- 1Department of Biological Sciences, International Islamic University, Islamabad, Pakistan
- 2Department of Biology, College of Sciences, United Arab Emirates University, Al Ain, United Arab Emirates
- 3Department of Agriculture, University of Swabi, Swabi, Pakistan
- 4Department of Agricultural Engineering, Khwaja Fareed University of Engineering and Information Technology, Rahim Yar Khan, Pakistan
- 5Department of Biological Sciences, Faculty of Science, King Abdulaziz University, Jeddah, Saudi Arabia
- 6Department of Biology, College of Science, Imam Abdulrahman Bin Faisal University, Dammam, Saudi Arabia
- 7Biology Department, Faculty of Science, University of Tabuk, Tabuk, Saudi Arabia
- 8College of Life Science, Linyi University, Linyi, China
- 9Department of Agricultural Extension Education and Communication, The University of Agriculture, Peshawar, Pakistan
- 10Hainan Key Laboratory for Sustainable Utilization of Tropical Bioresource, College of Tropical Crops, Hainan University, Haikou, China
- 11Department of Agronomy, The University of Haripur, Haripur, Pakistan
In context of the climate change, major abiotic stresses faced by plants include salt stress and drought stress. Though, plants have similar physiological mechanisms to cope with these salt and drought stresses. The physiological and biochemical response of native plants to the combined application of salinity and drought stresses are still not well-understood. Thus, to investigate the combined effect of salinity and drought stresses, an experiment was conducted on Salsola imbricata with four levels of salinity and four drought intensities under the arid climatic conditions. The experiment was conducted in a randomized complete block design with a split-plot arrangement replicated three times. S. imbricata had been found resistant to different levels of individual and combined salt and drought stresses. S. imbricata survived till the end of the experiment. Salt and water stress did not show any significant effects on shoot weight, shoot length, and root length. The drought stress affected the photosynthetic rate, ion uptake and leaf water potential. However, salt stress helped to counter this effect of drought stress. Thus, drought stress did not affect plant growth, photosynthesis rate, and ion uptake when combined with salt stress. Increased Na+ and Cl− uptake under the salt stress helped in osmotic adjustment. Therefore, the leaf water potential (LWP) decreased with increasing the salt stress from 5 dSm−1 until 15 dSm−1 and increased again at 20 dSm−1. At lower salt stress, ABA and proline content declined with increasing the drought stress. However, at higher salt stress, ABA content increased with increasing the drought stress. In conclusion, the salt stress had been found to have a protective role to drought stress for S. imbricata. S. imbricata utilized inorganic ion for osmotic adjustment at lower salinity stress but also accumulate the organic solutes to balance the osmotic pressure of the ions in the vacuole under combined stress conditions. Due to the physical lush green appearance and less maintenance requirements, S. imbricata can be recommended as a native substitute in landscaping under the salt and drought stresses conditions.
Introduction
Agriculture is facing serious threats from abiotic factors such as salt stress and drought stress (Wang et al., 2003). Environmental fluctuations are rapidly increasing including salt and drought stresses, limiting plant productivity by 10% of arable land and more than 50% of major crops (Bartels and Sunkar, 2005). Globally, salt stress is affecting more specifically the irrigated agricultural land, while drought is severely affecting the agricultural crops (Zhu, 2002; Zamin et al., 2019a). Therefore, studies on the plants' response to salt and drought stresses are of primary importance. Halophytes have the ability to withstand and even benefit from salt and drought stresses conditions, which are lethal to the cultivated crops (Zamin and Khattak, 2018). Studying halophytes can lead to produce salt-tolerant crops through genetic modification and effective breeding (Ben Amor et al., 2005). These studies may also help to develop sustainable arid landscapes with native plants, which can conserve drought resources used for landscape irrigations (Zamin et al., 2018).
The effect of abiotic stresses including salt and water stresses are often indistinguishable and interconnected. For example, salt and drought stresses disrupt homeostasis and ion distribution resulting from osmotic stress in the cell (Wang et al., 2003). The plants physiological mechanisms to cope with salt and drought stresses are similar up to some extent. The water potential under salinity and drought decreases significantly in the similar pattern because under salt stress the plants' available water is also decreasing (Hasegawa et al., 2000). The simultaneous incidence of different stresses has positive or negative impacts on plant performance, depending on the nature and duration of the stresses (Niinemets, 2010). With the help of cross-tolerance, the plants have developed some special mechanisms to adapt to one stress and become resistant to some other stresses. This phenomenon is still an important challenge for the researchers and the exact mechanism of cross-tolerance is still not well-understood. Scientists are still focusing to get stable multiple stress tolerant traits in agronomical crops to improve yield, particularly in xeric conditions (Bahmani and Maali-Amiri, 2017).
The photosynthesis is a primary process that is influenced by the salt and drought stresses because of stomatal closure and decreasing the net CO2 diffusion to the chloroplast (Gibberd et al., 2002; Tezara et al., 2002; Anjum et al., 2011). However, the salt or drought stress tolerance is mainly associated with the maintenance of the net photosynthetic rate (Kumar et al., 2000; Anjum et al., 2011). The leaf Na+ and Cl− concentrations increase significantly with increasing electrical conductivity (EC) of the irrigation water (Niu et al., 2012). The production of reactive oxygen species (ROS) is significantly increased under the salt and drought stresses (Miller et al., 2010). The ROS are generated during the stress metabolism as a toxic by-product and play an important role in signal transduction molecules during the plant responses to abiotic stresses (Miller et al., 2008). The overproduction of these ROS can cause oxidative damage to plants (Smirnoff, 1998). Plants have developed antioxidant defense mechanism, which can detoxify the adverse effect of ROS (Caverzan et al., 2012) and protect plant cells from oxidative damage by scavenging of ROS (Gill and Tuteja, 2010). Mostly, the researchers have investigated the responses of cultivated crops to salt and drought stresses on molecular levels (Umezawa et al., 2004). The ROS scavenging capacity of cultivated plants has been widely investigated by applying different stresses separately (Sekmen et al., 2014).
Native plants have the potential not only resist to the aforementioned stresses but also to provide many ecological benefits (Alam et al., 2017; Zamin et al., 2019b). Compared to cultivated relatives, native species have more ability to grow under the salt and drought stresses conditions (Morales et al., 2001; Fiedler, 2006; Stephens et al., 2006; Ochoa et al., 2009; Zamin and Khattak, 2017). According to Garci et al. (2004), many taxa are categorized as drought-resistant often based on the anecdotal observations. The physiological and molecular response of native plants to the combined application of salinity and drought stress is still not well-understood (Harb et al., 2010).
Salsola imbricata (Forssk.) (Arabic name: غضرب) belonging to the family Amaranthaceae is a perennial halophytic shrub that grows in deserts and arid regions of the Arabian Peninsula, southwestern Asia and North Africa. S. imbricata can also be used as a model plant to study the cross-tolerance for salt and drought stress and improve the stress resistance in many other plant species. Moreover, studying desert plants like S. imbricata for their field performance under the xeric conditions will provide guidelines for their proper maintenance in landscapes. We evaluated the field performance of S. imbricata under combined salt and drought stresses condition and study underlying stress resistance mechanism to overcome these stresses. Therefore, the study aimed to explore the suitability of S. imbricata for urban landscaping and to bring sustainability in landscaping.
Materials and Methods
Research Site
The field experiment to study the eco-physiological response of S. imbricata to different salt and drought stresses was carried out at the AL-Foa Research Farm, United Arab Emirates University, Al Ain, Abu Dhabi, UAE (24°12' N and 55°44' E) during 2015–2016. The experimental site was situated in the arid region, having a long hot summer season of 4 months, i.e., from May to September with a maximum temperature above 45°C. The winter prevails from mid-November to the end of February followed by a short spring season from March to April. The mean annual temperature varies between 12 and 45°C during winter and summer seasons, respectively (Statistics Center Abu Dhabi, 2015). The soil used in potting mix was sandy in nature, which was comprised of 87.5% sand, 5% silt, and 7.5% clay. More detailed soil properties are presented in Table 1 (Abdelfattah et al., 2009).
Experimental Design
Salsola imbricata seeds were sown in germinating trays with growing media of potting soil and sweet sand 1:1 by volume. The soil used in potting mix was sandy in nature having 24.53% carbonate content with pH 7.58 and EC 9.49. The Ca+ content of the soil sample was 25 mg/kg whereas Mg was 34.2 mg/kg and low K+ content, i.e., 7.53 mg/kg (Table 1). After 3 weeks of germination, seedlings were transplanted to pots with 20 cm diameter and 15 cm height filled with sweet desert sand which has lower EC values and is considered good for agriculture purposes. Seedlings were thinned to one seedling per pot. After 1 month of transplantation of seedlings from germination trays to the pots, four saltwater treatments were prepared by dissolving NaCl in freshwater supplied by Al- Ain in municipality, i.e., 5 dS m−1 (Control; S1), 10 dS m−1 (low salinity level; S2), 15 dS m−1 (moderate salinity level; S3), and 20 dS m−1 (high salinity level; S4; Al-Dakheel et al., 2015; Zamin et al., 2019a). Salinity treatments were prepared in four different water tanks. These water tanks were connected to the drip irrigation line to supply water to each pot individually with four irrigation intensities. To estimate the Field capacity, the fully water-saturated soil was weighed and then dried to constant weight at 105°C. The weight difference between water-saturated and oven-dried soil was taken as the weight of water needed to bring soil to field capacity and lower FC was calculated accordingly. Four irrigation intensities were: 100% field capacity (Control; C), 80% field capacity (low stress), 60% field capacity (moderate stress), and 40% field capacity (severe stress) (Álvarez et al., 2009). Plants were irrigated 2–3 times per week, depending upon evaporative demand using the drip irrigation system with one emitter per plant each delivering 2 Lh−1. The amount of water applied to the control varied between 788 and 1,182ml per pot per week. The average of water was 985 ml/week for the WL1 (control) and 787, 590, and 392ml/week for WL2, WL3, and WL4, respectively. The NPK @ 5–7 g/plant was applied to each plant before the start of experiment. Agronomic practices, e.g., weeding and crop maintenance, etc., were equally applied to all treatments during the entire growing period of plants. Experiment was conducted in open field and plants were grown under natural environmental conditions. The mean monthly temperature ranged between 32.9 and 33.4°C and humidity from 21 to 29% while 0mm rainfall was forecasted at the beginning and end of the experiment. The experiment was conducted in a randomized complete block design replicated three times. The salinity levels were allotted to the main plot while the irrigation intensities were allotted to the sub-plots.
Percent Survival, Harvesting, and Sampling
Plants that survived under each stress treatment were counted and the survival percentage was calculated. Three plants from each treatment were harvested after 6 months of treatment application to record morphological parameters. Plant samples from each treatment were collected and instantly ground in liquid nitrogen and stored at −80°C for the quantitative chemical analysis.
Morphological Traits
After harvest, the plant samples were carefully cleaned from sand, washed with distilled water, and dried with the help of tissue paper. After harvesting, each plant was divided into shoots and roots and root and shoot length were measured. Shoot length was measured from the base of stem till the apex end while root length was measured from the root base up to the end of primary root. Samples were oven-dried (60°C) for 24 h and weighted (±0.0001 g). For morphological traits, all the samples were put in Ziploc bags, placed in an ice bag at 4°C, and transferred to the laboratory.
Physiological Traits
The photosynthetic rate of upper, lower, and basal leaves was measured weekly using a Plant Photosynthesis Meter (EARS, Netherlands; Samarah, 2005). Replicated leaf water potential (MPa) was recorded during midday using a WP4C Dewpoint psychrometer (Decagon Devices, Inc., USA; Xiong et al., 2014). Leaf water potential was recorded after 1 and 5 months of treatment application. Phosphorus concentrations were estimated in plant leaves at the end of the experiment by Olsen (1954) methodology. Na+ content (μ mole g−1) of plant extracts was determined by the Flame Emission Spectroscopy at the end of the experiment. For Cl− content, 50mg of leaf and root samples were ground and heated in distilled water for 3 h (80°C). The Cl− content (μmole g−1) of the extract was then determined with the chloride analyzer at the end of the experiment.
Biochemical Traits
The ABA and proline extraction was performed on 10mg of freeze-dried leaf tissue as described by Forcat et al. (2008). The samples were analyzed for ABA and proline using LCMS/MS, and were filtered through a 0.45μm cellulose acetate syringe. The phytohormones separation was done using a C18 column (ZORBAX Eclipse Plus). An injection of 2 μl was loaded onto the C18 column (1.8μm particle size, 2.1mm inner diameter, and 50mm long) at a flow rate of 0.2 ml/min and the column temperature was kept at 35°C. The liquid chromatography was connected to an Agilent Technologies Mass Spectrometry (6420 Triple Quad detector). For elution, solvent A consists of formic acid (0.1%) with distilled water and solvent B consists of an LCMS grade acetonitrile were used. The analytical procedure was as follows.
Solvent A was used (5min), then the gradient from 0 to 100% solvent B was used (5–20min), after the solvent B was kept constant (5min) and at 25.1min solvent A was 100% was used for 30min. During the analysis with LC–MSMS only negative polarity mode was used for ABA and Proline analysis. For fragmentation, nitrogen gas was used. The capillary voltage was 4,000V, the gas flow was 8 L/min, the gas temperature was 300°C and the nebulizer pressure was 45 psi.
Statistical Analysis
Two way analysis of variance (ANOVA) was used to check the effect of salinity, drought, and their interaction on morphological, biochemical, and physiological traits. While the normality was checked with Shapiro–Wilk test. The post-hoc Tukey HSD was used to check the comparison between treatments. All the analysis was performed using the SPSS software at 5% probability level.
Results
Percent Survival, Root and Shoot Length and Weight
Results concerning the morphological response of S. imbricata to varying salt and drought stresses are given in Table 2. The ANOVA revealed that all the morphological parameters (percent survival, root and shoot length and weight) did not show any significant (p > 0.05) effect by the drought stress. A similar response was found in the case of salinity stress except root weight (g) which was significantly affected by increasing salinity. The percent survival of S. imbricata did not affect significantly (p > 0.05) by different levels of salt and drought stresses. The interaction between salt and drought stresses was also not significant and S. imbricata survived on all salt and drought levels with percent survival >90% (Table 2). However, the root weight was significantly affected by the salt stress while the drought stress had no significant effect. The root weight was maximum under the lower salinity and decreased with increasing drought stress. The root weight (10.22 g) observed at 5 dS m−1 was statistically at par to the root weight (7.50 g) at 10 dS m−1. Furthermore, the root weight (4.87 and 9.69 g) recorded at 15 and 20 dS m−1 was statistically similar with root weight at 10 dS m−1.
Photosynthetic Rate, Leaf Water Potential, Na+ Uptake, and Cl– Uptake
Physiological response of S. imbricata to different drought and salinity stresses is shown in Table 3. According to the ANOVA, varying responses were recorded for different physiological traits. The drought stress did not show any significant (p > 0.05) effect on all the parameters except leaf water potential (MPa) which was significantly affected. In contrast to drought stress, salinity stress had a significant (p < 0.05) effect on all the physiological parameters of S. imbricata except photosynthetic rate. However, the interaction between salt and drought stresses was found significant.
The interactive effect of salinity and drought stress was found significant for photosynthetic rate (Figure 1). Generally, under the drought stress, the photosynthetic rate decreases with increasing salinity levels up to 15 dSm−1 while at 20 dS m−1, increasing tendency was found. The maximum photosynthetic rate was observed at 100% field capacity under 5 dS m−1 which was statistically similar to S1WL2 and S3WL1. It was evident that S. imbricata can maintain the photosynthesis under combine drought and salinity stresses to cope with the harsh and adverse climatic conditions.
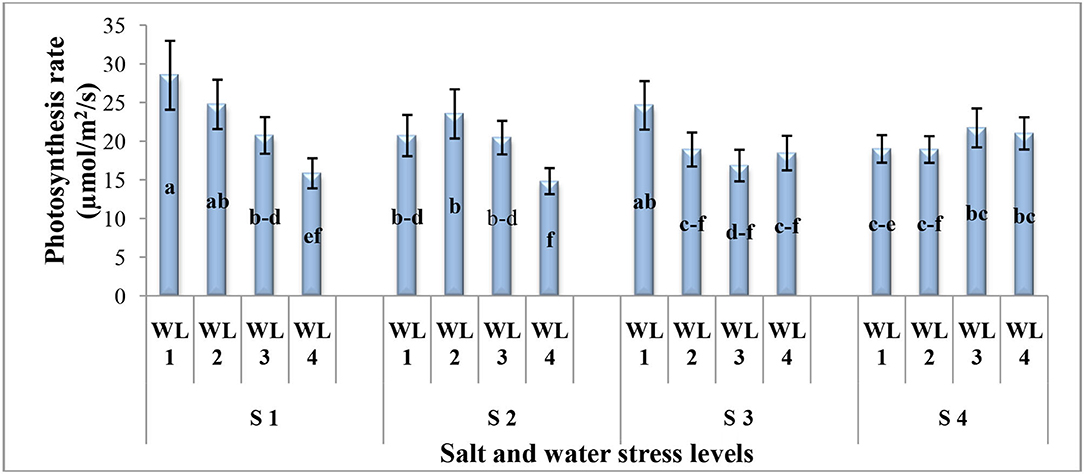
Figure 1. Interactive effect of salinity and drought stress on the photosynthesis rate (μmol m−2 S−1) of S. imbricata. Value bars with different letters are significantly different from each other at α = 0.05, while error bar represents the standard error of mean (n = 3). WL stands for water level (WL1 = 100% field capacity, WL2 = 80% field capacity, WL3 = 60% field capacity, and WL4=40% field capacity) while S represents salinity (S1 = 5 dS m−1, S2 = 10 dS m−1, S3 = 15 dS m−1, and S4 = 20 dS m−1).
Water potential was also significantly affected in response to the interaction of salinity and drought stress as indicated in Figure 2. Leaf water potential decreased with increasing salt stress from 5 dS m−1 until 15 dS m−1. However, at salinity stress of 20 dS m−1 leaf water potential was not much affected like 15 dSm−1. Increasing water stress also decreases the leaf water potential under all salt stress (Figure 2).
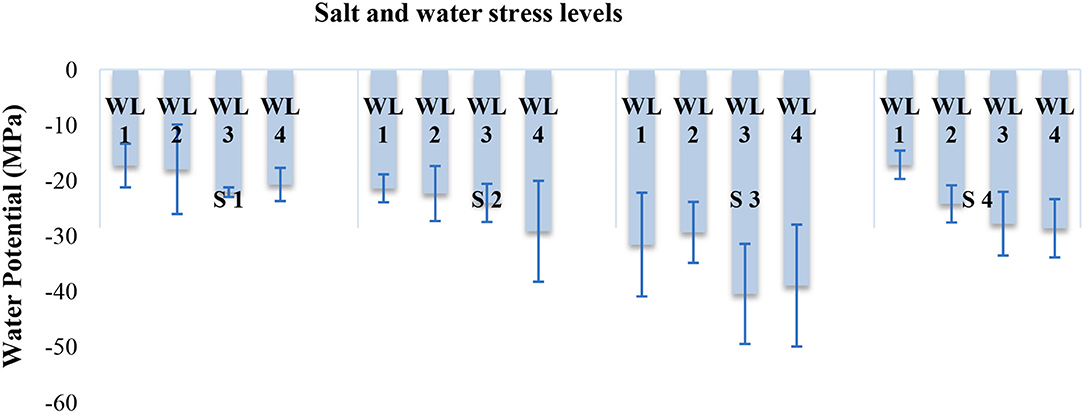
Figure 2. Interactive effect of salinity and drought stress on the water potential (MPa) of S. imbricata. Error bar represents the standard error of mean (n = 3). WL stands for water level (WL1 = 100% field capacity, WL2 = 80% field capacity, WL3 = 60% field capacity, and WL4 = 40% field capacity), while S represents salinity (S1 = 5 dS m−1, S2 = 10 dS m−1, S3 = 15 dS m−1, and S4 = 20 dS m−1).
As far as the interactive effect of salinity and drought stress is concerned, the ion uptake (Na+ and Cl− contents) was significantly affected by the salinity and drought stresses (Figures 3, 4). Na+ concentration had an interactive effect (p ≤ 0.05) on salt and water stress. The Na+ content increased with increasing the salt and water stress. Even at the low salt stress level, when external Na+ was low, Na+ concentration increased under the water stress in shoots (Figure 3). Generally, Cl− content increases with increasing salinity. The Cl− content increased with increasing the drought stress at lower salinity, while at higher salinity level, Cl− content decreased after the drought stress reached to a certain level (Figure 4). This shows that S. imbricata has the ability to enhance the ion uptake under the severe drought and salinity conditions.
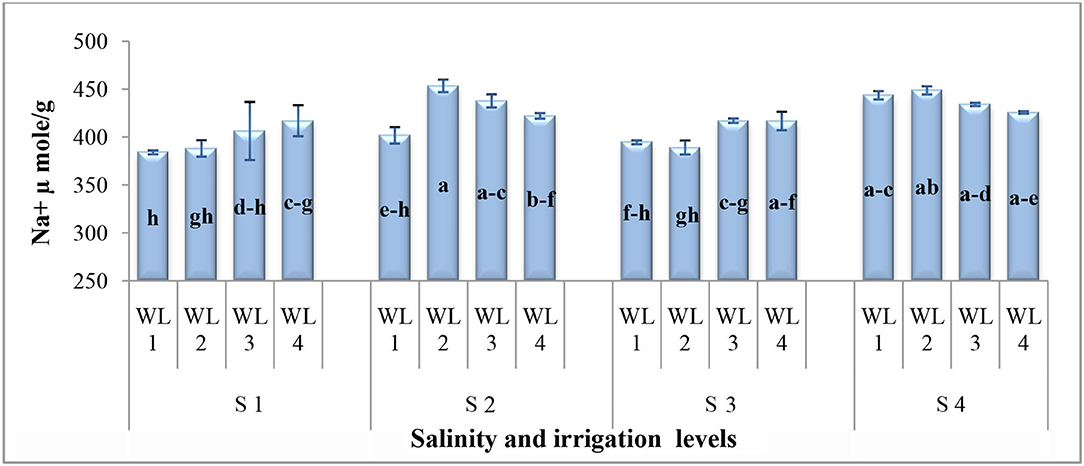
Figure 3. Interactive effect of salinity and drought stress on Na+ uptake (μmol g−1) of S. imbricata. Value bars with different letters are significantly different from each other at α = 0.05, while error bar represents the standard error of mean (n = 3). WL stands for water level (WL1 = 100% field capacity, WL2 = 80% field capacity, WL3 = 60% field capacity, and WL4 = 40% field capacity), while S represents salinity (S1 = 5 dS m−1, S2 = 10 dS m−1, S3 = 15 dS m−1, and S4 = 20 dS m−1).
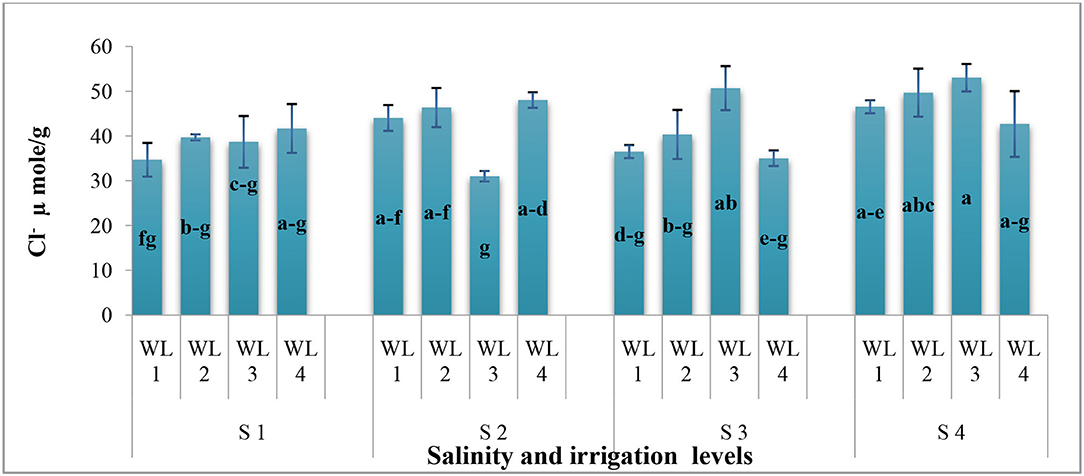
Figure 4. Interactive effect of salinity and drought stress on the Chloride uptake (μmol g−1) of S. imbricata. Value bars with different letters are significantly different from each other at α = 0.05, while error bar represents the standard error of mean (n = 3). WL stands for water level (WL1 = 100% field capacity, WL2 = 80% field capacity, WL3 = 60% field capacity, and WL4 = 40% field capacity), while S represents salinity (S1 = 5 dS m−1, S2 = 10 dS m−1, S3 = 15 dS m−1, and S4 = 20 dS m−1).
ABA (μg g–1 FW) and Proline (μg g–1 FW) Contents
Results concerning biochemical response of S. imbricata to varying drought and salinity stresses are presented in Table 4. The ANOVA revealed that ABA and proline content were significantly salt and drought stress interaction.
The interactive effect of salinity and drought stress was found significant for the ABA content (Figure 5). Generally, under combine salt and drought stress, the ABA content decreases with increasing drought stress for salinity levels up to 15 dS m−1 while at 20 dS m−1, the trend was the opposite. Under the salt stress of 20 dS m−1, maximum ABA production was recorded at 60% field capacity which is statistically at par with 40% field capacity. However, the ABA response to 60 and 40% field capacity was similar at all salinity levels. The maximum ABA content was observed at 60% field capacity under severe salinity (20 dS m−1) which was statistically similar to 100 and 80% field capacity at 10 and 15 dS m−1 and 100% field capacity at 5 dS m−1. It was evident that S. imbricata increased the ABA production under severe drought and salinity stress to combat their growth- and performance-related adversities.
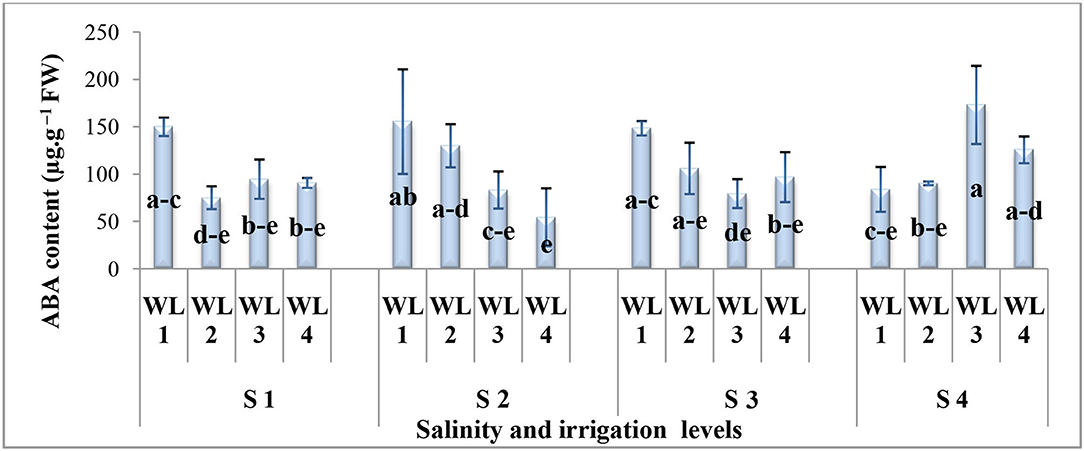
Figure 5. Interactive effect of salinity and drought stress on the ABA content (μg g−1 FW) of S. imbricata. Value bars with different letters are significantly different from each other at α = 0.05, while error bar represents the standard error of mean (n = 3). WL stands for water level (WL1 = 100% field capacity, WL2 = 80% field capacity, WL3 = 60% field capacity, and WL4 = 40% field capacity) while S represents the salinity (S1 = 5 dS m−1, S2 = 10 dS m−1, S3 = 15 dS m−1, and S4 = 20 dS m−1).
The proline content significantly varied in response to the interaction of salinity and drought stress as shown in Figure 6. The proline content decreases with increasing drought stress and salt stress together except S4. For salt stress of 20 dS m−1, proline content increased up to 60% field capacity and then declined. Maximum proline production was recorded 60% field capacity at 20 dS m−1 indicating that S. imbricata enhances the proline production under severe salinity and drought stress to cope with such stresses.
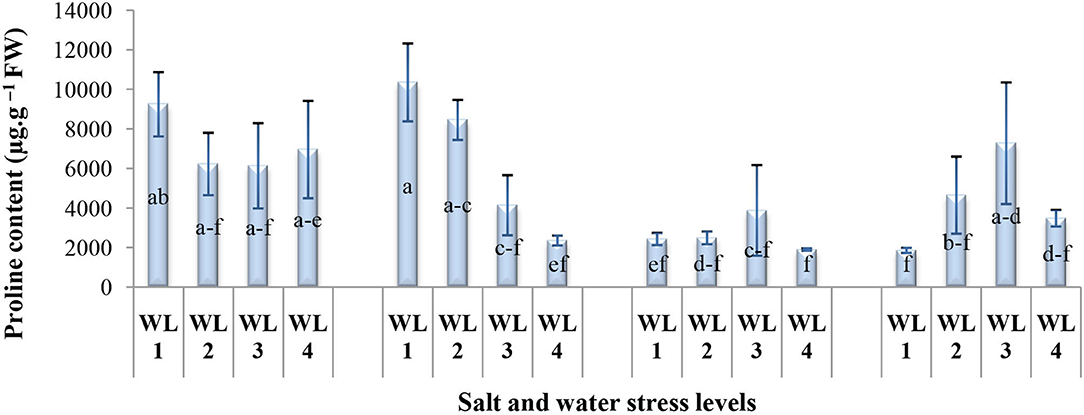
Figure 6. Interactive effect of salinity and drought stress on the proline content (μg g−1 FW) of S. imbricata. Value bars with different letters are significantly different from each other at α = 0.05, while error bar represents the standard error of mean (n = 3). WL stands for water level (WL1 = 100% field capacity, WL2 = 80% field capacity, WL3 = 60% field capacity, and WL4 = 40% field capacity), while S represents salinity (S1 = 5 dS m−1, S2 = 10 dS m−1, S3 = 15 dS m−1, and S4 = 20 dS m−1).
Discussion
In the present experiment, S. imbricata survived for 6 months with no significant effect of salt and drought stress on the growth parameters. It is evident that S. imbricata is resistant to salt and drought stress.
Salt and drought stress are considered as separate and additive factors contributing to growth reduction (Munns, 2002; Chaves et al., 2009). However, in the current experiment, salt and drought stress did not show any significant effect on shoot weight, shoot length, and root length of S. imbricata. Higher salinity helped to reduce the negative effects of drought stress. Only root weight decreased with increasing salt stress. Moderate salinity (50–250mM NaCl) can stimulate the growth of many halophytes (Flowers et al., 1986; Khan et al., 2000). NaCl may have positive effects under the drought stress, i.e., salt stress alleviated the negative effects of drought stress. These positive effects of salinity were reported for Atriplex nummularia (Hassine et al., 2008), Sesuvium portulacastrum (Slama et al., 2007a), A. canescens (Glenn and Brown, 1998), A. lentiformis (Meinzer and Zhu, 1999), Suaeda fruticosa (L.) Forssk (Khan et al., 2000), and A. halimus (Alla et al., 2012). This improved plant performance under combined salt and drought stress may be due to their effect on osmotic adjustment through higher Na+ and proline accumulation and decrease of K+ accumulation (Wu et al., 2015).
Salt and drought stresses showed a significant interactive effect on the photosynthetic rate of S. imbricata. At lower salt stress, the photosynthetic rate decreased with increasing drought stress. On the other hand, at severe salt stress (20 dS m−1), the drought stress had no significant effect on the photosynthetic rate. The salt stress had a protective effect on the photosynthetic rate (Figure 1). The current results are in line with Wang et al. (2011) for Tamarix chinensis Lour and with Miranda-Apodaca et al. (2018) for quinoa. Under salt or drought stress, leaf water potential and thus photosynthetic activity is decreased (Razzaghi et al., 2011). This reduction in photosynthesis can be caused by a stomatal limitation with stomatal closure (Nicolas et al., 1993; De Pascale and Barbieri, 1995; Goldstein et al., 1996) non-stomatal limitation (disturbance of photosynthetic activity; Downton, 1977; Drew et al., 1990) or both limitations at low and high salt concentration (Downton et al., 1990; Yeo et al., 1991). The drought stress can inhibit the activity of photosystem II and the rate of CO2 assimilation (Bloch et al., 2006; Monti et al., 2006) which in turn could decrease the photosynthesis (Wu et al., 2016).
The Na+ and Cl− uptake had significant results for the salt and drought stress interaction. Na+ and Cl− uptake was significantly increased with increasing the salt stress. Drought stress was also found to increase Na+ and Cl− uptake (Figure 3). However, S. imbricata decreased the Na+ uptake with increasing the drought stress at the highest salinity level (20 dS m−1). Studies carried out to evaluate the combined effects of salt and drought stress are in line with our findings (Martínez et al., 2005; Slama et al., 2008; Khalid and Cai, 2011; Khan et al., 2017a,b). Under saline conditions, Na+ in the growth medium might compete with K+ in the low absorption by the roots (Blumwald, 2000).
In response to low water potential under the salt and drought stress conditions additional solutes are accumulated which is referred as osmotic adjustment (OA; Zhang et al., 1999; Verslues et al., 2006). In halophyte species, Na+ presents in the vacuoles is involved in osmotic adjustment (Martínez et al., 2005; Slama et al., 2007b). Salt stress results in Na+ and Cl− accumulation in shoots which are more effective for osmatic adjustment than the production of organic solutes under drought stress (Liu et al., 2008; Slama et al., 2008; Sucre and Suarez, 2011; Álvarez et al., 2012). Hassine et al. (2008) reported that during the stress period, shoot water potential remained lower in Atriplex halimus plants exposed to PEG than in those exposed to the highest dose of NaCl. Miranda-Apodaca et al. (2018) stated that plants under salt stress exhibit a greater capacity for osmotic adjustment while plants subjected to drought stress treatment showed more dehydration. Thus, the NaCl addition mitigated the deleterious impact of osmotic stress on growth ( Martínez et al., 2005 :Wu et al., 2016). Increasing salinity beyond toxic levels can be managed by halophytes using the strategy of exclusion of salts via salts glands present on their lower surface of leaves (Zamin et al., 2019a).
Abscisic acid accumulates and involves in all the aspects of the low water potential response. ABA-derived root growth and stomatal conductance are important in the avoidance of lower growth (Schroeder et al., 2001; Sharp and LeNoble, 2002; Verslues et al., 2006). As a dehydration avoidance response, ABA induces the accumulation of compatible solutes (Ober and Sharp, 1994). ABA production is a signal for the stomatal closure and reduction of stomatal density to decrease water loss by transpiration (Razzaghi et al., 2011; Adolf et al., 2013). ABA induced the stomatal closure by a reduction in the turgor pressure of guard cells (Schroeder et al., 2001; Bartels and Sunkar, 2005). These responses improve the water-use efficiency of the plant for the short term (Waseem et al., 2011; Oliveira et al., 2013). The NaCl stress did not affect transpiration or ABA levels of Atriplex spongiosa up to 75mol m−3 but transpiration fell and ABA levels rose when the NaCl was increased upto 150mol m−3. The drought stress resulted an increase in the leaf ABA content while salt stress had no effect (Achuo et al., 2006). A report by Li et al. (2011) stated that Cotinus coggygria var. cinerea significantly reduced the relative growth rate, but increased the endogenous ABA under drought.
Proline accumulation relates more to the osmotic stress than any specific salt effect (Munns, 2002). Martínez et al. (2005) reported that 0 or 15% PEG had no impact on the proline concentration at low NaCl (50mM) concentration (Martínez et al., 2005). Atriplex spongiosa and Suaeda monoica recorded low proline contents at 300 and 500mol m−3 NaCl or below, respectively. However, a significant increase was detected at high salinities (Storey and Jones, 1979). Atriplex halimus showed similar responses after treating seedlings with either 50, 300, and 550mM NaCl or drought (control and withholding water). Proline was significantly increased only by the high salt stress and drought stress, nonetheless, combined treatments led to decrease if any (Alla et al., 2012). This significant increase was still in low concentration which was supposed to function as osmoprotectant.
Conclusion
Both salt and drought stress had no significant effect on the survival percentage and growth performance of S. imbricata. However, severe salt stress induced a decrease in root weight. Salt stress help to alleviate negative effects of drought stress through accumulation of Na+ and Cl− ions and organic solutes at higher salinity. In conclusion, S. imbricata can be classified as the salt includer halophyte. This species achieved osmoregulation by adopting intracellular compartmentalization of ions and avoid high concentration of these ions in cytoplasm. It can be concluded that S. imbricata can survive under drought and saline conditions up to 20 dS m−1 without affecting growth and morphology. Therefore, it can be recommended as substitute in landscaping under extreme drought and saline conditions. Further studies can be carried out to study the economical uses of S. imbricata and determine the optimal salinity and irrigation requirements of S. imbricata. Furthermore, studies can be carried out to find out the molecular mechanisms that make S. imbricata resistance to salt and drought stress.
Data Availability Statement
The raw data supporting the conclusions of this article will be made available by the authors, without undue reservation.
Author Contributions
HAla and MZ: conceptualization. MA, AS, SS, SH, HAlh, AB, and NA: methodology and formal analysis. SA and MZ: writing—original draft preparation. BA and SF: writing—review and editing. MZ: supervision. HAlh, AB, NA, SA, and BA: funding acquisition. All authors contributed to the article and approved the submitted version.
Funding
This project was funded by the Deputyship for Research and Innovation, Ministry of Education in Saudi Arabia under project number (IFPIP: 1132-130-1442).
Conflict of Interest
The authors declare that the research was conducted in the absence of any commercial or financial relationships that could be construed as a potential conflict of interest.
Publisher's Note
All claims expressed in this article are solely those of the authors and do not necessarily represent those of their affiliated organizations, or those of the publisher, the editors and the reviewers. Any product that may be evaluated in this article, or claim that may be made by its manufacturer, is not guaranteed or endorsed by the publisher.
Acknowledgments
The authors extend their appreciation to the Deputyship for Research and Innovation, Ministry of Education in Saudi Arabia for funding this research work through the project number (IFPIP: 1132-130-1442) and King Abdulaziz University, DSR, Jeddah, Saudi Arabia.
References
Abdelfattah, M. A., Dawoud, M. A. H., and Shahid, S. A. (2009). “Soil and water management for combating desertification–towards implementation of the United Nations convention to combat desertification from the UAE perspectives,” in International Conference Soil Degradation, Riga.
Achuo, E. A., Prinsen, E., and Höfte, M. (2006). Influence of drought, salt stress and abscisic acid on the resistance of tomato to Botrytis cinerea and Oidium neolycopersici. Plant Pathol. 55, 178–186. doi: 10.1111/j.1365-3059.2006.01340.x
Adolf, V. I., Jacobsen, S.-., E., and Shabala, S. (2013). Salt tolerance mechanisms in quinoa (Chenopodium quinoa Willd.). Environ. Exp. Bot. 92, 43–54. doi: 10.1016/j.envexpbot.2012.07.004
Alam, H., Khattak, J. Z. K., Ppoyil, S. B. T., Kurup, S. S., and Ksiksi, T. S. (2017). Landscaping with native plants in the UAE: A review. Emir. J. Food Agric. 729–741.
Al-Dakheel, A. J., Hussain, M. I., and Rahman, A. Q. M. A. (2015). Impact of irrigation water salinity on agronomical and quality attributes of Cenchrus ciliaris L. accessions. Agric. Water Manag. 159, 148–154. doi: 10.1016/j.agwat.2015.06.014
Alla, M. M. N., Khedr, A. H. A., Serag, M. M., Abu-Alnaga, A. Z., and Nada, R. M. (2012). Regulation of metabolomics in Atriplex halimus growth under salt and drought stress. Plant Growth Regul. 67, 281–304. doi: 10.1007/s10725-012-9687-1
Álvarez, S., Gómez-Bellot, M. J., Castillo, M., Bañón, S., and Sánchez-Blanco, M. J. (2012). Osmotic and saline effect on growth, water relations, and ion uptake and translocation in Phlomis purpurea plants. Environ. Exp. Bot. 78, 138–145. doi: 10.1016/j.envexpbot.2011.12.035
Álvarez, S., Navarro, A., Bañón, S., and Sánchez-Blanco, M. J. (2009). Regulated deficit irrigation in potted Dianthus plants: effects of severe and moderate water stress on growth and physiological responses. Sci. Horti. 122, 579–585. doi: 10.1016/j.scienta.2009.06.030
Anjum, S. A., Xie, X., Wang, L., Saleem, M. F., Man, C., and Lei, W. (2011). Morphological, physiological and biochemical responses of plants to drought stress. Afr. J. Agric. Res. 6, 2026–2032. doi: 10.5897/AJAR10.027
Bahmani, M., and Maali-Amiri, R. M. (2017). Genetic regulation of cross tolerance in plants under biotic and abiotic stresses. Res. Gate 13, 4076–4083.
Bartels, D., and Sunkar, R. (2005). Drought and salt tolerance in plants. Crit. Rev. Plant Sci. 24, 23–58. doi: 10.1080/07352680590910410
Ben Amor, N., Ben Hamed, K., Debez, A., Grignon, C., and Abdelly, C. (2005). Physiological and antioxidant responses of the perennial halophyte Crithmum maritimum to salinity. Plant Sci. 168, 889–899. doi: 10.1016/j.plantsci.2004.11.002
Bloch, D., Hoffmann, C. M., and Märländer, B. (2006). Impact of water supply on photosynthesis, water use and carbon isotope discrimination of sugar beet genotypes. Eur. J. Agron. 24, 218–225. doi: 10.1016/j.eja.2005.08.004
Blumwald, E. (2000). Sodium transport and salt tolerance in plants. Curr. Opin. Cell Biol. 12, 431–434. doi: 10.1016/S0955-0674(00)00112-5
Caverzan, A., Passaia, G., Rosa, S. B., Ribeiro, C. W., Lazzarotto, F., and Margis-Pinheiro, M. (2012). Plant responses to stresses: role of ascorbate peroxidase in the antioxidant protection. Gent. Mol. Biol. 35, 1011–1019. doi: 10.1590/S1415-47572012000600016
Chaves, M. M., Flexas, J., and Pinheiro, C. (2009). Photosynthesis under drought and salt stress: regulation mechanisms from whole plant to cell. Ann. Bot. 103, 551–560. doi: 10.1093/aob/mcn125
De Pascale, S., and Barbieri, G. (1995). Effects of soil salinity from long-term irrigation with saline-sodic water on yield and quality of winter vegetable crops. Sci. Hortic. 64, 145–157. doi: 10.1016/0304-4238(95)00823-3
Downton, W. J. S. (1977). Photosynthesis in salt-stressed grapevines. Funct. Plant Biol. 4, 183–192. doi: 10.1071/PP9770183
Downton, W. J. S., Loveys, B. R., and Grant, W. J. R. (1990). Salinity effects on the stomatal behaviour of grapevine. New Phytol. 116, 499–503. doi: 10.1111/j.1469-8137.1990.tb00535.x
Drew, M. C., Hold, P. S., and Picchioni, G. A. (1990). Inhibition by NaCl of net CO2 fixation and yield of cucumber. J. Am. Soc. Hortic. Sci. 115, 472–477. doi: 10.21273/JASHS.115.3.472
Fiedler, A. K. (2006). Evaluation of Michigan Native Plants to Provide Resources for Natural Enemy Arthropods. East Lansing, MI: Michigan State University.
Flowers, T. J., Hajibagheri, M. A., and Clipson, N. J. W. (1986). Halophytes. Q. Rev. Biol. 61, 313–337. doi: 10.1086/415032
Forcat, S., Bennett, M. H., Mansfield, J. W., and Grant, M. R. (2008). A rapid and robust method for simultaneously measuring changes in the phytohormones ABA, JA and SA in plants following biotic and abiotic stress. Plant Methods 4:16. doi: 10.1186/1746-4811-4-16
Garci, M. C., Evans, R. Y., and Montserrat, R. S. (2004). Estimation of relative water use among ornamental landscape species. Sci. Hortic. 99, 163–174. doi: 10.1016/S0304-4238(03)00092-X
Gibberd, M. R., Turner, N. C., and Storey, R. (2002). Influence of saline irrigation on growth, ion accumulation and partitioning, and leaf gas exchange of carrot (Daucus carota L.). Ann. Bot. 90, 715–724. doi: 10.1093/aob/mcf253
Gill, S. S., and Tuteja, N. (2010). Reactive oxygen species and antioxidant machinery in abiotic stress tolerance in crop plants. Plant Physiol. Biochem. 48, 909–930. doi: 10.1016/j.plaphy.2010.08.016
Glenn, E. P., and Brown, J. J. (1998). Effects of soil salt levels on the growth and water use efficiency of Atriplex canescens (Chenopodiaceae) varieties in drying soil. Am. J. Bot. 85, 10–16. doi: 10.2307/2446548
Goldstein, G., Drake, D. R., Alpha, C., Melcher, P., Heraux, J., and Azocar, A. (1996). Growth and photosynthetic responses of Scaevola sericea, a Hawaiian coastal shrub, to substrate salinity and salt spray. Int. J. Plant Sci. 157, 171–179. doi: 10.1086/297336
Harb, A., Krishnan, A., Ambavaram, M. M., and Pereira, A. (2010). Molecular and physiological analysis of drought stress in Arabidopsis reveals early responses leading to acclimation in plant growth. Plant Physiol. 154, 1254–1271. doi: 10.1104/pp.110.161752
Hasegawa, P. M., Bressan, R. A., Zhu, J. K., and Bohnert, H. J. (2000). Plant cellular and molecular responses to high salinity. Annu. Rev. Plant Biol. 51, 463–499.
Hassine, A. B., Ghanem, M. E., Bouzid, S., and Lutts, S. (2008). An inland and a coastal population of the Mediterranean xero-halophyte species Atriplex halimus L. differ in their ability to accumulate proline and glycinebetaine in response to salinity and water stress. J. Exp. Bot. 59, 1315–1326. doi: 10.1093/jxb/ern040
Khalid, K. A., and Cai, W. (2011). The effects of mannitol and salinity stresses on growth and biochemical accumulations in lemon balm. Acta Ecol. Sin. 31, 112–120. doi: 10.1016/j.chnaes.2011.01.001
Khan, A., Anwar, Y., Hasan, M., Iqbal, A., Ali, M., Alharby, H., et al. (2017a). Attenuation of drought stress in Brassica seedlings with exogenous application of Ca2+ and H2O2. Plants 6:20. doi: 10.3390/plants6020020
Khan, M. A., Ungar, I. A., and Showalter, A. M. (2000). Effects of salinity on growth, water relations and ion accumulation of the subtropical perennial halophyte, Atriplex griffithii var. Stocksii. Ann. Bot. 85, 225–232. doi: 10.1006/anbo.1999.1022
Khan, M. S. A., Chowdhury, J. A., Razzaque, M. A., Ali, M. Z., Paul, S. K., and Aziz, M. A. (2017b). Dry matter production and seed yield of soybean as affected by post-flowering salinity and water stress. Bangladesh Agron. J. 19, 21–27. doi: 10.3329/baj.v19i2.31849
Kumar, S. G., Lakshmi, A., Madhusudhan, K. V., Ramanjulu, S., and Sudhakar, C. (2000). Photosynthesis parameters in two cultivars of mulberry differing in salt tolerance. Photosynthetica 36, 611–616. doi: 10.1023/A:1007008608217
Li, Y., Zhao, H., Duan, B., Korpelainen, H., and Li, C. (2011). Effect of drought and ABA on growth, photosynthesis and antioxidant system of Cotinus coggygria seedlings under two different light conditions. Environ. Exp. Bot. 71, 107–113. doi: 10.1016/j.envexpbot.2010.11.005
Liu, X., Huang, W., Niu, Z., Mori, S., and Tadano, T. (2008). Interactive effect of moisture levels and salinity levels of soil on the growth and ion relations of halophyte. Commun. Soil Sci. Plant Anal. 39, 741–752. doi: 10.1080/00103620701879448
Martínez, J. P., Kinet, J. M., Bajji, M., and Lutts, S. (2005). NaCl alleviates polyethylene glycol-induced water stress in the halophyte species Atriplex halimus L. J. Exp. Bot. 56, 2421–2431. doi: 10.1093/jxb/eri235
Meinzer, F. C., and Zhu, J. (1999). Efficiency of C4 photosynthesis in Atriplex lentiformis under salinity stress. Funct. Plant Biol. 26, 79–86. doi: 10.1071/PP98143
Miller, G., Shulaev, V., and Mittler, R. (2008). Reactive oxygen signaling and abiotic stress. Physiol. Plant 133, 481–489. doi: 10.1111/j.1399-3054.2008.01090.x
Miller, G. A. D., Suzuki, N., Ciftci-Yilmaz, S., and Mittler, R. O. N. (2010). Reactive oxygen species homeostasis and signalling during drought and salinity stresses. Plant Cell Environ. 33, 453–467. doi: 10.1111/j.1365-3040.2009.02041.x
Miranda-Apodaca, J., Yoldi-Achalandabaso, A., Aguirresarobe, A., del Canto, A., and Pérez-López, U. (2018). Similarities and differences between the responses to osmotic and ionic stress in quinoa from a water use perspective. Agric. Water Manag. 203, 344–352. doi: 10.1016/j.agwat.2018.03.026
Monti, A., Brugnoli, E., Scartazza, A., and Amaducci, M. T. (2006). The effect of transient and continuous drought on yield, photosynthesis and carbon isotope discrimination in sugar beet (Beta vulgaris L.). J. Exp. Bot. 57, 1253–1262. doi: 10.1093/jxb/erj091
Morales, M. A., Olmos, E., Torrecillas, A., Sánchez-Blanco, M. J., and Alarcon, J. J. (2001). Differences in water relations, leaf ion accumulation and excretion rates between cultivated and wild species of Limonium sp. grown in conditions of saline stress. Flora 196, 345–352. doi: 10.1016/S0367-2530(17)30070-1
Munns, R. (2002). Comparative physiology of salt and water stress. Plant Cell Environ. 25, 239–250. doi: 10.1046/j.0016-8025.2001.00808.x
Nicolas, M. E., Munns, R., Samarakoon, A. B., and Gifford, R. M. (1993). Elevated CO2 improves the growth of wheat under salinity. Funct. Plant Biol. 20, 349–360. doi: 10.1071/PP9930349
Niinemets, Ü. (2010). Responses of forest trees to single and multiple environmental stresses from seedlings to mature plants: past stress history, stress interactions, tolerance and acclimation. For. Ecol. Manag. 260, 1623–1639. doi: 10.1016/j.foreco.2010.07.054
Niu, G., Osuna, P., Sun, Y., and Rodriguez, D. S. (2012). Seedling emergence, growth and mineral nutrition of ornamental chili peppers irrigated with saline water. Hort Sci. 47, 1653–1657. doi: 10.21273/HORTSCI.47.11.1653
Ober, E. S., and Sharp, R. E. (1994). Proline accumulation in maize (Zea mays L.) primary roots at low water potentials (I. Requirement for increased levels of abscisic acid). Plant Physiol. 105, 981–987. doi: 10.1104/pp.105.3.981
Ochoa, J., Muñoz, M., Vicente, M. J., Martínez-Sánchez, J. J., and Franco, J. A. (2009). Native ornamental species for urban landscaping and xero-gardening in semi-arid environments. II Int. Conf. Landsc. Urban Horticult. 881, 425–428. doi: 10.17660/ActaHortic.2010.881.68
Oliveira, D. A. B., Alencar, N. L. M., and Gomes-Filho, E. (2013). “Comparison between the water and salt stress effects on plant growth and development,” in Responses of Organisms to Water Stress. Rijeka: IntechOpen. doi: 10.5772/54223
Olsen, S. R. (1954). Estimation of Available Phosphorus in Soils by Extraction With Sodium Bicarbonate. East Lansing, MI: US Department of Agriculture.
Razzaghi, F., Ahmadi, S. H., Adolf, V. I., Jensen, C. R., Jacobsen, S. E., and Andersen, M. N. (2011). Water relations and transpiration of quinoa (Chenopodium quinoa Willd.) under salinity and soil drying. J. Agron. Crop. Sci. 197, 348–360. doi: 10.1111/j.1439-037X.2011.00473.x
Samarah, N. H. (2005). Effects of drought stress on growth and yield of barley. Agron. Sustain. Dev. 25, 145–149. doi: 10.1051/agro:2004064
Schroeder, J. I., Kwak, J. M., and Allen, G. J. (2001). Guard cell abscisic acid signalling and engineering drought hardiness in plants. Nature 410:327. doi: 10.1038/35066500
Sekmen, A. H., Ozgur, R., Uzilday, B., and Turkan, I. (2014). Reactive oxygen species scavenging capacities of cotton (Gossypium hirsutum) cultivars under combined drought and heat induced oxidative stress. Environ. Exp. Bot. 99, 141–149. doi: 10.1016/j.envexpbot.2013.11.010
Sharp, R. E., and LeNoble, M. E. (2002). ABA, ethylene and the control of shoot and root growth under water stress. J. Exp. Bot. 53, 33–37. doi: 10.1093/jexbot/53.366.33
Slama, I., Ghnaya, T., Hessini, K., Messedi, D., Savouré, A., and Abdelly, C. (2007b). Comparative study of the effects of mannitol and PEG osmotic stress on growth and solute accumulation in Sesuvium portulacastrum. Environ. Exp. Bot. 61, 10–17. doi: 10.1016/j.envexpbot.2007.02.004
Slama, I., Ghnaya, T., Messedi, D., Hessini, K., Labidi, N., Savoure, A., et al. (2007a). Effect of sodium chloride on the response of the halophyte species Sesuvium portulacastrum grown in mannitol-induced water stress. J. Plant Res. 120, 291–299. doi: 10.1007/s10265-006-0056-x
Slama, I., Ghnaya, T., Savour,é, A., and Abdelly, C. (2008). Combined effects of long-term salinity and soil drying on growth, water relations, nutrient status and proline accumulation of Sesuvium portulacastrum. C. R. Biol. 331, 442–451. doi: 10.1016/j.crvi.2008.03.006
Smirnoff, N. (1998). Plant resistance to environmental stress. Curr. Opin. Biotechnol. 9, 214–219. doi: 10.1016/S0958-1669(98)80118-3
Stephens, C. J., Schellhorn, N. A., Wood, G. M., and Austin, A. D. (2006). Parasitic wasp assemblages associated with native and weedy plant species in an agricultural landscape. Austral. Entomol. 45, 176–184. doi: 10.1111/j.1440-6055.2006.00519.x
Storey, R., and Jones, R. G. W. (1979). Responses of Atriplex spongiosa and Suaeda monoica to salinity. Plant Physiol. 63, 156–162. doi: 10.1104/pp.63.1.156
Sucre, B., and Suarez, N. (2011). Effect of salinity and PEG-induced water stress on water status, gas exchange, solute accumulation, and leaf growth in Ipomoea pes-caprae. Environ. Exp. Bot. 70, 192–203. doi: 10.1016/j.envexpbot.2010.09.004
Tezara, W., Mitchell, V., Driscoll, S. P., and Lawlor, D. W. (2002). Effects of water deficit and its interaction with CO2 supply on the biochemistry and physiology of photosynthesis in sunflower. J. Exp. Bot. 53, 1781–1781. doi: 10.1093/jxb/erf021
Umezawa, T., Yoshida, R., Maruyama, K., Yamaguchi-Shinozaki, K., and Shinozaki, K. (2004). SRK2C, a SNF1-related protein kinase 2, improves drought tolerance by controlling stress-responsive gene expression in Arabidopsis thaliana. Proc. Natl. Acad. Sci. U. S. A. 101, 17306–17311. doi: 10.1073/pnas.0407758101
Verslues, P. E., Agarwal, M., Katiyar-Agarwal, S., Zhu, J., and Zhu, J. K. (2006). Methods and concepts in quantifying resistance to drought, salt and freezing, abiotic stresses that affect plant water status. Plant J. 45, 523–539. doi: 10.1111/j.1365-313X.2005.02593.x
Wang, W., Vinocur, B., and Altman, A. (2003). Plant responses to drought, salinity and extreme temperatures: towards genetic engineering for stress tolerance. Planta 218, 1–14. doi: 10.1007/s00425-003-1105-5
Wang, W., Wang, R., Yuan, Y., Du, N., and Guo, W. (2011). Effects of salt and water stress on plant biomass and photosynthetic characteristics of Tamarisk (Tamarix chinensis Lour.) seedlings. African J. Biotech. 10, 17981–17989. doi: 10.5897/AJB11.1864
Waseem, M., Ali, A., Tahir, M., Nadeem, M. A., Ayub, M., Tanveer, A., et al. (2011). Mechanism of drought tolerance in plant and its management through different methods. C. J. Agric. Sci. 5, 10–25.
Wu, G. Q., Feng, R. J., Liang, N., Yuan, H. J., and Sun, W. B. (2015). Sodium chloride stimulates growth and alleviates sorbitol-induced osmotic stress in sugar beet seedlings. Plant Growth Regul. 75, 307–316. doi: 10.1007/s10725-014-9954-4
Wu, G. Q., Feng, R. J., and Shui, Q. Z. (2016). Effect of osmotic stress on growth and osmolytes accumulation in sugar beet (Beta vulgaris L.) plants. Plant Soil Environ. 62, 189–194. doi: 10.17221/101/2016-PSE
Xiong, D., Yu, T., Zhang, T., Li, Y., Peng, S., and Huang, J. (2014). Leaf hydraulic conductance is coordinated with leaf morpho-anatomical traits and nitrogen status in the genus Oryza. J. Exp. Bot. 66, 741–748. doi: 10.1093/jxb/eru434
Yeo, A. R., Lee, S., Izard, P., Boursier, P. J., and Flowers, T. J. (1991). Short-and long-term effects of salinity on leaf growth in rice (Oryza sativa L.). J. Exp. Bot. 42, 881–889. doi: 10.1093/jxb/42.7.881
Zamin, M., Fahad, S., Khattak, A. M., Adnan, M., Wahid, F., Raza, A., et al. (2019b). Developing the first halophytic turfgrasses for the urban landscape from native Arabian desert grass. Environ. Sci. Pollut. Res. 27, 39702–39716. doi: 10.1007/s11356-019-06218-3
Zamin, M., and Khattak, A. M. (2017). Performance of Sporobolus spicatus ecotypes, UAE native grass, under various salinity levels. Pure Appl. Biol. 6, 595–604. doi: 10.19045/bspab.2017.60061
Zamin, M., and Khattak, A. M. (2018). Evaluating Sporobolus spicatus ecotypes under different mowing heights for turf use. Sarhad. J. Agric. 34, 114–122. doi: 10.17582/journal.sja/2018/34.1.114.122
Zamin, M., Khattak, A. M., Alyafei, M. A., Sajid, M., Shakur, M., Shah, S., et al. (2018). Sporobolus spicatus, a potential turf grass under the climatic conditions of United Arab Emirates. J. Sci. Agric. 2, 1–8. doi: 10.25081/jsa.2018.v2.868
Zamin, M., Khattak, A. M., Salim, A. M., Marcum, K. B., Shakur, M., Shah, S., et al. (2019a). Performance of Aeluropus lagopoide s (mangrove grass) ecotypes, a potential turfgrass, under high saline conditions. Environ. Sci. Pollut. Res. 26, 13410–13421. doi: 10.1007/s11356-019-04838-3
Zhang, J., Nguyen, H. T., and Blum, A. (1999). Genetic analysis of osmotic adjustment in crop plants. J. Exp. Biol. 50, 291–302. doi: 10.1093/jxb/50.332.291
Keywords: biochemical, drought, landscaping, native plants, salinity, physiological
Citation: Alam H, Zamin M, Adnan M, Shah AN, Alharby HF, Bamagoos AA, Alabdallah NM, Alzahrani SS, Alharbi BM, Saud S, Hassan S and Fahad S (2022) Exploring Suitability of Salsola imbricata (Fetid Saltwort) for Salinity and Drought Conditions: A Step Toward Sustainable Landscaping Under Changing Climate. Front. Plant Sci. 13:900210. doi: 10.3389/fpls.2022.900210
Received: 20 March 2022; Accepted: 04 May 2022;
Published: 08 June 2022.
Edited by:
Mukhtar Ahmed, Pir Mehr Ali Shah Arid Agriculture University, PakistanReviewed by:
Riyazali Zafarali Sayyed, P.S.G.V.P.M's Arts, Science and Commerce College, IndiaZhang Zuo Lin, Hubei Academy of Agricultural Sciences, China
Neeshu Joshi, Agriculture University, Jodhpur, India
Copyright © 2022 Alam, Zamin, Adnan, Shah, Alharby, Bamagoos, Alabdallah, Alzahrani, Alharbi, Saud, Hassan and Fahad. This is an open-access article distributed under the terms of the Creative Commons Attribution License (CC BY). The use, distribution or reproduction in other forums is permitted, provided the original author(s) and the copyright owner(s) are credited and that the original publication in this journal is cited, in accordance with accepted academic practice. No use, distribution or reproduction is permitted which does not comply with these terms.
*Correspondence: Shah Fahad, c2hhaF9mYWhhZDgwJiN4MDAwNDA7eWFob28uY29t; Muhammad Adnan, bWFkbmFuc2VzJiN4MDAwNDA7Z21haWwuY29t; Shah Saud, c2F1ZGhvcnQmI3gwMDA0MDtnbWFpbC5jb20=
†These authors have contributed equally to this work and share first authorship