). The broad-sense heritability of both IT and DS was 0.97 (Table 2). Pairwise comparisons of the IT or DS values across years and within years were high, and all were significant (P < 0.001, Table 1). An ANOVA of IT and DS revealed significant differences (P < 0.01) in terms of the 119 RILs, 19 environments, and line × environment interactions (Table 2). No significant variations were detected among the replications within each experiment and the lines, suggesting that the expression of APR resistance was consistent across the different environments and over different years.
Figure 3. Frequency distributions of the mean infection type (IT) and disease severity (DS) for 119 RILs from the cross of Mingxian 169 (MX) × Pindong 34 (PD) grown at Mianyang (MY) and Yangling (YL) in 2016–2020. Arrows indicate the values of the parental lines.
Table 1. Correlation coefficients (r) of the mean infection type (IT) and disease severity (DS) of the Mingxian 169 × Pindong 34-derived recombinant inbred lines tested in different environments.
Table 2. Analysis of variance and estimate of broad-sense heritability of the infection type (IT) and disease severity (DS) among 119 RILs from the cross of Mingxian 169 × Pindong 34 tested at Mianyang and Yangling in 2016–2020.
A total of 11,346 SNP markers were used for linkage map construction, giving a total map length of 18,228.5 cM, with individual chromosomes ranging from 572.6 cM for chromosome 2B to 1,382.1 cM for chromosome 5B (Table 3). The number of markers per chromosome ranged from 76 for chromosome 4D to 342 for chromosome 5B, with an average number of 200 SNP markers. The average distance between neighboring SNP markers ranged from 2.5 cM/marker for chromosome 6A to 8.8 cM/marker for chromosome 7D, with an average number of 4.3 cM/marker. The map was used to identify significant associations between SNP markers and stripe rust resistance.
Table 3. Summary of chromosome assignment, number of SNP marker, map length, and marker density of the SNP genetic map of the 119 RILs from the cross of Mingxian 169 × Pindong 34.
The IT data of the 119 RILs tested with race CYR34 were used to identify QTLs for ASR. A total of eight markers representing four QTLs contributed by Pindong 34 were significantly associated with ASR, and one QTL each on 2AS, 2BL, and 7AS explained 9.69–13.58%, 9.49–12.07%, and 16.16% of the phenotypic variations in the tests with CYR34, respectively (Figure 4, Tables 4, 5). The lines without these QTLs were susceptible at the seedling stage and resistant at the adult-plant stage. The QTL QYrpd.swust-2AS, which was represented by the two closest flanking markers (IWB62645 and IWB52560) in an interval of 6.1 cM, explained 9.69–13.58% of the phenotypic variation. The QTL QYrpd.swust-2BL.4, which was flanked by markers IWB5978 and IWB62759 on chromosome 2BL with a genetic distance of 6.1 cM, explained 9.86-12.07% and 9.49-11.59% of the phenotypic variation in IT and DS, respectively. In the greenhouse test, QYrpd.swust-7AS was represented by the two closest flanking markers (IWB11337 and IWB72402) at a position of 2.6 cM on 7AS (Table 4). These three QTLs were effective against the tested race CYR34 and consistent with those determined through the ICIM-MET analysis of the RILs based on their IT for the race tests (Table 4).
Figure 4. Stripe rust resistance QTLs on the genetic map of chromosomes 1AS, 1BL, 2AS, 2BL, 3AL, 6BL, 6DL, 7AS, 7AL, and 7DL based on IT and DS data (A–M). The y-axis is in centimorgan distance (cM), and all genetic distance values shown are in the same scale; the x-axis indicates the LOD value, and all LOD values of QTL showed are in same scale except in K. The dotted line with different colors represents LOD value calculated based on the DS data from nine different environments. The solid line with different colors represents the LOD value calculated based on IT data from 10 different environments including greenhouse. The red rectangle on the genetic map indicates the corresponding QTL region.
Table 4. Summary of 15 stripe rust resistance QTLs identified using ICIM based on the mean disease severity (DS) and infection type (IT) of the 119 RILs from the cross of Mingxian 169 × Pindong 34 tested in Mianyang and Yangling in 2016–2020.
Table 5. Summary of 15 stripe rust resistance QTLs discovered using the MET for the RIL population from the cross of Mingxian 169 × Pindong 34 tested in Mianyang and Yangling in 2016–2020.
Because the RILs had various IT and DS values that did not form distinct resistance and susceptible classes, the IT and DS data of the 19 field experiments were used for QTL mapping. Inclusive composite interval mapping (ICIM) identified 12 APR QTLs on chromosome arms 1AS, 1BL, 1DL, 2AS, 2BL, 3AL, 6BL, 6DL, 7AL, and 7DL (Figure 4). All alleles for Pst resistance of the QTL located on chromosome arms were derived from Pindong 34 and designated as QYrpd.swust-1AS, QYrpd.swust-1BL, QYrpd.swust-1DL, QYrpd.swust-2BL.1, QYrpd.swust-2BL.2, QYrpd.swust-2BL.3, QYrpd.swust-3AL.1, QYrpd.swust-3AL.2, QYrpd.swust-6BL, QYrpd.swust-6DL, QYrpd.swust-7AL, and QYrpd.swust-7DL, and they were significant in some of the tests for both IT and DS (P < 0.005, LOD > 2.5). The lines containing these QTLs were susceptible to stripe rust at the seedling stage and resistant at the adult-plant stage. Table 4 presents a summary of the IT and DS effects of the individual QTLs across the 2016–2020 seasons in this study.
Among the 12 QTLs detected for APR resistance, QYrpd.swust-1BL, QYrpd.swust-3AL.1, QYrpd.swust-3AL.2, QYrpd.swust-6BL, QYrpd.swust-6DL, QYrpd.swust-7AL, and QYrpd.swust-7DL were significantly more abundant than in the five of the tested environments in terms of both IT and DS (P < 0.05, LOD > 2.5) (Tables 4, 5). QYrpd.swust-1BL explained an average of 9.24–13.33% of the phenotypic variation in IT and DS across the six environments of Mianyang and Yangling. This QTL was located within 7.0 cM between markers IWB42604 and IWB10630 on the long arm of chromosome 1B. QYrpd.swust-3AL.1 was represented by the two closest flanking markers (IWB34554 and IWB3508) at a genetic distance of 5.1 cM on 3AL, and it explained 11.41–14.80% of the IT and DS phenotypic variation in five environments. QYrpd.swust-3AL.2 was located in an interval of 7 cM between IWA95 and IWB13994 on chromosome 3AL, and it explained 11.54–17.0% of the IT and DS phenotypic variation in five environments. QYrpd.swust-6BL, which was flanked by markers IWB41242 and IWB21972 on chromosome 6BL with a genetic distance of 7.8 cM, explained 9.39–12.78% of the phenotypic variation in IT and DS in one of the six environments. QYrpd.swust-6DL explained 9.52% and 16.36% of the phenotypic variation of IT and DS on average, respectively, across nine environments. This QTL was located within a 9.1 cM interval between markers IWB71500 and IWA5986 on the long arm of chromosome 6D. QYrpd.swust-7AL explained 9.09–17.0% of the phenotypic variance depending upon the experiment, and the closest markers were IWB796 and IWA5986, with a genetic distance of 22.0 cM in eight environments. QYrpd.swust-7DL was flanked by markers IWB13547 and IWB14996 on chromosome 7DL with a genetic distance of 4.2 cM, and it explained 8.87–11.38% of the phenotypic variation in IT and DS in five environments (Table 4, Figure 4).
The remaining QTLs, including QYrpd.swust-1AS, QYrpd.swust-1DL, QYrpd.swust-2BL.1, QYrpd.swust-2BL.2, and QYrpd.swust-2BL.3, were significant in at least three environments and explained 11.05–12.72%, 9.81–13.05, 9.69–10.57%, 10.36–12.97%, and 9.54–13.15% of the phenotypic variation, respectively (Table 5). QYrpd.swust-1AS was located in an interval of 2.3 cM and flanked by markers IWB22569 and IWB6621 on chromosome 1AS, and QYrpd.swust-1DL was located in an interval of 2.2 cM and flanked markers IWB59384 and IWB27489 on chromosome 1DL. QYrpd.swust-2BL.1, QYrpd.swust-2BL.2, and QYrpd.swust-2BL.3 were located in three intervals of 0.9 cM, 6.5 cM, and 2.8 cM and flanked by markers IWB27661–IWB61115, IWB44066–IWB30421, and IWB7605–IWB25791, respectively. These three QTLs were present on the long arm of the 2B chromosome.
The ICIM-EPI scan for digenic epistatic QTLs within the MET function yielded 48 marker pairs with LOD scores > 10 and 22 pairs with LOD scores > 20. Only two of the 2 × 15 markers were located near a monogenic QTL (Table 6).
Table 6. Multi-environmental analysis and digenic epistatic QTLs based on an ICIM-EPI analysis of the mean infection type (IT) and disease severity (DS) of the RILs from the cross of Mingxian 169 × Pindong 34 tested in Mianyang and Yangling in 2016–2020.
To determine the effects of QTLs in various combinations for Pst resistance, the 119 RILs were classified into genotypic groups based on the presence of markers closely associated with the 15 QTLs (Table 4). These genotypes were further sorted into 13 groups based on the number of potential QTLs for Pst resistance. Figure 5 shows the differences in the mean IT and DS values of the 13 groups. In general, there were significant additive effects for stripe rust resistance in some RIL lines, and all QTL combinations were significantly different (P < 0.05) from the RILs with no resistance alleles. When a few QTLs were combined, the average IT and DS values were lower than those with only one or none (Figure 5), which is supported by the significant additive effects (P < 0.01) obtained by the QTL analysis.
Figure 5. Effects of individual QTLs and their combinations on stripe rust scores illustrated by the mean infection type (IT) and disease severity (DS) scores of the RILs from the cross of Mingxian 169 × Pindong 34 in combined environments (MY and YL). The box plots indicate the infection type (IT) and disease severity (DS) associated with the identified QTL and their combination. The resistance alleles present in the group are indicated by the name of the chromosome where the QTL is located. The number of RILs grouped in each class is indicated in parentheses by each class name. Error bars represent ± 1 SEM.
The wheat cultivar Pindong 34 has maintained good resistance to stripe rust under field conditions since it was initially bred in 1990. In 2014, Zhou identified and performed molecular mapping of its all-stage resistance gene Yr61 by employing bulk segregant analysis in combination with the resistance gene analog polymorphism (RGAP), sequence-tagged site (STS), and simple sequence repeat (SSR) genotyping methods in the F2 and F2:3 populations. In this study, a 90K SNP-chip genotyping assay and RIL population containing 119 lines were used to identify and analyze all-stage and adult-plant resistance, and a total of 12 QTLs for APR resistance together with 3 QTLs for all-stage resistance were obtained on 10 different chromosomes encompassing 15 regions. Compared to previous studies that performed QTL mapping, the results showed the highest number of QTLs for Pst resistance in a single wheat cultivar, which also explained why the cultivar Pingdong 34 could maintain a high level of field resistance for the long term.
QYrpd.swust-1AS is derived from Pingdong 34, and it was flanked by markers IWB22569 and IWB6621 and corresponds to the 1,174,937–3,256,249 bp region on chromosome 1AS of CS. It explained 11.05–12.72% of the phenotypic variation in five experimental environments. To date, some genes/quantitative trait loci (QTL) regions have been reported for stripe rust resistance on chromosome 1AS, and five of them were located on the short arm of chromosome 1AS: YrTtd-1AS (Liu et al., 2017), QYr.tam-1A.1 (Basnet et al., 2014a), QYrus.vt-1AS (Christopher et al., 2013), QYrMa.wgp-1AS (Liu et al., 2018), and QYr.cim-1AS (Lan et al., 2015). The gene YrTtd-1AS and QTLs QYrMa.wgp-1AS and QYr.cim-1AS are all-stage resistance (ASR) genes; therefore, they cannot be identical to QYrpd.swust-1AS, which is an adult-plant resistance gene (Lan et al., 2015; Liu et al., 2017, 2018). QYr.tam-1A.1 confers APR resistance against stripe rust, although it is a minor QTL (Basnet et al., 2014a). Based on the relative marker positions, the QTL QYrpd.swust-1AS in the current study differs from the resistance genes previously identified.
QYrpd.swust-1BL was located between markers IWB42604 and IWB10630 on the long arm of chromosome 1B and was mapped to the 520,810,752 bp−520,926,056 bp region of chromosome 1BL of the CS genome. To date, the number of QTLs/genes for stripe rust resistance on chromosome 1BL has been reported to be more than 10 based on the genetic populations and GWAS analysis, and they include YrChk (Liu et al., 2007), YrExp1 (Lin and Chen, 2008), YrP138 (Yue et al., 2010), YrTtd-1BL (Liu et al., 2017), QYr.cimmyt-1BL (William et al., 2006), and YrN.S-2 (Li et al., 2010), which confer all-stage resistance, and QYrPI181410.wgp-1BL (Liu et al., 2020), QYrdr.wgp-1BL.1 (Hou et al., 2015), QYrdr.wgp-1BL.2 (Hou et al., 2015), and QYrex.wgp-1BL (Lin and Chen, 2009), which confer HTAP resistance. The QTL QYrpd.swust-1BL identified in the present study confers adult resistance. Further study is needed to confirm whether the resistance of QYrpd.swust-1BL is dependent on temperature and to investigate the relationships between QYrpd.swust-1BL and the above HTAP genes. Yr29 (William et al., 2006), QYr.tam-1B (Basnet et al., 2014a), QYr.cim-1BL (Lan et al., 2014), QYrTtd-1BL (Liu et al., 2017), QPst.jic-1B (Melichar et al., 2008), and QYr.ucw-1BL (Cobo et al., 2018) all confer APR resistance. Yr29, a permanently designated gene for resistance to wheat stripe rust in wheat cultivar 'Pavon76,' was linked with SSR markers Xwmc44 and Xwmc367 and located at the distal end of chromosome 1BL. QYr.tam-1B, a QTL derived from the common wheat cultivar ‘Quaiu 3,' was linked with the markers wPt-668027 and cSLV46G22. QYr.cim-1BL, derived from wheat Francolin#1, was mapped between markers csLV46 and wPt-9028. QYrTtd-1BL, a QTL for resistance to wheat stripe rust in emmer wheat (Triticum turgidum ssp. dicoccum) was flanked by SNP marker IWB69464. QPst.jic-1B, a QTL derived from UK winter wheat cultivar Guardian, was flanked with the SSR markers Xgwm259 and Xgwm818. QYr.ucw-1BL was mapped to the SNP marker IWA8581 and derived from the wheat cultivar Klein Chajá. QYrsv.swust-1BL.1 was mapped within a 0.75-cM region in T. turgidum subsp. durum 'Svevo' on chromosome lBL, corresponding to the region of 670.7 to 671.5 Mb on the Chinese Spring chromosome 1BL. According to the resistance types and the physical location of molecular markers, it can be preliminarily inferred that the APR QTL QYrpd.swust-1BL identified in this study is different from the APR QTLs identified in previous studies.
QYrpd.swust-1DL was flanked by IWB59384 and IWB27489 and explained 9.81–13.05% of the phenotypic variation in four environments. The QYrpd.swust-1DL region was located in the physical region of 378,867,221 bp−379,141,846 bp. The permanently designated gene Yr25 was reported on chromosome 1D in some Pst races in other countries, although it has been largely ineffective in the Pst population in the US, and its specific chromosomal location is unknown (Calonnec and Johnson, 1998). There are some temporarily named genes for stripe rust reported on 1DL, e.g., QYrww.wgp.1D-3, a QTL for resistance to wheat stripe rust flanked by SNP marker IWA3446 and physically located at approximately 2,331,833 bp on 1DL, which differed from the location of QYrpd.swust-1DL (Tian et al., 2011).
QYrpd.swust-2AS was detected for all-stage resistance and flanked by IWB62645 and IWB52560. It explained 9.69–13.58% of the phenotypic variation in eight environments. Officially and temporarily designated Yr genes for resistance to wheat stripe rust reported on 2AS included Yr17 (Jia et al., 2011) and YrR61 (Hao et al., 2011). Yr17 from Aegilops tauschii conferred seedling (all-stage) resistance and was mapped to the distal end of chromosome arm 2AS, with the closest SSR markers Xgwm636 and Xgwm359 (Jia et al., 2011). YrR61, derived from soft red winter wheat Pioneer 26R61, was flanked by markers Xbarc124 and Xgwm359, and assigned to the distal 22% of the short arm of wheat chromosome 2A (Hao et al., 2011), such as QYrTtd-2AS (Liu et al., 2017), QYrww.wgp.2A-1 (Mu et al., 2020), Qyr.wpg-2A.2, Qyr.wpg-2A.3, and Qyr.wpg-2A.4 (Naruoka et al., 2015), while APR genes on the short arm of chromosome 2A included YrSph (Chen et al., 2012), QYrPI182103.wgp-2AS (Feng et al., 2018), and QYrMa.wgp-2AS (Liu et al., 2018); therefore, these QTLs cannot be identical to QYrpd.swust-2AS. On the short arm of 2A, most QTLs that confer ASR were in the distal region of QYrpd.swust-2AS, such as Yr69 (Hou et al., 2016) and YrSph (Chen et al., 2012). Yr56 confers ASR in the wheat cultivar Wollaroi (AUS99174) and was flanked by the closed SSR markers Xsun167 and Xsun168 (Bansal et al., 2014). QYrMa.wgp-2AS confers ASR in the winter wheat cultivar Madsen, and it was flanked with the closed SNP marker IWB35714. Based on the origin, disease resistance type, and linked markers, QYrzv.swust-2AS is unlikely to represent the previously reported QTL.
Three APR resistance QTLs, QYrpd.swust-2BL.1, QYrpd.swust-2BL.2, and QYrpd.swust-2BL.3, and one ASR QTL, QYrpd.swust-2BL.4, were identified on the long arm of chromosome 2B. QYrpd.swust-2BL.1 was located between markers IWB27661 and IWB61115 and mapped to the 773,785,836 bp−775,169,437 bp region of the CS genome on chromosome 2BL. QYrpd.swust-2BL.2 was located between markers IWB44066 and IWB30421 and mapped to the 775,368,259 bp−777,519,972 bp region of the CS genome on chromosome 2BL. QYrpd.swust-2BL.3 was located between markers IWB7605 and IWB25791 and mapped to the 793,151,400 bp−797,995,314 bp region of the CS genome on chromosome 2BL. QYrpd.swust-2BL.4 was located between markers IWB5978 and IWB62759 and mapped to the 782,534,687 bp−784,551,415 bp region of the CS genome on chromosome 2BL. To date, more than 20 genes and QTLs have been reported on chromosome 2BL based on genetic populations and GWAS analysis. QYr.caas-2BL.2 (Ren et al., 2015), QYrPI181410.wgp-2BL (Liu et al., 2020), and QYrdr.wgp-2BL (Hou et al., 2015) code for completely different types of resistance relative to the four QTLs in the present study. Based on the physical position in the durum genome, some genes and QTL loci are completely different from the QYrpd.swust-2BL.1, QYrpd.swust-2BL.2, QYrpd.swust-2BL.3, and QYrpd.swust-2BL.4 loci. These include Yr7 (Macer, 1963), Yr43 (Cheng and Chen, 2010), Yr44 (Sui et al., 2009), Yr53 (Xu et al., 2013), YrQz (Deng et al., 2004), YrSP (Feng et al., 2015b), YrSte (Chen et al., 1996), YrV23 (Yr3a) (Wang et al., 2006), YrTtd-2BL.1 and YrTtd-2BL.2 (Liu et al., 2017), QYr-2B (Boukhatem et al., 2002), QYr.tam-2BL (Basnet et al., 2014b), QYr.nafu-2BL (Zhou et al., 2015), QYrns.orz-2BL (Vazquez et al., 2015), QYrww.wgp.2B-4 (Mu et al., 2020), QYrdr.wgp-2BL (Hou et al., 2015), QYr.csiro-2BL (Rosewarne et al., 2008), QYR1 (Boukhatem et al., 2002), QYr.inra-2BL (Mallard et al., 2005), QYrcaas-2BL (Ren et al., 2012), and QYraq.cau-2BL (Ramburan et al., 2004).
Two QTLs were identified on the long arm of chromosome 3A. QYrpd.swust-3AL.1 was located between markers IWB34554 and IWB3508 and mapped to 731,222,152 bp−732,779,714 bp of the CS chromosome 3AL, and it confers APR resistance. QYrpd.swust-3AL.2 was located between markers IWA95 and IWB13994 and mapped to the 724,201,099 bp−725,738,951 bp of CS chromosome 3AL, and it confers APR resistance. The two QTLs YrTr2 (Chen and Line, 1992) and QYrne.vt-3A (Christopher et al., 2013) were mapped to chromosome 3A, although their specific chromosomal location is unknown. Other QTLs are mapped on the long arm of chromosome 3A, such as QYrdr.wpg-3AL, which confers HTAP resistance derived from the winter wheat cultivar Druchamp (Hou et al., 2015), are likely different from QYrpd.swust-3AL.1 and QYrpd.swust-3AL.2. QYrPI182103.wgp-3AL (Feng et al., 2018), YrTtd-3AL (Liu et al., 2017), and QYrww.wgp-3A (Mu et al., 2020) confer all-stage resistance, and QYrPI182103.wgp-3AL was linked with markers IWA899 and Xgwm2 and derived from spring wheat PI 182103. QTL YrTtd-3AL was linked with SNP marker IWB71901 and derived from emmer wheat (Triticum turgidum ssp. dicoccum). QTL QYrww.wgp.3A was linked with marker IWB44443 (Mu et al., 2020), and QTL QYrst.orr-3AL was linked with marker wPt1652 and derived from spring wheat Stephens (Vazquez et al., 2012). Based on the disease resistance type and linked markers, QYrpd.swust-3AL.1 and QYrpd.swust-3AL.2 are unlikely to be the above previously reported QTLs.
QYrpd.swust-6BL was located between markers IWB41242 and IWB21972 on the long arm of chromosome 6B and mapped to the 423,190,456 bp−651,418,439 bp region of the CS genome on chromosome 6BL, and it confers APR resistance. To date, more than nine QTLs/genes for stripe rust resistance have been reported on chromosome 6BL by genetic populations and GWAS analysis. For example, YrLM168a (derived from wheat resistance line LM168a, nearest flanking markers Xwmc756 and Xbarc146, Feng et al., 2015a) confers all-stage resistance and QYrdr.wgp-6BL.2 (derived from winter wheat Druchamp, most closely associated with SNP marker IWA6420) confers HTAP resistance (Hou et al., 2015). QYrdurum-6BL was derived from durum wheat and was linked with the SNP marker IWB72946 (Liu et al., 2017). The QTL derived from wheat cultivar Pastor conferred APR resistance and was mapped between markers XwPt-6329 and XwPt-5176 on chromosome 7BL (Rosewarne et al., 2012). QYrww.wgp.6B was linked with the SNP marker IWA1017 (Mu et al., 2020), and QYr.tam-6B originated from the wheat cultivar Quaiu and was linked with markers wpt-0171 and wpt-4164 (Basnet et al., 2014b). QYr.wsu-6B.2 and QYr.wsu-6B.3 (Bulli et al., 2016) were linked by XIWA4169 and XIWA349, respectively. The two QTLs Qyr.wpg-6B.1 and Qyr.wpg-6B.2 were detected on the long arm of 6B and linked with markers IWA7257 and IWA3222, respectively (Naruoka et al., 2015). QYr.ucw-6B_IWA7257 was identified with the linked marker XIWA7257 (Maccaferri et al., 2015). Based on the linked marker origins and disease resistance type, QYrpd.swust-6BL is unlikely to be the above previously reported QTLs.
QYrpd.swust-6DL was flanked by IWB71500 and IWA5986 on chromosome 6DL with a genetic distance of 9.1 cM, and it explained 9.52–16.36% of the phenotypic variation in nine environments in the BIP analysis and conferred APR resistance. The QYrpd.swust-6DL region was located in the 576,040,835 bp−72,231,237 bp region of the CS 6DL chromosome. The two genes Yr20 (Chen et al., 1995a,b) and Yr23 (Chen et al., 1995a,b) were also mapped on chromosome 6DL and conferred ASR, which was different from the resistance type of QYrpd.swust-6DL. Other QTLs mapped on the long arm of chromosome 6D, such as YrH9020a (flanking markers Xbarc202 and Xbarc96), which conferred APR resistance, were derived from wild relative P. huashanica H9020-1-6-8-3 (Liu et al., 2014). QYr.sicau-6D was linked with marker Xcfd95-2. QYr.ufs-6D was mapped based on the SSR markers Xgwm325 and Xbarc175 and is located in the 79,956,573 bp−79,956,710 bp region of the CS 6DL chromosome. QYr.sicau-6D overlapped with QYr.ufs-6D on chromosome 6D, and both the two QTLs conferred APR resistance (Agenbag et al., 2012; Yao et al., 2019). Based on the resistance type and linkage markers, QYrpd.swust-6DL is probably different from the above QTLs.
A QTL that conferred ASR was identified on the short arm of chromosome 7A. QYrpd.swust-7AS was located between markers IWB11337 and IWB72402 and mapped to the 18,877,040 bp−20,172,969 bp region of the CS genome on the chromosome 7AS. To date, only one formally named Yr gene, Yr61, has been reported on chromosome 7AS, and it confers all-stage resistance. Yr61 originated from the winter wheat cultivar Pindong 34, conferred all-stage resistance, and was located between STS markers Xwgp5765b and Xwgp5467 on the short arm of 7A (Zhou et al., 2014). The two STS markers could also be detected in the lines containing the QTL QYrpd.swust-7AS. YrTtd-7AS* was linked with SNP marker IWB61392 and derived from the Chinese cultivar emmer wheat (Triticum turgidum ssp. dicoccum) (Liu et al., 2017), and it was mapped to the 14,814,722 bp−14,814,722 bp region. Other previously reported QTLs, such as QYr.caas-7AS (Ren et al., 2012), QYrdurum-7AS.1, QYrdurum-7AS.2, QYrdurum-7AS.3 (Liu et al., 2017), QYrww.wgp.7A-1, QYrww.wgp.7A-2 (Mu et al., 2020), QYr.cim-7AS (Rosewarne et al., 2012), and QYrst.orr-7AS (Vazquez et al., 2012), were identified on the short arm of chromosome 7A, and they all conferred APR resistance; therefore, they were different from QYrpd.swust-7AS. As a result, QYrpd.swust-7AS is likely to be the previously reported QTL Yr61 based on the origin, effect, resistance type, and linked markers. The relationship between QYrpd.swust-7AS derived from Pindong 34 and the previously reported QTL Yr61 in the region was detected by the two STS markers, which proved the locus was Yr61.
The QTL identified in the present study, QYrpd.swust-7AL, confers APR resistance and was located between markers IWB796 and IWB14178 (region of 635,495,960 bp−708,137,655 bp) on the long arm of chromosome 7A. Several QTLs have also been previously reported on chromosome 7AL. A locus conferring ASR was controlled by a major gene, Yrzhong12-1, located in the interval of SSR markers Xcfd20 (3.1 cM) and Xbarc121 (4.9 cM) on chromosome 7AL (Ma et al., 2015). Kanwal et al. (2021) identified the adult-plant stripe rust resistance gene Yr75 in the Australian wheat cultivar Axe and mapped the gene on the long arm of chromosome 7A, and the two SNP markers sunKASP_430 and sunKASP_427 were closely linked to the gene.
QYrpd.swust-7DL was located between markers IWB13547 and IWB14996 on the long arm of chromosome 7D and mapped to the 432,435,291 bp−436,968, 553 bp region of the CS genome on chromosome 7DL. To date, only one formally named Yr gene, Yr33, has been reported on chromosome 7DL (Zahravi et al., 2003). Yr33 conferred all-stage resistance and was located between markers Xwgm11 and Xgwm437. The QYr.tam-7D locus was identified by markers wPt730042 and wPt730455, and it conferred HTAP resistance and was derived from wheat TAM 111 (Basnet et al., 2014a). QYr.sicau-7D conferred APR resistance and was located in the QTL region between 4,440,148 bp and 3,937,237 bp (Long et al., 2019). The relationship between QYrpd.swust-7DL derived from Pindong 34 and QYr.sicau-7D need to be further tested.
Separately, the identified QTLs could not account for the high resistance found in Pindong 34 based on the BIP analysis, which scanned each environment. The MET analysis pointed to additional QTLs that were the same as those detected by the BIP analysis. These QTLs were found due to the high power of multi-environmental analysis, which can detect QTLs (Li et al., 2015). The discovery of 14 marker pairs that show digenic epistatic QTLs (LOD > 15) can complete the picture and explain how Pindong 34 maintains immunity to stripe rust while none of the RILs exhibited this resistance level. The complexity of the genetics underlying Pindong 34 resistance will complicate the transfer of its resistance to other cultivars. Genomic selection methods might help.
The original contributions presented in the study are included in the article, further inquiries can be directed to the corresponding authors.
XZ constructed the RIL population, conducted the experiments, analyzed data, and wrote the manuscript. XL participated in phenotypic and genotypic analyses of the RIL population. DH made the cross and review the manuscript. SY conducted the experiments, analyzed the data, and reviewed the manuscript. ZK conceived the study and reviewed the manuscript. RR analyzed data and wrote the manuscript. All authors approved the final version of the manuscript.
This study was financially supported by the Key Research and Development Program of International Science and Technology Innovation Cooperation of Science and Technology Department of Sichuan Province, China (No. 2022YFH0032), and was partially funded by the National Natural Science Foundation of China (No. 32101707), Breakthrough in Wheat Breeding Material and Method Innovation and New Variety Breeding (Breeding Research Project, 2021YFYZ0002), PhD Foundation of Southwest University of Science and Technology (No. 18zx7159, 16zx7162), and Longshan Academic Talent Research Support Program of SWUST (No. 17LZX5).
The authors declare that the research was conducted in the absence of any commercial or financial relationships that could be construed as a potential conflict of interest.
All claims expressed in this article are solely those of the authors and do not necessarily represent those of their affiliated organizations, or those of the publisher, the editors and the reviewers. Any product that may be evaluated in this article, or claim that may be made by its manufacturer, is not guaranteed or endorsed by the publisher.
Agenbag, G. M., Pretorius, Z. A., Boyd, L. A., Bender, C. M., and Prins, R. (2012). Identification of adult plant resistance to stripe rust in the wheat cultivar Cappelle-Desprez. Theor. Appl. Genet. 125, 109–120. doi: 10.1007/s00122-012-1819-5
Bansal, U. K., Kazi, A. G., Singh, B., Hare, R. A., and Bariana, H. S. (2014). Mapping of durable stripe rust resistance in a durum wheat cultivar Wollaroi. Mol. Breed. 33, 51–59. doi: 10.1007/s11032-013-9933-x
Basnet, B. R., Ibrahim, A. M. H., Chen, X. M., Singh, R. P., Mason, E. R., Bowden, R. L., et al. (2014a). Molecular mapping of stripe rust resistance in hard red winter wheat TAM 111 adapted to the U.S. high plains. Crop Sci. 54, 1361–1373. doi: 10.2135/cropsci2013.09.0625
Basnet, B. R., Singh, R. P., Ibrahim, A. M. H., Herrera-Foessel, S. A., Huerta-Espino, J., Lan, C., et al. (2014b). Characterization of Yr54 and other genes associated with adult plant resistance to yellow rust and leaf rust in common wheat Quaiu 3. Mol. Breed. 33, 385–399. doi: 10.1007/s11032-013-9957-2
Boukhatem, N., Baret, P. V., Mingeot, D., and Jacquemin, J. M. (2002). Quantitative trait loci for resistance against yellow rust in two wheat-derived recombinant inbred line populations. Theor. Appl. Genet. 104, 111–118. doi: 10.1007/s001220200013
Bulli, P., Zhang, J. L., Chao, S. M., Chen, X. M., and Pumphrey, M. (2016). Genetic architecture of resistance to stripe rust in a global winter wheat germplasm collection. G3-Genes Genom. Genet. 6, 2237–2253. doi: 10.1534/g3.116.028407
Calonnec, A., and Johnson, R. (1998). Chromosomal location of genes for resistance to Puccinia striiformis in the wheat line TP1295 selected from the cross of Soissonais-Desprez with Lemhi. Eur. J. Plant Pathol. 104, 835–847. doi: 10.1023/A:1008660904975
Chen, S. S., Chen, G. Y., Chen, H., Wei, Y. M., Li, W., Liu, Y. X., et al. (2012). Mapping stripe rust resistance gene YrSph derived from Tritium sphaerococcum Perc. with SSR, SRAP, and TRAP markers. Euphytica 185, 19–26. doi: 10.1007/s10681-011-0593-9
Chen, X. M. (2005). Epidemiology and control of stripe rust Puccinia striiformis f. sp tritici on wheat. Can. J. Plant Pathol. 27, 314–337. doi: 10.1080/07060660509507230
Chen, X. M. (2013). Review article: high-temperature adult-plant resistance, key for sustainable control of stripe rust. Am. J. Plant Sci. 4, 608–627. doi: 10.4236/ajps.2013.43080
Chen, X. M. (2020). Pathogens which threaten food security: puccinia striiformis, the wheat stripe rust pathogen. Food Sec. 12, 239–251. doi: 10.1007/s12571-020-01016-z
Chen, X. M., Jones, S., and Line, R. F. (1995a). Chromosomal location of genes for stripe rust resistance in spring wheat cultivars Compair, Fielder, Lee, and Lemhi and interactions of aneuploid wheats with races of Puccinia striiformis. Phytopathology 85, 375–381. doi: 10.1094/Phyto-85-375
Chen, X. M., Jones, S. S., and Line, R. F. (1996). Chromosomal location of genes for resistance to Puccinia striiformis in seven wheat cultivars with resistance genes at the Yr3 and Yr4 loci. Phytopathology 86, 1228–1233. doi: 10.1094/Phyto-86-1228
Chen, X. M., and Line, R. F. (1992). Genes for resistance to stripe rust in 'Tres' wheat. Crop Sci. 32, 692–696. doi: 10.2135/cropsci1992.0011183X003200030024x
Chen, X. M., Line, R. F., and Jones, S.S. (1995b). Chromosomal location of genes for resistance to Puccinia striiformis in winter wheat cultivars Heines VII, Clement, Moro, Tyee, Tres, and Daws. Phytopathology 85, 1362–1367. doi: 10.1094/Phyto-85-1362
Cheng, P., and Chen, X. M. (2010). Molecular mapping of a gene for stripe rust resistance in spring wheat cultivar IDO377s. Theor. Appl. Genet. 121, 195–204. doi: 10.1007/s00122-010-1302-0
Christopher, M. D., Liu, S. Y., Hall, M. D., Marshall, D. S., Fountain, M. O., Johnson, J. W., et al. (2013). Identification and mapping of adult plant stripe rust resistance in soft red winter wheat VA00W-38. Crop Sci. 53, 871–879. doi: 10.2135/cropsci2012.02.0086
Cobo, N., Pfluger, L., Chen, X. M., and Dubcovsky, J. (2018). Mapping QTL for resistance to new virulent races of wheat stripe rust from two argentinean wheat cultivars. Crop Sci. 58, 2470–2483. doi: 10.2135/cropsci2018.04.0286
Deng, Z. Y., Zhang, X. Q., Wang, X. P., Jing, J. K., and Wang, D. W. (2004). Identification and molecular mapping of a stripe rust resistance gene from a common wheat line Qz180. Acta Bot. Sin. 46, 236–241. doi: 10.1614/WS-03-091R
Feng, J. Y., Chen, G. Y., Wei, Y. M., Liu, Y. X., Jiang, Q. T., Li, W., et al. (2015a). Identification and mapping stripe rust resistance gene YrLM168a using extreme individuals and recessive phenotype class in a complicate genetic background. Mol. Genet. Genom. 290, 2271–2278. doi: 10.1007/s00438-015-1077-8
Feng, J. Y., Wang, M. N., Chen, X. M., See, D. R., Zheng, Y. L., Chao, S. M., et al. (2015b). Molecular mapping of YrSP and its relationship with other genes for stripe rust resistance in wheat chromosome 2BL. Phytopathology 105, 1206–1213. doi: 10.1094/PHYTO-03-15-0060-R
Feng, J. Y., Wang, M. N., See, D. R., Chao, S. M., Zheng, Y. L., and Chen, X. M. (2018). Characterization of novel gene Yr79 and four additional quantitative trait loci for all-stage and high-temperature adult-plant resistance to stripe rust in spring wheat PI 182103. Phytopathology 108, 737–747. doi: 10.1094/PHYTO-11-17-0375-R
Hao, Y. F., Chen, Z. B., Wang, Y. Y., Bland, D., Buck, J., Brown-Guedira, G., et al. (2011). Characterization of a major QTL for adult plant resistance to stripe rust in US soft red winter wheat. Theo. Appl. Genet. 123, 1401–1411. doi: 10.1007/s00122-011-1675-8
Hou, L., Chen, X. M., Wang, M. N., See, D. R., Chao, S. M., Bulli, P., et al. (2015). Mapping a large number of qtl for durable resistance to stripe rust in winter wheat druchamp using SSR and SNP markers. PLoS ONE 10, e0126794. doi: 10.1371/journal.pone.0126794
Hou, L. Y., Jia, J. Q., Zhang, X. J., Li, X., Yang, Z. J., Ma, J., et al. (2016). Molecular mapping of the stripe rust resistance gene Yr69 on wheat chromosome 2AS. Plant Dis. 100, 1717–1724. doi: 10.1094/PDIS-05-15-0555-RE
Jia, J. Q., Li, G. R., Liu, C., Lei, M. P., and Yang, Z. J. (2011). Characterization of wheat yellow rust resistance gene Yr17 using EST-SSR and rice syntenic region. Cereal Res. Commun. 39, 88–99. doi: 10.1556/CRC.39.2011.1.9
Kanwal, M., Qureshi, N., Gessese, M., Forrest, K., Babu, P., Bariana, H., et al. (2021). An adult plant stripe rust resistance gene maps on chromosome 7A of Australian wheat cultivar Axe. Theor. Appl. Genet. 134, 2213–2220.
Kosambi, D. D. (2016). The Estimation of Map Distances From Recombination Values. DD Kosambi. New Delhi: Springer. 125–130.
Lan, C., Rosewarne, G. M., Singh, R. P., Herrera-Foessel, S. A., and Yang, E. (2014). QTL characterization of resistance to leaf rust and stripe rust in the spring wheat line Francolin#1. Mol. Breed. 34, 789–803. doi: 10.1007/s11032-014-0075-6
Lan, C. X., Zhang, Y. L., Herrera-Foessel, S. A., Basnet, B. R., Huerta-Espino, J., Lagudah, E. S., et al. (2015). Identification and characterization of pleiotropic and co-located resistance loci to leaf rust and stripe rust in bread wheat cultivar Sujata. Theor. Appl. Genet. 128, 549–561. doi: 10.1007/s00122-015-2454-8
Li, J. L., Wang, S., Yu, J., Wang, L., and Zhou, S. L. (2013). A modified CTAB protocol for plant DNA extraction. Chin. Bull. Bot. 48, 72–78. doi: 10.3724/SP.J.1259.2013.00072
Li, Q. A., Hu, M. L., Chen, J., Jing, J. X., Wang, B. T., and Zhou, X. C. (2010). Inheritance and molecular mapping of genes for all-stage resistance to stripe rust in wheat cultivar N. Strampelli. Can. J. Plant Sci. 90, 529–536. doi: 10.4141/CJPS09076
Li, S. S., Wang, J. K., and Zhang, L. Y. (2015). Inclusive composite interval mapping of QTL by environment interactions in biparental populations. PLoS ONE 10, e0132414. doi: 10.1371/journal.pone.0132414
Lin, F., and Chen, X. M. (2008). Molecular mapping of genes for race-specific overall resistance to stripe rust in wheat cultivar Express. Theor. Appl. Genet. 116, 797–806. doi: 10.1007/s00122-008-0713-7
Lin, F., and Chen, X. M. (2009). Quantitative trait loci for non-race-specific, high-temperature adult-plant resistance to stripe rust in wheat cultivar Express. Theor. Appl. Genet. 118, 631–642. doi: 10.1007/s00122-008-0894-0
Line, R. F., and Qayoum, A. (1992). Virulence, aggressiveness, evolution and distribution of races of Puccinia striiformis (the cause of stripe rust of wheat) in North America, 1968–87. Technical bulletin-United States Department of Agriculture, (1788).
Liu, F., Niu, Y., Deng, H., and Tan, G. (2007). Mapping of a major stripe rust resistance gene in Chinese native wheat variety chike using microsatellite markers. J. Genet. Genom. 34, 1123–1130. doi: 10.1016/S1673-8527(07)60128-3
Liu, L., Wang, M. N., Feng, J. Y., See, D. R., Chao, S. M., and Chen, X. M. (2018). Combination of all-stage and high-temperature adult-plant resistance QTL confers high-level, durable resistance to stripe rust in winter wheat cultivar Madsen. Theor. Appl. Genet. 131, 1835–1849. doi: 10.1007/s00122-018-3116-4
Liu, W. Z., Maccaferri, M., Bulli, P., Rynearson, S., Tuberosa, R., Chen, X. M., et al. (2017). Genome-wide association mapping for seedling and field resistance to Puccinia striiformis f. sp tritici in elite durum wheat. Theor. Appl. Genet. 130, 649–667. doi: 10.1007/s00122-016-2841-9
Liu, Y., Qie, Y. M., Li, X., Wang, M. N., and Chen, X. M. (2020). Genome-wide mapping of quantitative trait loci conferring all-stage and high-temperature adult-plant resistance to stripe rust in spring wheat landrace PI 181410. Int. J. Mol. Sci. 21,478. doi: 10.3390/ijms21020478
Liu, Z. G., Yao, W. Y., Shen, X. X., Chao, K. X., Fan, Y., Li, M. Z., et al. (2014). Molecular mapping of a stripe rust resistance gene YrH9020a transferred from psathyrostachys huashanica keng on wheat chromosome 6D. J. Integr. Agric. 13, 2577–2583. doi: 10.1016/S2095-3119(14)60755-3
Long, L., Yao, F., Yu, C., Ye, X., Cheng, Y., Wang, Y., et al. (2019). Genome-wide association study for adult-plant resistance to stripe rust in Chinese wheat landraces (Triticum aestivum L.) from the yellow and huai river valleys. Front. Plant Sci. 10, 596. doi: 10.3389/fpls.2019.00596
Ma, D. F., Peng, F., Fang, Z. W., Chao, K. X., Jing, J. X., and Zhang, C. Q. (2015). Genetic and molecular mapping of stripe rust resistance genes in wheat cultivar Zhongliang 12. J. Phytopathol. 163, 98–104. doi: 10.1111/jph.12284
Maccaferri, M., Zhang, J. L., Bulli, P., Abate, Z., Chao, S. M., Cantu, D., et al. (2015). A genome-wide association study of resistance to stripe rust (Puccinia striiformis f. sp tritici) in a worldwide collection of hexaploid spring wheat (Triticum aestivum L.). G3-Genes Genom. Genet. 5, 449–465. doi: 10.1534/g3.114.014563
Macer, R. (1963). “The formal and monosomic genetic analysis of stripe rust (Puccinia striiformis) resistance in wheat,” in Proceedings of the 2nd International Wheat Genetics Symposium. (Lund, Sweden), 127–142.
Mallard, S., Gaudet, D., Aldeia, A., Abelard, C., Besnard, A. L., Sourdille, P., et al. (2005). Genetic analysis of durable resistance to yellow rust in bread wheat. Theor. Appl. Genet. 110, 1401–1409. doi: 10.1007/s00122-005-1954-3
McIntosh, R. A., Dubcovsky, J., Rogers, W. J., Morris, C., and Xia, X. C. (2017). Catalogue of Gene Symbols for Wheat: 2017 Supplement. Available online at: http://www.shigen.nig.ac.jp/wheat/komugi/genes/macgene/supplement~2017.pdf (accessed March 03, 2022).
Melichar, J. P. E., Berry, S., Newell, C., MacCormack, R., and Boyd, L. A. (2008). QTL identification and microphenotype characterisation of the developmentally regulated yellow rust resistance in the UK wheat cultivar Guardian. Theor. Appl. Genet. 117, 391–399. doi: 10.1007/s00122-008-0783-6
Meng, L., Li, H., Zhang, L., and Wang, J. (2015). QTL IciMapping: integrated software for genetic linkage map construction and quantitative trait locus mapping in biparental populations. Crop J. 3, 269–283. doi: CNKI:SUN:CROP.0.2015-03-011
Mu, J. M., Liu, L., Liu, Y., Wang, M. N., See, D. R., Han, D. J., et al. (2020). Genome-wide association study and gene specific markers identified 51 genes or QTL for resistance to stripe rust in US winter wheat cultivars and breeding lines. Front. Plant Sci. 11, 998. doi: 10.3389/fpls.2020.00998
Naruoka, Y., Garland-Campbell, K. A., and Carter, A. H. (2015). Genome-wide association mapping for stripe rust (Puccinia striif ormis f. sp tritici) in US Pacific Northwest winter wheat (Triticum aestivum L.). Theor. Appl. Genet. 128, 1083–1101. doi: 10.1007/s00122-015-2492-2
Ramburan, V. P., Pretorius, Z. A., Louw, J. H., Boyd, L. A., Smith, P. H., Boshoff, W. H. P., et al. (2004). A genetic analysis of adult plant resistance to stripe rust in the wheat cultivar Kariega. Theor. Appl. Genet. 108, 1426–1433. doi: 10.1007/s00122-003-1567-7
Ren, Y., He, Z. H., Li, J., Lillemo, M., Wu, L., Bai, B., et al. (2012). QTL mapping of adult-plant resistance to stripe rust in a population derived from common wheat cultivars Naxos and Shanghai 3/Catbird. Theor. Appl. Genet. 125, 1211–1221. doi: 10.1007/s00122-012-1907-6
Ren, Y., Liu, L. S., He, Z. H., Wu, L., Bai, B., and Xia, X. C. (2015). QTL mapping of adult-plant resistance to stripe rust in a “Lumai 21xJingshuang 16' wheat population. Plant Breed. 134, 501–507. doi: 10.1111/pbr.12290
Rosewarne, G. M., Singh, R. P., Huerta-Espino, J., Herrera-Foessel, S. A., Forrest, K. L., Hayden, M. J., et al. (2012). Analysis of leaf and stripe rust severities reveals pathotype changes and multiple minor QTLs associated with resistance in an Avocet x Pastor wheat population. Theor. Appl. Genet. 124, 1283–1294. doi: 10.1007/s00122-012-1786-x
Rosewarne, G. M., Singh, R. P., Huerta-Espino, J., and Rebetzke, G. J. (2008). Quantitative trait loci for slow-rusting resistance in wheat to leaf rust and stripe rust identified with multi-environment analysis. Theor. Appl. Genet. 116, 1027–1034. doi: 10.1007/s00122-008-0736-0
Sui, X. X., Wang, M. N., and Chen, X. M. (2009). Molecular mapping of a stripe rust resistance gene in spring wheat cultivar Zak. Phytopathology 99, 1209–1215. doi: 10.1094/PHYTO-99-10-1209
Tian, Y. E., Huang, J., Li, Q., Hou, L., and Wang, B. T. (2011). Inheritance and SSR mapping of a stripe-rust resistance gene YrH122 derived from Psathyrostachys huashanica Keng. Acta Phytopathol. Sin. 41, 64–71. doi: 10.13926/j.cnki.apps.2011.01.007
Vazquez, M. D., Peterson, C. J., Riera-Lizarazu, O., Chen, X. M., Heesacker, A., Ammar, K., et al. (2012). Genetic analysis of adult plant, quantitative resistance to stripe rust in wheat cultivar 'Stephens' in multi-environment trials. Theor. Appl. Genet. 124, 1–11. doi: 10.1007/s00122-011-1681-x
Vazquez, M. D., Zemetra, R., Peterson, C. J., Chen, X. M. M., Heesacker, A., and Mundt, C. C. (2015). Multi-location wheat stripe rust QTL analysis: genetic background and epistatic interactions. Theor. Appl. Genet. 128, 1307–1318. doi: 10.1007/s00122-015-2507-z
Wan, A. M., Chen, X. M., and He, Z. H. (2007). Wheat stripe rust in China. Aust. J. Agric. Res. 58, 605–619. doi: 10.1071/AR06142
Wan, A. M., Zhao, Z. H., Chen, X. M., He, Z. H., Jin, S. L., Jia, Q. Z., et al. (2004). Wheat stripe rust epidemic and virulence of Puccinia striiformis f. sp tritici in China in 2002. Plant Dis. 88, 896–904. doi: 10.1094/PDIS.2004.88.8.896
Wang, J.K. (2009). Inclusive composite interval mapping of quantitative trait genes. Acta Phytopathol Sin. 35, 239–245. doi: 10.3724/SP.J.1006.2009.00239
Wang, Y. B., Xu, S. C., Xu, Z., Liu, T. G., and Lin, R. M. (2006). A microsatellite marker linked to the stripe rust resistance gene YrV23 in the wheat variety Vilmorin23. Hereditas 28, 306–310. doi: 10.16288/j.yczz.2006.03.011
Wellings, C. R. (2011). Global status of stripe rust: a review of historical and current threats. Euphytica 179, 129–141. doi: 10.1007/s10681-011-0360-y
William, H. M., Singh, R. P., Huerta-Espino, J., Palacios, G., and Suenaga, K. (2006). Characterization of genetic loci conferring adult plant resistance to leaf rust and stripe rust in spring wheat. Genome 49, 977–990. doi: 10.1139/g06-052
Xu, L. S., Wang, M. N., Cheng, P., Kang, Z. S., Hulbert, S. H., and Chen, X. M. (2013). Molecular mapping of Yr53, a new gene for stripe rust resistance in durum wheat accession PI 480148 and its transfer to common wheat. Theor. Appl. Genet. 126, 523–533. doi: 10.1007/s00122-012-1998-0
Yao, F. J., Zhang, X. M., Ye, X. L., Li, J., Long, L., Yu, C., et al. (2019). Characterization of molecular diversity and genome-wide association study of stripe rust resistance at the adult plant stage in Northern Chinese wheat landraces. BMC Genet. 20, 1–16. doi: 10.1186/s12863-019-0736-x
Yue, Y. L., Yao, Z. J., Ren, X. X., and Wang, L. (2010). Molecular mapping of a gene for resistance to stripe rust in wheat variety PIW138. Agric. Sci. China 9, 1285–1291. doi: 10.1016/S1671-2927(09)60218-2
Zahravi, M., Bariana, H. S., Shariflou, M. R., Balakrishma, P. V., and Ghannadha, M. R. (2003). “Bulk segregant analysis of stripe rust resistance in wheat (Triticum aestivum) using microsatellite markers,” in Proceedings 10th International Wheat Genetics Symposium, Instituto Sperimentale per Cerealcoltura, Rome, eds Pogna, N. E., Romano, M., Pogna, E. A., and Galterio, 861–863.
Zhao, J., Wang, M. N., Chen, X. M., and Kang, Z. S. (2016). Role of alternate hosts in epidemiology and pathogen variation of cereal rusts. In: Leach, J.E., Lindow, S. (eds). Annu. Rev. Phytopathol. 54, 207–228. doi: 10.1146/annurev-phyto-080615-095851
Zhou, X. L., Han, D. J., Chen, X. M., Gou, H. L., Guo, S. J., Rong, L., et al. (2014). Characterization and molecular mapping of stripe rust resistance gene Yr61 in winter wheat cultivar Pindong 34. Theor. Appl. Genet. 127, 2349–2358. doi: 10.1007/s00122-014-2381-0
Keywords: wheat, stripe rust, QTL mapping, resistance gene, 90K wheat SNP array
Citation: Zhou X, Li X, Han D, Yang S, Kang Z and Ren R (2022) Genome-Wide QTL Mapping for Stripe Rust Resistance in Winter Wheat Pindong 34 Using a 90K SNP Array. Front. Plant Sci. 13:932762. doi: 10.3389/fpls.2022.932762
Received: 30 April 2022; Accepted: 08 June 2022;
Published: 06 July 2022.
Edited by:
Zhiyong Liu, Institute of Genetics and Developmental Biology (CAS), ChinaReviewed by:
Jing Feng, Institute of Plant Protection (CAAS), ChinaCopyright © 2022 Zhou, Li, Han, Yang, Kang and Ren. This is an open-access article distributed under the terms of the Creative Commons Attribution License (CC BY). The use, distribution or reproduction in other forums is permitted, provided the original author(s) and the copyright owner(s) are credited and that the original publication in this journal is cited, in accordance with accepted academic practice. No use, distribution or reproduction is permitted which does not comply with these terms.
*Correspondence: Zhensheng Kang, a2FuZ3pzQG53c3VhZi5lZHUuY24=; Runsheng Ren, cnVuc2hlbmdyZW5AMTYzLmNvbQ==
†These authors have contributed equally to this work and share first authorship
Disclaimer: All claims expressed in this article are solely those of the authors and do not necessarily represent those of their affiliated organizations, or those of the publisher, the editors and the reviewers. Any product that may be evaluated in this article or claim that may be made by its manufacturer is not guaranteed or endorsed by the publisher.
Research integrity at Frontiers
Learn more about the work of our research integrity team to safeguard the quality of each article we publish.