- 1Department of Plant Biotechnology, College of Life Sciences and Biotechnology, Korea University, Seoul, South Korea
- 2Department of Forest Bio Resources, National Institute of Forest Science, Suwon, South Korea
- 3Department of Molecular Biotechnology, College of Agriculture and Life Sciences, Bioenergy Research Center, Chonnam National University, Gwangju, South Korea
- 4Institute of Life Science and Natural Resources, Korea University, Seoul, South Korea
Plants acquire nitrogen, an essential macronutrient, from the soil as nitrate. Since nitrogen availability is a major determinant of crop productivity, the soil is amended with nitrogenous fertilizers. Extensive use of irrigation can lead to the accumulation of salt in the soil, which compromises crop productivity. Our characterization of NODULE INCEPTION (NIN)-like PROTEIN 7 (NLP7), a transcription factor regulating the primary response to nitrate, revealed an intersection of salt stress and nitrate metabolism. The growth of loss-of-function mutant nlp7 was tolerant to high salinity that normally reduces the fresh weight and chlorophyll and protein content of wild type (Col-0). On a medium with high salinity, the nlp7 experienced less stress, accumulating less proline, producing less nitric oxide (NO) and reactive oxygen species (ROS), and expressing lower transcript levels of marker genes, such as RD29A and COR47, than Col-0. Nevertheless, more sodium ions were translocated to and accumulated in the shoots of nlp7 than that of Col-0. Since nlp7 also expressed less nitrate reductase (NR) activity, nitrate accumulated to abnormally high levels with or without salinity. We attributed the enhanced salt tolerance of nlp7 to the balanced accumulation of nitrate anions and sodium cations. Our results suggest that nitrate metabolism and signaling might be targeted to improve salt tolerance.
Introduction
Nitrogen is an essential macronutrient, and its availability may be a limiting factor for crop productivity. To meet the increasing demand for food, nitrogen-based fertilizers are increasingly utilized in agriculture worldwide (Nosengo, 2003), and consumption of fertilizer is expected to increase from 1.25 to 236 million tons by 2050. However, from the fertilizer applied to soil, only 30–50% of added nitrogen is actually absorbed by plants (Good and Beatty, 2011), and residual nitrogen is released into the surrounding environment, causing severe pollution and ecological imbalance through natural processes (Garnett et al., 2009). Thus, protecting the environment while increasing crop productivity is not an easy task. In addition, these competing demands must also take into account, global climate change.
The Arabidopsis NODULE INCEPTION (NIN)-LIKE PROTEIN (NLP) gene family encodes the core transcription factors that regulate nitrate signaling in plants (Wang et al., 2018). Among these, NLP7 acts as a master regulator, controlling the primary response in nitrate signaling (Konishi and Yanagisawa, 2013; Marchive et al., 2013; Liu et al., 2017; Zhang et al., 2021). The loss-of-function nlp7 exhibits a nitrogen-deficient phenotype as well as tolerance to drought (Castaings et al., 2009). While the vital role of NLP7 in the transcriptional response to nitrate has been characterized, the mechanism responsible for tolerance of nlp7 to abiotic stress has not been established.
Nitrogen metabolism also interacts with responses to salt stress. In particular, nitric oxide (NO) plays a pivotal role in responses to various abiotic stresses, such as drought and high osmotic potential. The NO accumulates with osmotic stress and is likely a product of nitrate reductase (NR) (Kolbert et al., 2010). Treatment of wheat with NO promotes stomatal closure and enhances drought tolerance (García-Mata and Lamattina, 2001), and supplying osmotically stressed wheat seedlings with NO that reduces water loss and promotes abscisic acid (ABA) accumulation (Xing et al., 2004). At low concentrations, NO positively contributes to stress signaling; however, at higher concentrations of NO, this free radical causes damage to cells (Del Rio et al., 2004).
Due to irrigation practices and climate change, soil salinization affects 1–10 billion hectares of arable land in over 100 countries (Zhang et al., 2017). In this new century, salinization is predicted to impact half of the arable land adversely. The major abiotic stressors, drought, and salinity, are already responsible for substantial crop production losses worldwide (Mahajan and Tuteja, 2005; Munns, 2011). To alleviate the effects of salt stress on crops and maximize crop productivity, an understanding of the various physiological phenomena contributing to salt tolerance at a molecular level is needed.
High salinity increases the osmotic potential of water in the soil, hindering the ability of plants to acquire water, and the water deficit ultimately leads to reduced growth (Machado and Serralheiro, 2017). Salinity also impacts plant growth by disrupting cell ion homeostasis through the toxic effects of excess sodium and chloride ions in the plant body (Munns et al., 2012) and inhibition of the uptake of essential nutrients (Paranychianakis and Chartzoulakis, 2005; Naeem et al., 2010).
Early signaling in response to salinity includes redistribution of intracellular calcium, generation of reactive oxygen species (ROS), phosphorylation by protein kinases, and accumulation of and transcriptional response to the hormone, ABA. The induction of ABA-independent genes in response to salt stress is also reported (Hussain et al., 2021). Salinity-induced signaling ultimately leads to altered physiology, such as changes in growth and development, redistribution of intracellular ions, and synthesis of compatible solutes (Acosta-Motos et al., 2017; Zhao et al., 2020).
In addition, salt stress is known to alter uptake, assimilation, and transport of nitrate in plant cells (Lin et al., 2008; Yao et al., 2008) through the inhibition of the activities of enzymes for assimilation of nitrate, including nitrate transporters and nitrate reductase (Goel and Singh, 2015). Moreover, in plants coping with the detrimental effects of high salinity, the downregulation and upregulation of NRT1.5 and NRT1.8, respectively, are associated with improved tolerance to salt (Fan et al., 2007; Han et al., 2016).
From an effort to understand the response of NLP7 to abiotic stress, we find that nlp7 performs better than wild type (Col-0) on saline medium, although NLP7 is induced by salinity. Moreover, the tolerance of nlp7 to salt stress is associated with attenuated responses indicative of salt stress. We attribute the better performance of nlp7 to the abnormal accumulation of nitrate resulting from reduced expression of NR activity in nlp7.
Materials and Methods
Plant Growth Materials
Two T-DNA-tagged mutants, namely, nlp7-1 [SALK_026134.54.75. (SALK_026134c)] and nlp7-2 [SALK_114886.35.50.x (CS868891)], were obtained from the Arabidopsis Biological Resource Center (ABRC), Ohio State University. The T-DNA right-border primer LBb1.3 (5′-ATTTTGCCGATTTCGGAAC-3′), the NLP7 full-length forward primer (5′-ATGTGCGAGCCCGATGATAATTC-3′), and the NLP7 full-length reverse primer (5′-TCACAATTCTCCAGTGCTCTCGCAG-3′) were used for PCR screening. Arabidopsis thaliana Col-0 was used as the wildtype. The seeds were sterilized, stored at 4°C for 3 days, and then inoculated on nitrogen-free half-strength Murashige and Skoog (MS) medium containing 2% sucrose, 0.5% phytagel, and 0.5 mM (low nitrate) or 5 mM (normal nitrate) KNO3 as the sole nitrogen source (pH 5.8). The seedlings were grown in a growth chamber at 23 ± 1°C under a 16/8 h light/dark cycle, 50–55 μmol photons m–2⋅s–1 photosynthetic photon flux density, and 70% humidity. After 4 days of germination, the seedlings were transferred on the same medium supplemented with 150 or 200 mM NaCl or KCl. The K+ concentration was adjusted to 10 mM by adding K2SO4 to all NaCl media with KNO3 concentration below 10 mM.
Quantitative Reverse Transcription Polymerase Chain Reaction
The RNA was extracted from whole 9-day-old plants, as described by Lee et al. (2021). Moloney Murine Leukemia Virus (M-MLV) reverse transcriptase was used to synthesize the first-strand of complementary DNA (cDNA). Real-time reverse transcription polymerase chain reaction (RT-PCR) was conducted by using EvaGreen 2 × qPCR MasterMix (Applied Biological Materials Inc., Richmond, Canada). The housekeeping gene, AtActin2 was used as the internal control. The primers used for PCR are listed in Supplementary Table 1.
Chlorophyll Assay
Spectrophotometry was used to detect chlorophyll content. Briefly, chlorophyll was extracted from the investigated seedlings in the phenotypic experiments. First, the samples (100 mg) were ground to a fine powder in liquid nitrogen. The powdered samples were transferred to a 1.5 mL tube and incubated at 21°C with 700 μL of 80% acetone solution. The solutions were gently mixed in the dark for 30 min to protect chlorophyll from light damage. The mixture was then centrifuged at 3,000 rpm at 4°C for 15 min. Absorbance was measured at 663 and 645 nm. The following equations were used to estimate chlorophyll content:
where, V = volume of the extract and W = fresh weight of leaves.
Bradford Assay
After nine days of growth in the control medium, Col-0 and nlp7 seedlings were exposed to 0, 150, or 200 mM NaCl for 1 day. Then, 500 μL of PRO-PREPTM protein extraction solution (iNtRON Biotechnology Inc., Gyeonggi, Republic of Korea) was added to the ground samples, and the mixtures were vortexed. Cell lysis was achieved by incubation at –20°C for 20–30 min, followed by centrifugation at 13,000 rpm and 4°C for 5 min. The supernatant was transferred to a fresh tube, and the samples were incubated at –20°C until used for further experiments. The protein content was determined using the BCA Protein assay kit (Merck Millipore, MA, United States). For the assay, 1 mL of bovine serum albumin solution was prepared in distilled water (10 mg⋅mL–1) and then diluted to 1 mg⋅mL–1 for use. The reaction mixtures were prepared in tubes. Next, 200 μL of the reaction mixture was transferred to a 96-well microplate, and absorbance was measured at 595 nm. Microsoft Excel was used for constructing a standard curve to calculate the protein content.
Intracellular Nitric Oxide Detection Assay
Endogenous NO content was semi-quantitatively analyzed using a NO-specific fluorescent probe (diaminofluorescein-based dye, DAF-FM DA), as Guo et al. (2003) described with some modifications. After passing through the cell membrane, DAF-FM DA accumulates within the cell. The binding of nonfluorescent DAF-FM to intracellular NO leads to the generation of a fluorescent triazole product (Li et al., 2016). In this assay, 9-day-old seedlings were exposed to 150 mM NaCl for 6 h and then stained with 5 μM DAF-FM DA in 20 mM HEPES-NaOH (pH 7.5) for 30 min in the dark. Next, the stained seedlings were washed with the same buffer three times for 5 min each and incubated in the dark for 1 h before visualizing under a laser confocal scanning microscope (LSM 700; Zeiss, Jena, Germany). The excitation wavelength was 488 nm, and the emission wavelength was 515–565 nm.
Nitrate Reductase Activity Assay
After 9 days of growth in the control medium, Col-0 and nlp7 seedlings were exposed to 0, 150, or 200 mM NaCl for 1 day. The NR was isolated, and its content was determined using an NR assay kit (BC0080, SolarBio, Beijing, China). Briefly, the samples (100 mg) were extracted in 1 ml of extraction solution and centrifuged at 4,000 × g for 10 min. The supernatant was collected and used for further analyses. Absorbance at 520 nm was used to calculate NR activity.
Nitrate Content Assay
Samples (50 mg) of 9-day-old Col-0 and nlp7 seedlings grown in the control medium and then treated with 0, 150, or 200 mM NaCl for 1 day were collected for nitrate content measurements as described by Zhao and Wang (2017). The pretreated samples were ground in liquid nitrogen. Then, 1 mL of distilled water was added to the samples, followed by boiling the samples for 20 min. The mixture was centrifuged at 13,000 rpm at 4°C for 10 min. Next, 100 μl of the supernatant was mixed with 400 μl of sulfosalicylic acid in a 15 ml Falcon tube and incubated at room temperature for 30 min. Then, 9.5 ml of 8% NaOH solution was added to the samples, and the mixture was cooled at 4°C for 5 min. Nitrate content was calculated based on absorbance at 410 nm.
Chloride Content Assay
Chloride content was analyzed using the Ferricyanide method described by Hunt (1982) and Pruefer (2001). Briefly, after 9 days of growth in the control medium, Col-0 and nlp7 seedlings were exposed to 0, 150, or 200 mM NaCl for 1 day. Samples (50 mg) of pretreated seedlings were collected and ground in liquid nitrogen. Collected samples were incubated in 5 ml of 0.5 M HNO3 solution in an oven at 80oC for 1 h. Then, solid materials were allowed to settle to the bottom of the vial. A stock solution containing mercuric thiocyanate solution, Hg(SCN)2 (4.17 g/l distilled water), and ferric nitrate solution, Fe(NO3)3•9H2O (202 g/l DW), was prepared. Then, a combined color regent was made by adding 150 ml stock Hg(SCN)2 solution to 150 ml stock Fe(NO3)3 solution and diluted to 1 L with distilled water. Next, 1 ml of the supernatant was mixed with 3 ml of the color regent in a 15 ml Falcon tube. Chloride content was calculated from a standard curve obtained with Cl– standard solutions using a spectrophotometer with the wavelength set at 480 nm.
15N-Uptake Assay
Nitrate uptake was analyzed using 15NO3–, as previously described by Lin et al. (2008). Briefly, seedlings were grown in a nitrogen-free half-strength MS medium with or without 200 mM NaCl for 9 days, treated with 0.1 mM CaSO4 for 1 min, and then transferred into a fresh nutrient solution supplemented with 20 mM K15NO3– (99% atom), as the sole N source, for 1 h. The seedlings were treated with 0.1 mM CaSO4 for 1 min once again. The roots were dried at 70°C to a constant weight and ground. The 15N content was analyzed using a continuous-flow isotope mass spectrometer (Thermo-MAT253) coupled to an elemental analyzer (Flash 2000 HT, Thermo Fisher Scientific Inc., MA, United States).
Promoter Activity Assay
An approximately 1.45 kb genomic fragment of the NLP7 promoter (–231 to –1,682) was PCR-amplified using the NLP7-proGUS forward (5′-GGGCCAACTATAGAGGAATGGT-3′) and reverse (5′-ACAATACAACTGTGCCCCAAAT3′) primers. After sequencing, the promoter fragment was cloned in front of the reporter GUS gene in the binary vector, pMDC162. This vector was then introduced into Agrobacterium tumefaciens and finally transformed into Col-0 using the floral dip method (Clough and Bent, 1998). Putative transformants were selected on half-strength MS media supplemented with hygromycin B (25 mg⋅L–1). The β-glucuronidase (GUS) staining was performed as described by Jefferson et al. (1987), with some modifications. Briefly, 9-day-old NLP7p::GUS transgenic seedlings were pretreated under the abovementioned conditions for 1 day, followed by GUS staining. The Leica EZ4D microscope (Leica Microsystems1) was used to examine the stained seedlings.
Proline Content Measurement
Proline content was measured as described previously (Bates et al., 1973). Briefly, proline was isolated from 100 mg of plant leaves by grinding in 1 ml of 3% sulfosalicylic acid. A 200 μl aliquot of the extract was allowed to react with 100 μl ninhydrin (80% glacial acetic acid, 6.8% phosphoric acid, and 70.17 mM ninhydrin) for 60 min at 100°C. An ice bath was used to stop the reaction. Then, the reaction mixture was treated with 200 μl of toluene and vortexed. The absorbance of the toluene layer was measured at 520 nm using a UV/VIS spectrophotometer. Finally, proline content was estimated by extrapolation on a standard curve and calculated on the FW basis as follows: [(ng proline ml–1× ml extraction buffer)/115.5 ng nmol] g–1 sample = nmol proline g–1 FW material.
Malondialdehyde Content Measurement
After 24 h of salt treatment, samples were harvested, and the malondialdehyde (MDA) content was assayed according to the study by Zhang et al. (2020) with small modifications. Seedlings were ground in liquid nitrogen, homogenized in 1.5 ml of 20% (w/v) trichloroacetic acid (TCA), and centrifuged at 10,000 × g for 5 min. To a 1 ml aliquot of the supernatant, 2 mL of thiobarbituric acid solution [0.5% (w/v) in 20% TCA] was added. Then, the mixture was heated at 95°C for 15 min, rapidly cooled in an ice bath, and centrifuged at 12,000 × g for 10 min. The absorbance of the supernatant was measured at 450, 532, and 600 nm, and the MDA content was calculated using the following equation:
where, OD = optical density.
Measurement of Abscisic Acid Content
Using 200 mg samples of seedlings treated with 0, 150, or 200 mM NaCl for 1 day, the ABA content was determined as described previously (Liu et al., 2014; Jeong et al., 2018) using a commercial kit (Phytodetek Elisa kit, PDK 09347, Agdia, Inc., Elkhart, Indiana, United States) according to the manufacturer’s instructions.
Na+ and K+ Ion Content Measurement
The cation content was determined as described by Choi and Gilroy (2015). Briefly, 100 mg samples of Col-0 and nlp7 seedlings germinated and grown on nitrogen-free half-strength MS medium containing 5 mM KNO3 and treated with 200 mM NaCl for 6–24 h were used. The pretreated seedlings were washed multiple times with deionized water to remove any Na+ and K+ on the surface. In 20 mL glass test tubes, the samples were digested with 0.6 mL of concentrated HNO3 at 150°C until the plant tissues were entirely dissolved. Next, 0.4 mL of HClO4 was added, and the samples were continuously digested further at 180°C until the total sample volume dropped below 0.5 mL. Then, the extracts were cooled down to room temperature, and the final volume was adjusted to 5 mL. The Na+ and K+ content were determined using ICP-OES (Agilent Technologies Inc., CA, United States) from a standard curve obtained with Na+ and K+ standard solutions. Moreover, the Na+-to-K+ ratio was calculated based on the measured Na+ and K+ content.
Gene Accession Numbers
The ABRC accession numbers of gene sequences used in the present article are provided in parentheses: NLP7 (AT4G24020), NLP6 (AT1G64530), NRT1.1 (AT1G12110), HY5 (AT5G11260), COR47 (AT1G20440), RD29A (AT5G52310), NIA1 (AT1G77760), NIA2 (AT1G37130), NRT1.5 (AT1G32450), NRT1.8 (AT4G21680), NCED3 (AT3G14440), BG1 (AT3G57270), and BG2 (AT3G57260), ACTIN2 (AT3G18780).
Statistical Analyses
To obtain reliable results, each experiment was independently repeated at least three times. All statistical analyses were performed using one-way ANOVA, followed by Tukey’s test, for the comparison of means with a 95% confidence level. Different letters (a, b, c, …) indicate significant differences at P < 0.05. Error bars represent standard deviation (SD).
Results
Salt-Induced NLP7 Conditions Tolerance to Salinity
A previous study showed that nlp7 exhibits tolerance to drought (Castaings et al., 2009), which led us to examine the tolerance of this mutant to salinity. Thus, we assessed the performance of nlp7 grown in stressful concentrations of NaCl. Four-day-old seedlings of Col-0, nlp7-1, and nlp7-2 were transferred from agar medium without added NaCl to medium supplemented with 0, 150 and 200 mM NaCl. After 2 weeks, there was no difference in the growth performance of Col-0 and nlp7 in the absence of NaCl; however, on high salinity, nlp7 showed a higher tolerance. Although all plants grew less on saline medium, nlp7 grew noticeably better than Col-0 (Figure 1A). Moreover, nlp7 exhibited higher fresh weight, chlorophyll content, and protein content than Col- 0 in the presence of 150 mM NaCl, and these differences were still greater at a higher NaCl concentration (200 mM) (Figure 1B). In contrast, the performance of Col-0 and nlp7 grown on medium supplemented with KCl (100 and 200 mM) was indistinguishable although all plants appeared more sensitive to KCl than to NaCl (Supplementary Figures 1A,B).
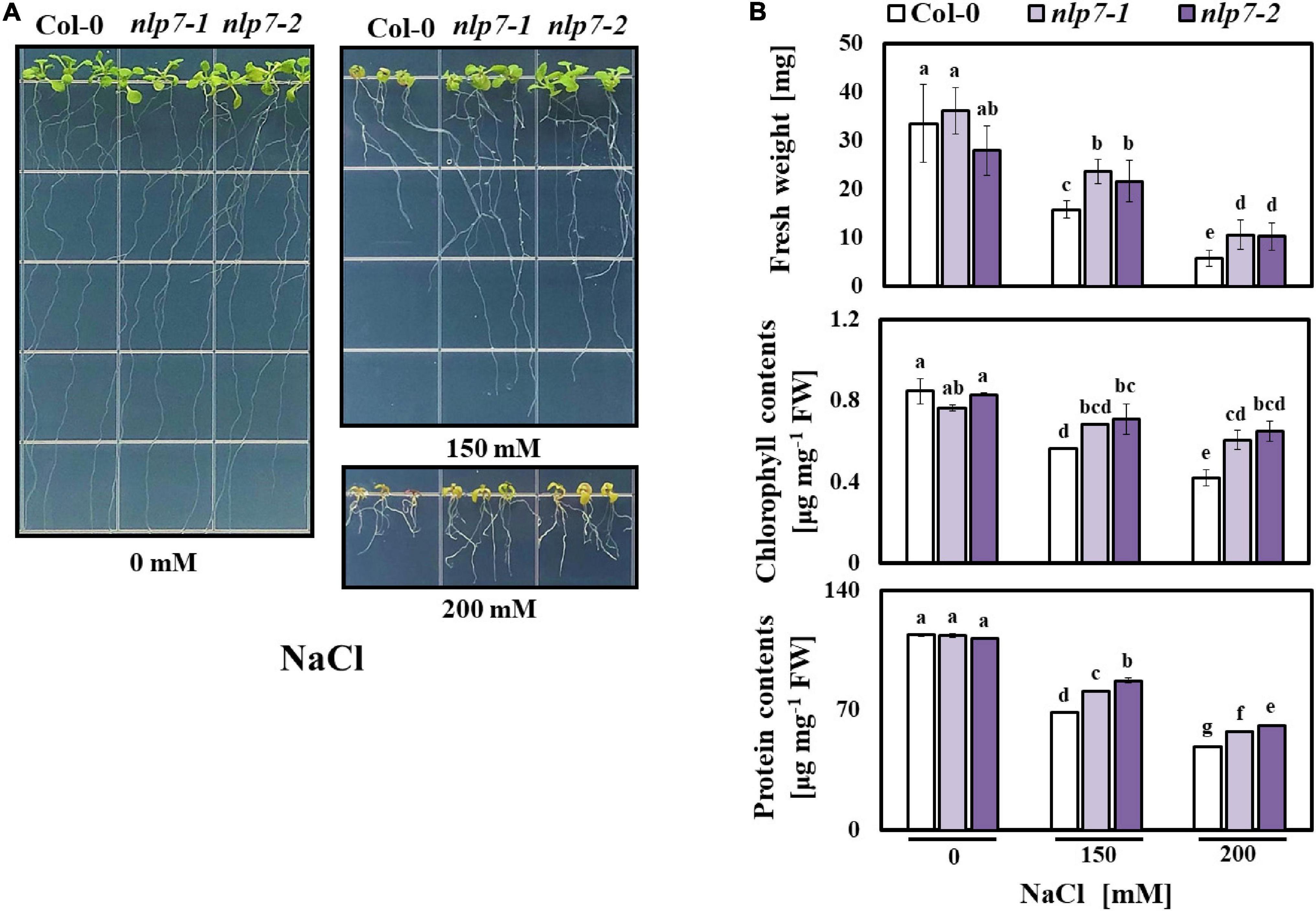
Figure 1. The nlp7 mutants showed enhanced salt tolerance. (A) Comparison of the growth performance of Col-0 and nlp7 plants under salt stress. Four-day-old Col-0, nlp7-1, and nlp7-2 seedlings were germinated and grown on a nitrogen-free half-strength MS medium containing 5 mM KNO3 and then transferred to a media supplemented with 0, 150, or 200 mM NaCl. After 2 weeks, each phenotype was confirmed. (B) Quantification of fresh weight, chlorophyll content, and protein content of Col-0, nlp7-1, and nlp7-2 seedlings in response to salt stress. Error bars represent the standard deviation of three biological replicates. Different letters (a, b, c, or d) within a treatment group indicate significant differences in the two-way ANOVA (P ≤ 0.05, Tukey’s test).
To examine whether the expression of NLP7 responds to salinity, we measured its transcript levels in Col-0 exposed to NaCl (200 mM) at time points of up to 48 h (5 min, 0.5 h, 1 h, 3 h, 6 h, 18 h, 24 h, and 48 h, in Figure 2). The expression of NLP7 initially remained low from 5 min to 1 h after exposure to NaCl, abruptly peaked at 3 and 6 h and then gradually declined out to 48 h. Transcription factors NLP7 and its close homolog NLP6 serve vital functions in nitrate signaling and, in particular, promote expression of NITRATE TRANSPORTER 1.1 (NRT1.1/NPLC 3.8, Konishi and Yanagisawa, 2013; Marchive et al., 2013). NRT1.1 encodes a transceptor (dual affinity transporter and sensor) involved in nitrate uptake and nitrate-dependent gene regulation (Huang et al., 1996; Wang et al., 1998; Liu et al., 1999; Ho et al., 2009). While the transcriptional response of NLP7 to NaCl peaked by 3 h, the transcript level of NRT1.1 was maximally induced only after 18 h. The induced expression of stress marker genes COR47 and RD29A confirmed that salt stress was successfully caused in the present study (Figure 2).
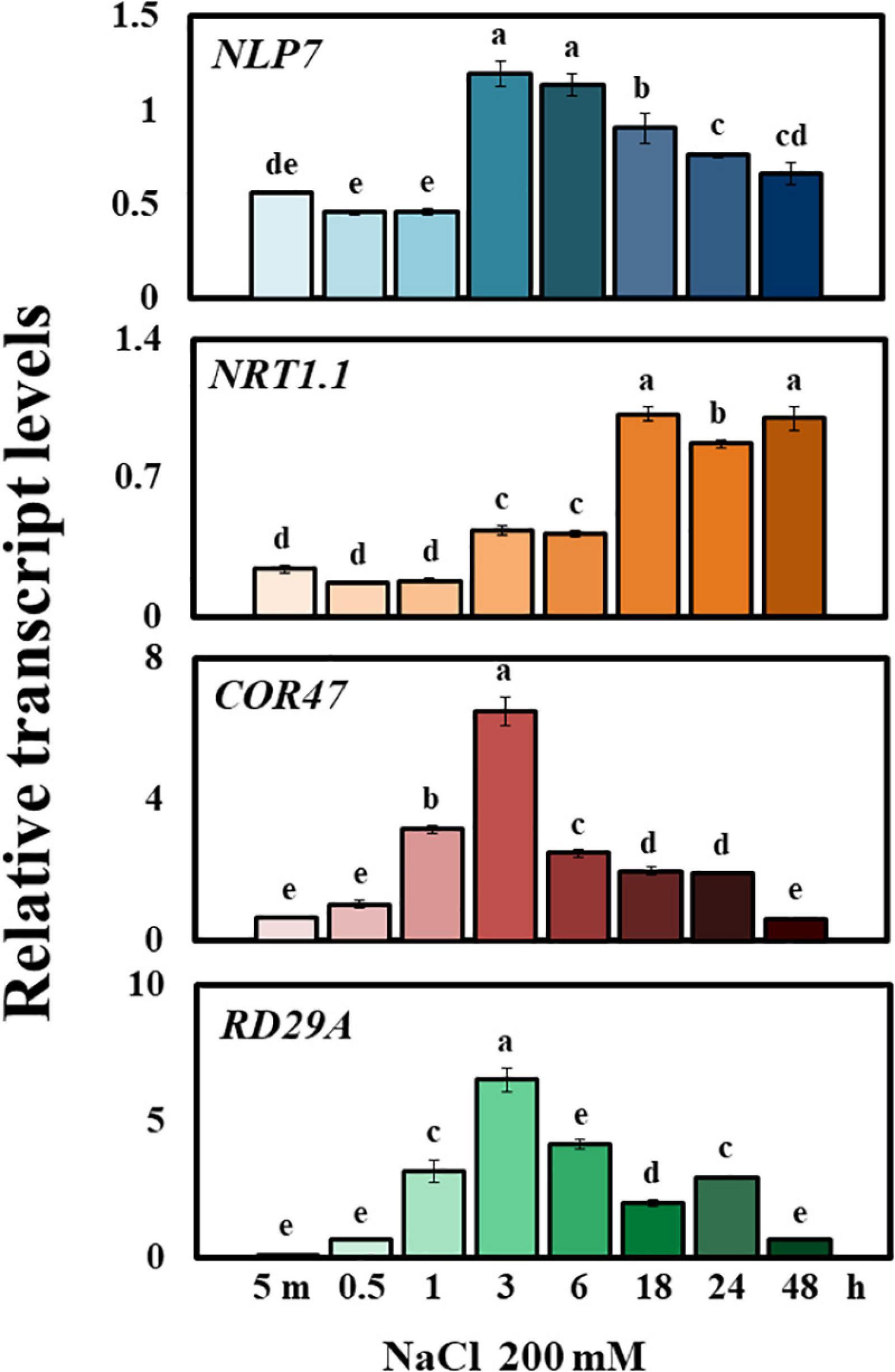
Figure 2. NLP7 transcript levels were elevated in response to external salt treatment. Relative transcript levels of NLP7, NRT1.1, COR47, and RD29A in Col-0 and nlp7 plants, as determined using qPCR. The seeds were germinated and grown on a nitrogen-free half-strength MS medium containing 5 mM KNO3 for 9 days and then treated with 200 mM NaCl for 5 min to 48 h. AtActin2 was used as the internal control. Error bars represent the standard deviation of three independent replicates. Different letters (a, b, c, d, or e) within a treatment group indicate significant differences in the two-way ANOVA (P ≤ 0.05, Tukey’s test).
The Promoter of NLP7 Responds to Salt and Osmotic Stress
Since salinity enhanced the transcript levels of NLP7, we examined the transcriptional regulation of the promoter of NLP7 using plants stably transformed with NLP7p::GUS, a fusion of genomic sequence (1.45 kb) upstream of NLP7 to the reporter gene, GUS. Following exposure to 200 mM NaCl, the GUS activity of transformants gradually increased with time under stress (at 6, 24, and 48 h); and the activity was higher in shoots than in the roots (Figure 3A). To determine whether promoter activity specifically responded to salt stress, we examined other treatments. Similar to NaCl, both mannitol (300 mM) and KCl (200 mM) activated NLP7p::GUS, whereas ABA (5 μM) and IAA (0.5 μM) did not activate NLP7p::GUS (Figure 3B). In a previous study, GUS activity of a reporter gene similar to NLP7p::GUS increased in the guard cells of transgenic plants, which induces drought resistance (Castaings et al., 2009). High salinity affects plants through two mechanisms, osmotic stress and ion toxicity (Ma et al., 2020). The early response of plants to salt stress is similar to drought stress due to a decline in the water potential (Navarro et al., 2008; Ma et al., 2020). Our results show that the promoter of NLP7 responded to osmotic stress and salinity.
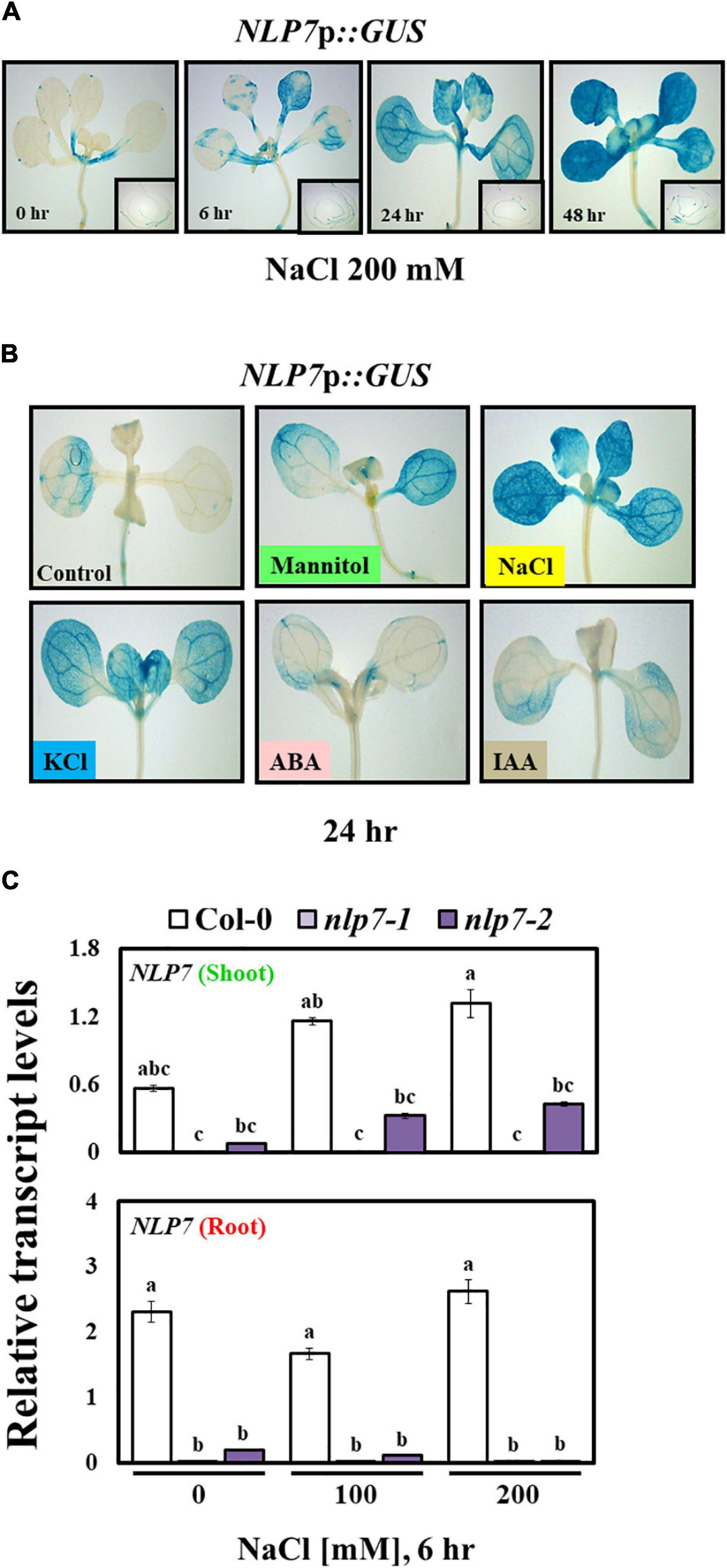
Figure 3. NLP7 promoter activity was induced by salt, mannitol, and KCl. (A) Histochemical β-glucuronidase (GUS) staining of transgenic Arabidopsis seedlings harboring the NLP7p::GUS fusion construct. The seedings were germinated and grown on half-strength MS medium containing 5 mM KNO3 for 9 days and then treated with 200 mM NaCl for 0, 6, 24, or 48 h. (B) Histochemical GUS staining of the 9-day-old seedlings grown on a nitrogen-free half-strength MS medium containing 5 mM KNO3 in response to 300 mM mannitol, 200 mM NaCl, 200 mM KCl, 5 μM ABA, and 0.5 μM IAA for 24 h; untreated seedlings were used as controls. (C) Relative transcript levels of NLP7 in the shoots and roots of 9-day-old Col-0, nlp7-1, and nlp7-2 seedlings following exposure to 0, 150, or 200 mM NaCl for 6 h. AtActin2 was used as the internal control. Error bars represent the standard deviation of three independent replicates. Different letters (a, b, or c) within a treatment group indicate significant differences in the two-way ANOVA (P ≤ 0.05, Tukey’s test).
We further examined the transcript accumulation of NLP7 separately in the shoots and the roots in response to salt stress. Following treatment with 150 and 200 mM NaCl, transcripts of NLP7 substantially accumulated in shoots and not in the roots of Col-0 in response to NaCl, which was consistent with the pattern of GUS activity in transgenic plants (Figure 3C). Similarly, following treatment with 100 and 200 mM KCl, the transcript levels of NLP7 appreciably increased in the shoots and not in the roots (Supplementary Figure 2). Considering that NLP7 is a transcriptional regulator of nitrate response and a member of a family of homologous genes, we examined how nlp7 mutants affect the expression of NLP6. The transcript level of NLP6 was unaffected by salt stress in both the shoots and the roots, and the levels were no different between Col-0 and nlp7 with or without salt stress (Supplementary Figure 3).
The nlp7 Accumulated More Nitrate Than Col-0 in the Shoots
Since NLP7 is involved in nitrate signaling (Zhao et al., 2018), we examined the possible role of NO, a signaling molecule for salt stress tolerance (Nabi et al., 2019), in the enhanced tolerance of nlp7 plants to salt stress. NO is enzymatically generated from nitrate as a byproduct of NR (Meyer et al., 2005). Thus, reduced NO levels may result from the reduced activity of NR in nlp7 (Figure 4). Fluorescence of the dye, DAF-FM DA was used to measure the endogenous NO levels in planta (Figure 5A). The roots of nlp7 showed lower NO levels following treatment with 0, 150, or 200 mM NaCl for 30 min to 6 h than those of Col-0 (Figure 5B).
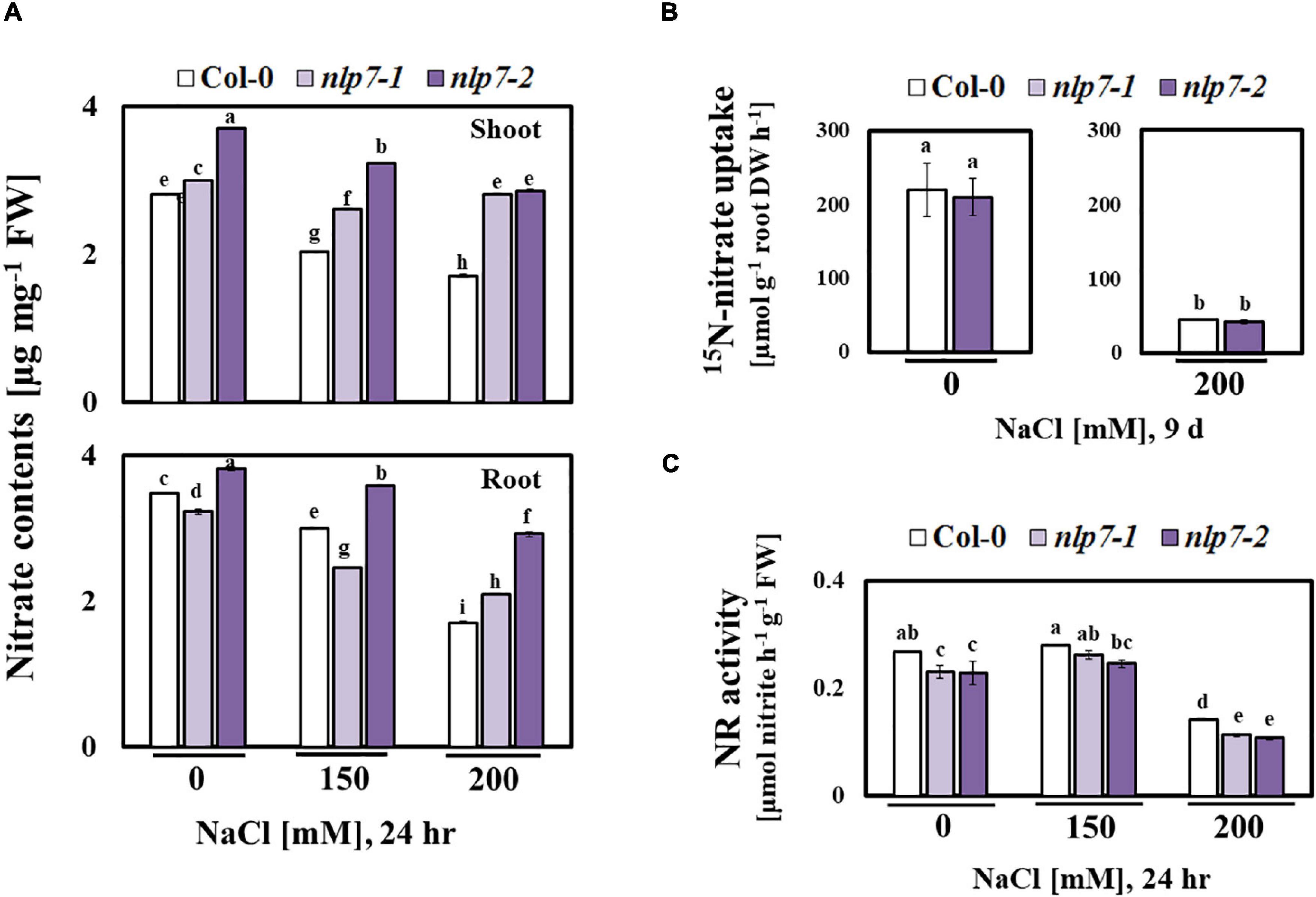
Figure 4. Nitrate content was elevated, and nitrate reductase activity was altered in nlp7 mutants under salt stress. (A) The nitrate content of the shoots and the roots of 9-day-old Col-0, nlp7-1, and nlp7-2 seedlings was measured. The seeds were germinated and grown on nitrogen-free half-strength MS medium containing 5 mM KNO3 for 9 days and then treated with 0, 150, and 200 mM NaCl for 24 h. Error bars represent the standard deviation of three independent replicates. (B) 15NO3 uptake in the roots of Col-0 and nlp7-2 seedlings. The seedlings were germinated and grown on a nitrogen-free half-strength MS medium with or without 200 mM NaCl for 9 days and then transferred to a medium containing 20 mM of 15NO3– and kept for 1 h. Error bars represent the standard deviation of three independentreplicates. (C) Comparison of nitrate reductase activity under salt stress between 9-day-old Col-0 and nlp7 seedlings. Error bars represent the standard deviation of three independent replicates. Different letters (a, b, c, d, e, f, g, h, or i) within a treatment group indicate significant differences in the two-way ANOVA (P ≤ 0.05, Tukey’s test).
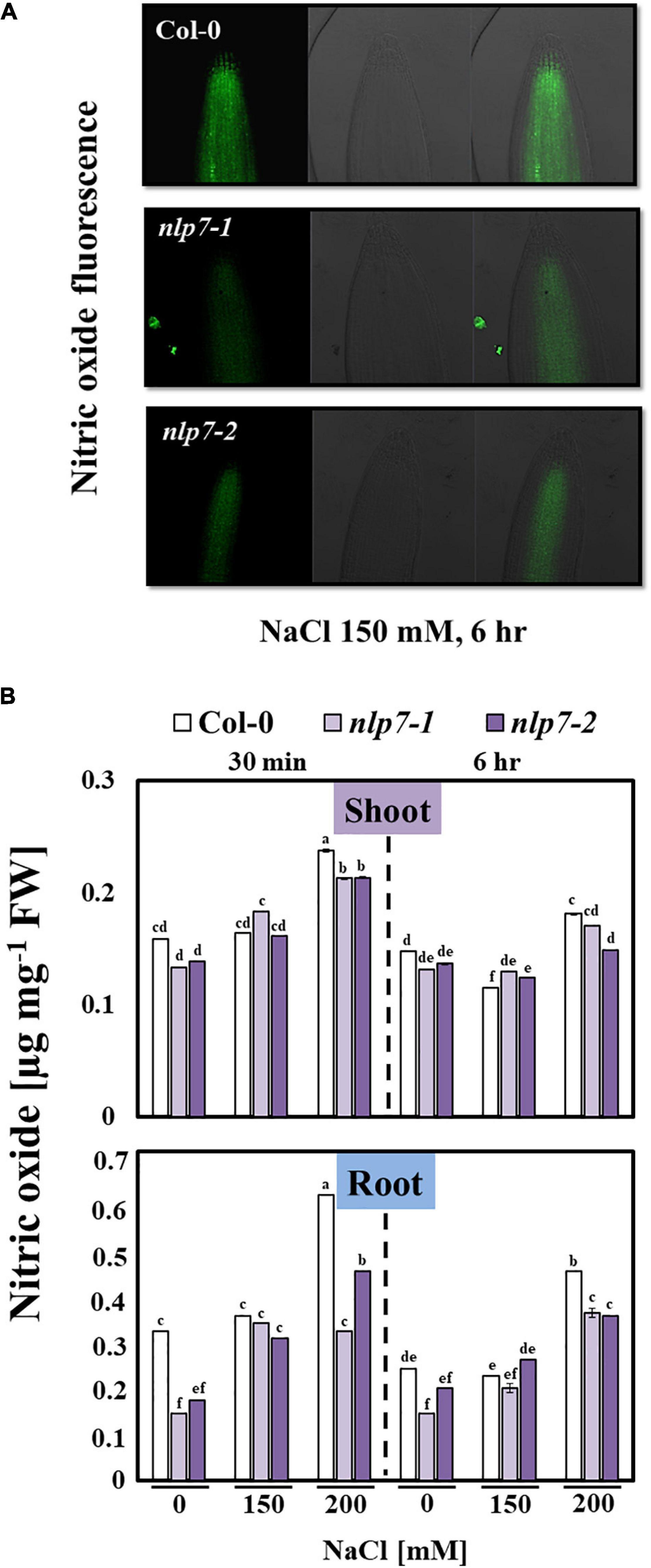
Figure 5. The nlp7 mutants showed reduced nitric oxide (NO) content under salt stress. (A) The NO accumulation in the root tips of Col-0 and nlp7 seedlings following exposure to 150 mM NaCl. The Col-0, nlp7-1, and nlp7-2 seedlings were germinated and grown on a nitrogen-free half-strength MS medium containing 5 mM KNO3 for 9 days and then treated with 200 mM NaCl for 6 h. The roots of the test seedlings were incubated with 5 μM of DAF-FM DA in 20 mM HEPES-NaOH (pH 7.5) for 30 min in the dark, and the NO-associated fluorescence was visualized using a laser confocal scanning microscope (LSM 700; Zeiss, Jena, Germany). The excitation wavelength was 488 nm, and emission wavelength was 515–565 nm. Images on the left were obtained under green fluorescence, and the ones in the middle were obtained under bright-field microscopy; the images of green fluorescence and bright field microscopy were merged and are shown on the right. (B) The NO content of the shoots and the roots of Col-0, nlp7-1, and nlp7-2 seedlings was measured following treatment with 0, 150, or 200 mM NaCl for 30 min to 6 h. Error bars represent the standard deviation of three independent replicates. Different letters (a, b, c, d, e, or f) within a treatment group indicate significant differences in the two-way ANOVA (P ≤ 0.05, using Tukey’s test).
To examine the nitrate content, we separately collected the shoot and root tissues of Col-0 and nlp7 and found no difference in the nitrate content of Col-0 and nlp7 in the absence of salt stress; however, the nitrate content of shoots (and not roots) of nlp7 was higher than Col-0 under high salinity (Figure 4A). Since plants could accumulate higher chloride anion under high salt stress, we also measured the chloride content and found no difference between Col-0 and nlp7 (Supplementary Figure 4). Moreover, the nitrate uptake of nlp7 and Col-0 was similar following treatment with 15N-labeled KNO3 with or without 200 mM NaCl (Figure 4B). Likewise, Yu et al. (2016) have reported a similar nitrate uptake by nlp7 and Col-0 plants in the absence of salinity.
Importantly, the transcription factor, NLP7 binds to the nitrate response element, a cis-element, in the promoter region of NIA1, encoding a critical component of NR, and regulates its transcription (Konishi and Yanagisawa, 2019). Thus, we measured the NR activity of Col-0 and nlp7 under salt stress. As shown in Figure 4C, the NR activity was lower in nlp7 than Col-0, suggesting that the accumulation of nitrate in nlp7 may be a consequence of reduced consumption by NR.
To explain how nitrate is taken up by the roots could have accumulated in the shoots of nlp7, we compared the transcript levels of various nitrate transporter genes in nlp7 and Col-0. In particular, we found that the expression of NRT1.5, encoding a transporter that uploads nitrate into the xylem (Raddatz et al., 2020), was noticeably reduced in the roots of nlp7 treated with 0 and 150 mM NaCl; whereas, the expression of NRT1.8, which unloads nitrate from the xylem (Raddatz et al., 2020), was elevated in the shoots and reduced in the roots of nlp7 (Figure 6). These results suggest that the excess nitrate accumulation in the shoots of nlp7 may be due to an enhanced expression of NRT1.8.
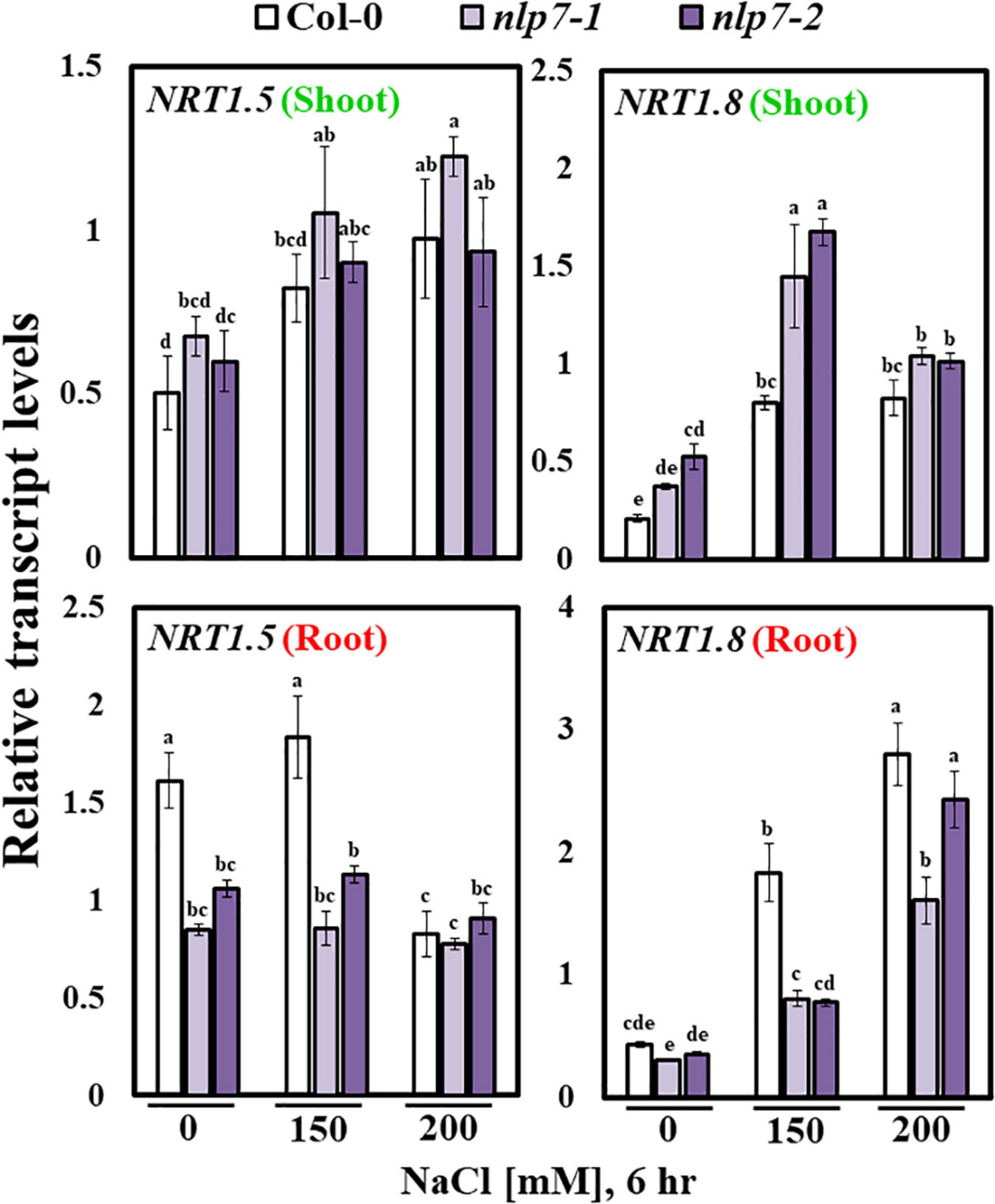
Figure 6. Transcript levels of nitrate transporter genes were altered in nlp7 mutants. The relative transcript levels of the nitrate transporter genes, NRT1.5 (transporter of nitrate from the roots to the xylem) and NRT1.8 (responsible for nitrate unloading from the xylem and transport to the surrounding shoot organs) in the shoots and the roots of Col-0 and nlp7 seedlings, as determined using qPCR. Nine-day-old seedlings were germinated and grown on a nitrogen-free half-strength MS medium containing 5 mM KNO3; treated with 0, 150, or 200 mM NaCl for 6 h; and then dissected into shoot and root parts. AtActin2 was used as the internal control. Error bars represent the standard deviation of three independent replicates. Different letters (a, b, c, d, e, f, g, or h) within a treatment group indicate significant differences in the two-way ANOVA (P ≤ 0.05, Tukey’s test).
Nitrate-Driven Ion Homeostasis May Contribute to the Enhanced Salt Tolerance of nlp7 Plants
Next, we addressed whether enhanced tolerance to salt was due to the reduced accumulation of Na+ in nlp7. The rate of translocation of Na+ from the roots to the shoots was measured at 6 h and 24 h after exposure to 200 mM NaCl. At 6 h, the rate was no different in Col-0 and nlp7; however, at 24 h, the rate increased substantially in Col-0 and not nlp7 (Figure 7A). In contrast to shoots, the accumulation of Na+ and K+ in roots under salt stress was lower in Col-0 and higher in nlp7 (Supplementary Figure 5). A higher ratio of Na+/K+ in the roots possibly accounted for the reduced root growth (Figure 7B) of nlp7. In addition to being an anabolic source of nitrogen, nitrate is a persistent anion in cells and contributes to the ion homeostasis of plants by balancing the excessive Na+ cations from salt stress (Raddatz et al., 2020).
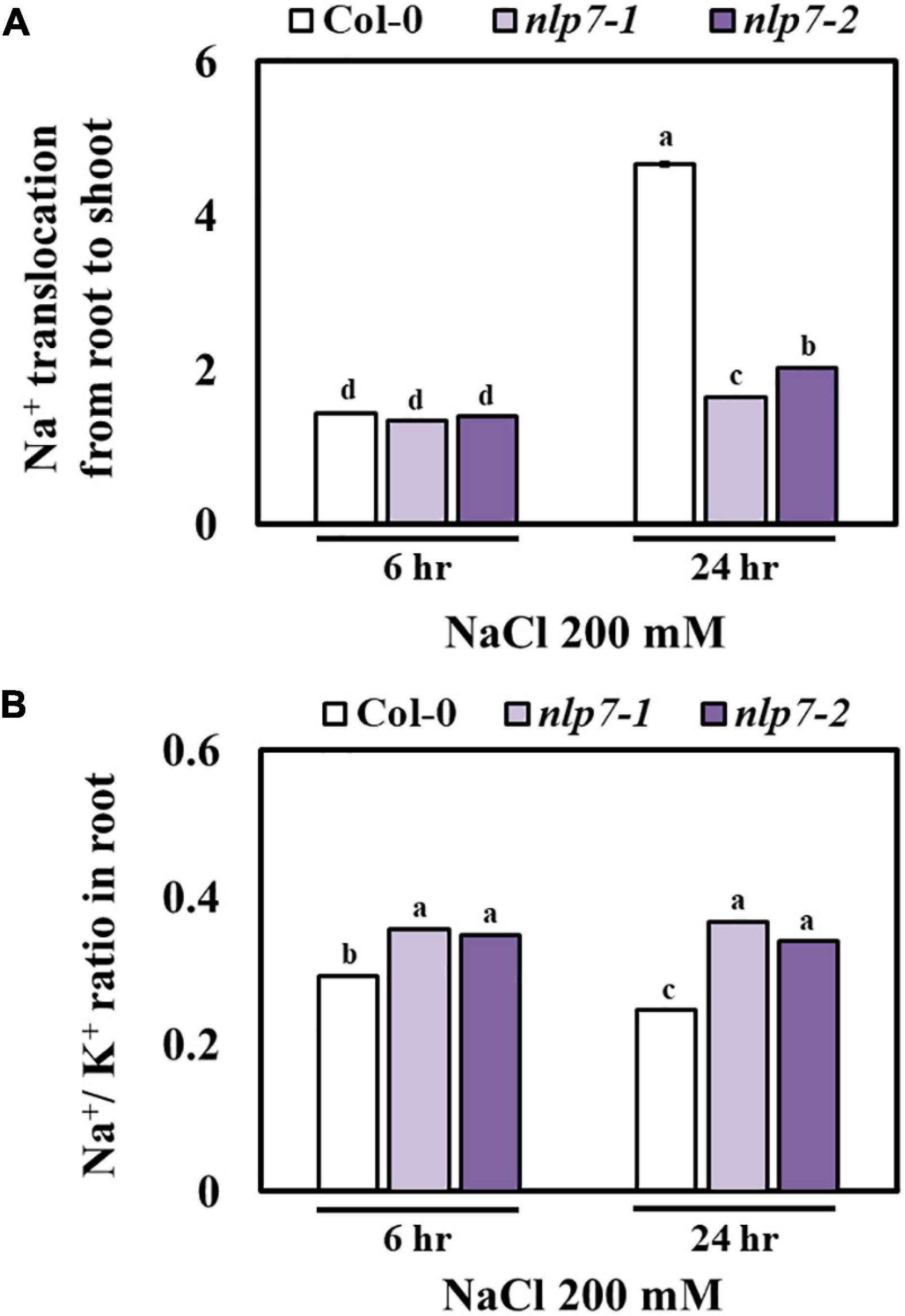
Figure 7. Na+ translocation to the shoots of nlp7 mutants was decreased under salt stress. (A) The Na+ translocation from the roots to the shoots in Col-0, nlp7-1, and nlp7-2 seedlings in response to salt stress. The seeds were germinated and grown on a nitrogen-free half-strength MS medium containing 5 mM KNO3 and then treated with 200 mM NaCl for 6–24 h. Na+ translocation from the roots to the shoots was estimated based on the Na+ and K+ content of the roots and the shoots using ICP-OES (Agilent) calculated from a standard curve obtained with Na+ and K+ standard solutions. (B) The Na+-to-K+ ratio was calculated based on the Na+ and K+ content of the roots and the shoots of salt-treated Col-0 and nlp7 seedlings. Error bars represent the standard deviation of three independent replicates. Different letters (a, b, or c) within a treatment group indicate significant differences in the two-way ANOVA (P ≤ 0.05, Tukey’s test).
Proline typically accumulates in plants stressed by salt or osmoticum (Nanjo et al., 1999; Maiale et al., 2004; Sannazzaro et al., 2007), and MDA accumulates in response to lipid peroxidation resulting from salt stress (Wu et al., 2017; Zhou et al., 2017). Interestingly, the levels of proline and MDA were lower in nlp7 than in Col-0 following treatment with 0, 150, or 200 mM NaCl (Figures 8A,B). Moreover, the transcript levels of the stress marker genes, such as RD29A and COR47, were slightly lower in nlp7 or similar to those in Col-0 (Figure 8C).
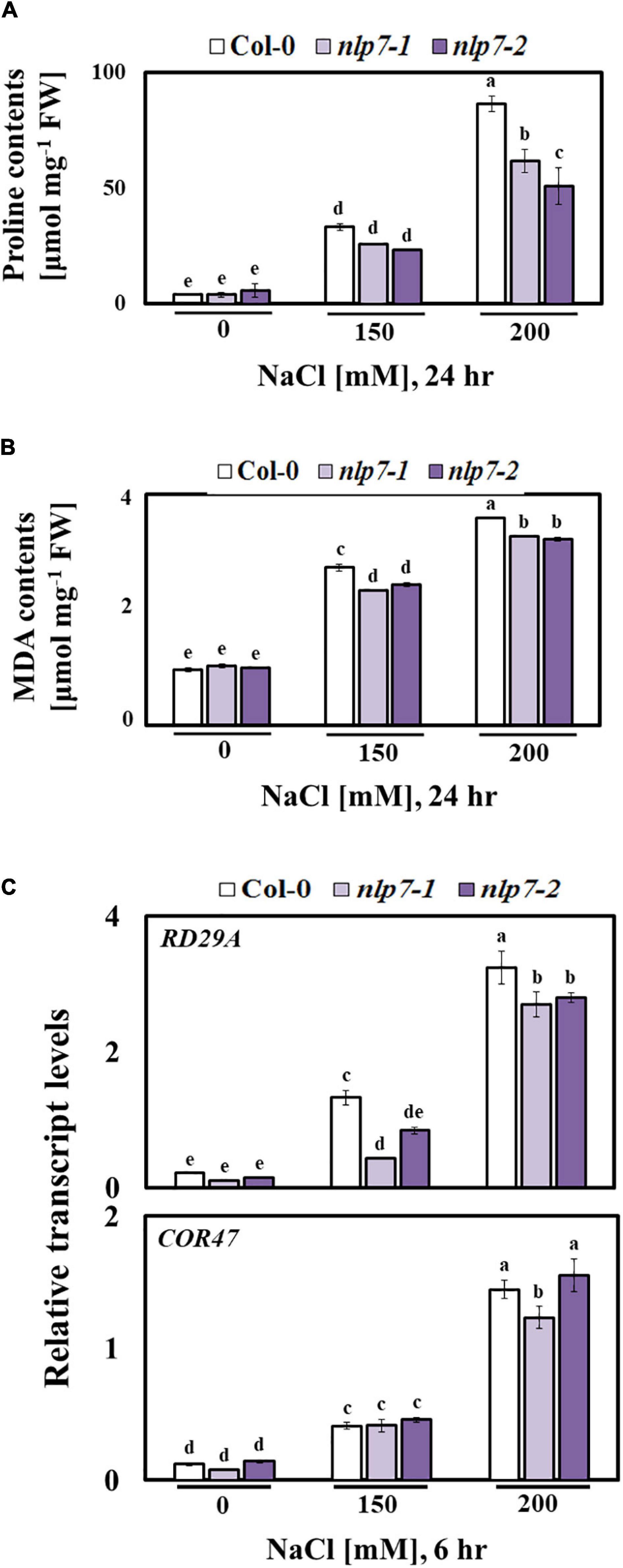
Figure 8. The nlp7 mutants showed reduced proline and malondialdehyde (MDA) content and decreased relative transcript levels of stress marker genes. (A, B) Proline and MDA content of 9-day-old Col-0 and nlp7 seedlings germinated and grown on a nitrogen-free half-strength MS medium containing 5 mM KNO3 and then treated with 0, 150, or 200 mM NaCl for 24 h. Error bars represent the standard deviation of three independent replicates. (C) Relative transcript levels of RD29A and COR47 (stress marker genes) in 9-day-old Col-0, nlp7-1, and nlp7-2 seedlings in response to salt stress, as determined using qPCR. The seeds were germinated and grown on a nitrogen-free half-strength MS medium containing 5 mM KNO3 for 9 days and then treated with 0, 150, or 200 mM NaCl for 6 h. AtActin2 was used as the internal control. Error bars represent the standard deviation of three independent replicates. Different letters (a, b, c, d, or e) within a treatment group indicate significant differences in the two-way ANOVA (P ≤ 0.05, Tukey’s test).
Yang et al. (2018) showed that HY5 is essential for tolerance to salt stress, so we assessed the expression of HY5. In the shoots, the transcripts of HY5 similarly accumulated in Col-0 and nlp7 under salt stress, while in the roots, the level of transcripts was lower in Col-0 than in nlp7 (Supplementary Figure 6). This finding suggests that a nitrate-dependent reduction in the accumulation of Na+ contributes to tolerance of nlp7to salt.
Under High Salinity, Abscisic Acid Accumulated Less in Col-0 Than in nlp7
Plant hormones play diverse roles in plants under stress. Among these, the signaling of ABA plays crucial roles in response to abiotic stress (Vishwakarma et al., 2017), and ionic and osmotic stress activates the ABA biosynthesis (Kim et al., 2010; Sah et al., 2016). To explore the involvement of NLP7 in salinity-triggering ABA biosynthesis, we measured the ABA content of Col-0 and nlp7 in response to added NaCl. Following treatment with 150 mM NaCl, nearly 30% less ABA accumulated in nlp7 than in Col-0, and this difference was two-fold greater following treatment with 200 mM NaCl (Figure 9A). Moreover, we examined the expression of genes involved in ABA biosynthesis or metabolism in 9-day-old seedlings of Col-0, nlp7-1, and nlp7-2 following exposure to 0, 150, or 200 mM NaCl for 6 h (Figure 9B). The transcript levels of 9-cisepoxycarotenoid dioxygenase (NCED3), encoding a key enzyme in ABA biosynthesis (Urano et al., 2009; Liu et al., 2016), was markedly reduced in nlp7; and, the transcript level of ß- GLUCOSIDASE 1 (BG1) and ß-GLUCOSIDASE 2 (BG2), involved in the conversion of inactive ABA-GE (located in the endoplasmic reticulum) to free active ABA (Xu et al., 2012), was reduced in nlp7 following treatment with 200 mM NaCl. These results suggest that nlp7 suffered less severe damage from high salinity because less ABA accumulated in comparison to Col-0 (Figure 9A). As shown in Supplementary Figure 7, any excess of ABA would be inappropriate for attaining maximal biomass. Thus, ABA content must be optimized for maximized salt tolerance (Supplementary Figures 7A,B).
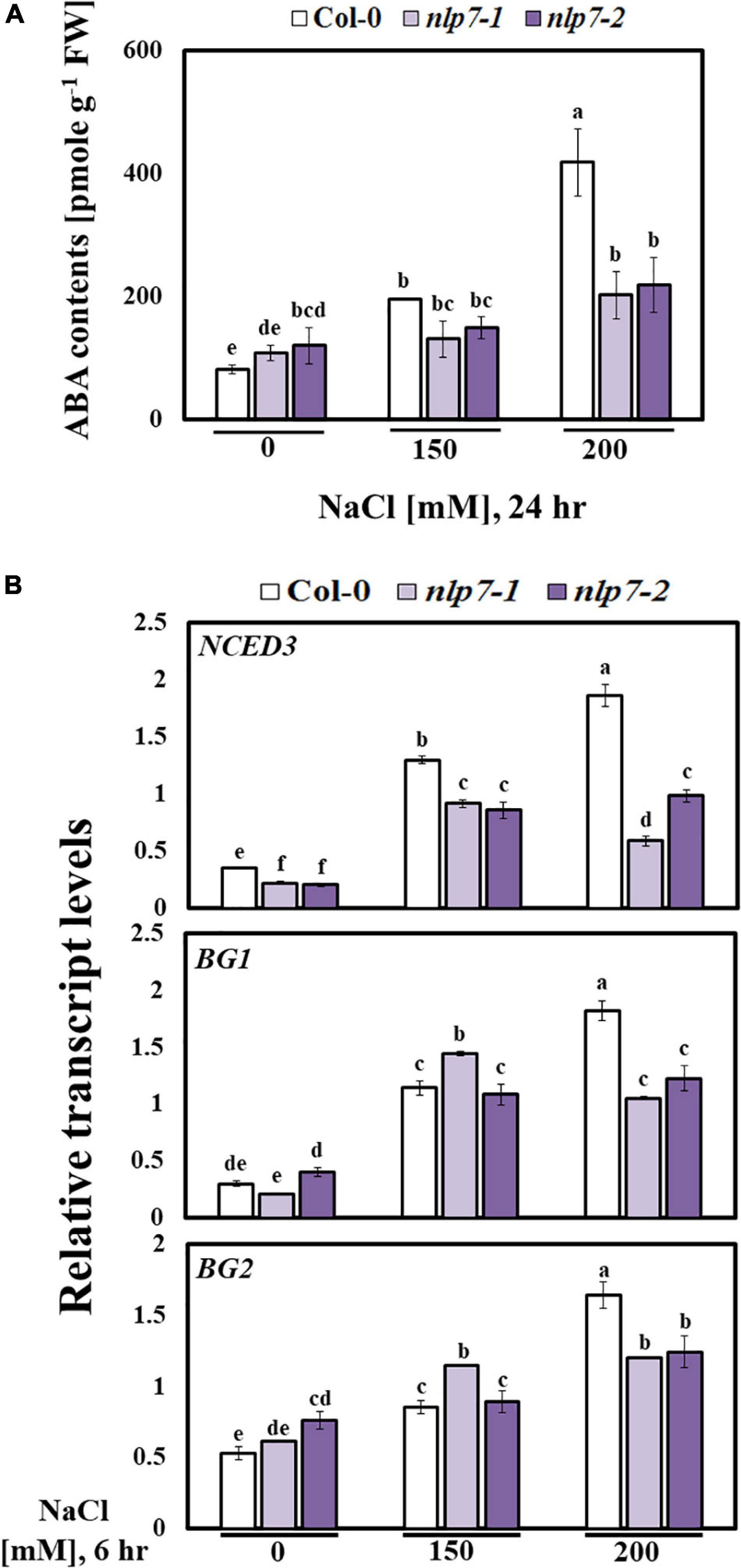
Figure 9. Abscisic acid accumulation was decreased in the nlp7 mutant under salt stress. (A) Comparison of the abscisic acid (ABA) content between Col-0 and nlp7 plants. Nine-day-old Col-0, nlp7-1, and nlp7-2 seedlings were germinated and grown on a nitrogen-free half-strength MS medium containing 5 mM KNO3 and then treated with 0, 150, or 200 mM NaCl for 24 h. Error bars represent the standard deviation of three independent replicates. (B) Comparison of the relative transcript levels of the ABA-related genes, such as NCED3, BG1, and BG2, in Col-0 and nlp7 plants. The seedlings were grown, treated, and analyzed as described in Figure 8C. AtActin2 was used as the internal control. Error bars represent the standard deviation of three independent replicates. Different letters (a, b, c, d, e, or f) within a treatment group indicate significant differences in the two-way ANOVA (P ≤ 0.05, Tukey’s test).
Discussion
The NLP7 is one of the extensively studied genes involved in nitrogen signaling. This gene belongs to the nodule inception (NIN) family (Castaings et al., 2009; Yu et al., 2016; Liu et al., 2017). NIN proteins harbor a 60-amino acid domain, called the RWPRK domain, which is similar to the DNA-binding domain of bZIP8 and bHLH/Z9 transcription factors (Schauser et al., 2005). Since NLP7 is a positive regulator of nitrogen signaling, we initially speculated that NLP7 could play a positive role under salt stress and could improve plant stress tolerance. However, the nlp7 plants not only weighed more than Col-0 when raised under high-salinity conditions, but they also showed higher content of chlorophyll and protein per unit weight, indicating that the loss of NLP7 appears to be in fact beneficial to the salt tolerance of Arabidopsis seedlings (Figure 1). A similar observation was also made by another research group (Castaings et al., 2009). Therefore, we further explored why the nlp7 plants are more resistant to salt stress than Col-0. The NLP7 seems to be associated with salt stress signaling because its transcript levels were apparently increased in response to salt stress (Figure 2). Moreover, the NLP7 promoter activity was increased not only by NaCl but also by KCl (Figure 3B).
Recently, NLP6 and NLP7, along with teosinte branched1/cycloidea/proliferating cell factor1-20 (TCP20), were shown to bind the promoter of the NIA1 gene under nitrogen starvation (Guan et al., 2017). Moreover, the NIT2 protein in Chlamydomonas reinhardtii, which is structurally related to the plant NIN proteins, activates NR by binding its promoter (Camargo et al., 2007). As expected, under salt stress, the activity of NR was lower in nlp7 plants than in Col-0 (Figure 4C), although nitrate uptake was equivalent between the two; thus, the reduced NR activity of nlp7 plants does not appear to be due to the decreased nitrate uptake under salt stress (Figure 4B). Contrary to these results, nitrate content was higher in nlp7 plants, perhaps due to the decreased NR activity (Figure 4C). Both the roots and the shoots of the nlp7 plants accumulated more nitrate than Col-0 (Figure 4A). These observations contradict the view that nitrate assimilation through NR action should be more active in the plants to tolerate stress conditions.
The NO is considered a key intracellular signaling molecule, which is involved in diverse developmental and physiological processes (Lamattina et al., 2003; Lamotte et al., 2005), particularly plant responses to abiotic stresses. Nevertheless, the functional roles of NO in salt stress responses remain elusive (Manai et al., 2014). There are two well-characterized enzymatic sources of NO in plants, namely NO synthase (NOS) and NR (Crawford, 2006). The primary function of NR encoded by NIA1 and NIA2 is nitrogen assimilation, in which nitrate is converted to nitrite through the activity of NR. Moreover, NR is the key enzyme for NO production in most plants (Tejada-Jimenez et al., 2019). The NR produces NO from nitrite in an NAD(P)H-dependent manner (Chen et al., 2016). This led us to measure the NO content of Col-0 and nlp7 plants, because at low levels, NO is also known to be a positive regulator of the stress response (Rockel et al., 2002; Del Rio et al., 2004). As shown in Figure 5, the NO content was lower in nlp7 than in Col-0. In plants, the various physiological functions of NO at the cellular level depend on the specific sites of action, where it is regulated and distributed, and its concentrations in the cell (Hasanuzzaman et al., 2018; Sánchez-Vicente et al., 2019). However, as several other salt stress responses (Figures 8, 9) were suppressed in nlp7 plants, we could not conclude that NO triggers the salt signaling cascade more effectively in this mutant than in Col-0. In fact, when produced abundantly, the NO can contribute to various ROS-induced cellular responses (Chen et al., 2014). The nlp7 plants likely produced less ROS, as evidenced by the lower NO accumulation in this mutant than in Col-0. Moreover, the nlp7 plants appeared to be less stressed than the Col-0 plants under high salinity, as evidenced by the lower levels of MDA (Figure 5A), an indicator of oxidative stress, in the mutant. MDA is a compound with high reactivity in the form of enol. It occurs naturally and is a reliable marker for oxidative stress (Del Rio et al., 2005). Furthermore, the lower proline content (Figure 5B) may be another evidence for the fact that the nlp7 plants were less stressed than the Col-0 plants. ABA is involved in plant stress response, and its content is increased under stress conditions (Cutler et al., 2010; Fujita et al., 2011; Sah et al., 2016). We observed that nlp7 plants accumulated less ABA than Col-0 under salt stress (Figure 9A). Moreover, the transcript levels of the ABA biosynthetic gene, NCED3 were decreased in the nlp7 plants. Likewise, the transcript levels of BG1 and BG2, which are involved in the conversion of inactive ABA to its active form (Xu et al., 2012; Ng et al., 2014), were reduced in the mutant (Figure 9B). Thus, the lower endogenous ABA levels in nlp7 plants again likely indicate that this mutant is less stressed than Col-0.
Upon plant exposure to salt stress, high external sodium concentrations lead to the rapid influx of Na+ ions into cells through various pathways (Keisham et al., 2018). Typically, the Na+ ions enter the cell via the K+ influx pathway, because the ionic radii of the hydrated form of Na+ and K+ are similar, making it difficult to discriminate between these two ions (Benito et al., 2014). Consequently, plants growing in highly saline soil often suffer from both Na+ toxicity and K+ deficiency (Hasegawa et al., 2000). In some halophytes, nitrates are transported to the shoots in a Na+-dependent manner (Junfeng et al., 2010; Nie et al., 2015). For instance, in Beta vulgaris, Na+ improved both nitrate uptake and its transport to the shoots (Kaburagi et al., 2014, 2015). Contrary to our expectation, Na+ content was much higher in the nlp7 plants than in Col-0 plants, particularly in the roots (Supplementary Figure 5). However, both Na+ and K+ levels were much higher in the nlp7 plants (Supplementary Figure 5). This finding implies that the nlp7 plants could accumulate more Na+ and K+ than Col-0 plants. A possible reason for this phenomenon is that the nlp7 plants accumulated more nitrates, which are anions, due to their reduced NR activity (Figure 4C), leading to the increased uptake of Na+, a cation, from the medium to balance the ionic charges. Under saline conditions, although Na+ influx may eventually lead to toxicity for Arabidopsis growth, these cations can also serve as an osmoticum (Alvarez-Aragon et al., 2016), although this aspect remains largely undervalued or omissive. Several studies have demonstrated that ionic solute uptake is one of the strategies of plants to adapt to low water potential in salinity environments (Alvarez-Aragon et al., 2016; Genc et al., 2016). For instance, in halophytes, high Na+ accumulation contributes to the adaptation to saline environments (Flowers and Colmer, 2015). In these plants, Na+ may be used as an osmolyte within the cell. Based on this, the nlp7 plants may be more tolerant of osmotic stress induced by high salinity. A similar phenomenon has been reported by Alvarez-Aragon and Rodriguez-Navarro (2017). The authors observed that in Arabidopsis, nitrates increased the absorption of Na+ and its loading into the xylem, resulting in high Na+ accumulation in the shoots. Under salt stress, Na+ serves an important osmotic function to prevent water loss and plant withering (Alvarez-Aragon and Rodriguez-Navarro, 2017), which supports our conclusion in the present study.
Nitrate assimilation is crucial for vascular plants. Moreover, NR activity is regulated by diverse, intricate mechanisms (for details, refer to the review by Lillo et al., 2004). NR is rapidly inactivated or activated through phosphorylation or dephosphorylation, respectively (MacKintosh and Meek, 2001). In addition, genes encoding NR are regulated at the posttranslational level to modulate enzyme activity in response to various external stimuli (Provan and Lillo, 1999; Lillo et al., 2003). The present study proposes that maintaining the optimal nitrate concentration through the modulation of NR activity may be an effective strategy to improve the salt tolerance of plants. As such, salt tolerance of plants can be enhanced through various mechanisms, and we cannot completely exclude the possibility that NLP7, which is rapidly induced under salt stress, acts as a transcriptional regulator of certain unknown genes related to the salt stress tolerance of Arabidopsis.
Data Availability Statement
The original contributions presented in the study are included in the article/Supplementary Material, further inquiries can be directed to the corresponding author.
Author Contributions
QL conducted nitrate reductase assay, nitrate content assay, 15N uptake assay, and proline content measurement and wrote the manuscript. WL conducted plant growth performance tests, chlorophyll assay, protein extraction and bradford assay, and statistical analyses and wrote the manuscript. JC performed MDA content measurements and DTN measured ABA contents. S-AL conducted quantitative RT-PCR. S-WH conducted statistical analyses. HT wrote the manuscript. HL designed the experiments and wrote the manuscript. All authors have reviewed and agreed to the content of the manuscript.
Funding
This work was supported by a grant from the National Research Foundation of Korea (to HL, 2019; grant number: NRF-2019R1A2C1088417).
Conflict of Interest
The authors declare that the research was conducted in the absence of any commercial or financial relationships that could be construed as a potential conflict of interest.
Publisher’s Note
All claims expressed in this article are solely those of the authors and do not necessarily represent those of their affiliated organizations, or those of the publisher, the editors and the reviewers. Any product that may be evaluated in this article, or claim that may be made by its manufacturer, is not guaranteed or endorsed by the publisher.
Supplementary Material
The Supplementary Material for this article can be found online at: https://www.frontiersin.org/articles/10.3389/fpls.2021.743832/full#supplementary-material
Abbreviations
ROS, reactive oxygen species; ABA, abscisic acid; NR, nitrate reductase; NO, nitric oxide; MS, Murashige and Skoog; MDA, malondialdehyde.
Footnotes
References
Acosta-Motos, J. R., Ortuño, M. F., Bernal-Vicente, A., Diaz-Vivancos, P., Sanchez-Blanco, M. J., and Hernandez, J. A. (2017). Plant responses to salt stress: adaptive mechanisms. Agronomy 7:18.
Alvarez-Aragon, R., Haro, R., Benito, B., and Rodriguez-Navarro, A. (2016). Salt intolerance in Arabidopsis: shoot and root sodium toxicity, and inhibition by sodium-plus-potassium overaccumulation. Planta 243, 97–114. doi: 10.1007/s00425-015-2400-7
Alvarez-Aragon, R., and Rodriguez-Navarro, A. (2017). Nitrate-dependent shoot sodium accumulation and osmotic functions of sodium in Arabidopsis under saline conditions. Plant J. 91, 208–219. doi: 10.1111/tpj.13556
Bates, L. S., Waldren, R. P., and Teare, I. D. (1973). Rapid determination of free proline for water-stress studies. Plant Soil 39, 205–207.
Benito, B., Haro, R., Amtmann, A., Cuin, T. A., and Dreyer, I. (2014). The twins K(+) and Na(+) in plants. J. Plant Physiol. 171, 723–731. doi: 10.1016/j.jplph.2013.10.014
Camargo, A., Llamas, A., Schnell, R. A., Higuera, J. J., González-Ballester, D., Lefebvre, P. A., et al. (2007). Nitrate signaling by the regulatory gene NIT2 in Chlamydomonas. Plant Cell 19, 3491–3503. doi: 10.1105/tpc.106.045922
Castaings, L., Camargo, A., Pocholle, D., Gaudon, V., Texier, Y., Boutet-Mercey, S., et al. (2009). The nodule inception-like protein 7 modulates nitrate sensing and metabolism in Arabidopsis. Plant J. 57, 426–435. doi: 10.1111/j.1365-313X.2008.03695.x
Chen, X., Tian, D., Kong, X., Chen, Q., E F, A. A., Hu, X., et al. (2016). The role of nitric oxide signalling in response to salt stress in Chlamydomonas reinhardtii. Planta 244, 651–669. doi: 10.1007/s00425-016-2528-0
Chen, Y., Mo, H. Z., Hu, L. B., Li, Y. Q., Chen, J., and Yang, L. F. (2014). The endogenous nitric oxide mediates selenium-induced phytotoxicity by promoting ROS generation in Brassica rapa. PLoS One 9:e110901. doi: 10.1371/journal.pone.0110901
Choi, W. G., and Gilroy, S. (2015). Quantification of sodium accumulation in Arabidopsis thaliana using inductively coupled plasma optical emission spectrometery (ICP-OES). Bio Protoc. 5:e1570. doi: 10.21769/BIOPROTOC.1570
Clough, S. J., and Bent, A. F. (1998). Floral dip: a simplified method for Agrobacterium-mediated transformation of Arabidopsis thaliana. Plant J. 16, 735–743. doi: 10.1046/j.1365-313x.1998.00343.x
Crawford, N. M. (2006). Mechanisms for nitric oxide synthesis in plants. J. Exp. Bot. 57, 471–478. doi: 10.1093/jxb/erj050
Cutler, S. R., Rodriguez, P. L., Finkelstein, R. R., and Abrams, S. R. (2010). Abscisic acid: emergence of a core signaling network. Annu. Rev. Plant Biol. 61, 651–679. doi: 10.1146/annurev-arplant-042809-112122
Del Rio, D., Stewart, A. J., and Pellegrini, N. (2005). A review of recent studies on malondialdehyde as toxic molecule and biological marker of oxidative stress. Nutr. Metab. Cardiovasc. Dis. 15, 316–328. doi: 10.1016/j.numecd.2005.05.003
Del Rio, L. A., Corpas, F. J., and Barroso, J. B. (2004). Nitric oxide and nitric oxide synthase activity in plants. Phytochemistry 65, 783–792.
Fan, X., Jia, L., Li, Y., Smith, S. J., Miller, A. J., and Shen, Q. (2007). Comparing nitrate storage and remobilization in two rice cultivars that differ in their nitrogen use efficiency. J. Exp. Bot. 58, 1729–1740. doi: 10.1093/jxb/erm033
Flowers, T. J., and Colmer, T. D. (2015). Plant salt tolerance: adaptations in halophytes. Ann. Bot. 115, 327–331. doi: 10.1093/aob/mcu267
Fujita, Y., Fujita, M., Shinozaki, K., and Yamaguchi-Shinozaki, K. (2011). ABAmediated transcriptional regulation in response to osmotic stress in plants. J. Plant Res. 124, 509–525. doi: 10.1007/s10265-011-0412-3
García-Mata, C., and Lamattina, L. (2001). Nitric oxide induces stomatal closure and enhances the adaptive plant responses against drought stress. Plant Physiol. 126, 1196–1204. doi: 10.1104/pp.126.3.1196
Garnett, T., Conn, V., and Kaiser, B. N. (2009). Root based approaches to improving nitrogen use efficiency in plants. Plant Cell Environ. 32, 1272–1283. doi: 10.1111/j.1365-3040.2009.02011.x
Genc, Y., Oldach, K., Taylor, J., and Lyons, G. H. (2016). Uncoupling of sodium and chloride to assist breeding for salinity in crops. New Phytol. 210, 145–156. doi: 10.1111/nph.13757
Goel, P., and Singh, A. K. (2015). Abiotic stresses downregulate key genes involved in nitrogen uptake and assimilation in Brassica juncea L. PLoS One 10:e0143645. doi: 10.1371/journal.pone.0143645
Good, A. G., and Beatty, P. H. (2011). “Biotechnological approaches to improving nitrogen use efficiency in plants: alanine aminotransferase as a case study,” in The Molecular and Physiological Basis of Nutrient Use Efficiency in Crops, eds M. J. Hawkesford and P. Barraclough (Hoboken: Wiley-Blackwell), 165–191.
Guan, P., Ripoll, J. J., Wang, R., Vuong, L., Bailey-Steinitz, L. J., Ye, D., et al. (2017). Interacting TCP and NLP transcription factors control plant responses to nitrate availability. Proc. Natl. Acad. Sci. U. S. A. 114, 2419–2424. doi: 10.1073/pnas.1615676114
Guo, F. Q., Okamoto, M., and Crawford, N. M. (2003). Identification of a plant nitric oxide synthase gene involved in hormonal signaling. Science 302, 100–103. doi: 10.1126/science.1086770
Han, Y. L., Song, H. X., Liao, Q., Yu, Y., Jian, S. F., Lepo, J. E., et al. (2016). Nitrogen use efficiency is mediated by vacuolar nitrate sequestration capacity in roots of Brassica napus. Plant Physiol. 170, 1684–1698. doi: 10.1104/PP.15.01377
Hasanuzzaman, M., Oku, H., Nahar, K., Bhuyan, M. H. M. B., Mahmud, J. A., Baluska, F., et al. (2018). Nitric oxide-induced salt stress tolerance in plants: ROS metabolism, signaling, and molecular interactions. Plant Biotechnol. Rep. 12, 77–92.
Hasegawa, P. M., Bressan, R. A., Zhu, J. K., and Bohnert, H. J. (2000). Plant cellular and molecular responses to high salinity. Annu. Rev. Plant Physiol. Plant. Mol. Biol. 51, 463–499. doi: 10.1146/annurev.arplant.51.1.463
Ho, C. H., Lin, S. H., Hu, H. C., and Tsay, Y. F. (2009). CHL1 functions as a nitrate sensor in plants. Cell 138, 1184–1194. doi: 10.1016/j.cell.2009.07.004
Huang, N. C., Chiang, C. S., Crawford, N. M., and Tsay, Y. F. (1996). CHL1 encodes a component of the low-affinity nitrate uptake system in Arabidopsis and shows cell type-specific expression in roots. Plant Cell 8, 2183–2191. doi: 10.1105/tpc.8.12.2183
Hunt, J. (1982). Dilute hydrochloric acid extraction of plant material for routine cation analysis. Commun. Soil Sci. Plant Anal. 13, 49–55.
Hussain, Q., Asim, M., Zhang, R., Khan, R., Farooq, S., and Wu, J. (2021). Transcription factors interact with ABA through gene expression and signaling pathways to mitigate drought and salinity stress. Biomolecules 11:1159. doi: 10.3390/biom11081159
Jefferson, R. A., Kavanagh, T. A., and Bevan, M. V. (1987). GUS fusions: β-glucuronidase as a sensitive and versatile gene fusion marker in higher plants. EMBO J. 6, 3901–3907.
Jeong, C. Y., Lee, W. J., Truong, H. A., Trịnh, C. S., Hong, S. W., and Lee, H. (2018). AtMybL-O modulates abscisic acid biosynthesis to optimize plant growth and ABA signaling in response to drought stress. Appl. Biol. Chem. 61, 473–477. doi: 10.1007/s13765-018-0376-2
Junfeng, Y., Changyan, T., and Gu, F. (2010). Effects of sodium on nitrate uptake and osmotic adjustment of Suaeda physophora. J. Arid Land 2, 190–196.
Kaburagi, E., Morikawa, Y., Yamada, M., and Fujiyama, H. (2014). Sodium enhances nitrate uptake in Swiss chard (Beta vulgaris var. cicla L.). Soil Sci. Plant Nutr. 60, 651–658.
Kaburagi, E., Yamada, M., and Fujiyama, H. (2015). Sodium, but not potassium, enhances root to leaf nitrate translocation in Swiss chard (Beta vulgaris var. cicla L.). Environ. Exp. Bot. 112, 27–32.
Keisham, M., Mukherjee, S., and Bhatla, S. C. (2018). Mechanisms of Sodium Transport in Plants - Progresses and Challenges. Int. J. Mol. Sci. 19:647. doi: 10.3390/ijms19030647
Kim, T.-H., Böhmer, M., Hu, H., Nishimura, N., and Schroeder, J. I. (2010). Guard cell signal transduction network: advances in understanding abscisic acid, CO2, and Ca2C Signaling. Annu. Rev. Plant Biol. 61, 561–591. doi: 10.1146/annurevarplant-042809-112226
Kolbert, Z., Ortega, L., and Erdei, L. (2010). Involvement of nitrate reductase (NR) in osmotic stress-induced NO generation of Arabidopsis thaliana L. roots. J. Plant Physiol. 167, 77–80. doi: 10.1016/j.jplph.2009.08.013
Konishi, M., and Yanagisawa, S. (2013). Arabidopsis NIN-like transcription factors have a central role in nitrate signalling. Nat. Commun. 4:1617. doi: 10.1038/ncomms2621
Konishi, M., and Yanagisawa, S. (2019). The role of protein-protein interactions mediated by the PB1 domain of NLP transcription factors in nitrate-inducible gene expression. BMC Plant Biol. 19:90. doi: 10.1186/s12870-019-1692-3
Lamattina, L., García-Mata, C., Graziano, M., and Pagnussat, G. (2003). Nitric oxide: the versatility of an extensive signal molecule. Annu. Rev. Plant Biol. 54, 109–136. doi: 10.1146/annurev.arplant.54.031902.134752
Lamotte, O., Courtois, C., Barnavon, L., Pugin, A., and Wendehenne, D. (2005). Nitric oxide in plants: the biosynthesis and cell signalling properties of a fascinating molecule. Planta 221, 1–4. doi: 10.1007/s00425-005-1494-8
Lee, Y. J., Lee, W. J., Le, Q. T., Hong, S.-W., and Lee, H. (2021). Growth performance can be increased under high nitrate and high salt stress through enhanced nitrate reductase activity in arabidopsis anthocyanin over-producing mutant plants. Front. Plant Sci. 12:644455. doi: 10.3389/fpls.2021.644455
Li, P. C., Huang, J. G., Yu, S. W., Li, Y. Y., Sun, P., Wu, C., et al. (2016). Arabidopsis YL1/BPG2 Is involved in seedling shoot response to salt stress through ABI4. Sci. Rep. 6:30163. doi: 10.1038/srep30163
Lillo, C., Lea, U. S., Leydecker, M. T., and Meyer, C. (2003). Mutation of the regulatory phosphorylation site of tobacco nitrate reductase results in constitutive activation of the enzyme in vivo and nitrite accumulation. Plant J. 35, 566–573. doi: 10.1046/j.1365-313x.2003.01828.x
Lillo, C., Meyer, C., Lea, U. S., Provan, F., and Oltedal, S. (2004). Mechanism and importance of post-translational regulation of nitrate reductase. J. Exp. Bot. 55, 1275–1282. doi: 10.1093/jxb/erh132
Lin, S. H., Kuo, H. F., Canivenc, G., Lin, C. S., Lepetit, M., Hsu, P. K., et al. (2008). Mutation of the Arabidopsis NRT1.5 nitrate transporter causes defective root-to-shoot nitrate transport. Plant Cell 20, 2514–2528. doi: 10.1105/tpc.108.060244
Liu, K. H., Huang, C. Y., and Tsay, Y. F. (1999). CHL1 is a dual-affinity nitrate transporter of Arabidopsis involved in multiple phases of nitrate uptake. Plant Cell 11, 865–874. doi: 10.1105/tpc.11.5.865
Liu, K. H., Niu, Y., Konishi, M., Wu, Y., Du, H., Sun Chung, H., et al. (2017). Discovery of nitrate-CPK-NLP signalling in central nutrient-growth networks. Nature 545, 311–316. doi: 10.1038/nature22077
Liu, N., Ding, Y., Fromm, M., and Avramova, Z. (2014). Endogenous ABA extraction and measurement from Arabidopsis leaves. Bio Protoc. 4:e1257. doi: 10.21769/bioprotoc.1257
Liu, S., Li, M., Su, L., Ge, K., Li, L., Li, X., et al. (2016). Negative feedback regulation of ABA biosynthesis in peanut (Arachis hypogaea): a transcription factor complex inhibits AhNCED1 expression during water stress. Sci. Rep. 6:37943. doi: 10.1038/srep37943
Ma, Y., Dias, M. C., and Freitas, H. (2020). Drought and salinity stress responses and microbe-induced tolerance in plants. Front. Plant Sci. 11:591911. doi: 10.3389/fpls.2020.591911
Machado, R. M. A., and Serralheiro, R. P. (2017). Soil salinity: effect on vegetable crop growth. management practices to prevent and mitigate soil salinization. Horticulturae 3:30.
MacKintosh, C., and Meek, S. E. M. (2001). Regulation of plant NR activity by reversible phosphorylation, 14-3-3 proteins and proteolysis. Cell. Mol. Life Sci. 58, 205–214. doi: 10.1007/PL00000848
Mahajan, S., and Tuteja, N. (2005). Cold, salinity and drought stresses: an overview. Arch. Biochem. Biophys. 444, 139–158. doi: 10.1016/j.abb.2005.10.018
Maiale, S., Sanchez, D. H., Guirado, A., Vidal, A., and Ruiz, O. A. (2004). Spermine accumulation under salt stress. J. Plant Physiol. 161, 35–42. doi: 10.1078/0176-1617-01167
Manai, J., Kalai, T., Gouia, H., and Corpas, F. J. (2014). Exogenous nitric oxide (NO) ameliorates salinity-induced oxidative stress in tomato (Solanum lycopersicum) plants. J. Soil Sci. Plant Nutr. 14, 433–446.
Marchive, C., Roudier, F., Castaings, L., Bréhaut, V., Blondet, E., Colot, V., et al. (2013). Nuclear retention of the transcription factor NLP7 orchestrates the early response to nitrate in plants. Nat. Commun. 4:1713. doi: 10.1038/ncomms2650
Meyer, C., Lea, U. S., Provan, F., Kaiser, W. M., and Lillo, C. (2005). Is nitrate reductase a major player in the plant NO (nitric oxide) game? Photosynth. Res. 83, 181–189. doi: 10.1007/S11120-004-3548-3
Munns, R. (2011). Plant adaptations to salt and water stress: differences and commonalities. Adv. Bot. Res. 57, 1–32.
Munns, R., James, R. A., Xu, B., Athman, A., Conn, S. J., Jordans, C., et al. (2012). Wheat grain yield on saline soils is improved by an ancestral Na+ transporter gene. Nat. Biotechnol. 30, 360–364.
Nabi, R. B. S., Tayade, R., Hussain, A., Kulkarni, K. P., Imran, Q. M., Mun, B. G., et al. (2019). Nitric oxide regulates plant responses to drought, salinity, and heavy metal stress. Environ. Exp. Bot. 161, 120–133.
Naeem, M. S., Jin, Z. L., Wan, G. L., Liu, D., Liu, H. B., Yoneyama, K., et al. (2010). 5-Aminolevulinic acid improves photosynthetic gas exchange capacity and ion uptake under salinity stress in oilseed rape (Brassica napus L.). Plant Soil 332, 405–415.
Nanjo, T., Kobayashi, M., Yoshiba, Y., Kakubari, Y., Yamaguchi-Shinozaki, K., and Shinozaki, K. (1999). Antisense suppression of proline degradation improves tolerance to freezing and salinity in Arabidopsis thaliana. FEBS Lett. 461, 205–210. doi: 10.1016/S0014-5793(99)01451-9
Navarro, A., Bañón, S., Conejero, W., and Sánchez-Blanco, M. J. (2008). Ornamental characters, ion accumulation and water status in Arbutus unedo seedlings irrigated with saline water and subsequent relief and transplanting. Environ. Exp. Bot. 62, 364–370. doi: 10.1016/j.envexpbot.2007.10.010
Ng, L. M., Melcher, K., Teh, B. T., and Xu, H. E. (2014). Abscisic acid perception and signaling: structural mechanisms and applications. Acta Pharmacol. Sin. 35, 567–584. doi: 10.1038/aps.2014.5
Nie, L., Feng, J., Fan, P., Chen, X., Guo, J., Lv, S., et al. (2015). Comparative proteomics of root plasma membrane proteins reveals the involvement of calcium signalling in NaCl-facilitated nitrate uptake in Salicornia europaea. J. Exp. Bot. 66, 4497–4510. doi: 10.1093/jxb/erv216
Paranychianakis, N. V., and Chartzoulakis, K. S. (2005). Irrigation of Mediterranean crops with saline water: from physiology to management practices. Agric. Ecosyst. Environ. 106, 171–187.
Provan, F., and Lillo, C. (1999). Photosynthetic post-translational activation of nitrate reductase. J. Plant Physiol. 154, 605–609.
Pruefer, A. (2001). Determination of Chloride by Flow Injection Colorimetry (Mercuric Thiocyanate Method). Lachat Instruments QuikChem Method 10-117-07-1-B. Milwaukee: Lachat Instruments.
Raddatz, N., Morales De Los Ríos, L., Lindahl, A. M., Quintero, F. J., and Pardo, J. M. (2020). Coordinated transport of nitrate, potassium and sodium. Front. Plant Sci. 11:247. doi: 10.3389/fpls.2020.00247
Rockel, P., Strube, F., Rockel, A., Wildt, J., and Kaiser, W. M. (2002). Regulation of nitric oxide (NO) production by plant nitrate reductase in vivo and in vitro. J. Exp. Bot. 53, 103–110.
Sah, S. K., Reddy, K. R., and Li, J. (2016). Abscisic acid and abiotic stress tolerance in crop plants. Front. Plant Sci. 7:571. doi: 10.3389/fpls.2016.00571
Sánchez-Vicente, I., Fernández-Espinosa, M. G., and Lorenzo, O. (2019). Nitric oxide molecular targets: reprogramming plant development upon stress. J. Exp. Bot. 70, 4441–4460. doi: 10.1093/jxb/erz339
Sannazzaro, A. I., Echeverria, M., Alberto’, E. O., Ruiz, O. A., and Menendez, A. B. (2007). Modulation of polyamine balance in Lotus glaber by salinity and arbuscular mycorrhiza. Plant Physiol. Biochem. 45, 39–46. doi: 10.1016/j.plaphy.2006.12.008
Schauser, L., Wieloch, W., and Stougaard, J. (2005). Evolution of NIN-like proteins in Arabidopsis, rice, and Lotus japonicus. J. Mol. Evol. 60, 229–237. doi: 10.1007/s00239-004-0144-2
Tejada-Jimenez, M., Llamas, A., Galván, A., and Fernández, E. (2019). Role of Nitrate Reductase in NO Production in Photosynthetic Eukaryotes. Plants (Basel) 8:56. doi: 10.3390/plants8030056
Urano, K., Maruyama, K., Ogata, Y., Morishita, Y., Takeda, M., Sakurai, N., et al. (2009). Characterization of the ABA regulated global responses to dehydration in Arabidopsis by metabolomics. Plant J. 57, 1065–1078. doi: 10.1111/j.1365-313X.2008.03748.x
Vishwakarma, K., Upadhyay, N., Kumar, N., Yadav, G., Singh, J., Mishra, R. K., et al. (2017). Abscisic acid signaling and abiotic stress tolerance in plants: a review on current knowledge and future prospects. Front. Plant Sci. 8:161. doi: 10.3389/fpls.2017.00161
Wang, R., Liu, D., and Crawford, N. M. (1998). The Arabidopsis CHL1 protein plays a major role in high-affinity nitrate uptake. Proc. Natl. Acad. Sci. U. S. A. 95, 15134–15139. doi: 10.1073/pnas.95.25.15134
Wang, Y. Y., Cheng, Y. H., Chen, K. E., and Tsay, Y. F. (2018). Nitrate transport, signaling, and use efficiency. Annu. Rev. Plant Biol. 69, 85–122. doi: 10.1146/annurev-arplant-042817-040056
Wu, W., Zhang, Q., Ervin, E. H., Yang, Z., and Zhang, X. (2017). Physiological mechanism of enhancing salt stress tolerance of perennial ryegrass by 24-epibrassinolide. Front. Plant Sci. 8:1017. doi: 10.3389/fpls.2017.01017
Xing, H., Tan, L., An, L., Zhao, Z., Wang, S., and Zhang, C. (2004). Evidence for the involvement of nitric oxide and reactive oxygen species in osmotic stress tolerance of wheat seedlings: inverse correlation between leaf abscisic acid accumulation and leaf water loss. Plant Growth Regul. 42, 61–68.
Xu, Z. Y., Lee, K. H., Dong, T., Jeong, J. C., Jin, J. B., Kanno, Y., et al. (2012). A vacuolar β-glucosidase homolog that possesses glucose-conjugated abscisic acid hydrolyzing activity plays an important role in osmotic stress responses in Arabidopsis. Plant Cell 24, 2184–2199. doi: 10.1105/tpc.112.095935
Yang, B., Song, Z., Li, C., Jiang, J., Zhou, Y., Wang, R., et al. (2018). RSM1, an Arabidopsis MYB protein, interacts with HY5/HYH to modulate seed germination and seedling development in response to abscisic acid and salinity. PLoS Genet. 14:e1007839. doi: 10.1371/journal.pgen.1007839
Yao, J., Shi, W. M., and Xu, W. F. (2008). Effects of salt stress on expression of nitrate transporter and assimilation-related genes in tomato roots. Russ. J. Plant Physiol. 55, 232–240. doi: 10.1134/S1021443708020106
Yu, L. H., Wu, J., Tang, H., Yuan, Y., Wang, S. M., Wang, Y. P., et al. (2016). Overexpression of Arabidopsis NLP7 improves plant growth under both nitrogen-limiting and -sufficient conditions by enhancing nitrogen and carbon assimilation. Sci. Rep. 6:27795. doi: 10.1038/srep27795
Zhang, H., Li, D., Zhou, Z., Zahoor, R., Chen, B., and Meng, Y. (2017). Soil water and salt affect cotton (Gossypium hirsutum L.) photosynthesis, yield and fiber quality in coastal saline soil. Agric. Water Manage. 187, 112–121.
Zhang, Q., Cai, W., Ji, T. T., Ye, L., Lu, Y. T., and Yuan, T. T. (2020). WRKY13 enhances cadmium tolerance by promoting D-CYSTEINE DESULFHYDRASE and hydrogen sulfide production. Plant Physiol. 183, 345–357. doi: 10.1104/pp.19.01504
Zhang, T. T., Kang, H., Fu, L. L., Sun, W. J., Gao, W. S., You, C. S., et al. (2021). NIN-like protein 7 promotes nitrate-mediated lateral root development by activating transcription of TRYPTOPHAN AMINOTRANSFERASE RELATED 2. Plant Sci. 303:110771. doi: 10.1016/j.plantsci.2020.110771
Zhao, C., Zhang, H., Song, C., Zhu, J. K., and Shabala, S. (2020). Mechanisms of plant responses and adaptation to soil salinity. Innovation (NY) 1:100017. doi: 10.1016/j.xinn.2020.100017
Zhao, L., and Wang, Y. (2017). Nitrate Assay for Plant Tissues. Bio Protocol. 7:e2029. doi: 10.21769/BioProtoc.2029
Zhao, L., Zhang, W., Yang, Y., Li, Z., Li, N., Qi, S., et al. (2018). The Arabidopsis NLP7 gene regulates nitrate signaling via NRT1.1–dependent pathway in the presence of ammonium. Sci. Rep. 8:1487. doi: 10.1038/s41598-018-20038-4
Keywords: Arabidopsis, NLP7, nitrate reductase, nitric oxide, salt stress tolerance
Citation: Le QT, Lee WJ, Choi JH, Nguyen DT, Truong HA, Lee S-A, Hong S-W and Lee H (2022) The Loss of Function of the NODULE INCEPTION-Like PROTEIN 7 Enhances Salt Stress Tolerance in Arabidopsis Seedlings. Front. Plant Sci. 12:743832. doi: 10.3389/fpls.2021.743832
Received: 19 July 2021; Accepted: 17 December 2021;
Published: 24 January 2022.
Edited by:
Paula Casati, Centro de Estudios Fotosintéticos y Bioquímicos (CEFOBI), ArgentinaReviewed by:
Noushina Iqbal, Jamia Hamdard University, IndiaAndrew Diener, Harvard Medical School, United States
Copyright © 2022 Le, Lee, Choi, Nguyen, Truong, Lee, Hong and Lee. This is an open-access article distributed under the terms of the Creative Commons Attribution License (CC BY). The use, distribution or reproduction in other forums is permitted, provided the original author(s) and the copyright owner(s) are credited and that the original publication in this journal is cited, in accordance with accepted academic practice. No use, distribution or reproduction is permitted which does not comply with these terms.
*Correspondence: Hojoung Lee, bGhvam91bmdAa29yZWEuYWMua3I=
†These authors have contributed equally to this work and share first authorship