- Laboratory of Plant Molecular Physiology, Plant Physiology Sector, Department of Biology, Federal University of Lavras, Lavras, Brazil
Coffee (Coffea arabica L.) presents an asynchronous flowering regulated by an endogenous and environmental stimulus, and anthesis occurs once plants are rehydrated after a period of water deficit. We evaluated the evolution of Abscisic Acid (ABA), ethylene, 1-aminocyclopropane-1-carboxylate (ACC) content, ACC oxidase (ACO) activity, and expression analysis of the Lysine Histidine Transporter 1 (LHT1) transporter, in the roots, leaves, and flower buds from three coffee genotypes (C. arabica L. cv Oeiras, Acauã, and Semperflorens) cultivated under field conditions with two experiments. In a third field experiment, the effect of the exogenous supply of ACC in coffee anthesis was evaluated. We found an increased ACC level, low ACO activity, decreased level of ethylene, and a decreased level of ABA in all tissues from the three coffee genotypes in the re-watering period just before anthesis, and a high expression of the LHT1 in flower buds and leaves. The ethylene content and ACO activity decreased from rainy to dry period whereas the ABA content increased. A higher number of opened and G6 stage flower buds were observed in the treatment with exogenous ACC. The results showed that the interaction of ABA-ACO-ethylene and intercellular ACC transport among the leaves, buds, and roots in coffee favors an increased level of ACC that is most likely, involved as a modulator in coffee anthesis. This study provides evidence that ACC can play an important role independently of ethylene in the anthesis process in a perennial crop.
Introduction
Globally, the production of most crops depends on flowering, and particularly, in some species, such as coffee (C. arabica L.), this process can directly influence the quality of the final product (cup quality). Coffee asynchronous flower bud development leads to uneven flowering (de Oliveira et al., 2014), and depending on the geographical location of the plantation, it can result in various flowering events. Flowering is an important step of the coffee reproductive phase, being influenced by different endogenous and environmental signals. In this process, one of the most important elements is water as a signaling transporter, as it has been shown that coffee anthesis occurs after rain events preceded by a period of moderate water stress (Drinnan and Menzel, 1994). Other factors, such as photoperiod, temperature, shade conditions, plant nutritional status, and phytohormones can also affect floral transition and plant development (Camargo, 1985; Ramírez et al., 2010).
Information on the involvement of plant hormones in coffee flowering regulation is scarce. Previous studies have shown that Abscisic Acid (ABA) increases during the dry period (Silva et al., 2018) and is associated with coffee flower bud dormant or “latent state” (Matsumoto and Lopez, 2014). After water withdrawing stress, dormant and non-dormant G4 flower buds (ranging from 3.2 to 6 mm in length) (Morais et al., 2008) could be distinguished based on their ethylene evolution (Schuch et al., 1992). In other species, changes in ethylene levels can either delay or promote flowering, as observed in Rice (Oryza sativa L.) (Wang Q. et al., 2013), Arabidopsis (Arabidopsis thaliana) (Achard et al., 2007), Pineapples (Ananas comosus L.) (Trusov and Botella, 2006), and Roses (Meng et al., 2014).
The relationship between ABA and ethylene in the regulation of different biological processes is well known. Similar to ethylene, ABA accumulation accelerates the senescence of cut flowers and flowering in potted plants (Liu et al., 2014). Exogenous applications of ABA in Roses (Rosa hybrida L.) increased the ethylene sensitivity as well as the expression of some ethylene receptors (Müller et al., 2000). In hibiscus (Hibiscus rosa-sinensis L.), ABA negatively regulates the expression of the ethylene biosynthesis genes during flower development (Trivellini et al., 2011). On the other hand, the exogenous application of ABA positively regulates ethylene biosynthesis, increasing the ACC content in the abscission zone of the Lupine flower (Lupinus luteus L.) (Wilmowicz et al., 2016).
Recently, it was proposed that ethylene can play an important role in coffee flowering, since rehydrating droughted plants can increase ethylene levels and ethylene sensitivity, regulating coffee anthesis (Lima et al., 2020). Ethylene is a volatile compound that can be easily transported by diffusion throughout cells and intercellular spaces (Alonso and Ecker, 2001). Ethylene is produced from the amino acid methionine, which is first converted into S-adenosylmethionine (S-AdoMet) through the S-AdoMet synthetase enzyme, and subsequently, 1-aminocyclopropane-1-carboxylate (ACC) synthase (ACS) converts S-AdoMet into 1-Aminocyclopropane-1-Carboxylic Acid (ACC), that is considered the limiting step of the ethylene biosynthesis pathway. Finally, ACC oxidase (ACO) generates ethylene by oxidizing ACC (Fa Yang and Hoffman, 1984).
The role of ACC, as a signaling molecule independent of ethylene biosynthesis, has been reviewed, showing that ACC is part of many biological processes in plants such as in cell wall metabolism, vegetative development, stomatal development, and pollen tube attraction (Van de Poel et al., 2014; Vanderstraeten and van Der Straeten, 2017; Polko and Kieber, 2019; Mou et al., 2020). In addition, it was also found that ACC has a distinct function than ethylene in the non-seed plant Marchantia polymorpha L. (An et al., 2010; Li et al., 2020; Katayose et al., 2021), and in the induction of sexual reproduction of the Marine red alga (Pyropia yezoensis) (Rhodophyta) (Uji et al., 2020).
These different biological functions attributed to ACC seem to have originated an evolutionarily conserved signal that predates its efficient conversion to ethylene in higher plants (Li et al., 2020). ACC transport throughout the plant occurs by two amino acid transporters, LHT1 and LHT2 (Shin et al., 2015; Choi et al., 2019), having the LHT1 higher activity described in root tissues and flower development (Mou et al., 2020). In light of this knowledge, research on the role of ACC associated with the development and growth of plants should be expanded, including the reconsideration of physiological processes primarily attributed to ethylene.
The main objective of this research was to evaluate the modulation activity of ACC in the coffee flowering process quantifying the evolution of ABA, ACO, ACC, and ethylene in the most critical development period for coffee flower buds, including flower bud development, bud dormancy, and anthesis; and the LHT1 gene expression evaluation as an ACC intracellular transporter. It is possible that ACC, in addition to being the ethylene precursor, may be involved in the regulation of anthesis and fertilization of the coffee flower. To corroborate this hypothesis, field experiments were carried out complemented with laboratory analysis.
Materials and Methods
To elucidate the modulation of ACC in coffee anthesis, three field experiments were designed. In each experiment, we sought to answer three complementary hypotheses related to hormonal crosstalk, the role of water, and the response of coffee plants in the exogenous application of ACC in the coffee anthesis process (Supplementary Table 1).
Field Experiment I
To evaluate the evolution of phytohormones (ABA, Ethylene), intermediaries in the ethylene biosynthesis pathway (ACC and ACO), and relative expression of LHT1 gene in coffee flowering development, three coffee genotypes (C. arabica L.) were selected: Oeiras, Acauã, and Semperflorens, classified as early, late, and continuous, respectively, regarding their flowering and fruit ripening pattern. The experiment was conducted in a 5-year-old coffee plantation at the Department of Agriculture of The Federal University of Lavras (UFLA) following a randomized block design with three biological repetitions, each one comprising ten plants. The experiment was carried out from May to August 2020, with samplings happening at the end of the rainy period (May 18th, late fall), end of dry period (-August 18th, winter), and re-watering period (August 28th, late winter, 10 days after the first rain event). For each period, the sampling comprised of roots (15 to 25 cm deep into the soil), leaves (young and fully expanded at the third or fourth node from plagiotropic branches), and flower buds (G2 buds with a broad and flat apex, G3 buds up to 3 mm in length, and G4 buds ranging from 3.2 to 6 mm in length) (Morais et al., 2008), taking place from 8:00 to 10:00 am. Samples were immediately frozen in liquid nitrogen and stored at −80°C to be evaluated for ACC and ABA content, ACO activity, and LHT1 expression analysis. For the ethylene analysis, leaves, buds, and root samples were collected in glass tubes. Plant water status was assessed by measuring predawn leaf water potential (from 03:30 till 05:30) using a Scholander-type pressure chamber (Figure 1A). Both quantifications were made according to Lima et al. (2020).
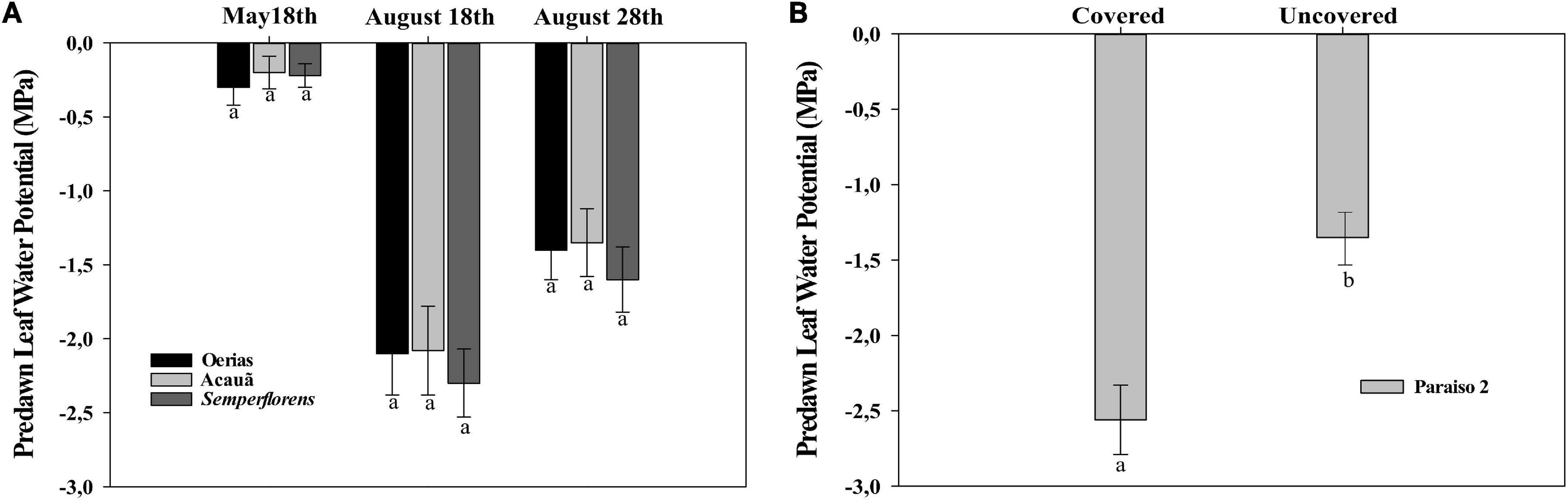
Figure 1. Predawn leaf water potential for coffee genotypes. (A) Coffee plants from field experiment I in the rainy period (May 18th), dry period (August 20th), and re-watering period (August 28th) for Oeiras, Acauã, and Semperflorens genotypes. (B) Coffee plants from field experiment II in the re-watering period (August 28th) for Paraiso 2 coffee cultivar. Data are the mean ± 95% of SD of the mean (n = 8). Different letters within the same period indicate statistical (P < 0.05) differences within the same period, respectively.
Field Experiment II
Aiming to evaluate the effect of rain and hormonal balance in coffee flowering corroborating the results of the field experiment I, a second experiment was conducted in a 3-year-old coffee (C. arabica cv. Paraiso 2) plantation. The experiment consisted of the evaluation of two treatments, plants under normal field conditions (Uncovered) and plants under rainfall exclusion (Covered), with three biological repetitions, each one composed of 10 plants in each treatment. Rainfall exclusion was achieved by the installation of translucent nylon (polypropylene) fixed on twelve 3.2 m high eucalyptus wood logs, deep 0.60 m in the soil with three wood logs 3 m distant from each other. Every eucalyptus wood log was arranged on each posterior side and in the middle of the coffee line. A translucent nylon piece of 0.2 micrometers was fixed on top of wood logs using a wooden piece of 5 × 7 cm with a small hobnail, covering a total of 81 m2 of rain exclusion area (Supplementary Figure 1). Leaf water potential measurement (Figure 1B) and tissue sampling for the evaluation of Ethylene, ACC and ABA content, ACO activity, and LHT1 expression analysis were performed similarly to field experiment I.
Field Experiment III
To better understand the effect of the ethylene pathway modulating coffee anthesis, exogenous treatments of ethylene and ACC were evaluated. Firstly, a test was carried out to determine the ideal concentrations for ethylene application using Ethrel 720 in three concentrations: (i) 1.3 ml, (ii) 0.50 ml, and (iii) 0.25 ml. The application was carried out using a total volume of 3 L in 3 five-year-old coffee plants (C. arabica cv Catuaí 144) wetting well leaves and flower buds. All concentrations accelerated the leaf senescence process (Supplementary Figure 2) and promoted the abortion of flower buds (data not shown). For this reason, the application of ethylene (Ethrel 720) for flowering analysis was excluded. For ACC, the experiment was carried out in the coffee germplasm collection on adult, 4-year-old coffee cultivar (C. arabica cv. Semperflorens) trees. The experiment was conducted in a randomized design with four treatments (One plant per treatment) and ten replicates per treatment (Ten branches per plant) with 5–6 nodes per branch. Four treatments were applied, ACC (0.1 mm), 1-Methylcyclopropene (1-MCP, 50 mg a.i. L–1), ACC + 1-MCP, and a control (water). It was added to all treatments a surfactant (Tween 20®, at 0.08%) to improve the solutions adherence and penetration in the coffee tissues; 200 mL of total solution per treatment was used during applications.
The ACC concentration applied was determined according to the ACC content found in coffee tissues in the re-watering period of field experiment I [Figures 2(1C)]. 1-MCP concentration applied was determined according to Lima et al. (2020). Each treatment was sprayed carefully in each branch, totally wetting the leaves and flower buds in the upper third of the plant. Three days after the application, a rain event of 35 mm occurred, having all treatments influenced by the same quantity of water in the field. Flower bud differentiation was evaluated 15 days after treatment application (previous to anthesis) counting the number of flower buds in G2, G3, G4, and G6 stages (Morais et al., 2008) from all nodes (5–6) in each branch (240–250 flower buds per treatment).
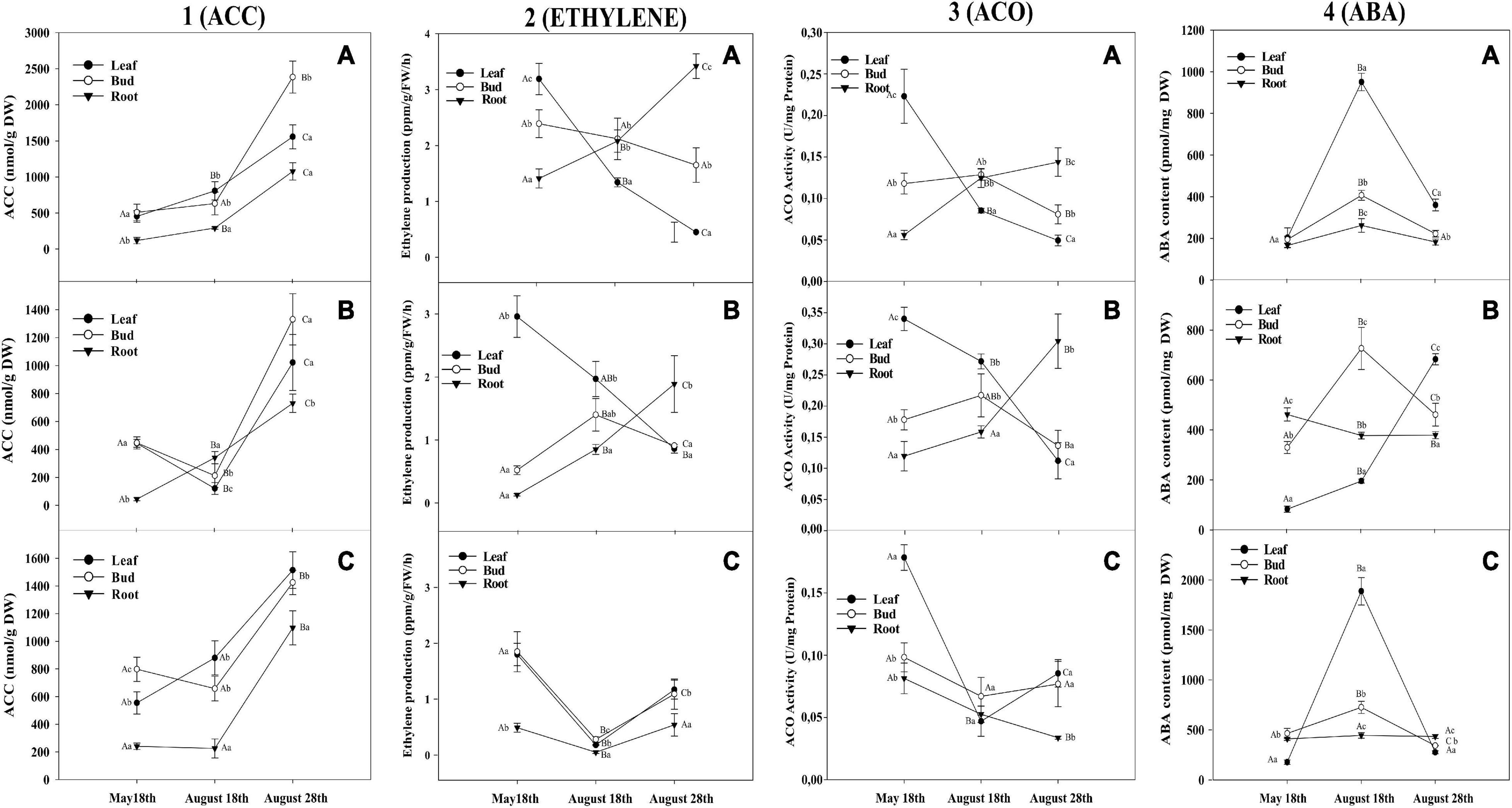
Figure 2. Plant hormones evolution for coffee genotypes. 1-aminocyclopropane-1-carboxylate (ACC) content (1), Ethylene production (2), ACC oxidase (ACO) activity (3), and abscisic acid (ABA) content (4) in leaves, flower buds, and roots from (A) Oeiras, (B) Acauã, and (C) Semperflorens plants at the rainy period (May 18th), at the dry period (August 18th), and after rain in the re-watering period (August 28th). Data are means ± 95% SD of the mean (n = 6). Different upper-case and lower-case letters indicate the statistical difference of each tissue among the different periods and different tissues within the same period, respectively.
Physiological and Biochemical Analysis
1-Aminocyclopropane-1-Carboxylate Quantification
Leaf, flower bud, and root ACC concentrations were determined by the Bulens method (Bulens et al., 2011) with minor modifications. Coffee tissues were ground in liquid nitrogen using a mortar and pestle. A total of 200 mg were transferred to 2 ml tubes for extraction with 1 ml of sulfosalicylic acid 5% (p/v). After homogenization, tubes were maintained at 4°C for 30 min, being gently mixed with 5 min intervals. After centrifugation at 3,090 g and 4°C for 30 min, 300 μl of the supernatant was transferred, in duplicates for each sample, to 10 ml vacutainer glass followed by 100 μl of 10 mm HgCl2. The tubes were subsequently sealed with a serum cap, and 300 μl of cold NaOH-NaOCl solution (NaOH 0.434 M and NaOCl 0.173 M) was added using a syringe. The mixture was then homogenized for 5 s and incubated for 4 min on ice. After mixing the samples for 5 s, 5 ml of the headspace was withdrawn and ethylene levels were measured using the F-900 Portable Ethylene Analyzer (Felix Instruments, United States) operating under the gas chromatography (GC) emulation mode. The ethylene ppm values were transformed to nmol using the gases equation, and the ACC content was expressed as nmol/g DW.
1-Aminocyclopropane-1-Carboxylate Oxidase Enzymatic Activity Quantification
Leaf, flower bud, and root ACO activity were determined by the Bulens method (Bulens et al., 2011), with some modifications. After tissue grinding in liquid nitrogen, 250 mg were transferred to 2 ml tubes with 50 mg of polyvinylpolypyrrolidone. ACO extraction was performed with 1 ml of extraction buffer [4-morpholinepropanesulfonic acid (MOPS) 400 mm pH 7.2; 30 mm of ascorbic acid, and glycerol 10% (v/v)]. Subsequently, samples were homogenized and centrifuged at 22,000 g for 30 min. Then, 800 μl of supernatant was collected and used for the subsequent analysis. A total of 400 μl of the enzymatic extract was added to 3.6 ml of a reaction buffer containing: 2.8 ml of MOPS buffer (MOPS 64.3 mm pH 7.2; glycerol 12.86% (v/v); sodium bicarbonate 25.8 mM; and iron sulfate 26 μM), 0.4 ml of ascorbic acid 45 mm, 0.1 mL of ACC 36 mm, and 0.3 ml of dithiothreitol (DDT) 12 mm. After sample homogenization, the reaction for ethylene release was carried out for 20 min at 30°C. After homogenization by 5 s, 5 ml of the headspace air was withdrawn and used for the ethylene measurements using the F-900 Portable Ethylene Analyzer (Felix Instruments, United States) operating under the GC emulation mode. The ethylene ppm values were transformed to nmol using the gases equation, and the protein content was determined by the Bradford method (Bradford, 1976) in duplicate, using bovine serum albumin (BSA) as standard. One unit of ACO activity was defined as 1 nmol of ACC converted to 1 nmol of ethylene per min at 30°C (Dong et al., 1992).
Ethylene Measurement
For all sampling periods, leaves, flower buds, and root tissues were immediately incubated in 10 ml vacutainer glass tubes, containing a moist tissue placed on the bottom of each vial, sealed with serum caps, and incubated for 24 h. For each biological sample, ethylene was quantified from the headspace gas using the F-900 Portable Ethylene Analyzer (Felix Instruments, United States) operating under the GC emulation mode in triplicate. Plant material was incubated in two separate vials, and the headspace gas was withdrawn from the vials with a 10 ml plastic syringe. Samples, made of 2.5 ml of gas from each vial, were extracted using the same syringe and subsequently injected into the Ethylene Analyzer. After ethylene measurement, the plant material was weighed and the ethylene production rate was expressed as ppm/g FW. h–1 (Fresh weight per hour).
Abscisic Acid Extraction and Concentration Measurement
The ABA content analysis in the leaves, flower buds, and roots was carried out according to Liu et al. (2014). Samples were ground in liquid nitrogen, then 500 μl of an extraction solution (methanol 90% (v/v) and sodium diethyldithiocarbamate trihydrate 200 mg/L) was added to 200 mg of plant material in a siliconized borosilicate tube. Samples were incubated overnight under dark conditions at 4°C and centrifuged at 8,000 g for 10 min at 4°C. The supernatant was transferred to a pre-cold 1.5 ml Eppendorf tube and evaporated in a vacuum centrifuge at room temperature. The residue was dissolved in a methanolic Tris buffer solution containing methanol 10% (v/v), Tris–HCl 50 mM pH 8.0, MgCl2 1 mm, and NaCl 150 mm. The ABA concentration in all tissues was measured with a Phytodetek ABA enzyme immunoassay test kit (Agdia; Elkhart, IN, United States, Catalog number: PDK 09347/0096), in duplicate according to the manufacturer’s instructions.
Molecular Analysis
In silico Analysis
Genes encoding for Lysine Histidine Transporter (LHT1) in A. thaliana were retrieved from The Arabidopsis Information Resource1 database. The protein sequences from these genes were used as input to perform similarity searches against the genomes of plant species from different orders such as Rubiaceae, Solanales, Rosales, Gentianales, Malpighiales, Vitales, Poales, and Amborellales by the Protein Basic Local Alignment Tool (BLASTp), at the National Center for Biotechnology Information (NCBI2) (Thompson et al., 1994). The sequences with significant similarity (e-value < 10-5) were selected and the predicted proteins in which the inputted sequence identity was below 70% were removed. Protein sequences were aligned using the Clustal W program with the standard patterns. The phylogenetic tree was drawn using the MEGA software version 6.03 (Tamura et al., 2013), with a neighbor-joining comparison model (Saitou and Nei, 1987) and bootstrap values from 5,000 replicate to assess the robustness of the tree. The LHT1 primer (Forward 5′TTCGTCGGTTGCTCATCTCA and reverse: 5′TTGCCTTCTTCAGCCGTT) design was performed using the sequence obtained in the in silico analysis and the Primer Express v2.0 program (Applied Biosystems) (Supplementary Table 2).
RNA Extraction, cDNA Synthesis, and RT-qPCR Assay
The total RNA from the leaves, flower buds, and roots was extracted according to Oliveira et al. (2015), with minor modifications. RNA samples (7.5 μg) were treated with DNase I using the Turbo DNA-free Kit (Ambion)4 to eliminate DNA contamination. The RNA integrity was analyzed in 1% agarose gel, and the RNA content, as well as quality, were accessed by spectroscopy (OD260/280 and OD260/230 > 1.8) (NanoVue GE Healthcare, Munich, Germany). One μg of the total RNA was reverse transcribed into cDNA using the High-Capacity cDNA Reverse Transcription Kit (Thermo Fisher Scientific, Waltham, MA, United States), according to the manufacturer’s protocol.
Real-time quantitative PCR (RT-qPCR) was performed using 15 ng of complementary DNA (cDNA), with a Rotor-Gene SYBR Green PCR Kit (Qiagen)5, using a Rotor Gene-Q(R) thermocycler (Venlo, Netherlands). Reactions were carried out in 15 μl total reaction volume: 7.5 μl of SYBR-green (QuantiFast SYBR Green PCR Kit – Qiagen), 0.3 μl of forward and reverse gene-specific primers, 1.5 μl of cDNA at 10 ng/μl, and 5.7 of RNase-DNase-free water. Three biological repetitions were used, and reactions were run in duplicate as technical replicates. Amplification was performed with the following reaction conditions: enzyme activation with 5 min at 95°C, then 40 cycles of 95°C for 5 s, followed by 10 s at 60°C, and completed by a melting curve analysis to assess the specificity of the reaction by raising the temperature from 60 to 95°C, with 1°C increase in temperature every 5 s. Relative fold differences were calculated based on the ΔΔCT method (Pfaffl, 2001), using MDH and RPL39 as reference genes (De Carvalho et al., 2013; Fernandes-Brum et al., 2017; Supplementary Table 3).
Statistical Analysis
For ACC, ethylene, ACO activity, and ABA, data analysis was performed using the InfoStat software6 (Di Rienzo et al., 2020). The statistical difference was determined by one-way ANOVA, followed by the Tukey test. Results were expressed as the mean ± SD. The values marked with different letters are significantly different at P < 0.05. For the gene expression, statistical analyses were performed by the R software (R Core Team, 2019). The expression rate and the confidence intervals were calculated according to the method proposed by Steibel et al. (2009), which considers the linear mixed model given by the following equation: yijklm = μ + TGijk + Il + eijklm where, yijklm is the Cq (Quantification cycle) obtained from the thermocycler software for the kth gene (reference or target) from the mth well, corresponding to the lth plant subject to the ith treatment (Wet, Dry, and Rainy) at the jth tissues (Leaf, Bud, and Root); TGijk is the effect of the combination of the ith treatment (May 18th, August 18th, and August 28th) at the jth tissues (Leaf, Bud, and Root). Graphics were performed with SigmaPlot v. 14 (Systat Software Inc.)7.
Results
Leaf Water Potential
In field experiment I, the leaf water potential was measured from −0.35 to −0.45 MPa in the rainy period (May 18th), decreasing dramatically at the end of the dry period (August 18th), reaching values from −2.5 to −2.7 MPa. After 22 mm of rain in the re-watering period (August 28th), the leaf water potential values increased to −1.5 MPa (Figure 1A). In field experiment II, the leaf water potential was measured only in the re-watering period, showing that values were higher in covered than in uncovered plants (Figure 1B).
Field Experiment I
1-Aminocyclopropane-1-Carboxylate Content
In general, higher ACC levels were observed in the cultivar Oeiras when compared to the other genotypes (1.5 times more than Acauã and Semperflorens). Within each cultivar, there were different patterns of ACC production across the periods. For Oeiras, ACC showed an increase along the sampling periods for leaves, flower buds, and roots [Figures 2(1A)], whereas, for Acauã, there was a decrease in the levels in the dry period for leaves and flower buds and an increase in the root, with increased levels being observed in all tissues after the rain event [Figures 2(1B)]. As for the cultivar Semperflorens, a pattern of ACC increase in the leaves was observed during the dry period, slightly decreasing in flower buds, and remaining stable in roots. Similar to the previous genotypes, ACC levels increased after the rain event for the leaves, flower buds, and roots [Figures 2(1C)].
Although all genotypes presented different patterns of ACC production in their different tissues between the rainy and dry periods, the first rain after the dry period provided increases of ACC observed in all tissues from the three coffee genotypes [Figure 2(1)]. Oeiras ACC levels increased 0.93, 2.77, and 2.7 times for leaves, flower buds, and roots, respectively, once plants were partially rehydrated. For Acauã plants, ACC also increased greatly in root and leaf (1.19 and 7.13 times, respectively), as well in Semperflorens, rain promoted ACC increases of 0.72 and 3.88 times for the leaves and roots, respectively, related to the dry period. When comparing the production of ACC among the three genotypes, it could be observed that there is a difference between Acauã and Oeiras where, although they showed similar ACC levels until the end of the dry period, ACC levels after the rain ranged from 800 to 1,200 nmol ACC/gr DW (Acauã) and 1,500 to 2,300 nmol ACC/gr DW (Oeiras) (Figures 2A,B). In Semperflorens plants the pattern of ACC production was similar to the one observed for Acauã plants [Figures 2(1C)].
Ethylene Production
Ethylene production showed different patterns among tissues and genotypes. Oeiras ethylene production decreased along the sampling periods for leaves and flower buds, with the opposite being observed for roots [Figures 2(2A)]. Acauã ethylene production decreased in leaves and increased for roots along the sampling periods, whereas for flower buds, an increase was observed during the dry period (0.94 times), decreasing after the rain in the re-watering period (0.54 times) [Figures 2(2B)]. In Semperflorens, ethylene production showed a similar pattern for the three tissues, decreasing during the dry period (2 times) and increasing once plants were partially rehydrated after the rain event (1 time) [Figures 2(2C)]. In general, ethylene production was similar for the three genotypes, showing a decreasing pattern in leaves along the sampling periods and an increasing pattern in roots of Oeiras and Acauã plants [Figures 2(2)].
1-Aminocyclopropane-1-Carboxylate Oxidase Activity
In Acauã and Oeiras, the ACO activity had a similar pattern [Figures 2(3A,B)], decreasing from the rainy period (May 18th) to the re-watering period (1.65 times) (August 28th) for leaves, and increasing in roots (0.87 times). Meanwhile, flower buds decreased ACO in the dry period (August 18th). In Semperflorens plants, ACO activity decreased from the rainy period (May 18th) to the dry period (1.23 times) (August 18th) for all tissues and increased in the re-watering period (August 28th) for leaves and buds but continued decreasing for roots [Figures 2(3C)].
Abscisic Acid Content
The ABA content was different among the analyzed sampling periods. In general, it was low in the rainy period (May 18th), increasing in the dry period (August 18th) and re-watering period (August 28th), observing different behaviors dependent on each cultivar. The ABA content was higher in Semperflorens than Oeiras and Acauã (5 and 10 times, respectively), especially for leaves. For Oeiras, the ABA level was similar for leaves, buds, and roots in the rainy period (May 18th), and showed higher levels for all tissues in the dry period but was even higher in leaves. Furthermore, in the re-watering period (August 28th), the ABA level decreased for all tissues, although was higher in leaves compared to other tissues, having levels similar to the dry period [Figures 2(4A)]. In Acauã leaves ABA content increased from the end of the rainy period to the re-watering period (3 times) (May 18th to August 28th), whereas, buds increased ABA from rainy to dry period (2 times) (May 18th to August 18th) and decreased in the re-watering period (August 28th). ABA in roots was high in the rainy period (May 18th), and stable during the dry and re-watering periods (August 18th and August 28th) [Figures 2(4B)]. In Semperflorens, the ABA content had a similar pattern to Oeiras, having low levels in the rainy period (May 18th), increasing in the dry period (August 18th), and decreasing in the re-watering period (August 28th) [Figures 2(4C)].
Lysine-Histidine Transporter 1 (LHT1) Gene Expression
In field experiment, I, the relative gene expression of the LHT1 transporter was compared for the same tissues (Leaves, buds, and roots) among sampling periods. In general, differences were observed in the relative gene expression for root and bud tissues in the re-watering period (August 28th) when compared to rainy and dry periods (May 18th and August 18th). For leaves tissues, no differences were observed between sampling periods (May 18th, August 18th, and August 28th) (Figure 3A). In field experiment II, the comparison of the LHT1 relative gene expression was made among leaves, buds, and roots for covered and uncovered plants in the re-watering period (August 28th). Results showed that LHT1 expression was higher in uncovered plants for the root and flower bud tissues, whereas, no differences in the relative gene expression were observed in leaves (Figure 3B), similarly to what was observed in leaves for field experiment I.
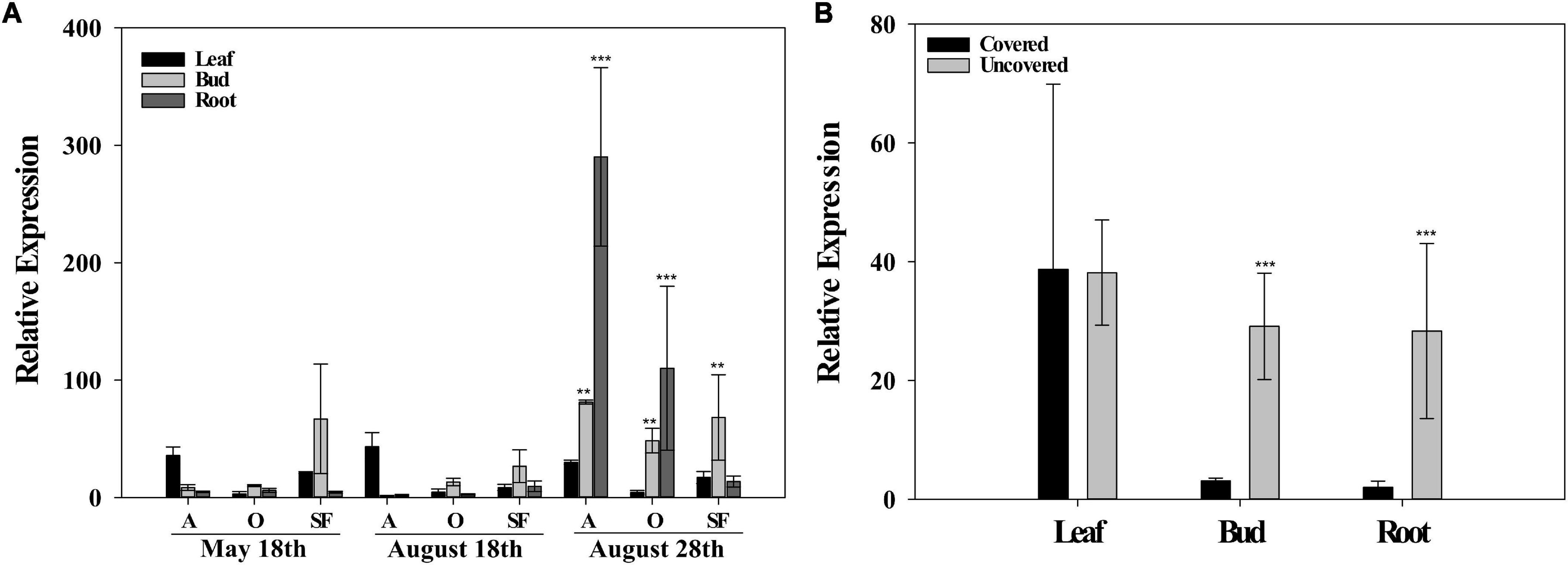
Figure 3. Relative expression of the lysine histidine transporter (LHT)1. (A) Field experiment I for the leaves, buds, and roots in rainy period (May 18th), dry period (August 18th), and re-watering period (August 28th) for Acauã (A), Oeiras (O), and Semperflorens (SF). (B) LHT1 relative expression in leaves, buds, and roots for field experiment II in re-watering period (August 28th) for Covered and Uncovered plants of coffee cultivar Paraiso 2. Data are means ± 95% SD of the mean (n = 6). ** = significative difference of tissues between periods and *** = high significant difference of tissues between periods.
Field Experiment II
The objective of field experiment II was to evaluate the effect of water on the evolution of ABA, ACC, ACO, and ethylene in the re-watering period (August 28th) using two treatments (Covered and uncovered plants). The content of ACC was higher in uncovered plants than in covered ones (Figure 4A). However, the ethylene production did not vary between treatments, as well as the ACO activity, except for buds that had higher production and activity in both parameters for uncovered plants (Figures 4B,C). On the other hand, the ABA content decreased in the uncovered plants (Figure 4D), possibly as an effect of the rehydration of the plant and the increase in the leaf water potential (Figure 1B).
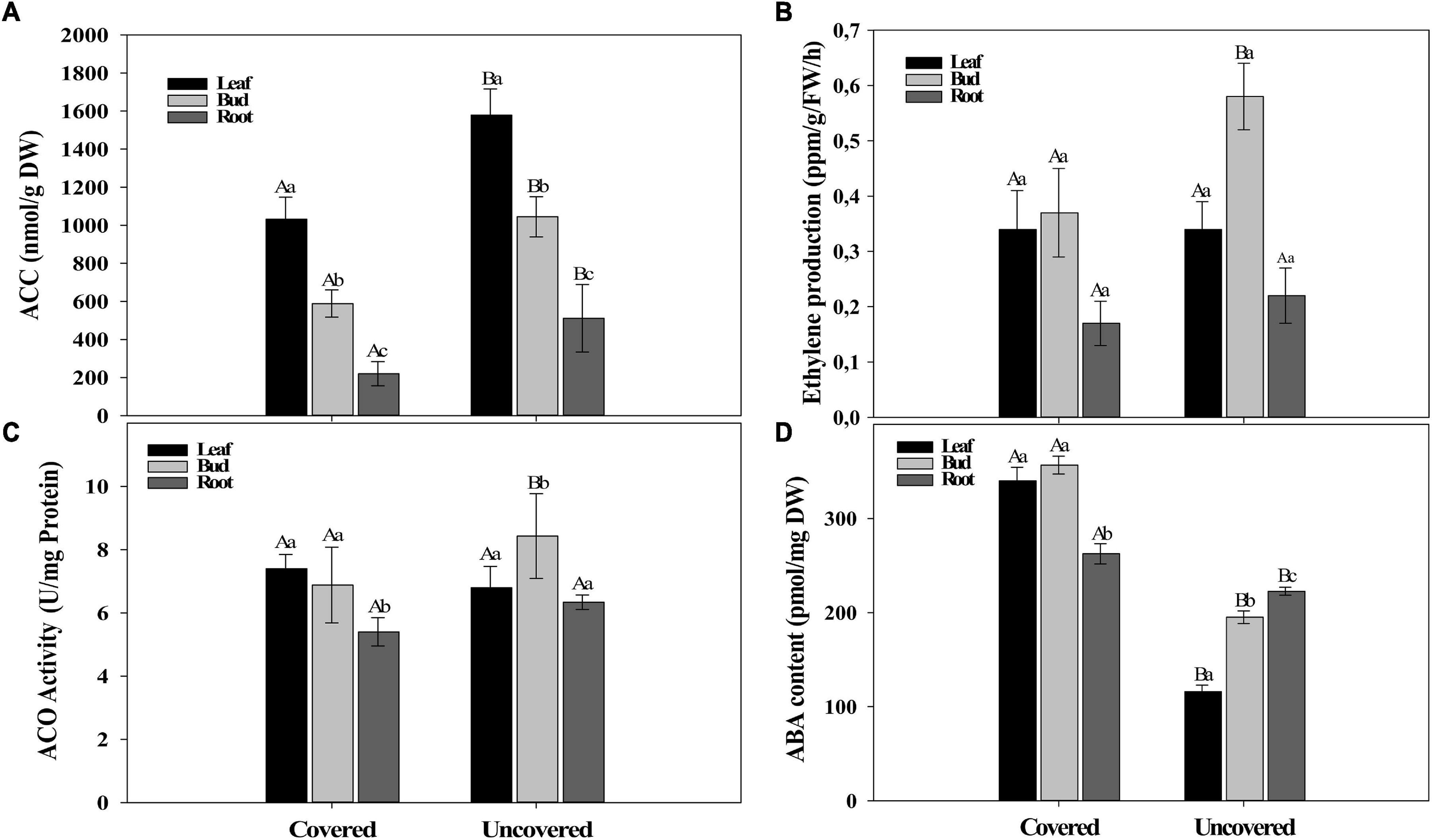
Figure 4. Phytohormones contents. (A) ACC, (B) Ethylene, (C) ACO, and (D) ABA quantification in leaves, flower buds, and roots in the field experiment II (covered and uncovered plants of Paraiso 2 coffee cultivar). Data are means ± 95% SD of the mean (n = 6). Different upper-case and lower-case letters indicate statistical differences of each tissue among the different treatments and the different tissues within the same treatment, respectively.
Field Experiment III
Coffee plants that had an exogenous application of ACC showed an increase in the number of flower buds (at the G6 stage). The number of flower buds at the G6 stage was higher in the treatment with ACC (14 flower buds – 3.63 times), followed by the treatment with 1-MCP + ACC (8 flower buds – 2.2 times), and 1-MCP (6 flower buds – 1.63 times) compared with the control treatment (3 flower buds) (Figure 5A). These results are congruent with the statistical differences found among treatments for flower buds atG6 stage (Figure 5B). Because all coffee plants had after 3 days of treatments imposition and 35 mm rain, the number of flowers bud at G6 stage in control treatment represent the effect of rain in coffee anthesis and the differences between control and the other treatments [(ACC), (1-MCP + ACC) and (1-MCP)] represent the effect those treatments on coffee anthesis.
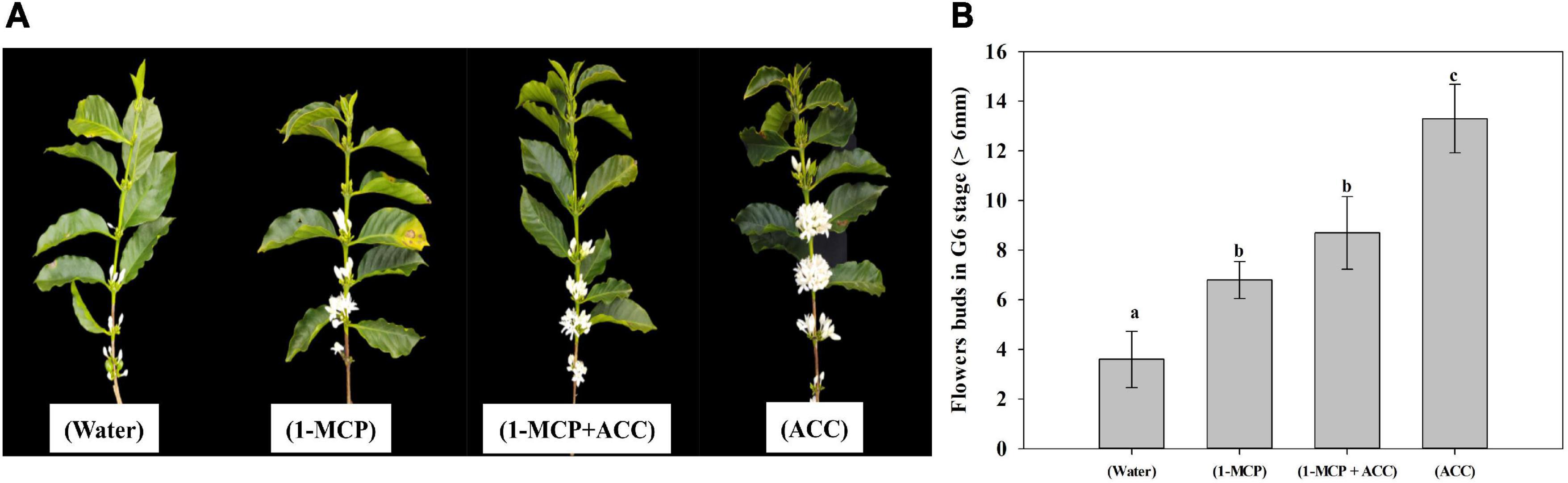
Figure 5. Effect of ACC treatment in coffee anthesis. (A) Branches with the opened flower as effect different treatments. (B) Statistical differences among treatments with the application of exogenous ACC and other treatments in flower buds at G6 stage.
Discussion
Water Stress and Flowering
The transition from the vegetative to reproductive phase is a very important event for plants because flowering time is crucial to ensure the success of next-generation and species perpetuity (Romera-Branchat et al., 2014; Song et al., 2015). This phase is marked by different endogenous processes that respond to environmental stimuli. Diverse stress factors can induce, accelerate, inhibit, or delay flowering in many species of plant including water deficit stress (Takeno, 2016). For field experiment, I, coffee plants of Oeiras, Acauã, and Semperflorens genotypes were under water deficit stress and leaf water potential reached −2.5 MPa in the dry period (August 18th) and then the average value of −1.5 MPa after rain in the re-watering period (August 28th) (Figure 1A) with anthesis occurring 10 days after 22 mm of rain (Figure 6A). For field experiment II, covered and uncovered plants showed differences in leaf water potential (Figure 1B), with only uncovered plants having anthesis (Figure 6B). Those results are corroborating that water is an important trigger of the coffee anthesis process.
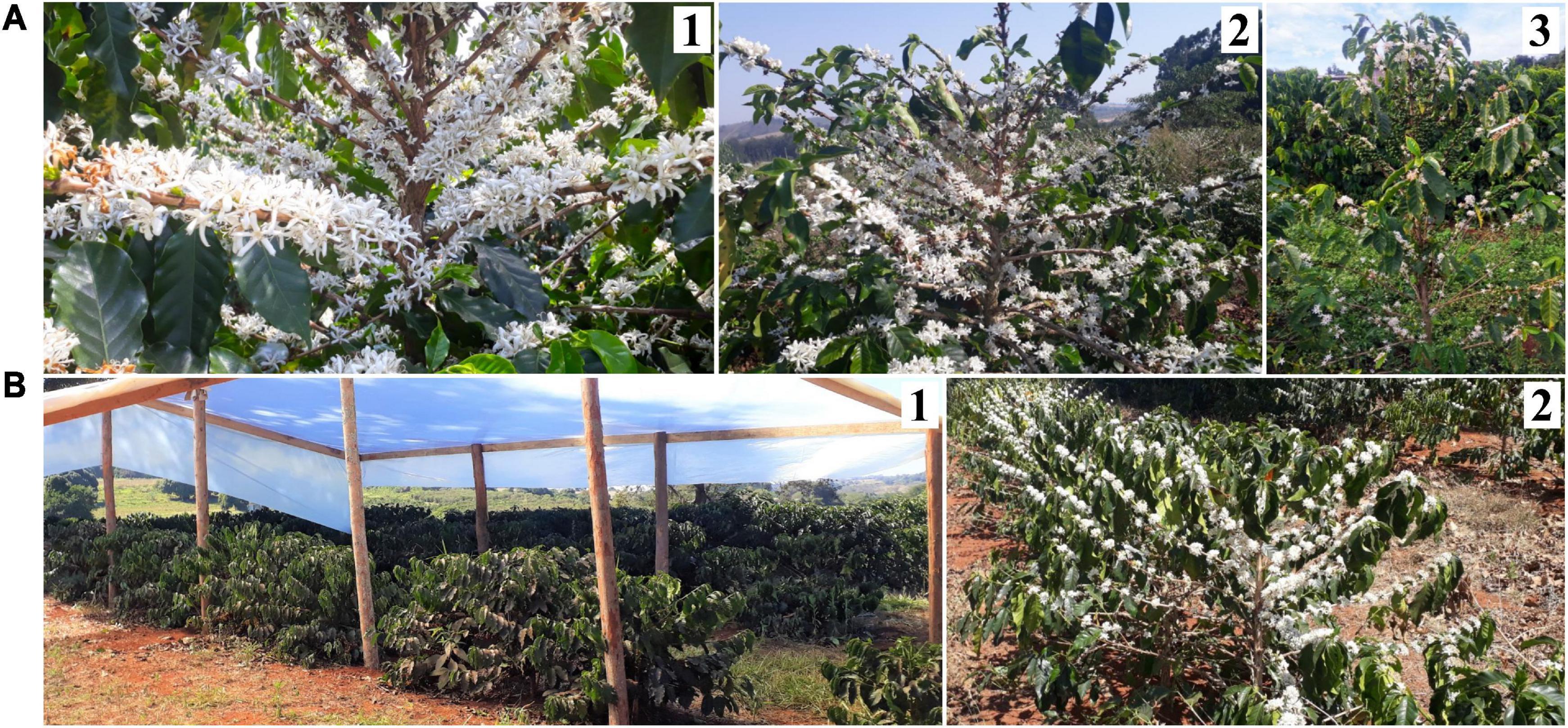
Figure 6. Coffee anthesis in (A) Oeiras (1), Acauã (2), and Semperflorens (3) in field experiment I. (B) Non-flowering and flowering plants of Paraiso 2 coffee cultivar using treatments of Covered and Uncovered environment at the field experiment II.
This effect can be observed in many tropical and subtropical trees, such as citrus, the most classic example. It was observed that experiments with lemon (Citrus limon L. Burm. f.) under a temperature range of 18–30°C, flowering was controlled by drought stress (Chaikiattiyos et al., 1994), and similar results were reported for “Tahiti” lime (Citrus latifolia Tanaka) as well (Southwick and Davenport, 1986). In Litchi (Litchi chinensis Sonn); the autumnal water stress period increased significantly flowering intensity and yield (Stern et al., 1993), such as in Star fruit (Averrhoa carambola L.) (Wu et al., 2017), Longan (Dimocarpus longan Lour.) (Zhou et al., 2014), and Coffee (C. arabica L.) (Crisosto et al., 1992).
In the agroecological conditions of Brazil, coffee anthesis occurs after a short rainy event preceded by a water deficit period during the winter, which is necessary to break the bud “latent state,” associated with endogenous and external factors (Ronchi and Miranda, 2020). One of the most important factors that contribute to flowering under water deficit stress conditions are phytohormones (Izawa, 2021). The main plant hormone studied in response to water stress is ABA, because it acts as an important signaling mediator for plants’ adaptive response to a variety of environmental stresses regulating many physiological processes, including bud dormancy, seed germination, stomatal development, and transcriptional and post-transcriptional regulation of stress-responsive gene expression (Ali et al., 2020).
For the three coffee genotypes, ABA levels increased from rainy to dry period (May 18th to August 18th) in the leaves and buds, which can be explained by the higher activity for stomatal closure in leaves to cope with the water restriction at this dry period. For Oeiras and Semperflorens, ABA content decreased after a re-watering period (August 28th), whereas in Acauã cultivar, the ABA content increased, as was observed by Martins et al. (2019). This contrast in ABA content can be associated with the intrinsic and phenotypic characteristics of flowering and ripening fruit patterns of each coffee cultivar, where Acauã is considered to be late, Oeiras is early, and Semperflorens is continuous. When the ABA level increases, it negatively modulates ethylene biosynthesis and ABA has shown a positive effect on floral transition under water stress, being able to participate in early flowering known as Drought Escape (Verslues and Juenger, 2011). Despite the ABA variation level between Acauã and the other genotypes, in this experiment for all coffee genotypes, ethylene production decreased from rainy to re-watering periods (May 18th to August 28th) in leaves, possibly due to the interaction of ABA in response to the water stress deficit.
Abscisic Acid-Ethylene Regulation
Abscisic Acid and ethylene are well-known phytohormones involved in the regulation of physiological processes in plants (Müller and Hasanuzzaman, 2021). They participate mainly in plant growth regulation, biotic and abiotic stress responses, bud and seed dormancy, leaf and flower senescence, fruit ripening, germination, and flowering (Binder, 2020; Chen et al., 2020). In our study, in field experiment I, basically, the ABA content was higher in the leaves and flower buds than in the root in the dry period for all genotypes (August 18th) [Figures 2(4)], probably because ABA is very active in leaves and buds due to water deficit to keep stomatal conductance in leaves (McAdam and Brodribb, 2018). Conversely, ethylene production decreased [Figures 2(2)] coinciding with a reduction of ACO activity, which serves as a catalyst in the oxidative process for the conversion of ACC into ethylene. This antagonism in response to water stress has been observed in other species directly related to the closing of the stomata to avoid dehydration (Daszkowska-Golec and Szarejko, 2013).
Concerning flowering, it is known that ethylene could act negatively by inhibiting flowering in A. thaliana (Achard et al., 2007) or delaying in rice (Wang Y. et al., 2013), demonstrating that ethylene signaling is delayed in both species. However, a positive effect on promoting flowering has been observed in pineapple (Ananas comosus L. Merr) (Trusov and Botella, 2006) and lilies (Triteleia laxa Bentham) (Han et al., 1989). Recently, it was proposed that ethylene is involved directly in coffee anthesis by the changes in the biosynthesis pathway and regulatory genes expression (Lima et al., 2020). In other words, the direct effect of ABA in flowering is not well understood, despite there being some reports about positive and negative influences in flowering (Izawa, 2021). Exogenous application of ABA negatively regulates flowering in A. thaliana, represented by AtABI5 overexpression delaying floral transition by upregulating FLOWERING LOCUS C (FLC) expression (Wang Y. et al., 2013).
In the same sense, ABA represses flowering by modulating SOC1 at the apex, when CO is needed by FT in the ABA-dependent floral induction (Riboni et al., 2013). The most known positive effect of ABA on flowering is the drought escape, which is a plant mechanism to avoid drought damage and reflecting in early flowering, to produce seeds before being affected by severe drought stress conditions (Franks, 2011; Gupta et al., 2020). Early flowering is characterized by an ABA increased level in response to water stress (Sherrard and Maherali, 2006; Shavrukov et al., 2017).
At the rainy period (May 18th), coffee plants showed no water deficit stress (−0.25 MPa) and the ethylene levels were higher than ABA, contrasting with the dry period (August 18th), where ethylene level decreased and ABA production increased in coffee plants under water deficit stress (−2.5 MPa) in leaves and flower bud. These results show a clear and opposite behavior in phytohormones content related to water stress conditions in coffee plants (Figure 2). This behavior suggests a direct ABA regulation on ethylene production, possibly by the downregulation of ACO activity. Negative ABA regulation in the ACO activity was observed in Hibiscus (Hibiscus rosa-sinensis L.) when activation of the ethylene biosynthesis pathway was reduced by exogenous ABA treatment (Trivellini et al., 2011). Similar results were observed in Lepidio (Lepidium sativum L.) when ABA inhibited seed germination decreasing ethylene content by the Lepidium ACO2 gene expression regulation (Linkies et al., 2009). Moreover, in Sugar beet, the radicle emergence is regulated by ABA-ethylene antagonism that affects ACC and ACO gene expression (Hermann et al., 2007) and, in Tomato (Solanum lycopersicum L.) was observed upregulation in ripening (Zhang et al., 2009).
In our research, anthesis occurred after a re-watering period (August 28th) by rain, the ethylene content continued to decrease, coinciding with a decrease in the ACO activity. Under Brazilian environmental conditions, coffee flowering is expected after a re-watering period (Lima et al., 2020). This behavior was observed for the leaves and buds, whereas in the roots, it was the opposite. The ABA content decreased in the leaves and flower buds for the Oeiras and Semperflorens genotypes, possibly because the water content for their rehydration was sufficient enough to reduce the water deficit stress in the plant, whereas in the Acauã cultivar, the ABA content increased in the leaves due to persistent water deficit stress. The regulation of stress response in plants by ABA and ethylene depends on the duration of stress, its genetic potential, the environmental conditions, and the developmental stages of the plant (Müller and Hasanuzzaman, 2021).
In field experiment II, the data from ACC, ABA, ethylene, and ACO were taken only after a re-watering period (August 28th) to support the previously hypothesized behavior in the coffee plant after rain. The results showed an increased level of ACC and decreased level of ethylene and ACO activity whereas ABA decreased after rain in response to rehydration of coffee plants (Figure 4). It is known that ABA is associated with drought tolerance in coffee since exogenous ABA applications in seedlings increase the relative water content in the soil and delayed the wilting point of coffee plants (Vu et al., 2020). These results corroborate with the results obtained in field experiment I, where an increased level of ACC was observed over an antagonistic relation between ABA and ethylene, participating in coffee anthesis after a water stress period. This is the first report of increased ACC levels associated with coffee flowering, supporting the theory that ACC is involved in many metabolic processes in plants (Vanderstraeten and van Der Straeten, 2017). Also, principal component analysis (PCA) and correlation analysis reinforce the antagonism in the evolution of ABA and ethylene compared in the three sampled periods (Supplementary Figure 3).
1-Aminocyclopropane-1-Carboxylate Beyond Ethylene Precursor, Acting as a Possible Flowering Signaling
Since ACC was discovered (Adams and Yang, 1979), it has always been described as an ethylene precursor, and external applications of ACC are used for many experiments with ethylene (Elías et al., 2018). However, new pieces of evidence and recent discoveries showed that ACC can act as a signaling molecule in plants, independently of ethylene. One of the most remarkable results of our experiment is the increase of ACC in all tissues and coffee genotypes after rain in the re-watering period (August 28th) [Figure 2(1)]. In our initial hypothesis, we believed that ACC accumulated in the root during the dry period and transported to the plant aerial part to become ethylene after the rain would be involved in the coffee anthesis (Lima et al., 2020).
However, this study showed that the increase in ACC found in all tissues does not have a positive correlation with the ethylene production in the coffee plant aerial part (buds and leaves), which coincides with a low activity of the ACO enzyme in the field experiment I and corroborated with the results in the field experiment II. It is possible that ACC, in addition to being a precursor molecule of ethylene, is involved in the fecundation and anthesis process in coffee. The results of the field experiment III allow us to confirm this hypothesis because the treatment imposition with exogenous ACC showed a higher number of flower buds at the G6 stage as compared with control treatment (Water) (Figures 5A,B). This high level of ACC observed in all tissues, after rain in the re-watering period (August 28th) [Figure 2(1)], could be derived from the amino acid methionine (Precursor of ACC) accumulated during the dry period, as a plant response to alleviate the water stress period as observed in previous studies in coffee (Marcheafave et al., 2019), wheat (le Roux et al., 2020), and Bitter gourd (Akram et al., 2020).
In the III field experiment, the number of opened and G6 stage flower buds of the treatment with 1-MCP were higher than that of control treatment, agreed with Supplementary Figure 2 and, indicating that ethylene inhibited coffee anthesis. There was no difference in flowering number between the 1-MCP + ACC treatment and 1-MCP treatment, the reason may be that the 1-MCP treatment promoted ethylene synthesis and decreased ACC concentration; or/and 1-MCP treatment inhibited the expression of LHT1. However, the number of flowering in ACC treatment was higher than that in 1-MCP treatment and 1-MCP + ACC treatment, the mechanism may be that the inhibition of LHT1 expression by 1-MCP was relieved, or the signal effect of ACC was stronger than that of ethylene. To confirm the regulatory effect of ACC on coffee flowering, co-treatment with Co2+, AIB and 1-MCP in the presence or absence of ACC could allow the study of the signaling effect of ACC (Le Deunff and Lecourt, 2016). In Arabidopsis, Mou et al. (2020) found that ACC in ovules stimulates transient Ca2+ elevation and Ca2+ influx, and this signaling in ovular sporophytic tissue is involved in pollen tube attraction. ACC may participate as signaling in pollen tube attraction in the coffee flower fecundation process. This process is very important in the coffee crop since the anthesis only occurs between 10 and 12 days after the rain, at which time flower fecundation has already occurred in a 90% because the coffee plant is self-pollinated (Veddeler et al., 2006).
1-aminocyclopropane-1-carboxylate has been shown to regulate some physiological mechanisms in plants such as stomatal development by guard cell differentiation (Yin et al., 2019), cell wall metabolism (Hofmann, 2008; Xu et al., 2008; Tsang et al., 2011), and vegetative development (Tsuchisaka et al., 2009; Vanderstraeten et al., 2019). In addition, it has been found that ACC has a distinct function than ethylene in the non-vascular plant Marchantia polymorpha (Li et al., 2020; Katayose et al., 2021) and sexual reproduction induction in the Marine red alga Pyropia yezoensis (Uji et al., 2020). In our study, the LHT1 expression, an intracellular amino acid transporter that also transports ACC (Shin et al., 2015), was evaluated. Although its best-known function is amino acid transporters of ACC, it might be involved in many biological processes related to ethylene (Yang et al., 2020).
In field experiment I, the LHT1 expression was higher in all coffee genotypes after rain in the re-watering period (August 28th), especially for roots and buds (Figure 3A), as well as in the field experiment II, wherein the uncovered coffee plants the LHT1 expression was higher for buds and roots than in covered plants, whereas, in leaves, it did not show the difference (Figure 3B). Notably, there was an increase in ACC in the coffee plant tissues [Figure 2(1)], which coincides with a higher LHT1 expression after the rain (Figure 3A), showing that LHT1 transporter might be associated with ACC intracellular transportation (Figure 4A). The LHT amino acid transporters family are linked with flower development because LHT2 and LHT4 were expressed in the tapetum, suggesting their role in delivering amino acids to pollen grains in Arabidopsis (Lee and Tegeder, 2004; Foster et al., 2008). Moreover, LHT5 and LHT6 expressions were detected along the transmitting tract of the pistil and the pollen tube, pointing to a function in amino acid uptake for successful fertilization (Foster et al., 2008). The study represents the first report of LHT1 transporter gene expression associated with ACC in coffee, suggesting intracellular transport and most likely, participating in the coffee anthesis. In light of these results, we propose that ACC participates in the anthesis process in the coffee plant, as represented in Figure 7.
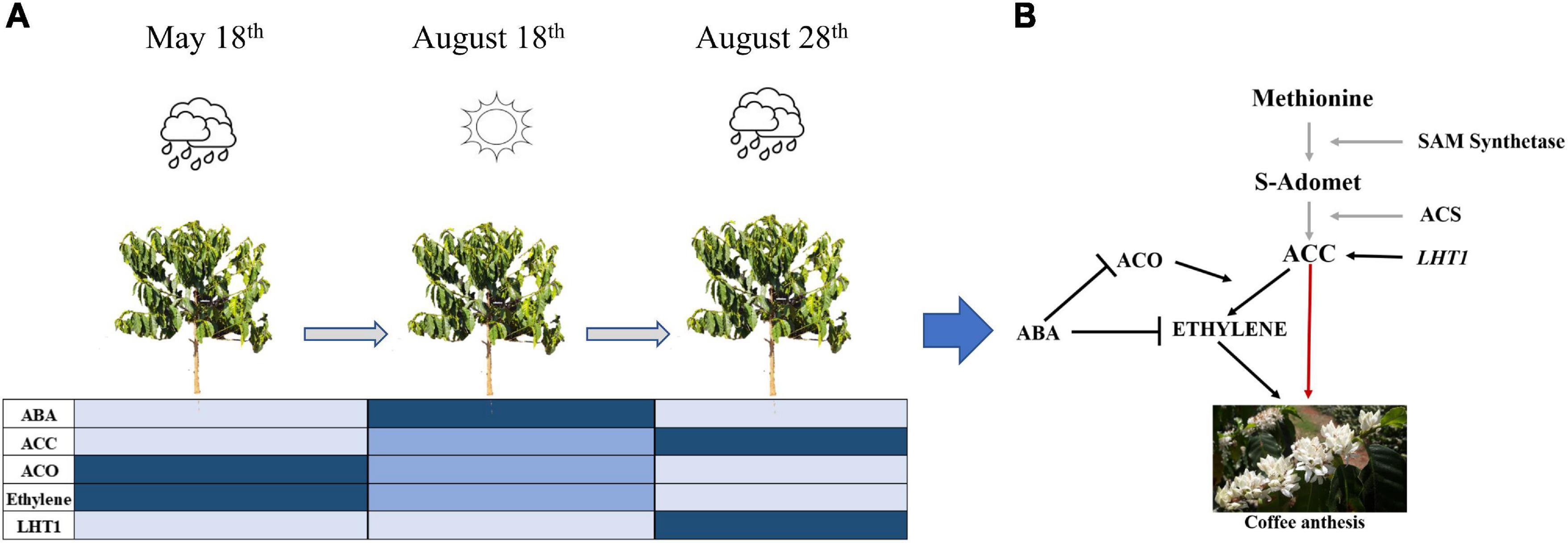
Figure 7. Proposed model of ABA-ACO-Ethylene regulation favoring ACC increased level influencing coffee anthesis in the ethylene biosynthesis pathway. (A) Evolution of phytohormones (ABA, ACC, ACO, Ethylene) and LHT1 transporter measured in this study by the sampling times (May 18th, August 18th, and August 28th). The color intensity in the chart means the level of plant hormone in the coffee plant as a whole (Light blue = low, blue = medium, dark blue = high). (B) Relationship among phytohormones according to our proposal, the black arrow means established plant hormones relation in this study, gray ones mean part of ethylene biosynthesis process described in the literature, the red arrow means a proposal of this study of ACC acting in coffee anthesis.
Conclusion
The results of this study showed that ACC acts as a modulator in the coffee flowering process, displaying more concentration in the anthesis period. We suggest that ACC has its activity modulated independently of the ethylene biosynthesis pathway. Furthermore, ABA is involved in the process, especially, because coffee anthesis is preceded by a water stress deficit period in which this plant hormone plays an important role in stomatal closure. On the other hand, this is the first report of LHT1 as an ACC transporter in coffee associated with anthesis. These results suggest that the increased ABA level during the dry period regulates the ACO activity decreasing the ethylene content and increasing the ACC level throughout an intracellular transport in roots, flower buds, and leaves, this may be directly associated with both fecundation and anthesis process in coffee in the re-watering period. Re-watering is an inductive stimulus for coffee anthesis after a water deficit period. Our results corroborate that making the water an important trigger for physiological processes of coffee anthesis is proven by covered plants not presenting anthesis while uncovered coffee plants bloomed.
Data Availability Statement
The original contributions presented in the study are included in the article/Supplementary Material, further inquiries can be directed to the corresponding author.
Author Contributions
ML, IS, AA, and AC-J designed the study. ML, IS, JE, and RM performed the field experiments. ML, RM, IS, and AJ performed the laboratory analysis. ML, RM, and CC analyzed the data. ML drafted the manuscript. All authors contributed to its improvement and agreed to its final content.
Funding
This work was supported by the “Coordenação de Aperfeiçoamento de Pessoal de Niìvel Superior (CAPES)”, and “Instituto Brasileiro de Ciência e Tecnologia do Café” (INCT/Café), under FAPEMIG grant (CAG APQ 03605/17). The Honduran Foundation for Agricultural Research (FHIA), “Programa Becas Honduras 2020” helped financially in support the ML.
Conflict of Interest
The authors declare that the research was conducted in the absence of any commercial or financial relationships that could be construed as a potential conflict of interest.
Publisher’s Note
All claims expressed in this article are solely those of the authors and do not necessarily represent those of their affiliated organizations, or those of the publisher, the editors and the reviewers. Any product that may be evaluated in this article, or claim that may be made by its manufacturer, is not guaranteed or endorsed by the publisher.
Acknowledgments
We would like to acknowledge AgroFresh Company, for kindly providing the Harvista used in the experiments.
Supplementary Material
The Supplementary Material for this article can be found online at: https://www.frontiersin.org/articles/10.3389/fpls.2022.824948/full#supplementary-material
Footnotes
- ^ https://www.arabidopsis.org/index.jsp
- ^ http://www.ncbi.nlm.nih.gov/
- ^ https://www.megasoftware.net/
- ^ https://www.thermofisher.com/br/en/home.html
- ^ https://www.qiagen.com/us/
- ^ https://www.infostat.com.ar/
- ^ http://www.sigmaplot.co.uk/
References
Achard, P., Baghour, M., Chapple, A., Hedden, P., Van Der Straeten, D., Genschik, P., et al. (2007). The plant stress hormone ethylene controls floral transition via DELLA-dependent regulation of floral meristem-identity genes. Proc. Natl. Acad. Sci. U.S.A. 104, 6484–6489. doi: 10.1073/pnas.0610717104
Adams, D. O., and Yang, S. F. (1979). Ethylene biosynthesis: Identification of 1-aminocyclopropane-1-carboxylic acid as an intermediate in the conversion of methionine to ethylene. Proc. Natl. Acad. Sci. U.S.A. 76, 170–174. doi: 10.1073/pnas.76.1.170
Akram, N. A., Umm-e-Hani, Ashraf, M., Ashraf, M., and Sadiq, M. (2020). Exogenous application of L-methionine mitigates the drought-induced oddities in biochemical and anatomical responses of bitter gourd (Momordica charantia L.). Sci. Hortic. (Amsterdam) 267, 109333. doi: 10.1016/J.SCIENTA.2020.109333
Ali, S., Hayat, K., Iqbal, A., and Xie, L. (2020). Implications of abscisic acid in the drought stress tolerance of plants. Agron 10, 1323. doi: 10.3390/AGRONOMY10091323
Alonso, J. M., and Ecker, J. R. (2001). The ethylene pathway: a paradigm for plant hormone signaling and interaction. Sci. STKE 2001:re1. doi: 10.1126/STKE.2001.70.RE1
An, F., Zhao, Q., Ji, Y., Li, W., Jiang, Z., Yu, X., et al. (2010). Ethylene-induced stabilization of ETHYLENE INSENSITIVE3 and EIN3-LIKE1 is mediated by proteasomal degradation of EIN3 binding F-box 1 and 2 that requires EIN2 in arabidopsis. Plant Cell 22, 2384–2401. doi: 10.1105/tpc.110.076588
Binder, B. M. (2020). Ethylene signaling in plants. J. Biol. Chem. 295, 7710. doi: 10.1074/JBC.REV120.010854
Bradford, M. M. (1976). A rapid and sensitive method for the quantitation of microgram quantities of protein utilizing the principle of protein-dye binding. Anal. Biochem. 72, 248–254. doi: 10.1006/ABIO.1976.9999
Bulens, I., Van de Poel, B., Hertog, M. L. A. T. M., De Proft, M. P., Geeraerd, A. H., and Nicolaï, B. M. (2011). Protocol: an updated integrated methodology for analysis of metabolites and enzyme activities of ethylene biosynthesis. Plant Methods 7, 1–10. doi: 10.1186/1746-4811-7-17
Camargo, ÂP. (1985). Florescimento e frutificação de café arábica nas diferentes regiões (cafeeiras) do Brasil. Pesqui. Agropecu. Bras. 20, 831–839.
Chaikiattiyos, S., Menzel, C. M., and Rasmussen, T. S. (1994). Floral induction in tropical fruit trees: effects of temperature and water supply. J. Hortic. Sci. 69, 397–415. doi: 10.1080/14620316.1994.11516469
Chen, K., Li, G. J., Bressan, R. A., Song, C. P., Zhu, J. K., and Zhao, Y. (2020). Abscisic acid dynamics, signaling, and functions in plants. J. Integr. Plant Biol. 62, 25–54. doi: 10.1111/JIPB.12899
Choi, J., Eom, S., Shin, K., Lee, R. A., Choi, S., Lee, J. H., et al. (2019). Identification of lysine histidine transporter 2 as an 1-aminocyclopropane carboxylic acid transporter in Arabidopsis thaliana by transgenic complementation approach. Front. Plant Sci. 10:1092. doi: 10.3389/fpls.2019.01092
Crisosto, C. H., Grantz, D. A., and Meinzer, F. C. (1992). Effects of water deficit on flower opening in coffee (Coffea arabica L.). Tree Physiol. 10, 127–139. doi: 10.1093/treephys/10.2.127
Daszkowska-Golec, A., and Szarejko, I. (2013). Open or close the gate – stomata action under the control of phytohormones in drought stress conditions. Front. Plant Sci. 4:138. doi: 10.3389/FPLS.2013.00138
De Carvalho, K., Bespalhok Filho, J. C., Dos Santos, T. B., De Souza, S. G. H., Vieira, L. G. E., Pereira, L. F. P., et al. (2013). Nitrogen starvation, salt and heat stress in coffee (Coffea arabica L.): identification and validation of new genes for qPCR normalization. Mol. Biotechnol. 53, 315–325. doi: 10.1007/S12033-012-9529-4
de Oliveira, R. R., Cesarino, I., Mazzafera, P., and Dornelas, M. C. (2014). Flower development in Coffea arabica L.: new insights into MADS-box genes. Plant Reprod. 27, 79–94. doi: 10.1007/s00497-014-0242-2
Di Rienzo, J. A., Casanoves, F., Balzarini, M. G., González, L., Tablada, M., and Robledo, C. W. (2020). InfoStat versión 2020. Córdoba: Centro de Transferencia InfoStat, FCA, Universidad Nacional de Córdoba.
Dong, J. G., Fernandez-Maculet, J. C., and Yang, S. F. (1992). Purification and characterization of 1-aminocyclopropane-1-carboxylate oxidase from apple fruit. Proc. Natl. Acad. Sci. U.S.A. 89, 9789–9793. doi: 10.1073/PNAS.89.20.9789
Drinnan, J. E., and Menzel, C. M. (1994). Synchronization of anthesis and enhancement of vegetative growth in coffee (Coffea arabica L.) following water stress during floral initiation. J. Hortic. Sci. 69, 841–849. doi: 10.1080/14620316.1994.11516520
Elías, J. M., Guerrero-Molina, M. F., Martínez-Zamora, M. G., Díaz-Ricci, J. C., and Pedraza, R. O. (2018). Role of ethylene and related gene expression in the interaction between strawberry plants and the plant growth-promoting bacterium Azospirillum brasilense. Plant Biol. (Stuttg.) 20, 490–496. doi: 10.1111/PLB.12697
Fa Yang, S., and Hoffman, N. E. (1984). Ethylene biosynthesis and its regulation in higher plants. Annu. Rev. Plant Physiol. 35, 155–189. doi: 10.1146/annurev.pp.35.060184.001103
Fernandes-Brum, C. N., Garcia, B. O., Moreira, R. O., Ságio, S. A., Barreto, H. G., Lima, A. A., et al. (2017). A panel of the most suitable reference genes for RT-qPCR expression studies of coffee: screening their stability under different conditions. Tree Genet. Genomes 13, 131. doi: 10.1007/s11295-017-1213-1
Foster, J., Lee, Y. H., and Tegeder, M. (2008). Distinct expression of members of the LHT amino acid transporter family in flowers indicates specific roles in plant reproduction. Sex. Plant Reprod. 21, 143–152. doi: 10.1007/S00497-008-0074-Z
Franks, S. J. (2011). Plasticity and evolution in drought avoidance and escape in the annual plant Brassica rapa. New Phytol. 190, 249–257. doi: 10.1111/J.1469-8137.2010.03603.X
Gupta, A., Rico-Medina, A., and Caño-Delgado, A. I. (2020). The physiology of plant responses to drought. Science 368, 266–269. doi: 10.1126/SCIENCE.AAZ7614
Han, S., Halevy, A. H., Sachs, R. M., and Reid, M. S. (1989). Effect of ethylene on growth and flowering of Triteleia laxa. Acta hortic. 261, 209–214. doi: 10.17660/actahortic.1989.261.26
Hermann, K., Meinhard, J., Dobrev, P., Linkies, A., Pesek, B., Heß, B., et al. (2007). 1-Aminocyclopropane-1-carboxylic acid and abscisic acid during the germination of sugar beet (Beta vulgaris L.): a comparative study of fruits and seeds. J. Exp. Bot. 58, 3047–3060. doi: 10.1093/JXB/ERM162
Hofmann, N. R. (2008). New role for ACC in cell wall biosynthesis. Plant Cell 20:2928. doi: 10.1105/TPC.108.201111
Izawa, T. (2021). What is going on with the hormonal control of flowering in plants? Plant J. 105, 431–445. doi: 10.1111/TPJ.15036
Katayose, A., Kanda, A., Kubo, Y., Takahashi, T., and Motose, H. (2021). Distinct functions of ethylene and ACC in the basal land plant Marchantia polymorpha. Plant Cell Physiol. 62, 858–871. doi: 10.1093/pcp/pcab042
Le Deunff, E., and Lecourt, J. (2016). Non-specificity of ethylene inhibitors: ‘double-edged tools to find out new targets involved in the root morphogenetic programme. Plant Biol. 18, 353–361. doi: 10.1111/plb.12405
le Roux, M. S. L., Burger, N. F. V., Vlok, M., Kunert, K. J., Cullis, C. A., and Botha, A. M. (2020). Wheat line “RYNO3936” is associated with delayed water stress-induced leaf senescence and rapid water-deficit stress recovery. Front. Plant Sci. 11:1053. doi: 10.3389/FPLS.2020.01053/BIBTEX
Lee, Y. H., and Tegeder, M. (2004). Selective expression of a novel high-affinity transport system for acidic and neutral amino acids in the tapetum cells of Arabidopsis flowers. Plant J. 40, 60–74. doi: 10.1111/J.1365-313X.2004.02186.X
Li, D., Flores-Sandoval, E., Ahtesham, U., Coleman, A., Clay, J. M., Bowman, J. L., et al. (2020). Ethylene-independent functions of the ethylene precursor ACC in Marchantia polymorpha. Nat. Plants 6, 1335–1344. doi: 10.1038/s41477-020-00784-y
Lima, A. A., Santos, I. S., Lopez, M. E., Cardon, C. H., Frois, C. F., Davies, W. J., et al. (2020). Drought and re-watering modify ethylene production and sensitivity, and are associated with coffee anthesis. Environ. Exp. Bot. 181:104289. doi: 10.1016/j.envexpbot.2020.104289
Linkies, A., Müller, K., Morris, K., Tureèková, V., Wenk, M., Cadman, C. S. C., et al. (2009). Ethylene interacts with abscisic acid to regulate endosperm rupture during germination: a comparative approach using Lepidium sativum and Arabidopsis thaliana. Plant Cell 21, 3803–3822. doi: 10.1105/tpc.109.070201
Liu, N., Ding, Y., Fromm, M., and Avramova, Z. (2014). Endogenous ABA extraction and measurement from Arabidopsis leaves. Bio Protoc. 4:e1257. doi: 10.21769/BIOPROTOC.1257
Marcheafave, G. G., Tormena, C. D., Afonso, S., Rakocevic, M., Bruns, R. E., and Scarminio, I. S. (2019). Integrated chemometric approach to optimize sample preparation for detecting metabolic changes provoked by abiotic stress in Coffea arabica L. leaf fingerprints. J. Braz. Chem. Soc. 30, 2085–2094. doi: 10.21577/0103-5053.20190068
Martins, S. C. V., Sanglard, M. L., Morais, L. E., Menezes-Silva, P. E., Mauri, R., Avila, R. T., et al. (2019). How do coffee trees deal with severe natural droughts? An analysis of hydraulic, diffusive and biochemical components at the leaf level. Trees Struct. Funct. 33, 1679–1693. doi: 10.1007/S00468-019-01889-4/FIGURES/6
Matsumoto, T. K., and Lopez, J. (2014). “Coffee harvest management by manipulation of coffee flowering with plant growth regulators,” in Proceedings of the XXIX International Horticultural Congress on Horticulture: Sustaining Lives, Livelihoods and Landscapes (IHC2014), Brisbane: Australia. Vol. 1130, 219–224. doi: 10.17660/actahortic.2016.1130.32
McAdam, S. A. M., and Brodribb, T. J. (2018). Mesophyll cells are the main site of abscisic acid biosynthesis in water-stressed leaves. Plant Physiol. 177, 911–917. doi: 10.1104/PP.17.01829
Meng, Y., Ma, N., Zhang, Q., You, Q., Li, N., Ali Khan, M., et al. (2014). Precise spatio-temporal modulation of ACC synthase by MPK6 cascade mediates the response of rose flowers to rehydration. Plant J. 79, 941–950. doi: 10.1111/tpj.12594
Morais, H., Caramori, P. H., Koguishi, M. S., and De Arruda Ribeiro, A. M. (2008). Escala fenológica detalhada da fase reprodutiva de Coffea arabica. Bragantia 67, 257–260. doi: 10.1590/s0006-87052008000100031
Mou, W., Kao, Y. T., Michard, E., Simon, A. A., Li, D., Wudick, M. M., et al. (2020). Ethylene-independent signaling by the ethylene precursor ACC in Arabidopsis ovular pollen tube attraction. Nat. Commun. 11, 1–11. doi: 10.1038/s41467-020-17819-9
Müller, M., and Hasanuzzaman, M. (2021). Foes or friends: ABA and ethylene interaction under abiotic stress. Plants 10, 448. doi: 10.3390/PLANTS10030448
Müller, R., Stummann, B. M., and Serek, M. (2000). Characterization of an ethylene receptor family with differential expression in rose (Rosa hybrida L.) flowers. Plant Cell Rep. 1912, 1232–1239. doi: 10.1007/S002990000251
Oliveira, R., Viana, A., Reátegui, A., and Vincentz, M. (2015). An efficient method for simultaneous extraction of high-quality RNA and DNA from various plant tissues. Genet. Mol. Res. 14, 18828–18838. doi: 10.4238/2015.December.28.32
Pfaffl, M. W. (2001). A new mathematical model for relative quantification in real-time RT-PCR. Nucleic Acids Res. 29, 45e–45e. doi: 10.1093/nar/29.9.e45
Polko, J. K., and Kieber, J. J. (2019). 1-aminocyclopropane 1-carboxylic acid and its emerging role as an ethylene-independent growth regulator. Front. Plant Sci. 10:1602. doi: 10.3389/FPLS.2019.01602/BIBTEX
R Core Team (2019). R: A Language and Environment for Statistical Computing. Vienna: R Foundation for Statistical Computing.
Ramírez, B. V. H., Arcila, J. P., Jaramillo, ÁR., Rendón-S, J. R., Cuesta, G. G., Menza, F. H. D., et al. (2010). Floración del café en colombia y su relación con la disponibilidad hídrica, térmica y de brillo solar. Cenicafé 61, 132–158.
Riboni, M., Galbiati, M., Tonelli, C., and Conti, L. (2013). GIGANTEA enables drought escape response via abscisic acid-dependent activation of the florigens and SUPPRESSOR OF OVEREXPRESSION OF CONSTANS. Plant Physiol. 162, 1706–1719. doi: 10.1104/PP.113.217729
Romera-Branchat, M., Andrés, F., and Coupland, G. (2014). Flowering responses to seasonal cues: what’s new? Curr. Opin. Plant Biol. 21, 120–127. doi: 10.1016/J.PBI.2014.07.006
Ronchi, C. P., and Miranda, F. R. (2020). Flowering percentage in arabica coffee crops depends on the water deficit level applied during the pre-flowering stage. Rev. Caatinga 33, 195–204. doi: 10.1590/1983-21252020v33n121rc
Saitou, N., and Nei, M. (1987). The neighbor-joining method: a new method for reconstructing phylogenetic trees. Mol. Biol. Evol. 4, 406–425. doi: 10.1093/oxfordjournals.molbev.a040454
Schuch, U. K., Fuchigami, L. H., and Nagao, M. A. (1992). Flowering, ethylene production, and ion leakage of coffee in response to water stress and gibberellic acid. J. Am. Soc. Hortic. Sci. 117, 158–163. doi: 10.21273/JASHS.117.1.158
Shavrukov, Y., Kurishbayev, A., Jatayev, S., Shvidchenko, V., Zotova, L., Koekemoer, F., et al. (2017). Early flowering as a drought escape mechanism in plants: how can it aid wheat production? Front. Plant Sci. 8:1950. doi: 10.3389/FPLS.2017.01950/BIBTEX
Sherrard, M. E., and Maherali, H. (2006). The adaptive significance of drought escape in avena barbata, an annual grass. Evolution (N. Y.) 60, 2478–2489. doi: 10.1111/J.0014-3820.2006.TB01883.X
Shin, K., Lee, S., Song, W. Y., Lee, R. A., Lee, I., Ha, K., et al. (2015). Genetic identification of ACC-RESISTANT2 reveals involvement of LYSINE HISTIDINE TRANSPORTER1 in the uptake of 1-aminocyclopropane-1-carboxylic acid in Arabidopsis thaliana. Plant Cell Physiol. 56, 572–582. doi: 10.1093/PCP/PCU201
Silva, V. A., Prado, F. M., Antunes, W. C., Paiva, R. M. C., Ferrão, M. A. G., Andrade, A. C., et al. (2018). Reciprocal grafting between clones with contrasting drought tolerance suggests a key role of abscisic acid in coffee acclimation to drought stress. Plant Growth Regul. 85, 221–229. doi: 10.1007/s10725-018-0385-5
Song, Y. H., Shim, J. S., Kinmonth-Schultz, H. A., and Imaizumi, T. (2015). Photoperiodic flowering: time measurement mechanisms in leaves. Annu. Rev. Plant Biol. 66, 441–464. doi: 10.1146/annurev-arplant-043014-115555
Southwick, S. M., and Davenport, T. L. (1986). Characterization of water stress and low temperature effects on flower induction in citrus. Plant Physiol. 81, 26–29. doi: 10.1104/PP.81.1.26
Steibel, J. P., Poletto, R., Coussens, P. M., and Rosa, G. J. M. (2009). A powerful and flexible linear mixed model framework for the analysis of relative quantification RT-PCR data. Genomics 94, 146–152. doi: 10.1016/j.ygeno.2009.04.008
Stern, R. A., Adato, I., Goren, M., Eisenstein, D., and Gazit, S. (1993). Effects of autumnal water stress on litchi flowering and yield in Israel. Sci. Hortic. (Amsterdam) 54, 295–302. doi: 10.1016/0304-4238(93)90108-3
Takeno, K. (2016). Stress-induced flowering: the third category of flowering response. J. Exp. Bot. 67, 4925–4934. doi: 10.1093/jxb/erw272
Tamura, K., Stecher, G., Peterson, D., Filipski, A., and Kumar, S. (2013). MEGA6: molecular evolutionary genetics analysis version 6.0. Mol. Biol. Evol. 30, 2725–2729. doi: 10.1093/molbev/mst197
Thompson, J. D., Higgins, D. G., and Gibson, T. J. (1994). CLUSTAL W: improving the sensitivity of progressive multiple sequence alignment through sequence weighting, position-specific gap penalties and weight matrix choice. Nucleic Acids Res. 22, 4673–4680. doi: 10.1093/nar/22.22.4673
Trivellini, A., Ferrante, A., Vernieri, P., and Serra, G. (2011). Effects of abscisic acid on ethylene biosynthesis and perception in Hibiscus rosa sinensis L. flower development. J. Exp. Bot. 62, 5437–5452. doi: 10.1093/jxb/err218
Trusov, Y., and Botella, J. R. (2006). Silencing of the ACC synthase gene ACACS2 causes delayed flowering in pineapple [Ananas comosus (L.) Merr.]. J. Exp. Bot. 57, 3953–3960. doi: 10.1093/jxb/erl167
Tsang, D. L., Edmond, C., Harrington, J. L., and Nühse, T. S. (2011). Cell wall integrity controls root elongation via a general 1-aminocyclopropane-1-carboxylic acid-dependent, ethylene-independent pathway. Plant Physiol. 156, 596–604. doi: 10.1104/PP.111.175372
Tsuchisaka, A., Yu, G., Jin, H., Alonso, J. M., Ecker, J. R., Zhang, X., et al. (2009). A combinatorial interplay among the 1-aminocyclopropane-1-carboxylate isoforms regulates ethylene biosynthesis in Arabidopsis thaliana. Genetics 183, 979–1003. doi: 10.1534/genetics.109.107102
Uji, T., Endo, H., and Mizuta, H. (2020). Sexual reproduction via a 1-aminocyclopropane-1-carboxylic acid-dependent pathway through redox modulation in the marine red alga Pyropia yezoensis (Rhodophyta). Front. Plant Sci. 11:60. doi: 10.3389/FPLS.2020.00060/BIBTEX
Van de Poel, B., Vandenzavel, N., Smet, C., Nicolay, T., Bulens, I., Mellidou, I., et al. (2014). Tissue specific analysis reveals a differential organization and regulation of both ethylene biosynthesis and E8 during climacteric ripening of tomato. BMC Plant Biol. 14:11. doi: 10.1186/1471-2229-14-11
Vanderstraeten, L., Depaepe, T., Bertrand, S., and Van Der Straeten, D. (2019). The ethylene precursor ACC affects early vegetative development independently of ethylene signaling. Front. Plant Sci. 10:1591. doi: 10.3389/fpls.2019.01591
Vanderstraeten, L., and van Der Straeten, D. (2017). Accumulation and transport of 1-aminocyclopropane-1-carboxylic acid (ACC) in plants: current status, considerations for future research and agronomic applications. Front. Plant Sci. 8:38. doi: 10.3389/fpls.2017.00038
Veddeler, D., Klein, A. M., Tscharntke Veddeler, T., Veddeler, D., Klein, A., and Tscharntke, T. (2006). Contrasting responses of bee communities to coffee flowering at different spatial scales. Oikos 112, 594–601. doi: 10.1111/J.0030-1299.2006.14111.X
Verslues, P. E., and Juenger, T. E. (2011). Drought, metabolites, and Arabidopsis natural variation: a promising combination for understanding adaptation to water-limited environments. Curr. Opin. Plant Biol. 14, 240–245. doi: 10.1016/J.PBI.2011.04.006
Vu, T., Park, M., Kim, S., Tran, T., and Jang, D. C. (2020). Effect of abscisic acid on growth and physiology of arabica coffee seedlings under water deficit condition. Sains Malays. 49, 1499–1508. doi: 10.17576/jsm-2020-4907-03
Wang, Q., Zhang, W., Yin, Z., and Wen, C. K. (2013). Rice CONSTITUTIVE TRIPLE-RESPONSE2 is involved in the ethylene-receptor signalling and regulation of various aspects of rice growth and development. J. Exp. Bot. 64, 4863–4875. doi: 10.1093/JXB/ERT272
Wang, Y., Li, L., Ye, T., Lu, Y., Chen, X., and Wu, Y. (2013). The inhibitory effect of ABA on floral transition is mediated by ABI5 in Arabidopsis. J. Exp. Bot. 64, 675–684. doi: 10.1093/JXB/ERS361
Wilmowicz, E., Frankowski, K., Kuæko, A., Świdziński, M., de Dios Alché, J., Nowakowska, A., et al. (2016). The influence of abscisic acid on the ethylene biosynthesis pathway in the functioning of the flower abscission zone in Lupinus luteus. J. Plant Physiol. 206, 49–58. doi: 10.1016/J.JPLPH.2016.08.018
Wu, P., Wu, C., and Zhou, B. (2017). Drought stress induces flowering and enhances carbohydrate accumulation in Averrhoa carambola. Hortic. Plant J. 3, 60–66. doi: 10.1016/J.HPJ.2017.07.008
Xu, S. L., Rahman, A., Baskin, T. I., and Kieber, J. J. (2008). Two leucine-rich repeat receptor kinases mediate signaling, linking cell wall biosynthesis and ACC synthase in Arabidopsis. Plant Cell 20, 3065–3079. doi: 10.1105/TPC.108.063354
Yang, G., Wei, Q., Huang, H., and Xia, J. (2020). Amino acid transporters in plant cells: a brief review. Plants 9:967. doi: 10.3390/PLANTS9080967
Yin, J., Zhang, X., Zhang, G., Wen, Y., Liang, G., and Chen, X. (2019). Aminocyclopropane-1-carboxylic acid is a key regulator of guard mother cell terminal division in Arabidopsis thaliana. J. Exp. Bot. 70, 897–908. doi: 10.1093/JXB/ERY413
Zhang, M., Yuan, B., and Leng, P. (2009). The role of ABA in triggering ethylene biosynthesis and ripening of tomato fruit. J. Exp. Bot. 60, 1579. doi: 10.1093/JXB/ERP026
Keywords: flowering, LHT1 transporter, drought, ACO, hormonal signaling
Citation: López ME, Silva Santos I, Marquez Gutiérrez R, Jaramillo Mesa A, Cardon CH, Espíndola Lima JM, Almeida Lima A and Chalfun-Junior A (2022) Crosstalk Between Ethylene and Abscisic Acid During Changes in Soil Water Content Reveals a New Role for 1-Aminocyclopropane-1- Carboxylate in Coffee Anthesis Regulation. Front. Plant Sci. 13:824948. doi: 10.3389/fpls.2022.824948
Received: 29 November 2021; Accepted: 03 March 2022;
Published: 06 April 2022.
Edited by:
Vicente Vives-Peris, Jaume I University, SpainReviewed by:
Juan Pablo Martinez, Instituto de Investigaciones Agropecuarias, ChileAnis Ali Shah, University of Education Lahore, Pakistan
Liyou Qiu, Henan Agricultural University, China
Copyright © 2022 López, Silva Santos, Marquez Gutiérrez, Jaramillo Mesa, Cardon, Espíndola Lima, Almeida Lima and Chalfun-Junior. This is an open-access article distributed under the terms of the Creative Commons Attribution License (CC BY). The use, distribution or reproduction in other forums is permitted, provided the original author(s) and the copyright owner(s) are credited and that the original publication in this journal is cited, in accordance with accepted academic practice. No use, distribution or reproduction is permitted which does not comply with these terms.
*Correspondence: Antonio Chalfun-Junior, Y2hhbGZ1bmp1bmlvckB1ZmxhLmJy