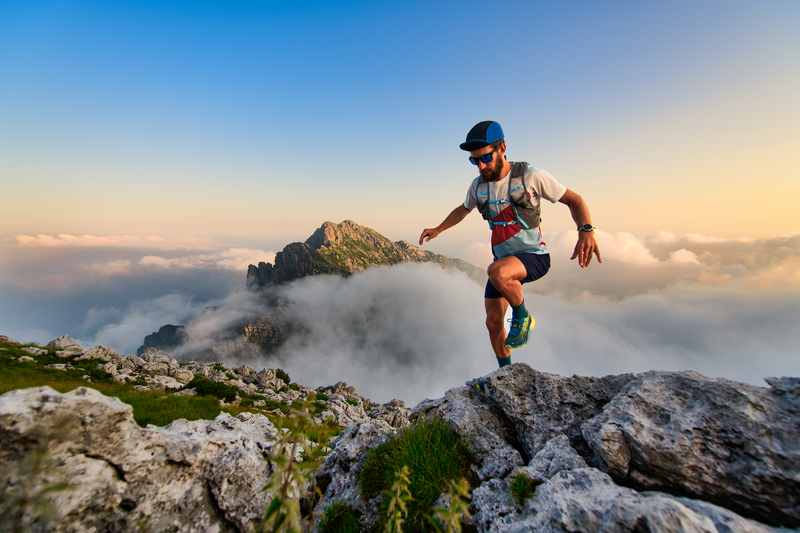
95% of researchers rate our articles as excellent or good
Learn more about the work of our research integrity team to safeguard the quality of each article we publish.
Find out more
ORIGINAL RESEARCH article
Front. Plant Sci. , 25 March 2022
Sec. Plant Abiotic Stress
Volume 13 - 2022 | https://doi.org/10.3389/fpls.2022.868807
This article is part of the Research Topic Metabolomics: A Tool to Understand Plant Protection Against Biotic and Abiotic Stresses View all 6 articles
Enhanced herbicide metabolism mediated by cytochrome P450s has been proposed as one of the major mechanisms of resistance to fenoxaprop-P-ethyl in a metabolic-herbicide-resistant biotype of Asia minor bluegrass (Polypogon fugax Nees ex Steud.). Upon pre-treatment with the P450 inhibitor piperonyl butoxide, a remarkable reduction in metabolic rates of the phytotoxic fenoxaprop-P has been observed in the resistant plants, implying that constitutive and/or fenoxaprop-P-ethyl-induced up-regulation of specific P450 isoforms are involved in the fenoxaprop-P-ethyl resistance. However, which P450 gene(s) were responsible for the metabolic resistance is still unknown. In this present study, based on the abundant gene resources of P. fugax established previously, a total of 48 putative P450 genes were isolated from the metabolic-herbicide-resistant plants and used for gene expression analysis. The most suitable reference genes for accurate normalization of real-time quantitative PCR data were first identified in P. fugax and recognized as actin (ACT), 18S rRNA (18S), and ribulose-1,5-bisphosphate carboxylase oxygenase (RUBP) under fenoxaprop-P-ethyl stress, glyceraldehyde-3-phosphate dehydrogenase (GAPDH) and elongation factor 1α (EF1α) under mesosulfuron-methyl stress, and ACT, EF1α, eukaryotic initiation factor 4a (EIF4A), and 25S rRNA (25S) at different growth stages. Expression analysis of the putative P450 genes revealed that six genes, respectively, annotated as CYP709B1, CYP71A1-4, CYP711A1, CYP78A9, P450-11, and P450-39 were up-regulated more than 10-fold in the resistant plants by fenoxaprop-P-ethyl treatment, and all of them exhibited constitutively and/or herbicide-induced higher transcript levels in the fenoxaprop-P-ethyl-resistant than in the susceptible plants. Three genes, respectively, annotated as CYPRO4, CYP313A4, and CYP51H11 constantly up-regulated in the resistant than in the susceptible plants after fenoxaprop-P-ethyl treatment. Up-regulated expressions of these specific P450 genes were consistent with the higher P450 contents determined in the resistant plants. These results will help to elucidate the mechanisms for P450-mediated metabolic-herbicide resistance in P. fugax as well as other grass weed species.
Asia minor bluegrass (Polypogon fugax Nees ex Steud.) is an annual or biennial hexaploid grass species widely spreading in Asia, Eurasia, and North America (Wang et al., 2016; Akopian and Ghukasyan, 2018). In China, P. fugax severely infests winter wheat (Triticum aestivum L.) fields with a rice (Oryza sativa L.) or maize (Zea mays L.) rotation. It competes with crop seedlings for natural resources like light, water, and fertilizer, reducing grain yields by up to 40% (Tang et al., 2015). Since the 1990s, the acetyl-CoA carboxylase (ACCase, EC. 6.4.1.2)-inhibiting herbicide fenoxaprop-P-ethyl has been widely adopted for control of this noxious weed. However, repeated use has facilitated the development of herbicide resistance in P. fugax. Tang et al. (2014) reported the first case of P. fugax resistance to fenoxaprop-P-ethyl from Sichuan Province, China, and more herbicide resistance of this weed have been in recent years (Zhao et al., 2019; Chen et al., 2020; Yu et al., 2021).
Weed resistance to herbicides is mainly categorized into target-site based resistance (TSR) and non-TSR (NTSR; Gaines et al., 2020). In the last decades, most of the herbicide-resistance cases reported have occurred via the former mechanism. TSR is certainly the most studied aspect to date and generally caused by structural changes to the herbicide-binding sites or overproduction of target proteins (Gaines et al., 2019). In contrast, NTSR contains many other mechanisms that reduce the amount of active herbicide reaching the target sites and is a more complex trait. Of these, enhanced herbicide metabolism is a frequently reported NTSR mechanism which under complex genetic controls. There is evidence that enhanced metabolism often involves multiple genes from different herbicide detoxifying-enzyme families, such as cytochrome P450 monooxygenases (P450s), glutathione S-transferases, UDP-glycosyltransferases, and transporter proteins (Délye et al., 2013). Most of the weed plants carrying metabolism-based resistance exhibit multiple-herbicide resistance and pose a great threat to modern agricultural production.
Of the herbicide detoxifying-enzyme families, P450s are ubiquitous hemethiolate monooxygenases that present in the genomes of virtually all organisms (Werck-Reichhart and Feyereisen, 2000). They can catalyze a wide variety of reactions involves monooxygenation and hydroxylation. In higher plants, hundreds of P450 genes exist in their genomes. Generally, the rates of herbicide metabolism are different among plant species, and the selectivity of many herbicides is based on the difference in rates of herbicide metabolism between crop and weed plants (Owen, 1991). In recent years, more and more weed plants exhibit high herbicide-detoxifying P450 activity, suggesting the development of P450-mediated herbicide resistance (Jugulam and Shyam, 2019). Omics-based tools have thus been widely adopted to identify candidate genes related to herbicide detoxification (Giacomini et al., 2017), and some P450 genes from crop and weed species have been demonstrated to metabolize numerous herbicides from various chemical backbones (Iwakami et al., 2019; Kato et al., 2020; Han et al., 2021). Nevertheless, herbicide-detoxifying P450s remain to be identified in most weed species, as many factors such as herbicide application history and genetic background may impact the evolution of P450-mediated herbicide resistance.
Plant P450 gene sequences are rich in diversity and lack of conserved regions. Polymerase chain reaction (PCR) using PERF/W and PFG motifs is a conventional method for isolating putative plant P450 genes, but the low efficiency and the few conserved P450 motifs have limited its application (Iwakami et al., 2014). Transcriptomic is a robust tool to identify unknown genes related to a quantitative trait in specific species without a complete genome. Especially, third-generation sequencing by PacBio platform can provide long-reads up to 10 kb, making it possible to accurately reconstruct full-length splice variants. Therefore, the full-length reference transcriptome assembled by third-generation sequencing will present a whole landscape of genes annotated as related to P450s in a given species. This approach can improve the efficiency of gene amplification and isolate more diverse P450 genes, which will help to investigate the herbicide-detoxifying P450s in arable weed species.
Real-time quantitative PCR (RT-qPCR) is a powerful tool for measuring gene expression and will be essential for deciphering molecular mechanisms of herbicide resistance. More and more studies have suggested that the expression of reference genes may vary among species and under various conditions (Xu et al., 2017). Therefore, it is necessary to systematically identify stably expressed genes as internal controls before conducting any meaningful RT-qPCR analysis. So far, suitable reference genes have been identified in many important crop species (Jarošová and Kundu, 2010; Kong et al., 2016; Kiarash et al., 2018), and lack of detailed genomic information has limited the assessment of reference genes in weed species. Identification of stably expressed gene(s) in these plants under different conditions will provide useful guidelines for accurate gene expression studies.
Previously, we have identified a fenoxaprop-P-ethyl-resistant P. fugax population (AHHY) and preliminarily characterized its TSR and NTSR mechanisms (Zhao et al., 2019). Trp-1999-Ser mutation of ACCase and P450s-mediated enhanced metabolism were simultaneously involved in the resistance phenotypes (Zhao et al., 2019, 2022b). However, whether ACCase overexpression played a role in the resistance or not and which P450 gene(s) were responsible for the metabolic resistance are still unknown. Although previous RNA sequencing has suggested several genes may be involved in the resistance (Zhao et al., 2022b), some key genes may be ignored due to sampling simplicity and higher false positive. To address these issues, in the current study, seven candidate reference genes were first isolated from P. fugax, and their expression stability was systematically assessed in P. fugax plants under herbicide stress as well as other experimental conditions. ACCase expression was then determined in different biotypes of P. fugax to rule out the gene overexpression as a resistance mechanism. Thereafter, the entire P450 genes were isolated from the full-length transcriptome assembled previously, and their temporal expression patterns were detailedly characterized in P. fugax under fenoxaprop-P-ethyl stress by RT-qPCR. The comparison of P450 genes between susceptible and fenoxaprop-P-ethyl-resistant plants will help to uncover the mechanism for P450-mediated herbicide resistance in P. fugax.
Two populations of P. fugax—a susceptible population (S, SDTS) and a resistant population (R, AHHY)—were used in this study. Seed collection and herbicide resistance testing for each population have been described in Zhao et al. (2019). Of these, the SDTS was susceptible to the ACCase inhibitor fenoxaprop-P-ethyl, while the AHHY had evolved both TSR and NTSR to it. Besides, both populations were susceptible to mesosulfuron-methyl, isoproturon, and cypyrafluone.
Seeds were randomly selected from each population and pre-germinated in Petri dishes containing 5 ml of distilled water. After their germination, 12 seeds were sown into 20-cm-diameter plastic pots filled with loam soil and grown in a controlled greenhouse (25/15°C day/night, 12-h photoperiod, natural light, with ∼75% relative humidity). All the pots were watered and re-arranged every other day.
Polypogon fugax seedlings at the three- to four-leaves stage were treated with fenoxaprop-P-ethyl (69 g L−1 emulsion in water, Bayer, Hangzhou, China) at 62.1 g ai ha−1 or mesosulfuron-methyl (30 g L−1 oil-miscible flowable, Bayer) at 9.0 g ai ha−1. Herbicides were sprayed by using a laboratory cabinet sprayer equipped with a Teejet 9503EVS flatfan nozzle (Zhao et al., 2018). A time-course experiment was performed to determine the stably expressed genes in susceptible and resistant plants with or without herbicide treatment. Fresh leaves from each biotype were separately harvested at 0 (untreated), 2, 6, 24, 48, 72, and 96 h after treatment (HAT), after which they were immediately frozen in liquid nitrogen and stored at −80°C until RNA extraction. A total of 56 samples (two populations × two treatments × seven different sampling time points × two biological replicates) were collected in this study. They were divided into two sample subsets based on the herbicide received (i.e., a fenoxaprop-P-ethyl subset and a mesosulfuron-methyl subset).
Fresh leaf tissues were separately harvested from the S and R biotypes of P. fugax at the three- to four-leaf stage, tillering stage, jointing stage, and heading stage. All samples were immediately frozen in liquid nitrogen and stored at −80°C. A total of 16 samples (two populations × four growth stages × two biological replicates) were collected and grouped into a growth-stage subset.
Fresh roots, stems, leaves, and spikes were separately harvested from the S and R biotypes of P. fugax prior to ripening at the fruit-bearing stage. All samples were immediately frozen in liquid nitrogen and stored at −80°C. A total of 16 samples (two populations × four organs × two biological replicates) were collected and grouped into an organ subset.
Total RNA was isolated from each sample using the TRIzol Reagent (Invitrogen, Carlsbad, CA, United States) according to the manufacturer’s procedures. The quality and integrity of the RNA samples were assessed by using a NanoDrop 2000 spectrophotometer (Thermo Fisher Scientific, Middlesex, MA, United States) and by 1% (w/v) agarose gel electrophoretic analysis, respectively. All the RNA samples used in the following experiments had passed the quality controls. The trace of contaminating genomic DNA (gDNA) was eliminated and the first-strand cDNA was synthesized from approximately 1 μg of total RNA by using the EasyScript® All-in-One First-Strand cDNA Synthesis SuperMix for qPCR (One-Step gDNA Removal; TransGen Biotech, Beijing, China) according to the manufacturer’s procedures. The obtained cDNAs were stored at −20°C until further use.
A total of seven candidate genes were selected for the analysis in this study, including actin (ACT), eukaryotic initiation factor 4a (EIF4A), elongation factor 1α (EF1α), 18S rRNA (18S), 25S rRNA (25S), ribulose-1,5-bisphosphate carboxylase oxygenase (RUBP), and glyceraldehyde-3-phosphate dehydrogenase (GAPDH; Table 1). These genes are frequently used as internal controls in studies investigating plant responses to various abiotic stresses (Nicot et al., 2005; Dombrowski and Martin, 2009; Duhoux and Délye, 2013; Wrzesińska et al., 2016; Kiarash et al., 2018). Sequence for each gene was obtained by querying the full-length transcriptome of P. fugax assembled recently (Accession number: MZ221767–MZ221773). Primers were designed using Primer Premier v.5.0 software (PREMIER Biosoft International, Palo Alto, CA, United States) and were synthesized by TsingKe Biotech Co., Ltd. (Beijing, China).
Reverse transcription (RT)-PCR was performed to amplify specific fragment of each candidate gene from cDNA using 2 × Taq MasterMix (Vazyme, Nanjing, China). PCR product sizes were checked on 1% (w/v) agarose gels, and the expected amplicons were sequenced to confirm the amplification of target genes (Supplementary Table S1).
RT-qPCR was performed in 0.2 ml 8-Tube qPCR Strips (PCR-0108-LP-RT-C, Union City, CA, United States) using CFX96 Touch Real-Time PCR Detection System (Bio-Rad, Hercules, CA, United States). PCR reactions were prepared by using 2 × TransStart Top Green qPCR SuperMix (TransGen Biotech) as described elsewhere (Zhao et al., 2018). Two-step RT-qPCR was adopted for primers whose melting temperature (Tm) was ≥ 60°C, and three-step RT-qPCR was adopted for the other primers. Each PCR reaction was performed in technical triplicates with non-template controls (NTCs) and no reverse transcriptase (no-RT) controls.
PCR product specificity of each primer pair was verified through melting curve analyses, and the amplification efficiency (E) and correlation coefficients (r2) of each primer pair were calculated using the standard curve method (Zhao et al., 2018).
Three statistical algorithms, including geNorm (Vandesompele et al., 2002), NormFinder (Andersen et al., 2004), and the comparative ΔCq method (Silver et al., 2006) were used to systematically assess the expression stability of each candidate gene. A web-tool RefFinder1 was finally used to compare the results of different statistical programs to generate a comprehensive ranking of stability for the candidate genes (Xie et al., 2012). In the comparative ΔCq method and RefFinder, the raw Cq values calculated by CFX maestro v.4.1 software (Bio-Rad) were directly used. In geNorm and NormFinder, the raw Cq values were transformed to relative quantities by the 2−ΔCq method, with the lowest Cq value serving as a calibrator.
Based on the stably expressed reference genes identified, ACCase gene expression was compared on extracts from three S (SDTS) and three R (AHHY) plants with each sample subjected to a RT-qPCR analysis in triplicate. The primer pair ACCase-F1 (5′-CACTGAAGGGAAGCGATTGGGT-3′) and ACCase-R1 (5′-CTTCAGATGCATTTGTTTGAG-3′) with a Tm of 60°C was designed to amplify a 175-bp fragment of ACCase. Because the different alleles of ACCase have a very high homology (Zhao et al., 2019), the ACCase expression determined here represents the total expression of all ACCase alleles. The oligonucleotide was designed to overlap to intron junction by analysis of the assembled transcriptome to the corresponding genomic region of maize (GenBank No. XM_008664833) using EMBOSS EST2Genome (Rice et al., 2000). RT-qPCR was performed using the two-step method as described above, and the results were analyzed by the 2−ΔΔCq method using CFX Maestro v.4.1 software (Bio-Rad). When comparing the expression levels of a target gene between two groups of samples, the software considers the respective amplification efficiencies of target gene and reference gene(s).
P450 contents for the S and R biotypes were determined through the method described by Wang et al. (2013) with minor modifications. Seeds of the S and R biotypes were pre-germinated and sown in square plastic pots (10 cm × 10 cm × 8.5 cm) filled with quartz sand. After planting, each pot was put in a plastic bowl containing half-strength Hoagland’s nutrient solution (Coolaber, Beijing, China), wrapped with aluminum foil to exclude light, and grown in a climatic chamber at 25/15°C without light. The nutrient solution was changed every 3 days. At 18 days after planting, the seedlings were sprayed with fenoxaprop-P-ethyl at 31.1 g ai ha−1 as described above. The etiolated shoots of the weed seedlings were then collected at 0, 1, 2, 3, 5, and 7 days after treatment and used for microsome isolation. P450 content in each sample was quantified using reduced carbon monoxide difference spectroscopy and expressed as nmol mg−1 microsomal protein (Estabrook and Werringloer, 1978; Jefcoate, 1978). The experiment was conducted twice with each treatment had three replicates.
In our previous study, a reference transcriptome containing 24,972 full-length isoforms with a N50 of 2,575 bp was established for P. fugax through third-generation PacBio sequencing (Zhao et al., 2022b). Gene functional annotation by BLAST against the NCBI non-redundant protein (Nr) database revealed that a total of 169 unigenes were annotated as related to putative P450s (Supplementary Table S2). However, P. fugax is a hexaploid (2n = 6x = 42) grass weed, and multiple alleles encoding a specific enzyme were expected to be present in this polyploid species (Akopian and Ghukasyan, 2018; Zhao et al., 2019). Therefore, in the current study, the contigs having a high sequence similarity (≥80%) and sharing a same functional annotation were considered as the different alleles encoding a same P450 enzyme (Supplementary Table S2). Based on the selection standard, a total of 48 putative P450 genes were successfully identified and isolated from the reference transcriptome (Supplementary Table S3).
Plants of the S and R lines were grown together in a growth chamber at 25/15°C day/night with a 12-h photoperiod (200 μmol m−2 s−1 photosynthetic photon flux density, with ~75% relative humidity). Weed seedlings at the three- to four-leaves stage were treated with fenoxaprop-P-ethyl at the field-recommended rate (62.1 g ai ha−1). Fresh shoots of the S and R plants were sampled at the same time at 0 (untreated), 6, 12, and 24 HAT, frozen in liquid N2, and stored at −80°C. RNA was extracted from each sample using the TRIzol Reagent (Invitrogen) and assessed as described above.
Primers were designed using Primer Premier v.5.0 software (PREMIER Biosoft International) and summarized in Supplementary Table S3. For the genes encoded by multiple alleles, primers were designed based on the conserved regions of different alleles. RT-qPCR was performed on the CFX96 Touch Real-Time PCR Detection System (Bio-Rad). cDNA was prepared as described above from 0, 6, 12, and 24-h RNAs extracted from the S and R plants. The expression of each putative P450 gene was normalized relative to the mean of ACT, 18S, and RUBP, and the results were analyzed by the 2−ΔΔCq method. Two threshold values including the fold change (twofold) and t-test (p < 0.05) were used to determine the up- or down-regulation of a target gene by fenoxaprop-P-ethyl treatment.
A total of seven candidate genes were selected and assessed in this study (Table 1). Every gene showed a single DNA band in agarose gel electrophoresis (Supplementary Figure S1) and a single-peak melting curve in RT-qPCR analysis (Supplementary Figure S2), confirming the absence of primer dimers and non-specific amplicons. Gene sequencing and alignment suggested the specific amplification of the targeted amplicons and the absence of gDNA contaminations (Supplementary Table S1). The NTCs and no-RT controls detected no amplicon peak or RT-qPCR signal, indicating the absence of cross-contamination. The RT-qPCR efficiencies (E) obtained for each primer pair ranged from 85 to 105%, and the corresponding correlation coefficients (r2) comprised between 0.95 and 1.00 (Table 1; Supplementary Figure S3). This indicates the developed RT-qPCR system was reliable and comparable.
The Cq values of each gene generated in RT-qPCR were used to create box-and-whisker plots to present an overview of the transcript levels under different experimental conditions (Figure 1). In this study, different genes showed distinct expression levels, with mean Cq values ranging from 12.6 to 26.4 (Table 1). Each specific gene exhibited a similar expression pattern in P. fugax plants under different experimental conditions (Figure 1). Based on the median and mean Cq values, 18S and EIF4A were considered to express to higher and lower levels than the other six genes, respectively.
Figure 1. Overview of transcript levels of seven candidate reference genes in Polypogon fugax under herbicide stress as well as other experimental conditions. Top and bottom borders represent the 25th and 75th percentiles, respectively. The horizontal line within the box indicates the median. Whiskers represent the maximum and minimum values.
Polypogon fugax samples were classed into different data (sub)sets according to their corresponding experimental conditions: an all-samples data set, a fenoxaprop-P-ethyl subset, a mesosulfuron-methyl subset, a growth-stage subset, and an organ subset. The expression stability of the seven candidate genes was ranked in each sample grouping.
In the fenoxaprop-P-ethyl subset, geNorm indicated 18S, 25S, RUBP, ACT, and EF1α as the stable genes (Supplementary Figure S4) and suggested three genes would beadequate for accurate data normalization (Supplementary Figure S5). NormFinder identified ACT as the most stable gene and suggested EF1α and 18S should be conjointly used for accurate data normalization (Supplementary Table S4). The comparative ΔCq method ranked ACT, 18S, EF1α, and RUBP as the four most stable genes (Supplementary Table S5). Considering the comprehensive ranking of stability presented by RefFinder (Table 2), we recommended ACT, 18S, and RUBP as suitable reference genes in P. fugax leaves treated with fenoxaprop-P-ethyl.
Table 2. Ranking of seven candidate reference genes according to their expression stability using RefFinder.
In the mesosulfuron-methyl subset, different algorithms produced distinct results (Supplementary Figure S4, Supplementary Tables S4, S5). geNorm indicated that two genes would be adequate for accurate data normalization (Supplementary Figure S5). Based on the comprehensive ranking of stability calculated by RefFinder (Table 2), we considered the GAPDH and EF1α as suitable internal controls.
Similarly, ACT, EF1α, EIF4A, and 25S were recommended as a suitable gene combination for data normalization at different growth stages of P. fugax (Table 2). However, no specific gene or gene combination was suitable for accurate data normalization in the different organs of P. fugax, nor in the all-samples data set.
To investigate if target gene overexpression participated in the resistance phenotypes, the transcript abundance of ACCase was compared in the S and R biotypes of P. fugax. The expression of ACCase was normalized relative to the mean of ACT, 18S, and RUBP. PCR efficiency (E) for ACCase was 98.6% with a r2 of 0.969, and the mean Cq value was 25.17. Based on the data obtained, ACCase expression showed no significant difference between plants with contrasting phenotypes with or without herbicide treatment (Figure 2). This indicates that ACCase overexpression did not occur in the R biotype of P. fugax.
Figure 2. Relative expression levels of ACCase in the R relative to the S plants of Polypogon fugax at 0 (control), 24, 48, 72, and 96 h after fenoxaprop-P-ethyl treatment. Data are the mean values ± SEM.
Before herbicide treatment, P450 content in the R plants was approximately 0.76 nmol mg−1 protein, which was significantly higher (p < 0.05) than that in the S plants (Figure 3). After fenoxaprop-P-ethyl treatment, the P450 content increased markedly by 2.39-fold in the R plants, while no serious change in the S plants (Figure 3). Notably, the P450 content in the R plants was always higher (p < 0.05) than that in the S plants during 1–7 days after fenoxaprop-P-ethyl treatment (Figure 3). This suggested that P450s could be induced by fenoxaprop-P-ethyl treatment and were expected to be involved in the metabolism-based resistance phenotypes.
Figure 3. Changes in cytochrome P450 contents in the S and R biotypes of Polypogon fugax after fenoxaprop-P-ethyl treatment. The difference in P450 contents was presented as ratio of the R relative to the S biotype. Data are the mean values ± SEM. *p < 0.05.
The expression patterns of the 48 putative P450 genes were first analyzed in the susceptible and fenoxaprop-P-ethyl-resistant biotypes at different times after herbicide treatment. Generally, these P450 genes performed differently in their relative expression levels during 0 to 24 HAT (Figure 4). Of these, 22 genes were up-regulated more than threefold for at least one time point after fenoxaprop-P-ethyl treatment in the R line (black and blue dots marked genes in Figure 4), 40 genes were up-regulated more than threefold in the S line (black and orange dots marked genes in Figure 4), and 20 genes were up-regulated more than threefold in both the S and R lines (black dots marked genes in Figure 4). The expression levels of the herbicide-treatment-inducible genes also differed substantially. In the R line, six genes, respectively, annotated as CYP709B1, CYP71A1-4, CYP711A1, CYP78A9, P450-11, and P450-39 were up-regulated more than 10-fold, and the strongest up-regulation was observed for the gene annotated as CYP709B1 which had a more than 10,000-fold increase in expression at 6 h. In comparison, 18 genes were up-regulated more than 10-fold in the S line, and the strongest up-regulation was observed for the gene annotated as P450-39 (more than 600-fold).
Figure 4. Temporal dynamic changes in relative transcript levels of each putative P450 gene in the S (●) and R (○) lines of Polypogon fugax under fenoxaprop-P-ethyl stress. The X-axis represents the time (h) after fenoxaprop-P-ethyl treatment, and the Y-axis represents the transcript levels relative to the untreated sample (0 h). Data are the mean values ± SEM. Black dots marked genes were up-regulated more than threefold in both the S and R lines by fenoxaprop-P-ethyl treatment for at least one time point, orange dots marked genes were up-regulated more than threefold only in the S line, and blue dots marked genes were up-regulated more than threefold only in the R line.
The transcript levels of the 48 putative P450 genes were compared between the susceptible and fenoxaprop-P-ethyl-resistant biotypes at 0 (control), 12, 24, and 48 h after herbicide treatment. Under non-herbicide-stressed conditions, eight genes were constitutively up-regulated more than threefold in the R relative to the S line (black and blue dots marked genes in Figure 5). These include one CYP57 gene, two CYP71 genes, one CYP92 gene, one CYP94 gene, one CYP97 gene, and two other genes (P450-13 and P450-39) without specific annotations. All the above genes showed moderate transcription increases ranging from threefold to fivefold. Under fenoxaprop-P-ethyl stress, 24 genes were up-regulated more than threefold in the R relative to the S line for at least one time point (black and orange dots marked genes in Figure 5). Of these, three genes had constantly higher transcript abundances in the R than in the S line during 6–24 HAT, including CYPRO4, CYP313A4, and CYP51H11 (asterisk marked genes in Figure 5). The maximum difference was observed for CYPRO4 at 6 HAT, with an approximately 10-fold difference in transcript abundance. Similarly, the transcript levels of CYP51H11 also showed the maximum difference at 6 HAT. By contrast, the maximum difference in CYP313A4 transcript levels was observed at 12 HAT, with more than fourfold difference in transcript abundance.
Figure 5. Comparison of relative expression levels of each putative P450 gene in the S and R lines of Polypogon fugax before and after fenoxaprop-P-ethyl treatment. The X-axis represents the time (h) after fenoxaprop-P-ethyl treatment, and the Y-axis represents the ratio of relative transcript levels in the R line relative to that in the S line at each sampling time point. Data are the mean values ± SEM. Blue dots marked genes were constitutively up-regulated more than threefold in the R than in the S line, orange dots marked genes were fenoxaprop-P-ethyl-induced up-regulated more than threefold in the R than in the S line for at least one time point, and black dots marked genes were both constitutively and herbicide-induced up-regulated more than threefold in the R than in the S line. Asterisk marked genes were constantly up-regulated in the R than in the S line during 6–24 h after treatment. *Foldchange ≥2 and p < 0.05.
As described in the above two sections, a total of six genes were up-regulated more than 10-fold in the R line by fenoxaprop-P-ethyl treatment. The transcript levels of these six genes were then compared between the susceptible and fenoxaprop-P-ethyl-resistant lines. Of these, CYP709B1, CYP71A1-4, and P450-39 were constitutively up-regulated in the R relative to the S line, while CYP709B1, CYP71A1-4, CYP711A1, CYP78A9, and P450-11 were fenoxaprop-P-ethyl-induced up-regulated in the R relative to the S line for at least one time point after fenoxaprop-P-ethyl treatment.
To improve the rigor of the gene selection and filter possible false-positive genes, we selected the candidate fenoxaprop-P-ethyl metabolism-related P450 genes according to the following criteria: the gene (1) was up-regulated by herbicide treatment more than 10-fold in the R line and (2) up-regulated in the R relative to the S line before and/or after fenoxaprop-P-ethyl treatment. In addition, the genes constantly up-regulated in the R relative to the S line after herbicide treatment were also selected. Therefore, a total of nine genes separately annotated as CYPRO4, CYP313A4, CYP51H11, CYP709B1, CYP71A1-4, CYP711A1, CYP78A9, P450-11, and P450-39 were identified as potential fenoxaprop-P-ethyl metabolism-related genes in P. fugax.
Overexpression of genes encoding the target enzymes such as ACCase or herbicide-detoxifying ones such as P450s is a common mechanism of herbicide resistance (Gaines et al., 2020). To investigate the internal molecular mechanisms, gene expression analysis by RT-qPCR is usually conducted in weed plants subjected to herbicide stress. A set of stably expressed reference genes is fundamental for RT-qPCR data normalization in specific plant species. Polypogon fugax is one of the most problematic weeds in the world, which severely infests Chinese wheat fields (Tang et al., 2015). Our previous study has found that the P. fugax population AHHY was highly resistant to the ACCase-inhibiting herbicide fenoxaprop-P-ethyl, and pre-treatment with the P450 inhibitor piperonyl butoxide markedly increased their susceptibility to fenoxaprop-P-ethyl (by c. 55%; Zhao et al., 2019). Here we further determined significantly higher P450 contents in the AHHY than in the susceptible plants before and after fenoxaprop-P-ethyl treatment (Figure 3), implying that P450s are most likely to play a key role in the resistance. To determine which P450 genes could be responsible for the metabolic resistance, this current study first identified a set of stably expressed reference genes for RT-qPCR data normalization in P. fugax under herbicide stress as well as other experimental conditions. The entire P450 genes were then successfully isolated from the full-length transcriptome established previously, and their transcript abundances were compared between susceptible and fenoxaprop-P-ethyl-resistant biotypes of P. fugax with or without herbicide treatment.
Since the first study identifying TUB, GAPDH, and UBQ as the suitable internal controls in A. myosuroides, stably expressed reference genes have also been determined in several other arable weeds subsequently (Xu et al., 2016, 2017; Scarabel et al., 2017; Zhao et al., 2018; Su et al., 2020). In this study, seven candidate reference genes stably expressed in different plant species were selected and assessed by using two P. fugax populations with contrasting phenotypes. Three algorithms, namely geNorm, NormFinder, and the comparative ΔCq method, were used to determine the most stably expressed genes. Although the results calculated from different algorithms were similar in each data (sub)set, the specific ranking of expression stability was different. Therefore, RefFinder was finally used to generate an overall ranking based on the geometric mean of weights of each gene calculated by each algorithm. Generally, genes with average Cq values ranged from 15.0 to 30.0 were acceptable for using as internal controls (Picard et al., 2006). In the present study, the expression levels of candidate genes were varied, with their mean Cq values ranged from 12.6 to 26.4. All the seven candidate genes were included as optional genes to determine the most stably expressed genes in P. fugax. The most suitable reference genes characterized in the invasive weed P. fugax under herbicide stress as well as other experimental conditions will greatly facilitate studies related to biological science in this grass species.
Polypogon fugax is reported to be a hexaploid grass species (Akopian and Ghukasyan, 2018), and multiple copies of ACCase are expected in this weed. Previously, we had isolated at least four loci encoding plastidic ACCase from resistant P. fugax plants and found the Trp-1999-Ser mutation occurred in their ACCase1,1–2 alleles (Zhao et al., 2019). However, if ACCase overexpression was also involved in the resistance remains unclear. In the present study, triplicated experiments consistently revealed similar ΔCq values for ACCase between the S and R biotypes, indicating that the R biotype survived the fenoxaprop-P-ethyl treatment without involving in the overexpression of the plastid ACCase. Similar results are also observed in cyhalofop-butyl-resistant Chinese sprangletop (Leptochloa chinensis (L.) Nees; Deng et al., 2019; Zhao et al., 2022a) and mesosulfuron-methyl-resistant American sloughgrass (Beckmannia syzigachne Steud.; Wang et al., 2019), in which target-gene overexpression is not involved in the resistance phenotypes.
In recent years, gene duplication of 5-enolpyruvylshikimate-3-phosphate synthase (EPSPS) has been frequently identified in glyphosate-resistant biotypes of arable weeds (Gaines et al., 2010; Salas et al., 2012; Nandula et al., 2014; Chatham et al., 2015; Malone et al., 2016; Dillon et al., 2017). This implies there are “easier” evolutionary solutions, such as target-site mutation and enhanced herbicide metabolism, to achieve resistance to the majority of other herbicides (Tranel, 2017). Recently, Laforest et al. (2017) reported ACCase amplification (about fivefold to eightfold more copies) as a resistance mechanism to fenoxaprop-P-ethyl in large crabgrass (Digitaria sanguinalis), indicating the gene amplification may be not a rare herbicide-resistance mechanism. Target gene overexpression was also identified in several other weed species exhibiting resistance to herbicides with different modes of action (Zhao et al., 2018; Sen et al., 2021). Although ACCase overexpression is not related to the fenoxaprop-P-ethyl resistance in P. fugax, relevant reports still inspire us to investigate gene overexpression or amplification as a potential resistance mechanism more routinely.
P450s-medidated enhanced metabolism has been identified as a major NTSR mechanism in many herbicide-resistant weed biotypes, such as Japanese foxtail (Alopecurus japonicus Steud.; Chen et al., 2018), tall morningglory (Ipomoea purpurea (L.) Roth; Van Etten et al., 2020), corn poppy (Papaver rhoeas L.; Torra et al., 2021), and poverty brome (Bromus sterilis L.; Sen et al., 2021). As documented, plant P450s catalyze diverse chemical reactions, while the reactions for P450-mediated herbicide metabolism primarily involve alkyl-hydroxylation, N-demethylation, O-demethylation, and aryl-hydroxylation (Dimaano and Iwakami, 2021). Higher P450 activity may be caused by higher specific activities of the enzymes in the resistant plants and/or by higher expression levels of the genes encoding the P450 enzymes (Iwakami et al., 2014). In the present study, higher P450 contents were also determined in the resistant than in the susceptible plants without or with fenoxaprop-P-ethyl treatment. A total of 48 putative P450 genes were then isolated from P. fugax, and they showed distinct expression patterns in the S and R plants. Of these, six genes, respectively, annotated as CYP709B1, CYP71A1-4, CYP711A1, CYP78A9, P450-11, and P450-39 were fenoxaprop-P-ethyl-induced up-regulated more than 10-fold in the R plants, and all of them showed higher transcript levels in the R than in the S plants for at least one sampling time point. Three genes, respectively, annotated as CYPRO4, CYP313A4, and CYP51H11 constantly up-regulated in the R than in the S plants after fenoxaprop-P-ethyl treatment. Collectively, these results indicate the above nine genes can be regarded as the primary candidates for fenoxaprop-P-ethyl metabolism mediated by higher P450 activity. Interestingly, compared with our previous omics analysis (Zhao et al., 2022b), several new P450s except that annotated as into families of CYP709 and CYP81 were additionally identified as major candidates for fenoxaprop-P-ethyl metabolism. The temporal dynamic changes in P450 expressions under herbicide stress may fill the gap caused by sampling simplicity and higher false positive in omics analysis and thus further supplement the results. Notably, herbicide selectivity is normally guaranteed by the crop-specific high expression of herbicide-metabolizing gene(s) (Iwakami et al., 2014). It will be of great significant to investigate whether these genes are orthologous genes for crop selectivity, which may reveal if the resistance is conferred by completely different P450 family members.
In summary, here we systematically characterized the expression pattern of entire P450 genes in a metabolic-herbicide-resistant biotype of P. fugax. The basal and/or herbicide-induced up-regulation of several specific P450 genes was positively related to the higher P450 contents in the resistant plants, which can be served as a foundation for uncovering the mechanism for P450-mediated herbicide resistance. Future studies such as ectopic overexpression and gene knockdown will aid in elucidating the molecular mechanisms for metabolic-herbicide resistance in P. fugax.
The original contributions presented in the study are included in the article/Supplementary Material, further inquiries can be directed to the corresponding author.
NZ designed the research study. JY, MJ, and SJ performed the experiments. NZ and JY carried out data analysis. NZ wrote the manuscript. All authors contributed to the article and approved the submitted version.
This research was funded by the Anhui Provincial Natural Science Foundation (No. 2108085QC115), the National Natural Science Foundation of China (No. 32102237), and the Talent Research Project of Anhui Agricultural University (No. rc342004).
The authors declare that the research was conducted in the absence of any commercial or financial relationships that could be construed as a potential conflict of interest.
All claims expressed in this article are solely those of the authors and do not necessarily represent those of their affiliated organizations, or those of the publisher, the editors and the reviewers. Any product that may be evaluated in this article, or claim that may be made by its manufacturer, is not guaranteed or endorsed by the publisher.
We thank the editors and reviewers for their useful and insightful comments on drafts of the manuscript.
The Supplementary Material for this article can be found online at: https://www.frontiersin.org/articles/10.3389/fpls.2022.868807/full#supplementary-material
Akopian, J., and Ghukasyan, A. (2018). Taxonomical inventory and karyological studies of Poaceae family representatives of Ararat salt marshes. Electron J. Nat. Sci. 30, 12–22.
Andersen, C. L., Jensen, J. L., and Ørntoft, T. F. (2004). Normalization of real-time quantitative reverse transcription-PCR data: a model-based variance estimation approach to identify genes suited for normalization, applied to bladder and colon cancer data sets. Cancer Res. 64, 5245–5250. doi: 10.1158/0008-5472.CAN-04-0496
Chatham, L. A., Wu, C., Riggins, C. W., Hager, A. G., Young, B. G., Roskamp, G. K., et al. (2015). EPSPS gene amplification is present in the majority of glyphosate-resistant Illinois waterhemp (Amaranthus tuberculatus) populations. Weed Technol. 29, 48–55. doi: 10.1614/WT-D-14-00064.1
Chen, W., Wu, L., Wang, J., Yu, Q., Bai, L., and Pan, L. (2020). Quizalofop-p-ethyl resistance in Polypogon fugax involves glutathione S-transferases. Pest Manag. Sci. 76, 3800–3805. doi: 10.1002/ps.5931
Chen, G., Xu, H., Zhang, T., Bai, C., and Dong, L. (2018). Fenoxaprop-P-ethyl resistance conferred by cytochrome P450s and target site mutation in Alopecurus japonicus. Pest Manag. Sci. 74, 1694–1703. doi: 10.1002/ps.4863
Délye, C., Jasieniuk, M., and Le Corre, V. (2013). Deciphering the evolution of herbicide resistance in weeds. Trends Genet. 29, 649–658. doi: 10.1016/j.tig.2013.06.001
Deng, W., Cai, J., Zhang, J., Chen, Y., Chen, Y., Di, Y., et al. (2019). Molecular basis of resistance to ACCase-inhibiting herbicide cyhalofop-butyl in Chinese sprangletop (Leptochloa chinensis (L.) Nees) from China. Pestic. Biochem. Physiol. 158, 143–148. doi: 10.1016/j.pestbp.2019.05.004
Dillon, A., Varanasi, V. K., Danilova, T. V., Koo, D. H., Nakka, S., Peterson, D. E., et al. (2017). Physical mapping of amplified copies of the 5-enolpyruvylshikimate-3-phosphate synthase gene in glyphosate-resistant Amaranthus tuberculatus. Plant Physiol. 173, 1226–1234. doi: 10.1104/pp.16.01427
Dimaano, N. G., and Iwakami, S. (2021). Cytochrome P450-mediated herbicide metabolism in plants: current understanding and prospects. Pest Manag. Sci. 77, 22–32. doi: 10.1002/ps.6040
Dombrowski, J. E., and Martin, R. C. (2009). Evaluation of reference genes for quantitative RT-PCR in Lolium temulentum under abiotic stress. Plant Sci. 176, 390–396. doi: 10.1016/j.plantsci.2008.12.005
Duhoux, A., and Délye, C. (2013). Reference genes to study herbicide stress response in Lolium sp.: up-regulation of P450 genes in plants resistant to acetolactate-synthase inhibitors. PLoS One 8:e63576. doi: 10.1371/journal.pone.0063576
Estabrook, R. W., and Werringloer, J. (1978). The measurement of difference spectra: application to the cytochromes of microsomes. Methods Enzymol. 52, 212–220. doi: 10.1016/S0076-6879(78)52024-7
Gaines, T. A., Duke, S. O., Morran, S., Rigon, C., Tranel, P. J., Küpper, A., et al. (2020). Mechanisms of evolved herbicide resistance. J. Biol. Chem. 295, 10307–10330. doi: 10.1074/jbc.REV120.013572
Gaines, T. A., Patterson, E. L., and Neve, P. (2019). Molecular mechanisms of adaptive evolution revealed by global selection for glyphosate resistance. New Phytol. 223, 1770–1775. doi: 10.1111/nph.15858
Gaines, T. A., Zhang, W., Wang, D., Bukun, B., Chisholm, S. T., Shaner, D. L., et al. (2010). Gene amplification confers glyphosate resistance in Amaranthus palmeri. Proc. Natl. Acad. Sci. U. S. A. 107, 1029–1034. doi: 10.1073/pnas.0906649107
Giacomini, D. A., Gaines, T., Beffa, R., and Tranel, P. J. (2017). Optimizing RNA-seq studies to investigate herbicide resistance. Pest Manag. Sci. 74, 2260–2264. doi: 10.1002/ps.4822
Han, H., Yu, Q., Beffa, R., González, S., Maiwald, F., Wang, J., et al. (2021). Cytochrome P450 CYP81A10v7 in Lolium rigidum confers metabolic resistance to herbicides across at least five modes of action. Plant J. 105, 79–92. doi: 10.1111/tpj.15040
Iwakami, S., Kamidate, Y., Yamaguchi, T., Ishizaka, M., Endo, M., Suda, H., et al. (2019). CYP81A P450s are involved in concomitant cross-resistance to acetolactate synthase and acetyl-CoA carboxylase herbicides in Echinochloa phyllopogon. New Phytol. 221, 2112–2122. doi: 10.1111/nph.15552
Iwakami, S., Uchino, A., Kataoka, Y., Shibaike, H., Watanabe, H., and Inamura, T. (2014). Cytochrome P450 genes induced by bispyribac-sodium treatment in a multiple-herbicide-resistant biotype of Echinochloa phyllopogon. Pest Manag. Sci. 70, 549–558. doi: 10.1002/ps.3572
Jarošová, J., and Kundu, J. K. (2010). Validation of reference genes as internal control for studying viral infections in cereals by quantitative real-time RT-PCR. BMC Plant Biol. 10:146. doi: 10.1186/1471-2229-10-146
Jefcoate, C. R. (1978). Measurement of substrate and inhibitor binding to microsomal cytochrome P-450 by optical difference spectroscopy. Methods Enzymol. 52, 258–279. doi: 10.1016/S0076-6879(78)52029-6
Jugulam, M., and Shyam, C. (2019). Non-target-site resistance to herbicides: recent developments. Plants 8:417. doi: 10.3390/plants8100417
Kato, S., Yokota, Y., Suzuki, R., Fujisawa, Y., Sayama, T., Kaga, A., et al. (2020). Identification of a cytochrome P450 hydroxylase, CYP81E22, as a causative gene for the high sensitivity of soybean to herbicide bentazon. Theor. Appl. Genet. 133, 2105–2115. doi: 10.1007/s00122-020-03580-6
Kiarash, J. G., Wilde, H. D., Amirmahani, F., Moemeni, M. M., Zaboli, M., Nazari, M., et al. (2018). Selection and validation of reference genes for normalization of qRT-PCR gene expression in wheat (Triticum durum L.) under drought and salt stresses. J. Genet. 97, 1433–1444. doi: 10.1007/s12041-018-1042-5
Kong, Q., Gao, L., Cao, L., Liu, Y., Saba, H., Huang, Y., et al. (2016). Assessment of suitable reference genes for quantitative gene expression studies in melon fruits. Front. Plant Sci. 7:1178. doi: 10.3389/fpls.2016.01178
Laforest, M., Soufiane, B., Simard, M. J., Obeid, K., Page, E., and Nurse, R. E. (2017). Acetyl-CoA carboxylase overexpression in herbicide resistant large crabgrass (Digitaria sanguinalis). Pest Manag. Sci. 73, 2227–2235. doi: 10.1002/ps.4675
Malone, J. M., Morran, S., Shirley, N., Boutsalis, P., and Preston, C. (2016). EPSPS gene amplification in glyphosate-resistant Bromus diandrus. Pest Manag. Sci. 72, 81–88. doi: 10.1002/ps.4019
Nandula, V. K., Wright, A. A., Bond, J. A., Ray, J. D., Eubank, T. W., and Molin, W. T. (2014). EPSPS amplification in glyphosate-resistant spiny amaranth (Amaranthus spinosus): a case of gene transfer via interspecific hybridization from glyphosate-resistant palmer amaranth (Amaranthus palmeri). Pest Manag. Sci. 70, 1902–1909. doi: 10.1002/ps.3754
Nicot, N., Hausman, J. F., Hoffmann, L., and Evers, D. (2005). Housekeeping gene selection for real-time RT-PCR normalization in potato during biotic and abiotic stress. J. Exp. Bot. 56, 2907–2914. doi: 10.1093/jxb/eri285
Owen, W. J. (1991). “Herbicide metabolism as a basis for selectivity,” in Metabolism of Agrochemicals in Plants. ed. T. Roberts (Chichester, UK: John Wiley & Sons, Ltd), 211–258.
Picard, C., Silvy, M., and Gabert, J. (2006). “Overview of real-time RT-PCR strategies for quantification of gene rearrangements in the myeloid malignancies,” in Myeloid Leukemia. eds. I. Harry, H. Mark, and M. Paula (New Jersey: Humana Press), 27–68.
Rice, P., Longden, I., and Bleasby, A. (2000). EMBOSS: the European molecular biology open software suite. Trends Genet. 16, 276–277. doi: 10.1016/S0168-9525(00)02024-2
Salas, R. A., Dayan, F. E., Pan, Z., Watson, S. B., Dickson, J. W., Scott, R. C., et al. (2012). EPSPS gene amplification in glyphosate-resistant Italian ryegrass (Lolium perenne ssp. multiflorum) from Arkansas. Pest Manag. Sci. 68, 1223–1230. doi: 10.1002/ps.3342
Scarabel, L., Milani, A., Panozzo, S., and Rasori, A. (2017). Suitable reference genes for accurate gene expression analysis in Papaver rhoeas under 2,4-D herbicide stress. Pestic. Biochem. Physiol. 143, 66–72. doi: 10.1016/j.pestbp.2017.09.008
Sen, M. K., Hamouzová, K., Mikulka, J., Bharati, R., Košnarová, P., Hamouz, P., et al. (2021). Enhanced metabolism and target gene overexpression confer resistance against acetolactate synthase-inhibiting herbicides in Bromus sterilis. Pest Manag. Sci. 77, 2122–2128. doi: 10.1002/ps.6241
Silver, N., Best, S., Jiang, J., and Thein, S. L. (2006). Selection of housekeeping genes for gene expression studies in human reticulocytes using real-time PCR. BMC Mol. Biol. 7:33. doi: 10.1186/1471-2199-7-33
Su, X., Lu, L., Li, Y., Zhen, C., Hu, G., Jiang, K., et al. (2020). Reference gene selection for quantitative real-time PCR (qRT-PCR) expression analysis in Galium aparine L. PLoS One 15:e0226668. doi: 10.1371/journal.pone.0226668
Tang, W., Xu, X. Y., Shen, G. Q., and Chen, J. (2015). Effect of environmental factors on germination and emergence of aryloxyphenoxy propanoate herbicide-resistant and-susceptible Asia minor bluegrass (Polypogon fugax). Weed Sci. 63, 669–675. doi: 10.1614/WS-D-14-00156.1
Tang, W., Zhou, F. Y., Chen, J., and Zhou, X. G. (2014). Resistance to ACCase-inhibiting herbicides in an Asia minor bluegrass (Polypogon fugax) population in China. Pestic. Biochem. Physiol. 108, 16–20. doi: 10.1016/j.pestbp.2013.11.001
Torra, J., Rojano-Delgado, A. M., Menéndez, J., Salas, M., and de Prado, R. (2021). Cytochrome P450 metabolism-based herbicide resistance to imazamox and 2, 4-D in Papaver rhoeas. Plant Physiol. Biochem. 160, 51–61. doi: 10.1016/j.plaphy.2021.01.007
Tranel, P. J. (2017). Herbicide-resistance mechanisms: gene amplification is not just for glyphosate. Pest Manag. Sci. 73, 2225–2226. doi: 10.1002/ps.4679
Van Etten, M., Lee, K. M., Chang, S. M., and Baucom, R. S. (2020). Parallel and nonparallel genomic responses contribute to herbicide resistance in Ipomoea purpurea, a common agricultural weed. PLoS Genet. 16:e1008593. doi: 10.1371/journal.pgen.1008593
Vandesompele, J., De Preter, K., Pattyn, F., Poppe, B., Van Roy, N., De Paepe, A., et al. (2002). Accurate normalization of real-time quantitative RT-PCR data by geometric averaging of multiple internal control genes. Genome Biol. 3:research0034.1. doi: 10.1186/gb-2002-3-7-research0034
Wang, L. F., Jin, S., Wu, L. M., Zhou, X. M., Liu, X. Y., and Bai, L. Y. (2016). Influence of environmental factors on seed germination and emergence of Asia minor bluegrass (Polypogon fugax). Weed Technol. 30, 533–538. doi: 10.1614/WT-D-15-00043
Wang, H. C., Li, J., Lv, B., Lou, Y. L., and Dong, L. Y. (2013). The role of cytochrome P450 monooxygenase in the different responses to fenoxaprop-P-ethyl in annual bluegrass (Poa annua L.) and short awned foxtail (Alopecurus aequalis Sobol.). Pestic. Biochem. Physiol. 107, 334–342. doi: 10.1016/j.pestbp.2013.10.001
Wang, M., Liu, B., Li, Y., Luo, X., and Li, L. (2019). Non-target site based resistance to the ALS-inhibiting herbicide mesosulfuron-methyl in American sloughgrass (Beckmannia syzigachne). Weed Sci. 67, 527–533. doi: 10.1017/wsc.2019.22
Werck-Reichhart, D., and Feyereisen, R. (2000). Cytochromes P450: a success story. Genome Biol. 1:reviews3003.1. doi: 10.1186/gb-2000-1-6-reviews3003
Wrzesińska, B., Kierzek, R., and Obrępalska-Stęplowska, A. (2016). Evaluation of six commonly used reference genes for gene expression studies in herbicide-resistant Avena fatua biotypes. Weed Res. 56, 284–292. doi: 10.1111/wre.12209
Xie, F., Xiao, P., Chen, D., Xu, L., and Zhang, B. (2012). miRDeepFinder: a miRNA analysis tool for deep sequencing of plant small RNAs. Plant Mol. Biol. 80, 75–84. doi: 10.1007/s11103-012-9885-2
Xu, H., Li, J., Wu, R., Su, W., Wu, X., Wang, L., et al. (2017). Identification of reference genes for studying herbicide resistance mechanisms in Japanese foxtail (Alopecurus japonicus). Weed Sci. 65, 557–566. doi: 10.1017/wsc.2017.19
Xu, X., Liu, X., Chen, S., Li, B., Wang, X., Fan, C., et al. (2016). Selection of relatively exact reference genes for gene expression studies in flixweed (Descurainia sophia) by quantitative real-time polymerase chain reaction. Pestic. Biochem. Physiol. 127, 59–66. doi: 10.1016/j.pestbp.2015.09.007
Yu, X., Wu, H., Zhang, J., Yang, Y., Tang, W., and Lu, Y. (2021). Resistance mechanism to metsulfuron-methyl in Polypogon fugax. Plants 10:1309. doi: 10.3390/plants10071309
Zhao, N., Ge, L., Yan, Y., Bai, S., Wang, D., Liu, W., et al. (2019). Trp-1999-Ser mutation of acetyl-CoA carboxylase and cytochrome P450s-involved metabolism confer resistance to fenoxaprop-P-ethyl in Polypogon fugax. Pest Manag. Sci. 75, 3175–3183. doi: 10.1002/ps.5435
Zhao, N., Jiang, M., Li, Q., Gao, Q., Zhang, J., Liao, M., et al. (2022a). Cyhalofop-butyl resistance conferred by a novel Trp-2027-Leu mutation of acetyl-CoA carboxylase and enhanced metabolism in Leptochloa chinensis. Pest Manag. Sci. 78, 1176–1186. doi: 10.1002/ps.6734
Zhao, N., Yan, Y., Wang, H., Bai, S., Wang, Q., Liu, W., et al. (2018). Acetolactate synthase overexpression in mesosulfuron-methyl-resistant shortawn foxtail (Alopecurus aequalis Sobol.): reference gene selection and herbicide target gene expression analysis. J. Agric. Food Chem. 66, 9624–9634. doi: 10.1021/acs.jafc.8b03054
Keywords: fenoxaprop-P-ethyl metabolism, P450 gene expression, internal control, mesosulfuron-methyl, Polypogon fugax, RT-qPCR
Citation: Yang J, Jiang M, Jia S, Liao M, Cao H and Zhao N (2022) Expression Pattern of Entire Cytochrome P450 Genes and Response of Defenses in a Metabolic-Herbicide-Resistant Biotype of Polypogon fugax. Front. Plant Sci. 13:868807. doi: 10.3389/fpls.2022.868807
Received: 03 February 2022; Accepted: 07 March 2022;
Published: 25 March 2022.
Edited by:
Joel Torra, Universitat de Lleida, SpainReviewed by:
Chandrima Shyam, Kansas State University, United StatesCopyright © 2022 Yang, Jiang, Jia, Liao, Cao and Zhao. This is an open-access article distributed under the terms of the Creative Commons Attribution License (CC BY). The use, distribution or reproduction in other forums is permitted, provided the original author(s) and the copyright owner(s) are credited and that the original publication in this journal is cited, in accordance with accepted academic practice. No use, distribution or reproduction is permitted which does not comply with these terms.
*Correspondence: Ning Zhao, emhhb25AYWhhdS5lZHUuY24=
Disclaimer: All claims expressed in this article are solely those of the authors and do not necessarily represent those of their affiliated organizations, or those of the publisher, the editors and the reviewers. Any product that may be evaluated in this article or claim that may be made by its manufacturer is not guaranteed or endorsed by the publisher.
Research integrity at Frontiers
Learn more about the work of our research integrity team to safeguard the quality of each article we publish.