- Key Laboratory of Agricultural Biotechnology, College of Life Science, Shihezi University, Shihezi, China
Fructose-1,6-biphosphate aldolase (FBA) is a multifunctional enzyme in plants, which participates in the process of Calvin-Benson cycle, glycolysis and gluconeogenesis. Despite the importance of FBA genes in regulating plant growth, development and abiotic stress responses, little is known about their roles in cotton. In the present study, we performed a genome-wide identification and characterization of FBAs in Gossypium hirsutum. Totally seventeen GhFBA genes were identified. According to the analysis of functional domain, phylogenetic relationship, and gene structure, GhFBA genes were classified into two subgroups. Furthermore, nine GhFBAs were predicted to be in chloroplast and eight were located in cytoplasm. Moreover, the promoter prediction showed a variety of abiotic stresses and phytohormone related cis-acting elements exist in the 2k up-stream region of GhFBA. And the evolutionary characteristics of cotton FBA genes were clearly presented by synteny analysis. Moreover, the results of transcriptome and qRT-PCR analysis showed that the expression of GhFBAs were related to the tissue distribution, and further analysis suggested that GhFBAs could respond to various abiotic stress and phytohormonal treatments. Overall, our systematic analysis of GhFBA genes would not only provide a basis for the understanding of the evolution of GhFBAs, but also found a foundation for the further function analysis of GhFBAs to improve cotton yield and environmental adaptability.
Introduction
It has been proved that photosynthesis is the physiological basis in crop yield formation, and the intensity of photosynthesis determines the level of crop production. Ribulose-1,5-bisphosphate carboxylase/oxygenase (Rubisco), as the catalyticase in the first step of Calvin-Benson cycle with low catalytic efficiency, was always thought to be the main rate-limiting mechanism of photosynthesis under normal growth conditions (Portis, 2003). However, according to the analysis of antisense transgenic plants with reduced rubisco activity, some researches suggested that rubisco is not the only factor that control photosynthesis rate (Hudson et al., 1992; Stitt and Hurry, 2002), the enzymes in ribulose-1,5-bisphosphate (RuBP) regeneration pathway have significantly higher control coefficients than Rubisco in photosynthetic carbon flow (Raines, 2003; Cai et al., 2018).
Fructose-1,6-bisphosphate aldolase (FBA; EC 4.1.2.13) constitute a vital part of RuBP regeneration, it reversibly catalyzes the synthesis of fructose-1,6-diphosphate (FBP) from glyceraldehyde-3-phosphate (G3P) and dihydroxyacetone phosphate (DHAP). Additionally, FBA also catalyzes the synthesis of sedoheptulose 1,7-bisphosphate (SBP) from DHAP and erythrose 4-phosphate (E4P) (Flechner et al., 1999). And evidences showed that FBA might play an important role in the control of carbon metabolism rate and the regeneration of RuBP in Calvin-Benson cycle (Haake et al., 1998).
FBAs could be broadly classified into two classes according to their catalytic mechanisms and evolutionary origin (Marsh and Lebherz, 1992; Nakahara et al., 2003). The catalytic activity of class-I FBAs are not inhibited by ethylene diamine tetraacetic acid (EDTA) or affected by potassium ions, and class-I FBAs are most found in bacteria, animals and plants. While the enzyme activity of class-II subgroup is affected by EDTA and the members usually occur in bacteria, yeast, fungi and some higher plants (Penhoet et al., 1967; Tolan et al., 1987; Henze et al., 1998). In higher plants, the FBA genes were located in cytosolic (cFBA) and chloroplast/plastid (cpFBA) (Lebherz and Rutter, 1969; Bukowiecki and Anderson, 1974). Both cFBAs and cpFBAs are nuclear-encoded genes and play vital roles in carbohydrate metabolism (Anderson et al., 1995).
Hitherto, a number of works have been done to analyze the characteristics of FBA gene family in several plant, including A. thaliana (AtFBA1-8) (Lu et al., 2012), O. sativa (OsFBA1-7), S. lycopersicum (SlFBA1-8) (Cai et al., 2016), B. napus (BnaFBA1-22) (Zhao et al., 2019) and T. aestivum (TaFBA1-21) (Lv et al., 2017). FBAs have been shown to be related to diverse physiological and biochemical processes in plants. Over-expressing AtFBA could improved plant growth in transgenic tobacco (Uematsu et al., 2012). While decreased the expression level of SlFBA7 would significantly reduce the biomass of transgenic tomato (Cai et al., 2018). Furthermore, FBA genes were also reported to be involved in signal transduction (Oelze et al., 2014; Zhang et al., 2014), secondary metabolism (Zeng et al., 2014) and resistance to abiotic stresses (Michelis and Gepstein, 2000; Yamada et al., 2000; Oztur et al., 2002; Larkindale and Vierling, 2008; Purev et al., 2008; Xue et al., 2008; Fan et al., 2009; Lu et al., 2012). Moreover, the fact that FBAs appear in the nucleus indicated FBA genes might function as transcriptors in regulating gene expression directly (Páez-Valencia et al., 2008). All these results indicated that FBA genes hold tremendous potentials for genetic engineering to improve the crop yield and stress resistance.
Cotton is an important economic crops that provide textile and oil materials worldwide (Huang et al., 2015). There are more than 50 species in the Gossypium genus (Wendel and Cronn, 2003), including six tetraploid (2n = 4×) species and 46 diploid (2n = 2×) species. Due to its high yield and high-quality fiber, G. hirsutum is the most widely cultivated among all cotton species. However, some abiotic stresses, including temperature, drought and salt, are all restrictions on cotton growth, fiber quality and yield (Yang et al., 2012; Gu et al., 2018; Li, 2018; Sun, 2019). As mentioned before, FBA genes play key roles in photosynthetic carbon flow and stress resistance, but only a few researches about FBA in cotton have been reported up to now (Xu et al., 2013). The whole-genome sequencing of G. raimondii, G. arboreum and G. hirsutum provided an opportunity to have a novel insights into the FBA family at genome-wide level (Paterson et al., 2012; Li et al., 2014; Yang et al., 2019).
In present study, we systematically identified 17 putative FBA genes in G.hirsutum, then the phylogenetic relationships, gene characteristics, structures, and chromosomal distribution of the identified FBA genes were further analyzed in detail. Additionally, the expression of FBAs in different tissues and in response to different stresses and different phytohormones were further analyzed. This research would supply a valuable reference for function analysis of FBA genes in cotton and other species.
Materials and Methods
Identification of Cotton FBA Sequences
The genome sequences and annotation files of G. arboreum (Du et al., 2018), G. raimondii (Paterson et al., 2012), and G. hirsutum (Yang et al., 2019) were downloaded from CottonFGD.1 To identify the FBA in three cotton species, the Hidden Markov Model files corresponding to the Glycolytic domain (PF00274) and fructose-bisphosphate aldolase class-II domain (PF01116) were downloaded from Pfam protein family database.2 Then the FBA genes were extracted from the three cotton genome database by using HMMER (version 3.0) with default parameters, the isoforms were removed manually. For the further confirmation of FBA members, all the candidate FBAs genes were submitted to the CDD database and SMART database3 for the further examined.
Sequence Analysis
The ExPASy website4 was used to compute the length of sequences, molecular weights (MW) and isoelectric point (pI) of cotton FBA genes. The coding sequences (CDS) and the corresponding full-length DNA sequences were used to predict the structures of FBA genes through the online bioinformatics tool Gene Structure Display Server (GSDS5). And the subcellular localization of cotton FBA genes were predicted by WoLF PSORT.6 Then the MEME website7 was used to identify the conserved motifs of cotton FBA genes. To obtain the cis-acting elements information in GhFBA promoters, 2-kb upstream sequences of each cotton FBA genes were intercepted and submitted to PlantCARE database for further analysis.
Multiple Alignments and Phylogenetic Analysis
ClustaW was used to align the putative GhFBA amino acid sequences with default parameters, and then MEGA7.0 (Tamura et al., 2013) was employed to construct phylogenetic trees using Neighbor-Joining (NJ) method with 1000 bootstrap replications. The information of FBA proteins from Arabidopsis, tomato (Cai, 2017), wheat and rice were obtained according to the description of related reporters and the sequences were downloaded from TAIR and NCBI database.
Chromosome Localization and Gene Duplication Analysis
The physical location information of GhFBA genes in the chromosomes were obtained from the genome annotation files of three species. And the duplication events within the subgenome were detected by Multiple Collinearity Scan toolkit (MCScanX) (Wang et al., 2012) with default settings. Then the TBtools8 was used to exhibit the chromosomes distribution and the synteny relationship of orthologous FBA genes that identified from G. raimondii, G. arboreum, and G. hirsutum. Furthermore, KaKs Calculator 2.0 was employed to estimate the non-synonymous (ka) and synonymous (ks) substitution rates of the duplicated cotton FBA genes.
Expression Analysis of GhFBA Genes
The public available transcriptome data of G. hirsutum in different tissues and in respond to different stress were downloaded from CottonFGD database (BioProject ID: PRJNA49062) (Hu et al., 2019). And Transcripts Per Kilobase of exon model per Million mapped reads (TPM) was used to quantify gene expression. Then the TPM values were perform treatment of log2(TPM + 1) to construct the heatmap by TBtools.
Expression Profile Analysis of GhFBAs in Response to Distinct Treatments
To validate the accuracy and authenticity of the transcriptome data and have a further understanding of the expression patterns of GhFBA genes in response to different phytohormonal treatments, eight GhFBA genes were selected for further qRT-PCR analysis. Full shape and disease-free seeds were selected and planted in pots, the greenhouse conditions were set at 28°C with 16 h light/8 h dark cycle. After 4 weeks cultivation, plants at three-leafs were selected for different treatments. The plants were treated with ABA (200 μM), ethylene (400 μM), MeJA (2 mM), SA (2 mM), 4°C, Nacl (200 mM) and PEG (20% w/v) for 1, 3, 6, 12, and 24 h, all selected samples were quickly frozen in liquid nitrogen and preserved at −80°C for subsequent analysis.
Total RNA of the samples was extracted using Plant RNA miniprep kit (Polysaccharides & Polyphenolics-rich, Biomiga, United States, R8611). Then the RevertAid First Strand cDNA Synthesis Kit (Thermo, United States, K1662) was used to synthesize the first strand of cDNA from the high quality DNA-free RNA. qRT-PCR was performed by using Roche LightCycler® 480 instrument with SYBR Green (Transgen, China, AQ601). The qRT-PCR reaction conditions were as follows: 95°C for 5 min; 40 cycles of 95°C/15 s, 60°C/20 s, 72°C/30 s. Every treatments contained three three biological replications, and each analysis was repeated by three times. The date from qRT-PCR was analyzed using 2–△△Ct method and the expression levels were represented by the mean values of the three replicates. Student’s t test was carried out to determine whether the changes in gene expression were significant. And sequences of the primers used in this study were shown in detail in Supplementary Table 1.
Subcellular Location of GhFBAs
To verify the results of subcellular localization predication, the coding open reading frame (ORF) sequences without terminate codon of 7 GhFBA genes were cloned into pCambia1300-eGFP vector. The recombinant vectors pCambia1300-GhFBAs-eGFP and the empty vector pCambia1300-eGFP were transformed into Agrobacterium tumefaciens GV3101, then the Agrobacterium tumefaciens contained vectors were injected into three- to four-week old Nicotiana benthamiana leaves. After 2 days cultured in dark environment, the GFP signals were monitored using a laser scanning microscope (NIKON, C2+, Japan).
Pearson Correlation Analysis
Based on the transcriptome data, the Pearson correlation coefficients (PCCs) and p-value of the expression levels of GhFBA gene pairs were calculated by SPSS (Version 26.0). The heatmap of correlations was generated by Tbtools. And the co-regulatory networks were constructed by Cytoscape (version 3.8.2) based on the PCCs of GhFBAs genes pairs with p-value <0.05.
Results
Identification of FBA Genes in Cotton
Totally 17 putative FBA gene sequences were obtained G. hirsutum genome dataset (Table 1), furthermore, 9 GaFBAs amd 9 GrFBAs were obtained from G. arboreum and G. raimondii with the same methods (Supplementary Table 2). Based on the sequences information, gene characteristics, including the length of CDS and protein sequences, the MW and pI of FBA proteins, and the potential subcellular localization were all analyzed. As results, the length of deduced proteins of all FBAs ranged from 177 (GhFBA2) amino acid (aa) to 1373 (GhFBA3) aa. The highest MW was 147.87 kDa of GhFBA3 protein and the lowest one was 19.57kDa of GhFBA2. The grand average of hydropathy (GRAVY) ranged from −0.244 (GhFBA6) to 0.096 (GhFBA11). The result of subcellular localization prediction showed that nine GhFBAs proteins (GhFBA2/3/6/7/8/11/14/15/17) were predicted to be located in chloroplast, and the other 8 GhFBAs proteins (GhFBA1/4/5/9/10/12/13/16) were located in cytoplasm (Table 1). Furthermore, 5 GaFBAs and 5 GrFBAs were predicted to be located in chloroplast, four GaFBAs and four GrFBAs were predicted to be cytoplasm-localized, respectively (Supplementary Table 2). To verify the predicted results of subcellular location, 7 GhFBA genes were selected and fused with eGFP protein to assay their presence in tobacco leaf cell. As shown in Figure 1, GhFBA2/6/7/8 were located in chloroplast, and GhFBA 4/5/9 were located in cytoplasm. This result was consistent with the prediction.
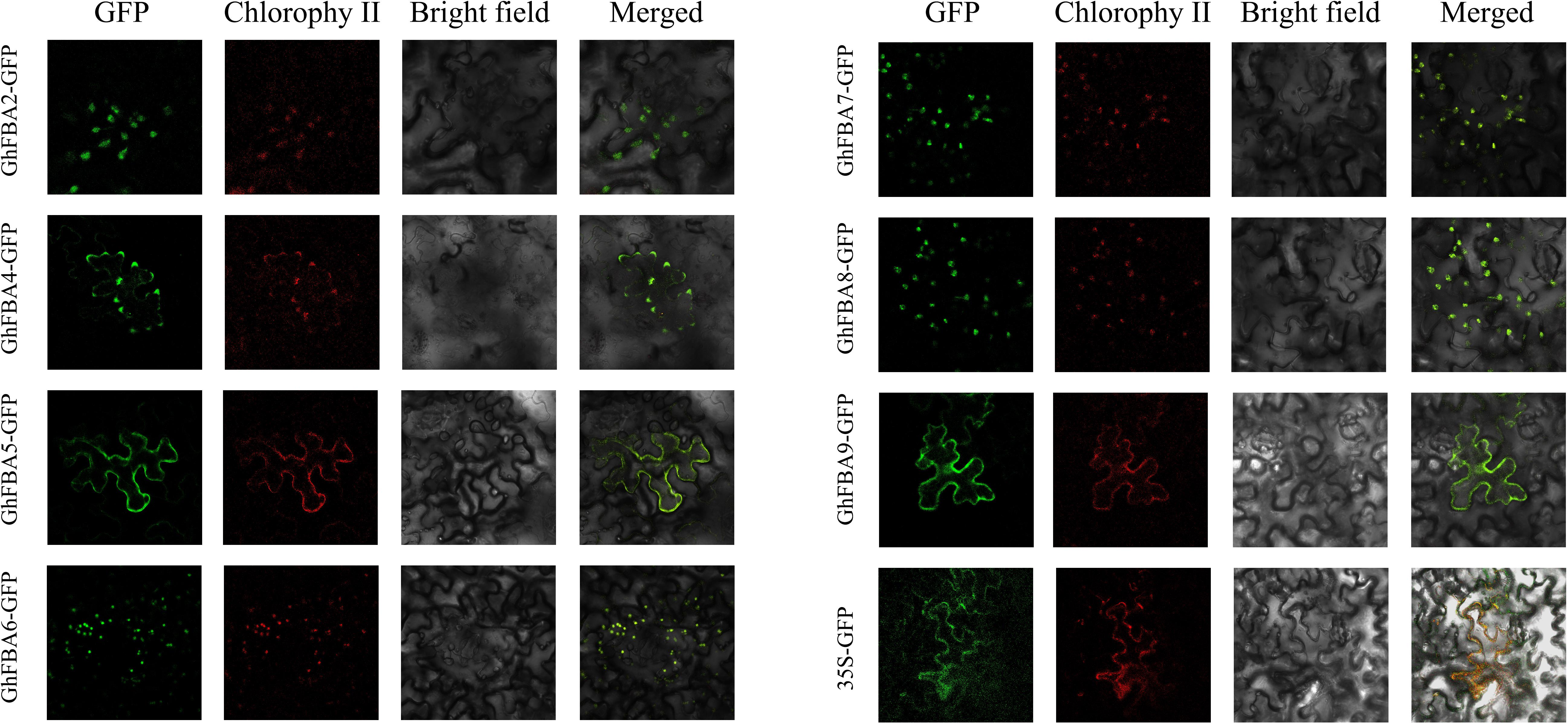
Figure 1. Subcellular location of GhFBAs in tobacco epidermal cells. GhFBAs-eGFP and empty control vector (35S-eGFP) were transiently expressed in tobacco epidermal cells. And the fluorescent signal was collected by confocal microscope.
Phylogenetic Analysis of GhFBA Gene Family
To investigate the molecular phylogenetic relationships of the members in GhFBA gene family, totally 80 FBA genes from G. hirsutum (Gh), G. arboreum (Ga), G. raimondii (Gr), A. thaliana (At), S. lycopersicum (Sl), T. aestivum (Ta) and O. sativa (Os) were extracted to construct an unrooted phylogenetic tree. Based on the phylogenetic relationships of the selected genes, all the tested FBAs could be broadly classified into two major classes (Figure 2). Moreover, the class-I group was further divided into five subclasses (ClassIa-e). Among 17 GhFBA proteins, 2 belong to class-Ia, 5 belong to class-Ib, 2 belong to class-Ic, 6 belong to class-Ie and 2 belong to class-II. Furthermore, the absence of dicotyledonous FBA genes in group class-Id (Figure 2) indicated the evolutionary differences between monocotyledons and dicotyledons.
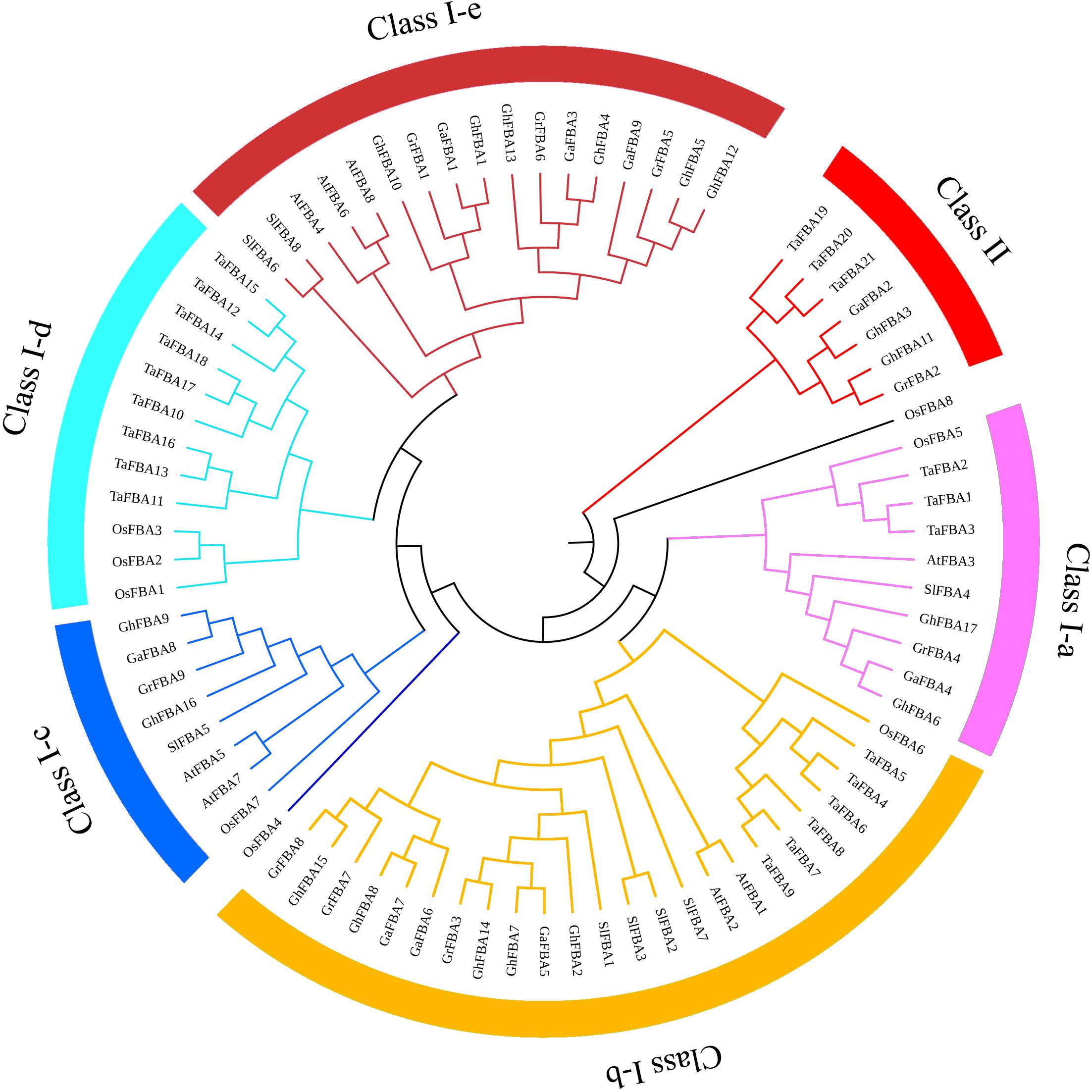
Figure 2. An unrooted phylogenetic tree representing relationships among FBA proteins from G. hirsutum (Gh), G. arboreum (Ga), G. raimondii (Gr), A. thaliana (At), T. aestivum (Ta), O. sativa (Os) and S. lycopersicum (Sl). The different colors arcs indicate different classes or subclasses. Clustal W was used to align the sequences, MEGA7.0 was used to construct phylogenetic trees with neighbor-joining method.
Exon/Intron Organization and Motif Composition Analysis of Cotton FBA Genes
In order to provide enough proof for the phylogenetic analysis, we carried out a comparison of the predicted CDS of all identified FBA genes in G. hirsutum, G. arboreum and G. raimondii. As shown in Figure 3B, the distribution of exons and introns of cotton FBA genes was variable, the number of exons and the length of sequences were widely ranged between different subclasses. The number of exons ranged from one to six in class-I, and GhFBA13 was the gene with only one exon, the genes in class-II all have 42 exons. Moreover, the members in class-Ia and class-Ib have more exons than the members in class-Ic and class-Ie. Further analysis showed that the members within the same subclasses usually shared similar structures, for example, except for GhFBA2, other genes in class-Ib subclass all contained five introns and six exons. Additionally, the FBA genes in G. hirsutum exhibited the same gene structures with its diploid parent.
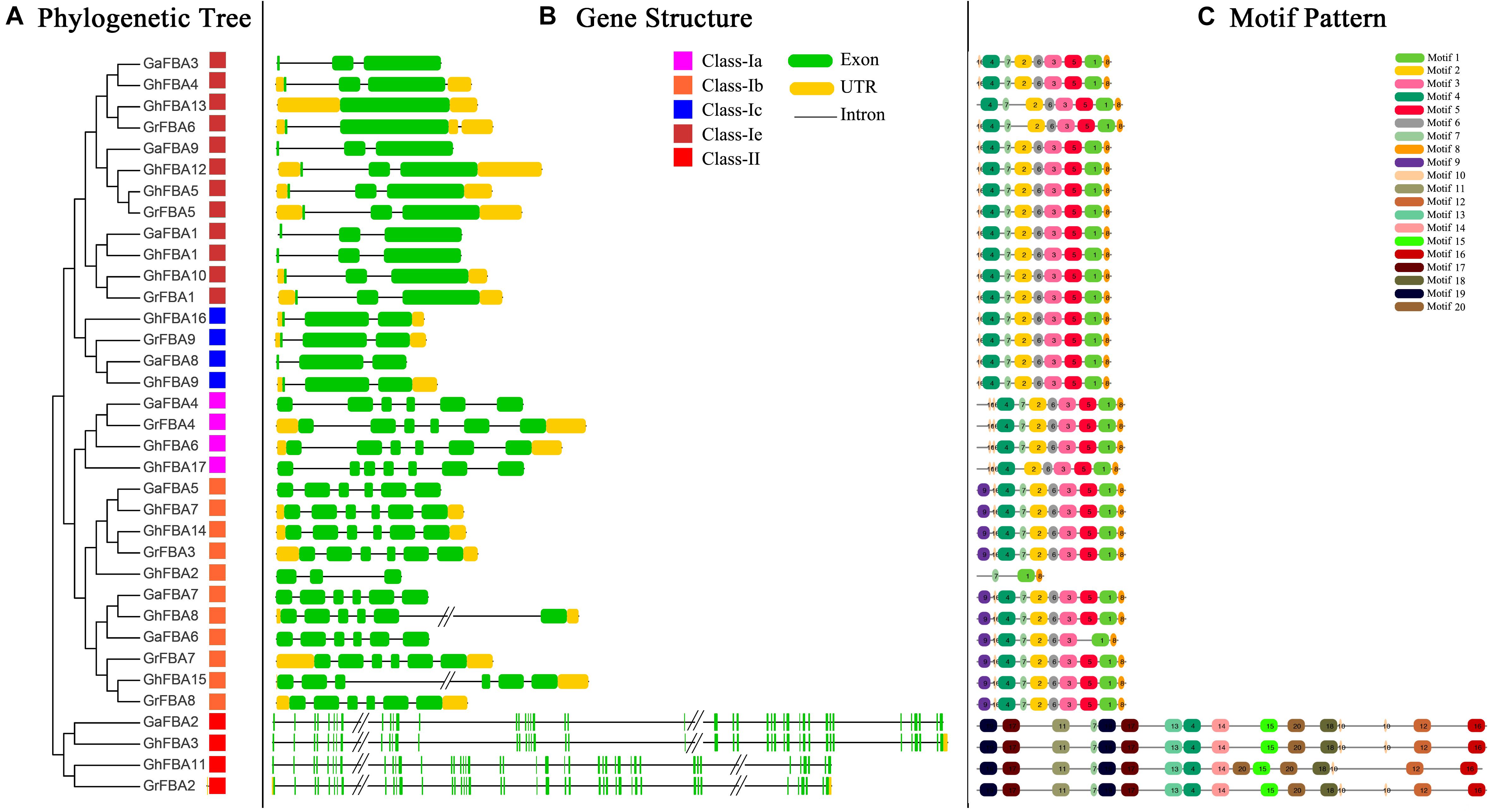
Figure 3. Phylogenetic relationships, gene structure and architecture of conserved protein motifs in FBA genes from G. hirsutum (Gh), G. arboreum (Ga) and G. raimondii (Gr). (A) The phylogenetic tree of all identified cotton FBA genes. Full-length protein sequences of FBA genes were used to generate the phylogenetic tree, and the different color squares represent phylogenetic subclasses. (B) Gene structure features of cotton FBA genes. Green boxes indicate exons, black lines indicate introns and yellow boxes indicate 3′ and 5′ untranslated regions. (C) The motif architecture of cotton FBA proteins.
Besides the study of gene structure features, predicted amino acid sequences of cotton FBA proteins were submitted to the MEME website for architecture analysis. As shown in Figure 3C, the differences of motifs between class-I and class-II were significant. Motif 2, motif 3, motif 5 and motif 8 were unique to class-I, while motif 15 motif 16 and motif 19 were specific to class-II. Except for the special motifs, some motifs were widely distributed in class-I and class-II, such as motif 7, motif 10 and motif 4. Additionally, within the same subclasses, the types and distribution of motifs shared high similarities, indicating that the protein architecture of cotton FBA genes was highly conserved within a specific subclass. According to the previous studies, FBA genes were related to diverse physiological and biochemical processes in plants, so further and deeper researches are needed to be carried out to expound the function of these conserved motifs.
Cis-Acting Elements Analysis in the Putative Promoter Regions of GhFBA Genes
The cis-acting elements within promoter regions of GhFBA genes were analyzed in this study (Supplementary Table 3). As shown in Figure 4, various cis-acting regulatory elements were detected in the promoter regions of GhFBA genes. Phytohormone responsive elements, such as MeJARE (MeJA-responsive element), ABRE (abscisic acid-responsive element), SARE (salicylic acid-responsive element) were included in the promoter regions (Figure 4), suggesting that the expression of GhFBA genes might be regulated by multiple phytohormones. Additionally, some stress-related cis-acting elements, like DSRE (drought and stress-responsive element), LTRE (low-temperature-responsive element) and HSRE (heat stress-responsive element) were also found in the GhFBA gene promoter regions (Table 2), these results indicated that GhFBA genes might be closely related to the responses to multiple abiotic stresses. Moreover, two phytohormone-related elements (ABRE, MeJARE) and a stress-responsive element (HSRE) were frequently detected in the putative promoters of GhFBA genes. Notably, each GhFBAs contain multiple copies of LRE (light-responsive element), suggesting that GhFBA genes was an important component of light response in G. hirsutum.
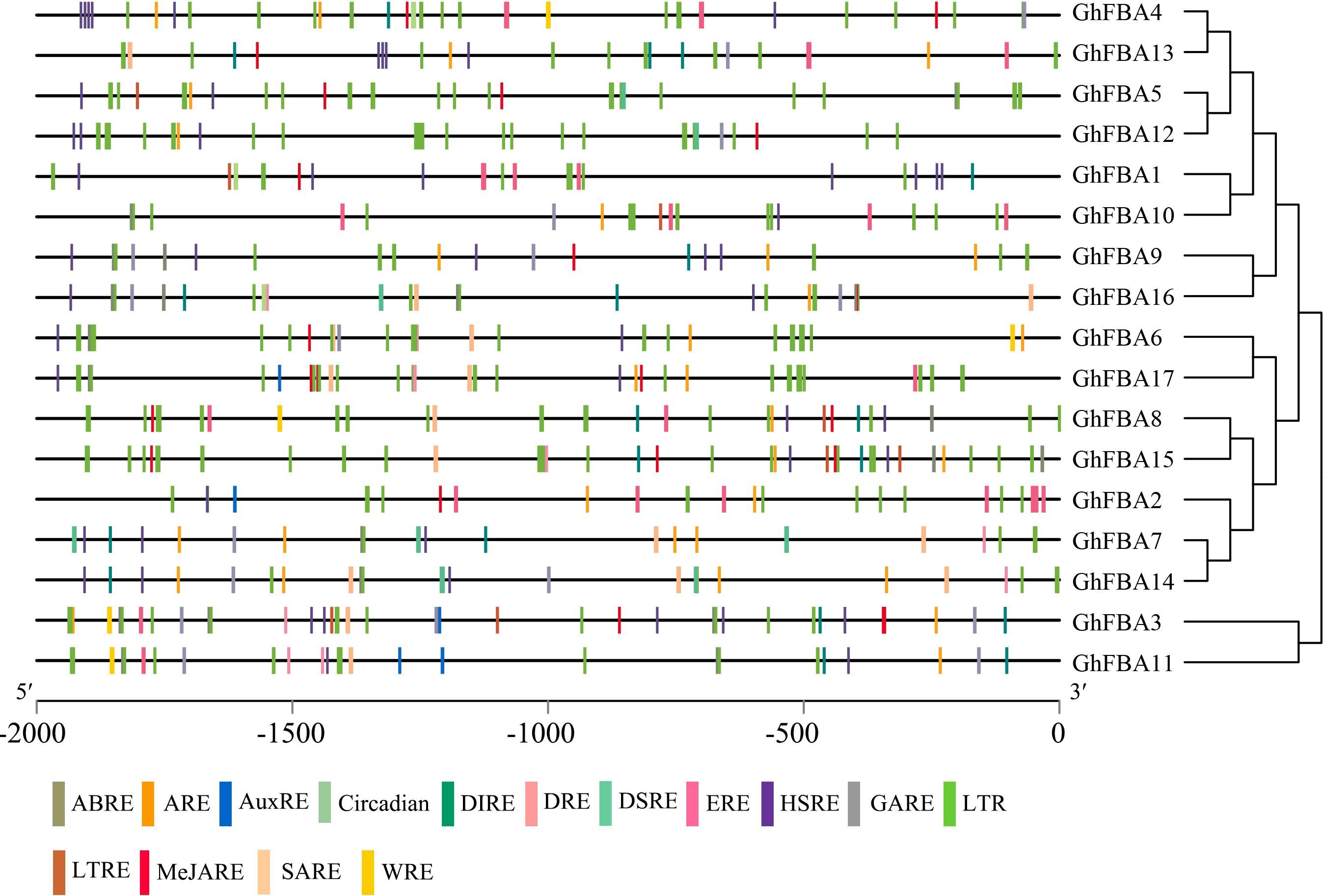
Figure 4. Analysis of the responsive cis-acting elements in GhFBA genes promoter regions. The 2-kb sequences of GhFBA gene promoter regions were extracted and analyzed, and different cis-acting elements were color-coded in specific colors.
Chromosomal Distribution and Synteny Analysis of GhFBA Genes
In order to have a further investigation into the evolution of GhFBA genes, the gene distribution in chromosomes and duplication events were analyzed. As shown in Figure 5, GhFBA1-16 were distributed in 12 chromosomes, and GhFBA17 was located on an unattributed contig (UN2). The number of GhFBAs in each chromosome ranged from 1 to 2, chromosome AD04, AD13, AD17 and AD26 contained two GhFBA genes. Additionally, 9 GhFBA genes were distributed over seven A subgenome chromosomes of G. hirsutum and 7 genes were located on five D subgenome chromosomes. Besides, no evidence showed that there was a positive correlation between the gene number and the length of chromosomes.
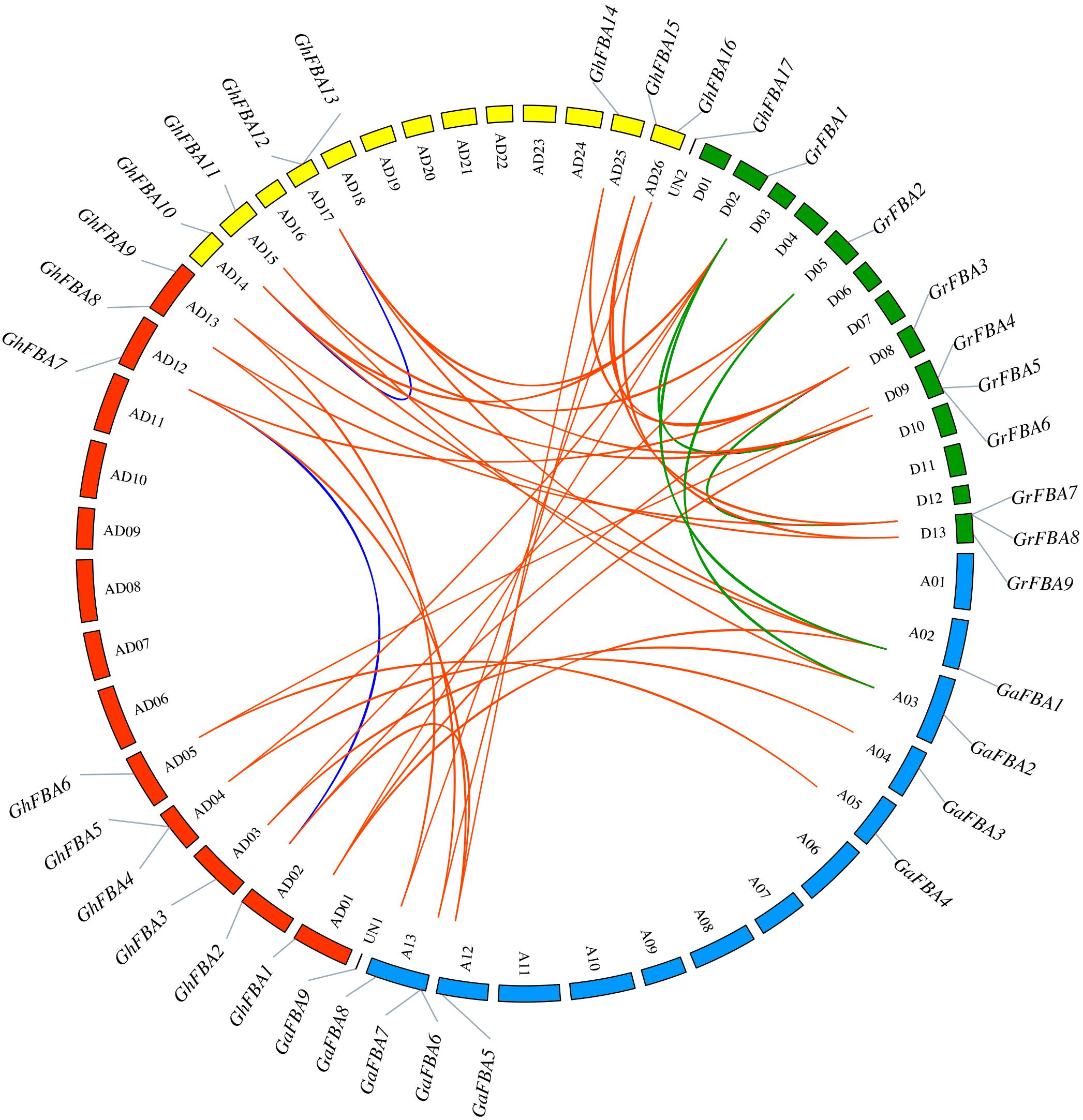
Figure 5. Chromosomal distribution and collinear correlations of FBA members of G. hirsutum (Gh), G. arboreum (Ga) and G. raimondii (Gr). The chromosome number is indicated by the alphanumeric codes within the circle, UN1 and UN2 represent the scaffold that contain FBA genes in G. arboreum and G. hirsutum, respectively. The blue lines indicate the duplicated FBA gene pairs within A subgenome and D subgenome of G. hirsutum, the orange lines represent the syntenic FBA gene pairs between G. hirsutum and other species, the green lines represent the syntenic FBA gene pairs between G. arboreum and G. raimondii.
In the A subgenome of G. hirsutum, one segmental duplication event with two genes GhFBA2/GhFBA7 was identified, and in the D subgenome a segmental duplication event and a tandem duplication event were identified (Supplementary Table 4). Moreover, for the sake of a better understanding of the evolutionary mechanism of GhFBA gene family, syntenic gene analysis was constructed among G. hirsutum, G. arboreum and G. raimondii. As results, 13 orthologous gene pairs were found between G. hirsutum and G. arboreum, 16 gene pairs between G. hirsutum and G. raimondii, 3 gene pairs between G. arboreum and G. raimondii (Figure 5). Additionally, the Ka/Ks ratios of all segmental and tandem duplicated GhFBA gene pairs, and the orthologous FBA gene pairs were lower than 1 (Supplementary Table 5), suggesting that the purifying selection may play an essential role during the GhFBA gene family evolution.
Expression Profiles of GhFBA Genes in Different Tissues
To have a further understanding of the functional roles of GhFBA members, the expression profiles of GhFBA genes in different tissues were analyzed (Figure 6A and Supplementary Table 6). And qRT-PCR experiments were carried out to validate the accuracy and authenticity of the transcriptome data on four representative samples of eight GhFBA genes (Figure 6B and Supplementary Table 9). As results, eight genes were commonly (TPM > 1 in all samples) detected in all tissues and four were highly (TPM > 30) expressed. Additionally, some GhFBA genes showed very low expression levels in all tissues, such as GhFBA1, GhFBA2 and GhFBA10. Besides, some genes displayed significant tissue-specific expression patterns, such as GhFBA7 and GhFBA14 were highly expressed in stem, leaf and bract, but GhFBA8 and GhFBA15 were highly expressed in stem, leaf, bract, pistil, sepal and torus. In addition, two members of class-II, GhFBA3 and GhFBA11, exhibited low but constitutive expression patterns across the detected tissues.
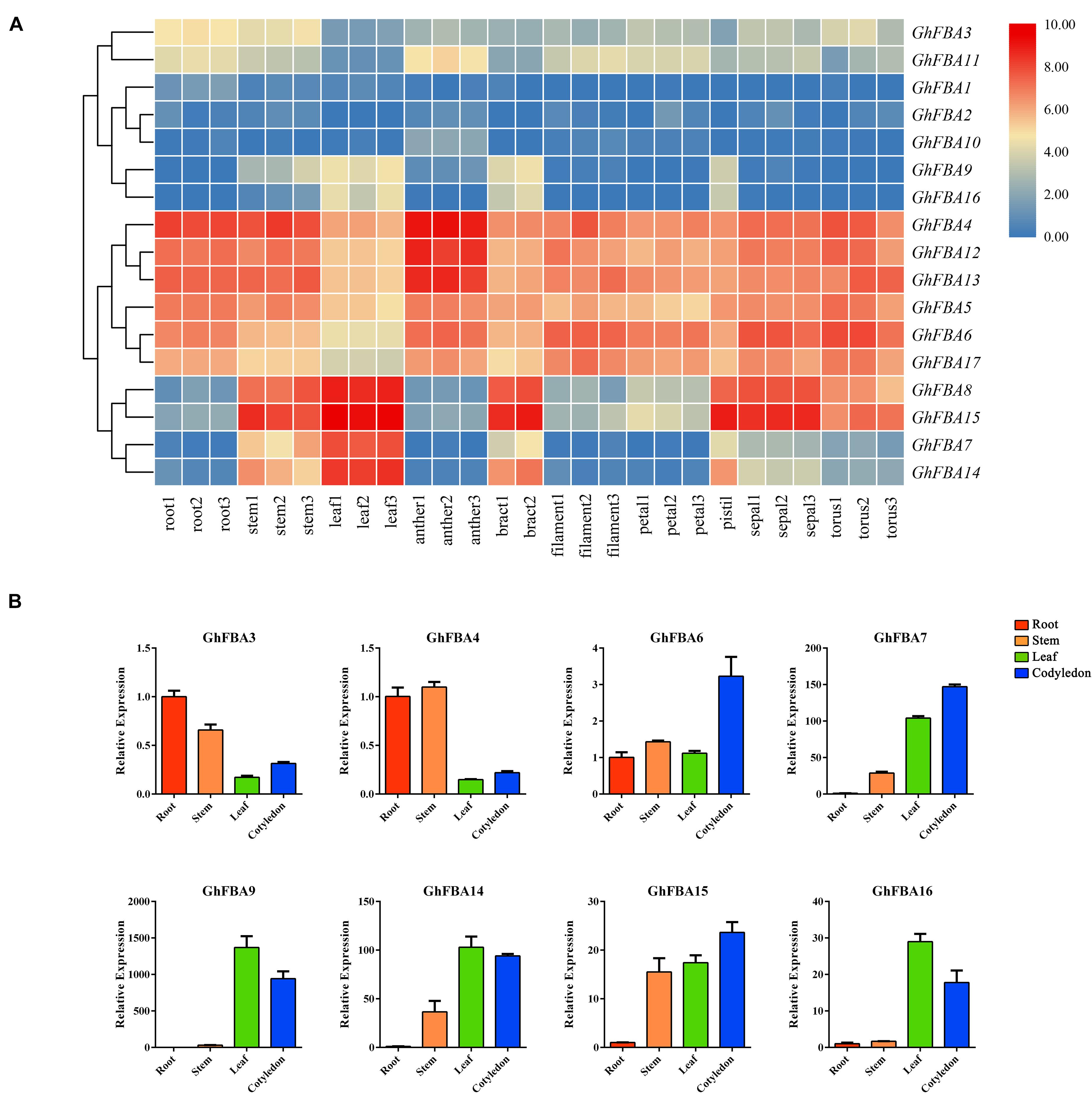
Figure 6. Expression profiles of the GhFBA genes in various tissues. (A) Hierachical clustering of expression profiles of GhFBA genes in various tissues. The TPM values were perform treatment of log2(TPM + 1), then the results were used to visualize the heatmap. (B) Expression analysis of eight representative GhFBA genes in different tissues by qRT-PCR, and GhHIS gene was used as a reference gene, vertical bars indicate standard deviation.
Expression Patterns of GhFBA Members Under Abiotic Stress Treatments
To further analyze the physiological and biochemical functions of GhFBA genes under different environment conditions, the expression patterns of GhFBA members in response to drought, cold, salt and heat stresses were further investigated according to the transcriptome date (Figure 7A). And the reliability of the transcriptome date was further validate by qRT-PCR based on three samples and eight GhFBA genes (Figure 7B). Overall, most numbers of GhFBA family were affected by abiotic stresses (Figure 7 and Supplementary Table 7). When treated with cold stress, the expression levels of GhFBA7/8/14/15/16 were significantly up-regulated. Under heat stress, the expression of GhFBA7/8/9/14/15/16 was sharply down-regulated at 1 h, but then they were strongly induced at 3 h. Additionally, the expression of GhFBA4/5/12/13 was up-regulated at 1 h, and gradually returned to normal levels. When treated with drought stress, the expression levels of GhFBA7/9/14/15 were up-regulated compared to the control groups. Furthermore, under salt stress, the expression levels GhFBA7/8/9/14/15/16 were up-regulated after 24 h treatment. The expression profiles of GhFBA11 and GhFBA17 were not affected by abiotic stress, indicating that they might be house keeping genes. Taken together, GhFBA genes displayed various expression patterns in response to adverse environmental conditions, suggesting that GhFBA genes might play an important role in stress resistance.
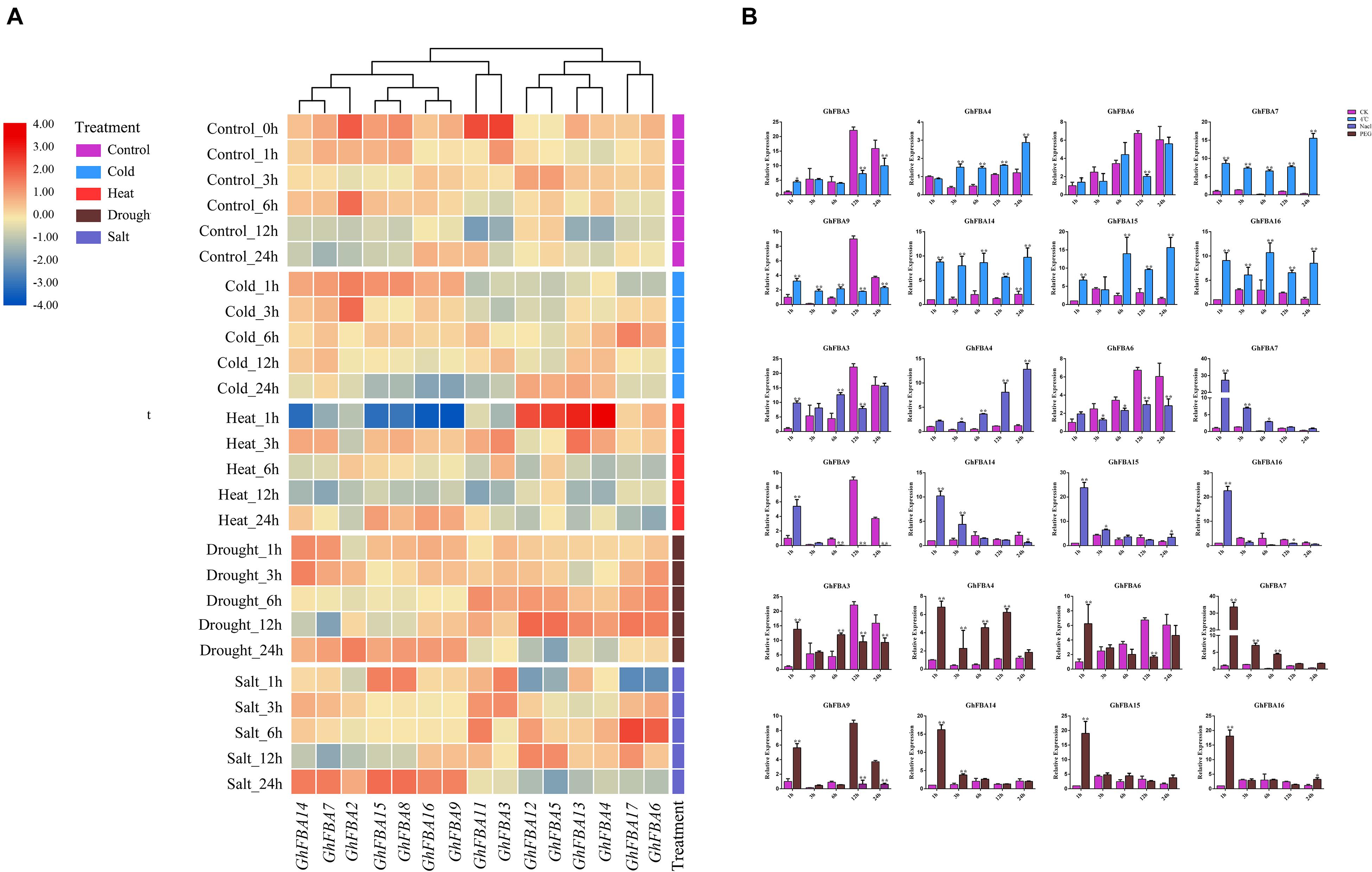
Figure 7. Expression profiles of GhFBA genes under different stresses. (A) The log2(TPM + 1) values of TPM were used to create the heatmap, and z-score method was used to normalize the results by line. The transcript abundances were represented by color scales ranging from blue (low) to red (high). (B) Verification of the transcriptome data by qRT-PCR, GhHIS gene was used as reference gene, and asterisks indicate the significant differences between treatment groups and control groups (*P < 0.05, **P < 0.01, Student’s t-test).
Expression Analysis of GhFBA Genes Under Different Phytohormonal Treatment
In order to have a further understanding of the regulatory mechanisms of GhFBA expression under different phytohormone treatments, 8 GhFBA members from different subclasses were selected for qRT-PCR analysis. Overall, phytohormone treatments had a significant effect on the expression levels of the detected genes, but the regulation mechanisms were quite different (Figure 8 and Supplementary Table 8). For instance, GhFBA3 was significantly induced by SA and ethylene. GhFBA6 was response to MeJA, SA and ethylene. GhFBA4/14 showed opposite expression patterns when treated with different hormones, the expression was induced by SA and ethylene but repressed by ABA and MeJA. While the expression of GhFBA14 was induced by ABA, MeJA and SA, but repressed by ethylene. Moreover, the transient increase of the expression of GhFBA7/9/15/16 after the phytohormone treatment suggested that these GhFBA genes might function as signal molecules in the regulatory pathway of cotton growth and stress tolerance.
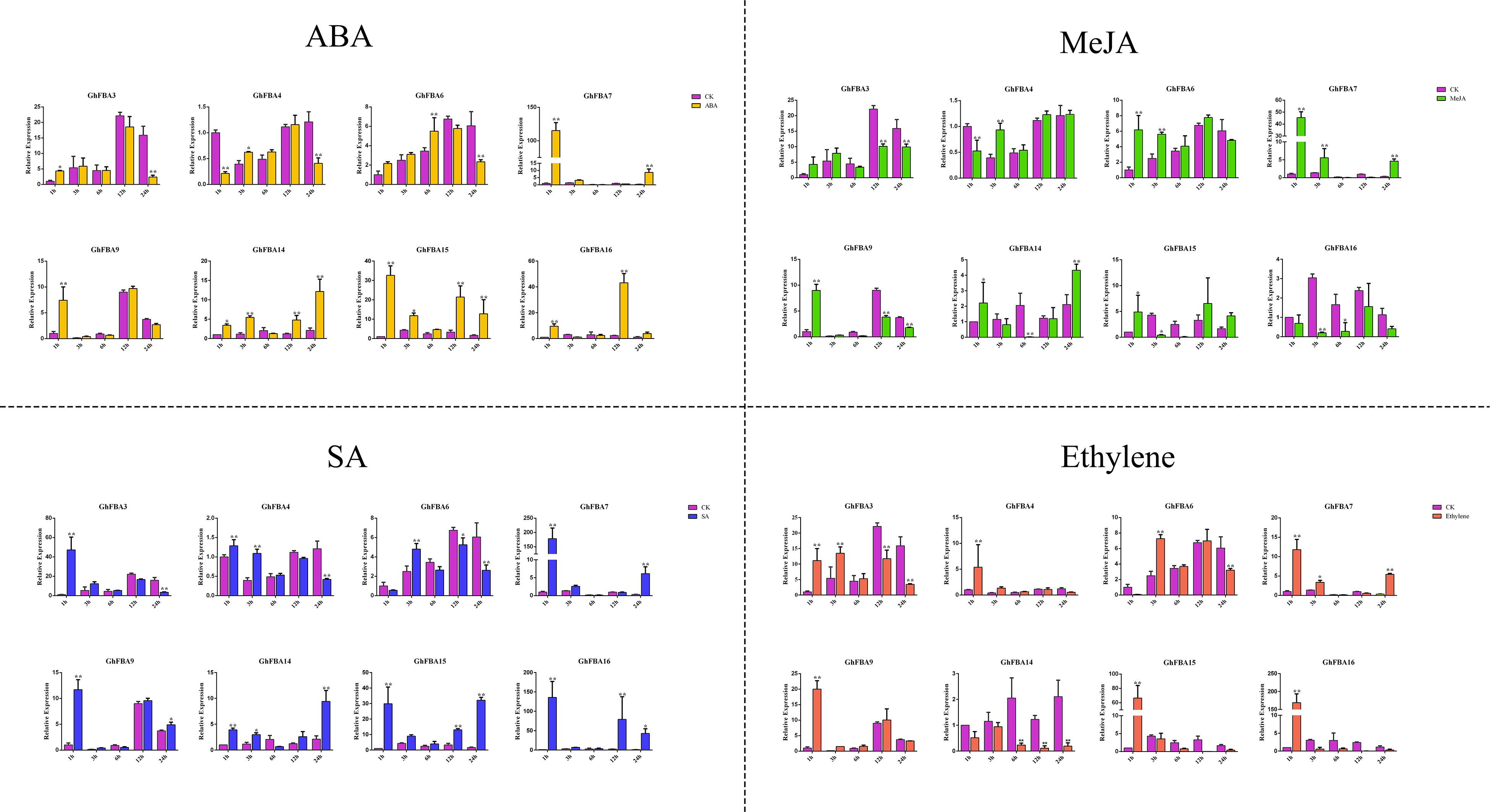
Figure 8. Expression patterns of 8 selected GhFBA genes in response to phytohormone treatments. qRT-PCR was performed to analyze the relative expression levels, and GhHIS was used as a reference gene. Asterisks indicate the significant differences between treatment groups and control groups (*P < 0.05, **P < 0.01, Student’s t-test).
Co-regulatory Networks of GhFBA Genes
Based on the public RNA-seq datasets of G. hirsutum, the Pearson correlation coefficients (PCCs) were calculated and the co-regulatory networks were constructed. As shown on Figure 9A, the genes within the same subclass usually represent positive correlations, for example, within class-Ia, GhFBA7, GhFBA 8, GhFBA14 and GhFBA15 showed positive correlations with each other. Likewise, two members of class-Ic, GhGBA9 and GhFBA16 also exhibited positive correlations. Moreover, positive correlations were observed between the members from different subclasses, such as the members of class-Ia, GhFBA6/17, showed positive correlations with GhFBA4/5/12/13 that belong class-Ie. However, GhFBA6/17 showed negative correlations with GhFBA7/8/14/15 and GhFBA9/16. Furthermore, all significant PCCs (p < 0.05 and | PCCs| > 0.5) of GhFBAs were extracted and used to construct the co-regulatory networks. Overall, the co-regulatory networks were constituted with 16 nodes and 70 edges, and only GhFBA2 showed no correlation with other members. As shown in Figure 9B, 30 GhFBA gene pairs showed negative correlations (PCCs < −0.5 and p-value < 0.05), 40 gene pairs showed positive correlations and 24 pairs showed strong positive correlations (0.5 < PCCs < 1 and p-value < 0.05).
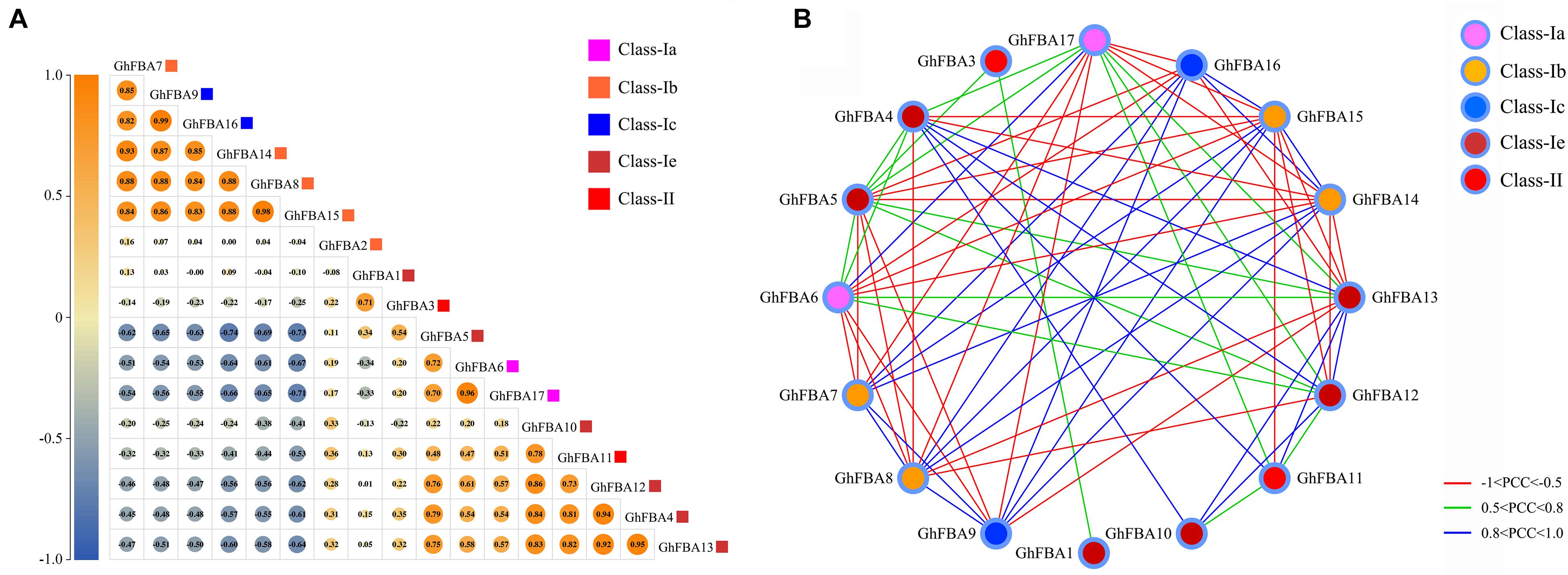
Figure 9. Pairwise correlation and co-regulatory networks of GhFBA genes. (A) Correlation analysis of GhFBA genes. The correlations were based on the PCC values and represented by the size and color of the circles. The squares with different colors were used to represent different subclasses. (B) Co-regulatory networks of GhFBA gene pairs with | PCC| > 0.5 and p-value <0.05. The edge lines with different colors represent the correlation levels of GhFBA gene pairs and the nodes with different colors indicate the information of different subclasses.
Discussion
As the initial stage of photosynthetic carbon fixation, Calvin-Benson cycle plays an indispensable role during plant growth and maturation. In recent years, more and more researches suggested that the rubisco was not the only factor that controls photosynthesis rate (Fukayama et al., 2012; Wostrikoff et al., 2012; Feiz et al., 2014; Bracher et al., 2017; Salesse-Smith et al., 2018). FBA, as the first bifurcation point of Calvin-Benson cycle, could be a potential target of genetic engineering to increase the photosynthetic carbon CO2 fixation rate.
Cotton is not only the main source of renewable textlie fiber, but also an important material for cottonseed oil production. Therefore, as a key enzyme in both photosynthesis and glycolysis (Haritatos et al., 2000; Sasaki and Nagano, 2004). FBA genes are of great significance to cotton production. In current study, we totally identified 17 FBA genes G. hirsutum. The size of FBA family in G. hirsutum was larger than A. thaliana (8) (Lu, 2011), rice (7) and tomato (8) (Cai et al., 2016), but smaller than wheat (21) (Lv et al., 2017) and B. napus (22) (Zhao et al., 2019), this result indicated that the number of genes within a FBA family might be associated with the genome size. Based on the function domains, GhFBAs could be classified into two groups and the members in both groups have the ability to catalyze the hydrolysis of fructose 1,6-bisphosphate (Capodagli et al., 2014). But there was no similarities between them in gene sequence, protein structure or catalytic mechanism, so they are always considered to have developed from different origins (Lebherz and Rutter, 1969; von der Osten et al., 1989; Schnarrenberger et al., 1990; Zgiby et al., 2000). Moreover, we analyzed the FBA characteristics in G. raimondii and G. arboreum (Supplementary Table 1, 9) FBA genes in each species were identified. In theory, the genes of tetraploid G. hirsutum should have one-to-one correspondence with the diploid parents, but only 17 GhFBAs were found in G. hirsutum. A proper explanation of the phenomenon was that after the speciation of G. hirsutum, some genes were lost because of the evolutionary divergence between different subgenomes.
The phylogenetic and homologous analysis could provide the information about evolution relationships. Based on the phylogenetic tree, 17 GhFBAs members were divided into two classes, and classs-I could be further classified into five subclasses (Figure 2). The members within the same subclass usually shared high similarity in sequences, gene structure and motif composition (Figure 3). Additionally, allopolyploid cotton species, including G. hirsutum, appears to have emerged in the last 1-2 million years, so the evolution relationship among these three species are very close. In this study, we totally identified 32 orthologous gene pairs of FBAs among the three species (Figure 5). Moreover, gene duplication events played an essential role in forcing the evolution process of genomes and genetic systems (Moore and Purugganan, 2003; Zhu et al., 2014). Segmental, tandem, and transposition events were thought to be the three major patterns (Kong et al., 2007). While in plants, segmental and tandem duplication events were regarded as the primary motivation for the expansion of gene family (Cannon et al., 2004). In this study, 2 segmental duplication evens and one tandem duplication event were identified in G. hirsutum (Figure 5), suggesting segmental and tandem duplication events might play important roles in the gene family expansion of GhFBAs.
According to the transcriptome date, some valuable information about the potential functions of GhFBAs were obtained. GhFBA4, GhFBA5, GhFBA6, GhFBA12 and GhFBA13 exhibited constitutive expression patterns in all tissues, which indicated their key roles in the development of G. hirsutum. Besides, it’s worth noting that some GhFBA members displayed significant tissues-specific expression patterns. For example, the expression of GhFBA7 and GhFBA14 were relatively higher in stem and leaf. And GhFBA8 was specificly expressed in leaf and sepal. High expression levels in green organs suggested that GhFBA7/14 and GhFBA8/15 might play important roles in photosynthetic carbon fixation, so they are of great potentials in genetic engineering for improving the photosynthetic efficiency in the future. Furthermore, the diverse expression patterns in different tissues among the members of GhFBA family indicated a clear work division of GhFBA genes in the growth and development of G. hirsutum.
There are considerable evidences that FBA genes play important roles in conferring tolerance including cold stress, heat stress, drought stress and high light acclimation (Fan et al., 2009; Lu et al., 2012; Khanna et al., 2014; Oelze et al., 2014; Mu et al., 2021). As the most widespread abiotic stress in field, drought have seriously negative effect on plant growth and development, it usually cause drastically decreases in the yield and quality of crops. Based on the drought transcriptome data, the expression of GhFBA7/9/14/16 were significantly induced (Figure 7). Further analysis showed that GhFBA14 have a close phylogenetic relationship with AtFBA1, a typical drought-related FBA gene in Arabidopsis (Lu, 2011; Lu et al., 2012), suggesting GhFBA4 possibly plays a critical role in cotton drought stress regulation. Besides drought, temperature is another key factor that affects cotton yield and fiber quality. And it’s noteworthy that the members from class I-e, GhFBA4/5/12/13, were all up-regulated when treated with heat stress. Moreover, the complete opposite expression patterns of GhFBA7/8/9/14/15/16 under heat and cold stresses suggested the their importance in the temperature adaptation. In Arabidopsis, almost all AtFBAs were up-regulated under salt stress after 6 h, but only six GhFBAs were significantly up-regulated after 24 h. The insensitivity to salt stress indicated a functional difference between AtFBAs and GhFBAs.
As important signal molecules, phytohormones play crucial roles in regulating the plant growth and stresses resistance. However, only a few literature suggested that the expression of FBA genes were regulated by phytohormones (Konishi et al., 2004; Tanaka et al., 2004; Osakabe et al., 2005). According to the qRT-PCR results, all selected genes were significantly affected by phytohormone, but the expression patterns were quite different. And these complex regulation networks indicated that GhFBA genes participate in multiple biological process during plant growth through phytohormones pathways in cotton. Furthermore, the expression of GhFBA7, GhFBA9, GhFBA15 and GhFBA16 was immediately up-regulated at 1 h after the treatment and then return to the normal levels quickly within 3 h. Based on these dramatic changes of the expression, it could be hypothesized that GhFBA7/9/14/16 might act as signal molecules in cotton phytohormone signal transduction, and further experiments were needed to verify this theory.
According to the expression analysis, most GhFBA members have one or more partners with similar expression patterns. Together with the result that AtFBA single mutant always showed no phenotypic variation (Lu, 2011), we conjectured that cotton FBA genes are functionally redundant. As an indispensable part of glycolysis and Calvin-Benson cycle, the existence of multiple members with similar functions ensures the normal metabolism of cell and eventually lead to the redundancy of FBA members. Furthermore, most GhFBAs were response to multiple phytohormone and stress treatments, suggesting there is a remarkable function crosstalk and work division among the members. The redundancy of GhFBA genes ensure the normal growth when some members lose their function, and the work division allow cotton to quickly respond and adapt to new environment when living conditions change.
In Brassica napus and Triticum aestivum (Lv et al., 2017; Zhao et al., 2019), the FBA genes showed close correlations according to the expression patterns and could be classified into different clusters. While in G. hirsutum, the co-regulatory networks were more complex and hardly to be classified (Figure 9B). The origin of G. hirsutum might be a key factor for this results. In the diploid parents of G. hirsutum, two complex regulatory networks of FBA genes have already existed, after the interspecific hybridization, two networks interacted with each other and eventually formed a more complicated co-regulatory network of GhFBA genes. Additionally, the complexity of co-regulatory networks further indicated the coexistence of work division and functional redundancy between GhFBA genes.
Overall, current study performed a genome-wide analysis of FBA gene family in G.hirsutum for the first time. And the results will shed light on the functional analysis of GhFBA genes and provide valuable resources to deeply explore their biological roles in improving cotton yield and quality.
Data Availability Statement
The datasets presented in this study can be found in online repositories. The names of the repository/repositories and accession number(s) can be found in the article/Supplementary Material.
Author Contributions
Z-QL and YZ: bioinformation analysis and data processing. HL, T-TS, C-GL, and Z-CH: cotton plants cultivating, material treatment and sample collection. Z-QL, HL, and T-TS: RNA extraction and qRT-PCR experiment performing. Z-QL: manuscript writing. YZ: discussion writing and manuscript review. All authors contributed to the article and approved the submitted version.
Funding
This work was supported by Genetically Modified Cotton Safety Evaluation Technology (2016zx08011002-004).
Conflict of Interest
The authors declare that the research was conducted in the absence of any commercial or financial relationships that could be construed as a potential conflict of interest.
Publisher’s Note
All claims expressed in this article are solely those of the authors and do not necessarily represent those of their affiliated organizations, or those of the publisher, the editors and the reviewers. Any product that may be evaluated in this article, or claim that may be made by its manufacturer, is not guaranteed or endorsed by the publisher.
Supplementary Material
The Supplementary Material for this article can be found online at: https://www.frontiersin.org/articles/10.3389/fpls.2021.696698/full#supplementary-material
Supplementary Table 1 | Primers used in qRT-PCR analysis.
Supplementary Table 2 | Characteristics information of FBA family genes in G. raimondii and G. arboreum.
Supplementary Table 3 | Annotation of cis-acting regulatory elements in the promoters of GhFBA genes.
Supplementary Table 4 | Gene duplication of FBA gene in Gossypium hirsutum.
Supplementary Table 5 | One-to-one orthologous relationships between G. hirsutum and other two cotton species.
Supplementary Table 6 | The public available RNA-seq date of GhFBA genes in different tissues.
Supplementary Table 7 | The public available RNA-seq date of GhFBA genes under different stress treatments.
Supplementary Table 8 | Expression profiles of 8 GhFBA genes in response to different stress and phytohormone treatments.
Supplementary Table 9 | Expression patterns of 8 GhFBA genes in four different tissues.
Abbreviations
Rubisco, Ribulose-1,5-bisphosphate carboxylase/oxygenase; RuBP, ribulose-1,5-bisphosphate; FBP, fructose-1,6-diphosphate; G3P, glyceraldehyde-3-phosphate; DHAP, dihydroxyacetone phosphate; SBP, sedoheptulose 1,7-bisphosphate; E4P, erythrose 4-phosphate; cFBA, cytosolic located FBA; cpFBA, chloroplast/plastid located FBA; TPM, transcripts per million; qRT-PCR, quantitative real-time PCR; ABA, abscisic acid; SA, salicylic acid; MeJA, Methyl Jasmonate.
Footnotes
- ^ https://cottonfgd.org
- ^ http://pfam.xfam.org
- ^ http://smart.embl-heidelberg.de/
- ^ https://web.expasy.org/protparam/
- ^ http://gsds.cbi.pku.edu.cn
- ^ http://www.genscript.com/wolf-psort.html
- ^ http://meme.nbcr.net/meme/intro.html
- ^ https://github.com/CJ-Chen/TBtools
References
Anderson, L. E., Wang, X., and Gibbons, J. T. (1995). Three enzymes of carbon metabolism or their antigenic analogs in pea leaf nuclei. Plant Physiol. 108, 659–667. doi: 10.1104/pp.108.2.659
Bracher, A., Whitney, S., Hartl, F., and Hayer-Hartl, M. (2017). Biogenesis and Metabolic Maintenance of Rubisco. Annu. Rev. Plant Biol. 68, 29–60. doi: 10.1146/annurev-arplant-043015-111633
Bukowiecki, A. C., and Anderson, L. E. (1974). Multiple forms of aldolase and triose phosphate isomerase in diverse plant species. Plant Sci. Lett. 3, 381–386. doi: 10.1016/0304-4211(74)90019-4
Cai, B. (2017). Expression Pattern and Regulation of Fructose 1,6-Diphosphate Aldolase Gene on Photosynthesis in Tomato. Ph. D. Thesis. Shandong: Shandong Agricultural University.
Cai, B., Li, Q., Liu, F., Bi, H., and Ai, X. (2018). Decreasing fructose-1,6-bisphosphate aldolase activity reduces plant growth and tolerance to chilling stress in tomato seedlings. Physiol. Plant 163, 247–258. doi: 10.1111/ppl.12682
Cai, B., Li, Q., Xu, Y., Yang, L., Bi, H., and Ai, X. (2016). Genome-wide analysis of the fructose 1,6-bisphosphate aldolase (FBA) gene family and functional characterization of FBA7 in tomato. Plant physiol. Biochem. PPB 108, 251–265. doi: 10.1016/j.plaphy.2016.07.019
Cannon, S. B., Mitra, A., Baumgarten, A., Young, N. D., and May, G. (2004). The roles of segmental and tandem gene duplication in the evolution of large gene families in Arabidopsis thaliana. BMC Plant Biol. 4:10. doi: 10.1186/1471-2229-4-10
Capodagli, G. C., Sedhom, W. G., Jackson, M., Ahrendt, K. A., and Pegan, S. D. (2014). A noncompetitive inhibitor for Mycobacterium tuberculosis’s class IIa fructose 1,6-bisphosphate aldolase. Biochemistry 53, 202–213. doi: 10.1021/bi401022b
Du, X., Huang, G., He, S., Yang, Z., Sun, G., Ma, X., et al. (2018). Resequencing of 243 diploid cotton accessions based on an updated A genome identifies the genetic basis of key agronomic traits. Nat. Genet. 50, 796–802. doi: 10.1038/s41588-018-0116-x
Fan, W., Zhang, Z., and Zhang, Y. (2009). Cloning and molecular characterization of fructose-1,6-bisphosphate aldolase gene regulated by high-salinity and drought in Sesuvium portulacastrum. Plant Cell Rep. 28, 975–984. doi: 10.1007/s00299-009-0702-6
Feiz, L., Williams-Carrier, R., Belcher, S., Montano, M., Barkan, A., and Stern, D. (2014). A protein with an inactive pterin-4a-carbinolamine dehydratase domain is required for Rubisco biogenesis in plants. Plant J. Cell Mol. Biol. 80, 862–869. doi: 10.1111/tpj.12686
Flechner, A., Gross, W., Martin, W. F., and Schnarrenberger, C. (1999). Chloroplast class I and class II aldolases are bifunctional for fructose-1,6-biphosphate and sedoheptulose-1,7-biphosphate cleavage in the Calvin cycle. FEBS Lett. 447, 200–202. doi: 10.1016/s0014-5793(99)00285-9
Fukayama, H., Ueguchi, C., Nishikawa, K., Katoh, N., Ishikawa, C., Masumoto, C., et al. (2012). Overexpression of rubisco activase decreases the photosynthetic CO2 assimilation rate by reducing rubisco content in rice leaves. Plant Cell Physiol. 53, 976–986. doi: 10.1093/pcp/pcs042
Gu, L., Li, L., Wei, H., Wang, H., Su, J., Guo, Y., et al. (2018). Identification of the group IIa WRKY subfamily and the functional analysis of GhWRKY17 in upland cotton (Gossypium hirsutum L.). PLoS One 13:e0191681. doi: 10.1371/journal.pone.0191681
Haake, V., Zrenner, R., Sonnewald, U., and Stitt, M. (1998). A moderate decrease of plastid aldolase activity inhibits photosynthesis, alters the levels of sugars and starch, and inhibits growth of potato plants. Plant J. Cell Mol. Biol. 14, 147–157. doi: 10.1046/j.1365-313x.1998.00089.x
Haritatos, E., Medville, R., and Turgeon, R. (2000). Minor vein structure and sugar transport in Arabidopsis thaliana. Planta 211, 105–111. doi: 10.1007/s004250000268
Henze, K., Morrison, H. G., Sogin, M. L., and Müller, M. (1998). Sequence and phylogenetic position of a class II aldolase gene in the amitochondriate protist, Giardia lamblia. Gene 222, 163–168. doi: 10.1016/s0378-1119(98)00499-5
Hu, Y., Chen, J., Fang, L., Zhang, Z., Ma, W., Niu, Y., et al. (2019). Gossypium barbadense and Gossypium hirsutum genomes provide insights into the origin and evolution of allotetraploid cotton. Nat. Genet. 51, 739–748. doi: 10.1038/s41588-019-0371-5
Huang, J., Pang, C., Fan, S., Song, M., Yu, J., Wei, H., et al. (2015). Genome-wide analysis of the family 1 glycosyltransferases in cotton. Mol. Genet. Genomics 290, 1805–1818. doi: 10.1007/s00438-015-1040-8
Hudson, G. S., Evans, J. R., von Caemmerer, S., Arvidsson, Y. B., and Andrews, T. J. (1992). Reduction of ribulose-1,5-bisphosphate carboxylase/oxygenase content by antisense RNA reduces photosynthesis in transgenic tobacco plants. Plant Physiol. 98, 294–302. doi: 10.1104/pp.98.1.294
Khanna, S. M., Taxak, P. C., Jain, P. K., Saini, R., and Srinivasan, R. (2014). Glycolytic enzyme activities and gene expression in Cicer arietinum exposed to water-deficit stress. Appl. Biochem. Biotechnol. 173, 2241–2253. doi: 10.1007/s12010-014-1028-6
Kong, H., Landherr, L. L., Frohlich, M. W., Leebens-Mack, J., Ma, H., and dePamphilis, C. W. (2007). Patterns of gene duplication in the plant SKP1 gene family in angiosperms: evidence for multiple mechanisms of rapid gene birth. Plant J. 50, 873–885. doi: 10.1111/j.1365-313X.2007.03097.x
Konishi, H., Yamane, H., Maeshima, M., and Komatsu, S. (2004). Characterization of fructose-bisphosphate aldolase regulated by gibberellin in roots of rice seedling. Plant Mol. Biol. 56, 839–848. doi: 10.1007/s11103-004-5920-2
Larkindale, J., and Vierling, E. (2008). Core genome responses involved in acclimation to high temperature. Plant Physiol. 146, 748–761. doi: 10.1104/pp.107.112060
Lebherz, H. G., and Rutter, W. J. (1969). Distribution of fructose diphosphate aldolase variants in biological systems. Biochemistry 8, 109–121. doi: 10.1021/bi00829a016
Li, F., Fan, G., Wang, K., Sun, F., Yuan, Y., Song, G., et al. (2014). Genome sequence of the cultivated cotton Gossypium arboreum. Nat. Genet. 46, 567–572. doi: 10.1038/ng.2987
Li, S. (2018). Effects of Exogenous 2,4-epibrassinolide on Cotton under Low Temperature Stress. Ph. D. Thesis. Beijing: Chinese Academy of Agricultural Sciences.
Lu, W. (2011). Genome-wide Analysis of the Fructose Bisphosphate Aldolases in Arabidopsis. Ph. D. Thesis. Shandong: Shandong Agricultural University.
Lu, W., Tang, X., Huo, Y., Xu, R., Qi, S., Huang, J., et al. (2012). Identification and characterization of fructose 1,6-bisphosphate aldolase genes in Arabidopsis reveal a gene family with diverse responses to abiotic stresses. Gene 503, 65–74. doi: 10.1016/j.gene.2012.04.042
Lv, G., Guo, X., Xie, L., Xie, C., Zhang, X., Yang, Y., et al. (2017). Triticum aestivumMolecular Characterization, Gene Evolution, and Expression Analysis of the Fructose-1, 6-bisphosphate Aldolase (FBA) Gene Family in Wheat (L.). Front. Plant Sci. 8:1030. doi: 10.3389/fpls.2017.01030
Marsh, J. J., and Lebherz, H. G. (1992). Fructose-bisphosphate aldolases: an evolutionary history. Trends Biochem. Sci. 17, 110–113. doi: 10.1016/0968-0004(92)90247-7
Michelis, R., and Gepstein, S. (2000). Identification and characterization of a heat-induced isoform of aldolase in oat chloroplast. Plant Mol. Biol. 44, 487–498. doi: 10.1023/a:1026528319769
Moore, R. C., and Purugganan, M. D. (2003). The early stages of duplicate gene evolution. Proc. Natl. Acad. Sci. U S A. 100, 15682–15687. doi: 10.1073/pnas.2535513100
Mu, J., Fu, Y., Liu, B., Zhang, Y., Wang, A., Li, Y., et al. (2021). SiFBA5, a cold-responsive factor from Saussurea involucrata promotes cold resilience and biomass increase in transgenic tomato plants under cold stress. BMC Plant Biol. 21:75. doi: 10.1186/s12870-021-02851-8
Nakahara, K., Yamamoto, H., Miyake, C., and Yokota, A. (2003). Purification and characterization of class-I and class-II fructose-1,6-bisphosphate aldolases from the cyanobacterium Synechocystis sp. PCC 6803. Plant Cell Physiol. 44, 326–333. doi: 10.1093/pcp/pcg044
Oelze, M. L., Muthuramalingam, M., Vogel, M. O., and Dietz, K. J. (2014). The link between transcript regulation and de novo protein synthesis in the retrograde high light acclimation response of Arabidopsis thaliana. BMC Genomics 15:320. doi: 10.1186/1471-2164-15-320
Osakabe, Y., Maruyama, K., Seki, M., Satou, M., Shinozaki, K., and Yamaguchi-Shinozaki, K. (2005). Leucine-rich repeat receptor-like kinase1 is a key membrane-bound regulator of abscisic acid early signaling in Arabidopsis. Plant Cell 17, 1105–1119. doi: 10.1105/tpc.104.027474
Oztur, Z., Talamé, V., Deyholos, M., Michalowski, C., Galbraith, D., Gozukirmizi, N., et al. (2002). Monitoring large-scale changes in transcript abundance in drought- and salt-stressed barley. Plant Mol. Biol. 48, 551–573. doi: 10.1023/a:1014875215580
Páez-Valencia, J., Valencia-Mayoral, P., Sánchez-Gómez, C., Contreras-Ramos, A., Hernández-Lucas, I., Martínez-Barajas, E., et al. (2008). Identification of Fructose-1,6-bisphosphate aldolase cytosolic class I as an NMH7 MADS domain associated protein. Biochem. Biophys. Res. Commun. 376, 700–705. doi: 10.1016/j.bbrc.2008.09.064
Paterson, A. H., Wendel, J. F., Gundlach, H., Guo, H., Jenkins, J., Jin, D., et al. (2012). Repeated polyploidization of Gossypium genomes and the evolution of spinnable cotton fibres. Nature 492, 423–427. doi: 10.1038/nature11798
Penhoet, E., Kochman, M., Valentine, R., and Rutter, W. J. (1967). The subunit structure of mammalian fructose diphosphate aldolase. Biochemistry 6, 2940–2949. doi: 10.1021/bi00861a039
Portis, A. R. Jr. (2003). Rubisco activase - Rubisco’s catalytic chaperone. Photosynth. Res. 75, 11–27. doi: 10.1023/a:1022458108678
Purev, M., Kim, M., Samdan, N., and Yang, D. (2008). [Isolation of a novel fructose-1,6-bisphosphate aldolase gene from Codonopsis lanceolata and analysis of the response of this gene to abiotic stresses]. Molekuliarnaia Biol. 42, 206–213.
Raines, C. A. (2003). The Calvin cycle revisited. Photosynth. Res. 75, 1–10. doi: 10.1023/a:1022421515027
Salesse-Smith, C., Sharwood, R., Busch, F., Kromdijk, J., Bardal, V., and Stern, D. (2018). Overexpression of Rubisco subunits with RAF1 increases Rubisco content in maize. Nat. Plants 4, 802–810. doi: 10.1038/s41477-018-0252-4
Sasaki, Y., and Nagano, Y. (2004). Plant acetyl-CoA carboxylase: structure, biosynthesis, regulation, and gene manipulation for plant breeding. Biosci. Biotechnol. Biochem. 68, 1175–1184. doi: 10.1271/bbb.68.1175
Schnarrenberger, C., Jacobshagen, S., Müller, B., and Krüger, I. (1990). Evolution of isozymes of sugar phosphate metabolism in green algae. Prog. Clin. Biol. Res. 344, 743–764.
Stitt, M., and Hurry, V. (2002). A plant for all seasons: alterations in photosynthetic carbon metabolism during cold acclimation in Arabidopsis. Curr. Opin. Plant Biol. 5, 199–206. doi: 10.1016/s1369-5266(02)00258-3
Sun, H. (2019). Molecular Mechanism Analysis of Cotton in Response to Salt Stress. Ph. D. Thesis. Huazhong: Huazhong Agricultural University.
Tamura, K., Stecher, G., Peterson, D., Filipski, A., and Kumar, S. (2013). MEGA6: Molecular Evolutionary Genetics Analysis version 6.0. Mol. Biol. Evol. 30, 2725–2729. doi: 10.1093/molbev/mst197
Tanaka, N., Konishi, H., Khan, M. M., and Komatsu, S. (2004). Proteome analysis of rice tissues by two-dimensional electrophoresis: an approach to the investigation of gibberellin regulated proteins. Mol. Genet. Genomics 270, 485–496. doi: 10.1007/s00438-003-0929-9
Tolan, D. R., Niclas, J., Bruce, B. D., and Lebo, R. V. (1987). Evolutionary implications of the human aldolase-A, -B, -C, and -pseudogene chromosome locations. Am. J. Hum. Genet. 41, 907–924.
Uematsu, K., Suzuki, N., Iwamae, T., Inui, M., and Yukawa, H. (2012). Increased fructose 1,6-bisphosphate aldolase in plastids enhances growth and photosynthesis of tobacco plants. J. Exp. Bot. 63, 3001–3009. doi: 10.1093/jxb/ers004
von der Osten, C. H., Barbas, C. F. III, Wong, C. H., and Sinskey, A. J. (1989). Molecular cloning, nucleotide sequence and fine-structural analysis of the Corynebacterium glutamicum fda gene: structural comparison of C. glutamicum fructose-1,6-biphosphate aldolase to class I and class II aldolases. Mol. Microbiol. 3, 1625–1637. doi: 10.1111/j.1365-2958.1989.tb00148.x
Wang, Y., Tang, H., Debarry, J. D., Tan, X., Li, J., Wang, X., et al. (2012). MCScanX: a toolkit for detection and evolutionary analysis of gene synteny and collinearity. Nucleic Acids Res. 40:e49. doi: 10.1093/nar/gkr1293
Wendel, J. F., and Cronn, R. C. (2003). Polyploidy and the evolutionary history of cotton. Adv. Agronomy 78, 139–186. doi: 10.1016/s0065-2113(02)78004-8
Wostrikoff, K., Clark, A., Sato, S., Clemente, T., and Stern, D. (2012). Ectopic expression of Rubisco subunits in maize mesophyll cells does not overcome barriers to cell type-specific accumulation. Plant Physiol. 160, 419–432. doi: 10.1104/pp.112.195677
Xue, G., McIntyre, C., Glassop, D., and Shorter, R. (2008). Use of expression analysis to dissect alterations in carbohydrate metabolism in wheat leaves during drought stress. Plant Mol. Biol. 67, 197–214. doi: 10.1007/s11103-008-9311-y
Xu, W., Wang, C., Xu, X., Cai, C., and Guo, W. (2013). Cloning and Expression Analysis of A Novel Gene Encoding Fructose-1,6-bisphosphatase in Cotton. Cotton Sci. 29, 549–556.
Yamada, S., Komori, T., Hashimoto, A., Kuwata, S., Imaseki, H., and Kubo, T. (2000). Differential expression of plastidic aldolase genes in Nicotiana plants under salt stress. Plant Sci. 154, 61–69. doi: 10.1016/s0168-9452(00)00188-6
Yang, S., Liu, X., Pan, Z., Nan, F., Zhu, Y., Shi, Y. Z., et al. (2012). Research Progress on Cotton Drought Tolerance Breeding in Shanxi Province. China Cotton 39, 1–4. doi: 10.1007/s11032-014-0015-5
Yang, Z., Ge, X., Yang, Z., Qin, W., Sun, G., Wang, Z., et al. (2019). Extensive intraspecific gene order and gene structural variations in upland cotton cultivars. Nat. Commun. 10:2989. doi: 10.1038/s41467-019-10820-x
Zeng, Y., Tan, X., Zhang, L., Jiang, N., and Cao, H. (2014). Identification and expression of fructose-1,6-bisphosphate aldolase genes and their relations to oil content in developing seeds of tea oil tree (Camellia oleifera). PLoS One 9:e107422. doi: 10.1371/journal.pone.0107422
Zgiby, S. M., Thomson, G. J., Qamar, S., and Berry, A. (2000). Exploring substrate binding and discrimination in fructose1, 6-bisphosphate and tagatose 1,6-bisphosphate aldolases. Eur. J. Biochem. 267, 1858–1868. doi: 10.1046/j.1432-1327.2000.01191.x
Zhang, G., Liu, Y., Ni, Y., Meng, Z., Lu, T., and Li, T. (2014). Exogenous calcium alleviates low night temperature stress on the photosynthetic apparatus of tomato leaves. PLoS One 9:e97322. doi: 10.1371/journal.pone.0097322
Zhao, W., Liu, H., Zhang, L., Hu, Z., Liu, J., Hua, W., et al. (2019). Genome-Wide Identification and Characterization of FBA Gene Family in Polyploid Crop Brassica napus. Int. J. Mol. Sci. 20:ijms20225749. doi: 10.3390/ijms20225749
Keywords: cotton, Calvin-Benson cycle, evolution, expression profiles, FBA
Citation: Li Z-Q, Zhang Y, Li H, Su T-T, Liu C-G, Han Z-C, Wang A-Y and Zhu J-B (2021) Genome-Wide Characterization and Expression Analysis Provide Basis to the Biological Function of Cotton FBA Genes. Front. Plant Sci. 12:696698. doi: 10.3389/fpls.2021.696698
Received: 30 April 2021; Accepted: 30 July 2021;
Published: 19 August 2021.
Edited by:
Fuguang Li, Chinese Academy of Agricultural Sciences (CAAS), ChinaReviewed by:
Dhruv Lavania, University of Alberta, CanadaChaoyou Pang, Chinese Academy of Agricultural Sciences (CAAS), China
Fafu Shen, Shandong Agricultural University, China
Copyright © 2021 Li, Zhang, Li, Su, Liu, Han, Wang and Zhu. This is an open-access article distributed under the terms of the Creative Commons Attribution License (CC BY). The use, distribution or reproduction in other forums is permitted, provided the original author(s) and the copyright owner(s) are credited and that the original publication in this journal is cited, in accordance with accepted academic practice. No use, distribution or reproduction is permitted which does not comply with these terms.
*Correspondence: Jian-Bo Zhu, emh1amlhbmJvc2h6QDE2My5jb20=
†These authors have contributed equally to this work and share first authorship