- 1State Key Laboratory of Tree Genetics and Breeding, Experimental Center of Forestry in North China, National Permanent Scientific Research Base for Warm Temperate Zone Forestry of Jiulong Mountain in Beijing, Chinese Academy of Forestry, Beijing, China
- 2State Key Laboratory of Tree Genetics and Breeding, Non-timber Forestry Research and Development Center, Chinese Academy of Forestry, Zhengzhou, China
- 3Department of Plant Sciences and Plant Pathology, Montana State University, Bozeman, MT, United States
Aquaporins (AQPs) are essential channel proteins that play a major role in plant growth and development, regulate plant water homeostasis, and transport uncharged solutes across biological membranes. In this study, 33 AQP genes were systematically identified from the kernel-using apricot (Prunus armeniaca L.) genome and divided into five subfamilies based on phylogenetic analyses. A total of 14 collinear blocks containing AQP genes between P. armeniaca and Arabidopsis thaliana were identified by synteny analysis, and 30 collinear blocks were identified between P. armeniaca and P. persica. Gene structure and conserved functional motif analyses indicated that the PaAQPs exhibit a conserved exon-intron pattern and that conserved motifs are present within members of each subfamily. Physiological mechanism prediction based on the aromatic/arginine selectivity filter, Froger’s positions, and three-dimensional (3D) protein model construction revealed marked differences in substrate specificity between the members of the five subfamilies of PaAQPs. Promoter analysis of the PaAQP genes for conserved regulatory elements suggested a greater abundance of cis-elements involved in light, hormone, and stress responses, which may reflect the differences in expression patterns of PaAQPs and their various functions associated with plant development and abiotic stress responses. Gene expression patterns of PaAQPs showed that PaPIP1-3, PaPIP2-1, and PaTIP1-1 were highly expressed in flower buds during the dormancy and sprouting stages of P. armeniaca. A PaAQP coexpression network showed that PaAQPs were coexpressed with 14 cold resistance genes and with 16 cold stress-associated genes. The expression pattern of 70% of the PaAQPs coexpressed with cold stress resistance genes was consistent with the four periods [Physiological dormancy (PD), ecological dormancy (ED), sprouting period (SP), and germination stage (GS)] of flower buds of P. armeniaca. Detection of the transient expression of GFP-tagged PaPIP1-1, PaPIP2-3, PaSIP1-3, PaXIP1-2, PaNIP6-1, and PaTIP1-1 revealed that the fusion proteins localized to the plasma membrane. Predictions of an A. thaliana ortholog-based protein–protein interaction network indicated that PaAQP proteins had complex relationships with the cold tolerance pathway, PaNIP6-1 could interact with WRKY6, PaTIP1-1 could interact with TSPO, and PaPIP2-1 could interact with ATHATPLC1G. Interestingly, overexpression of PaPIP1-3 and PaTIP1-1 increased the cold tolerance of and protein accumulation in yeast. Compared with wild-type plants, PaPIP1-3- and PaTIP1-1-overexpressing (OE) Arabidopsis plants exhibited greater tolerance to cold stress, as evidenced by better growth and greater antioxidative enzyme activities. Overall, our study provides insights into the interaction networks, expression patterns, and functional analysis of PaAQP genes in P. armeniaca L. and contributes to the further functional characterization of PaAQPs.
Introduction
Aquaporins (AQPs) are involved in water transport and facilitate the passage of other small solutes, such as CO2, boric acid, H2O2, glycerol, urea, and silicic acid, through cell membranes (Maurel et al., 2008, 2009). In plants, AQPs play a central role in maintaining the hydraulic conductivity balance and water homeostasis and are involved in the tolerance of abiotic stresses such as cold, drought, or salt stresses. In addition, the roles of AQPs in various growth processes, such as fruit ripening, petal and stomatal regulation, male fertility, seed germination, petal movement, and guard cell closure, have been documented (Heinen and Chaumont, 2009; Mitani-Ueno et al., 2011).
According to the cluster analysis of gene members, AQPs can be classified into seven subfamilies: small basic intrinsic proteins (SIPs), tonoplast intrinsic proteins (TIPs), plasma membrane intrinsic proteins (PIPs), uncategorized X intrinsic proteins (XIPs), nodulin26-like intrinsic proteins (NIPs), hybrid intrinsic proteins (HIPs), and GlpF-like intrinsic proteins (GIPs) (Chaumont et al., 2001; Kaldenhoff and Fischer, 2006; Maurel et al., 2015). Plant AQPs usually include four subfamilies: TIPs, PIPs, SIPs, and NIPs (Anderberg et al., 2012). Although XIP subfamily members are found in some dicots, such as common bean (Ariani and Gepts, 2015), poplar (Gupta and Sankararamakrishnan, 2009), potato (Venkatesh et al., 2013), and Glycine max (Cheng et al., 2013), they have not been found in monocots or certain dicots, such as Arabidopsis thaliana (Danielson and Johanson, 2008). GIP and HIP subfamily members have been reported in Physcomitrella (Danielson and Johanson, 2008) and Selaginella (Anderberg et al., 2012).
Recent studies have shown that AQP families are composed of a large number of genes in plants. For instance, there are 34 AQPs in Oryza sativa (Nguyen et al., 2013), 53 in Chinese cabbage (Tao et al., 2014), 66 in Glycine max (Zhang et al., 2013), 35 in A. thaliana (Johanson et al., 2001), 41 in common bean (Ariani and Gepts, 2015), 55 in Populus trichocarpa (Gupta and Sankararamakrishnan, 2009), and 31 in Zea mays (Chaumont et al., 2001). The structural features of AQPs are highly conserved and involve an α-helical bundle forming six transmembrane domains (TM1-TM6), which are connected by five loops (LA-LE). Two conserved Asn-Pro-Ala (NPA) motifs are located in loop B (LB) and loop E (LE) and are involved in transport selectivity (Bansal and Sankararamakrishnan, 2007; Kayum et al., 2017). The ar/R selectivity filter is formed by each residue from TM2 (H2), TM5 (H5), and two residues from LE (LE1 and LE2) and determines the substrate specificity (Hove and Bhave, 2011; Mitani-Ueno et al., 2011). Froger positions P1-P5 represent five conserved amino acid residues and discriminate between glycerol-transporting AQPs (aquaglyceroporins, GLPs) and water-conducting AQPs (Froger et al., 1998). NPA motifs, aromatic/arginine (ar/R) selectivity, and Froger’s position are crucial for the physiological functions of AQPs.
Kernel-using apricot (P. armeniaca L., syn. Armeniaca vulgaris Lam., also considered by some researchers to be P. armeniaca L. × P. sibirica L.) is an economically and ecologically important forest tree species in the three northern regions of China (northwestern China, northern China, and northeastern China). Because of its broad adaptability, strong resistance to drought, and easy management, it is one of the pioneer tree species used for ecological construction in mountainous areas and sandy lands, especially in western China. It is one of the six major nut-producing species in the world and is an important species for the production of woody oil and protein beverages. It can also contribute to the economies of poverty-stricken mountainous areas in China. The first high-quality apricot plant (Chuanzhihong) genome has been sequenced and released, laying a foundation for research on the molecular mechanisms underlying important agronomic characteristics of apricot (Jiang et al., 2019). Owing to its short dormancy period and early flowering period, kernel-using apricot (P. armeniaca L.) easily suffers from late frost damage, resulting in yield reduction or even no production. An effective strategy to solve the problem of a low and unstable yield of kernel-using apricot is to mine key genes related to flower bud dormancy and cold resistance, determine gene expression patterns during dormancy and sprouting periods (SPs), elucidate possible functional mechanisms and determine how the flowering period can be artificially manipulated.
Physiological dormancy (PD) (which is generally called endodormancy) is defined as the stage in which buds cannot burst even under optimal environmental conditions, while ecological dormancy (ED) (which is generally called ecodormancy) is the stage in which buds cannot burst because of lacking environmental factors (especially the temperature requirement accumulation for flower buds in ED periods is not enough). Endodormant buds, which cannot burst under preferred conditions due to internal physiological factors (such as embryos that are not mature physiologically, have a hard seed coat with poor permeability, or contain substances inhibiting germination) (Mitrakos and Diamantoglou, 2006; Gao et al., 2020), transition into the ecodormant (ecologically dormant) stage and acquire bud break capacity after exposure to a certain period of low temperature. When external environmental conditions (the temperature requirement accumulation for flower buds in ecologically dormant periods is enough) (Mazzitelli et al., 2007; Rohde and Bhalerao, 2007) are satisfied, the flower buds enter the SP and germination stage (GS), showing growth signs and sprouting, after which they can fully develop.
Cold stress can cause chilling injury in late spring, which obviously limits plant development and influences fruit yield in economic forests. Previous studies have shown that AQP family members play vital roles in different dormancy periods and lead to freezing tolerance and cold acclimation. For example, in A. thaliana, the expression of AtPIP2-5 and AtPIP2-6 is significantly upregulated by cold stress in roots and other parts of the plant (Alexandersson et al., 2010). The expression of rice OsTIP1-1 is downregulated under cold stress conditions (Sakurai et al., 2005) but upregulated in response to water and salinity stress (Liu et al., 1994). Transgenic studies on AQPs have been conducted to examine their responses to abiotic stresses such as cold, drought, and salinity. A. thaliana plants overexpressing AtPIP1;4 or AtPIP2-5 exhibit greater tolerance to cold stress (Afzal et al., 2016). Compared with wild-type tobacco plants, transgenic tobacco plants overexpressing the wheat TaAQP7 (PIP2) gene display improved cold tolerance (Zhou et al., 2012). Overexpression of MusaPIP1-2 in transgenic banana plants enhances tolerance to cold and drought conditions (Shekhawat and Ganapathi, 2013). Although AQPs have been extensively studied in both dicots and monocots through genome-wide and functional analyses (Nguyen et al., 2013), our knowledge of AQPs in P. armeniaca is still limited, and the expression profile of AQP members in flower buds in the different dormancy and sprouting stages is largely unknown in this species.
In this study, the genome sequence of P. armeniaca (Longwangmao) and the sequences of known AQPs from other species (such as A. thaliana and Prunus persica) were used to identify the genes encoding AQP members. The phylogenetic relationships, chromosome distribution, conserved residues and elements, protein–protein interactions, and expression patterns of AQPs were investigated to identify their functions in cold stress in kernel-using apricot. These results provide valuable information for further studies on the molecular mechanism of AQPs, which will facilitate the selection of candidate genes for cold tolerance improvement through genetic engineering in kernel-using apricot.
Materials and Methods
Identification of Aquaporin Family Members in P. armeniaca
The predicted peptide sequences of AQP members were acquired from the genome database (unpublished) of kernel-using apricot (P. armeniaca L.), also known as Longwangmao (Chinese Pinyin name), to construct a local protein database. BLASTP searches were performed using AQP protein sequences of A. thaliana and poplar (Table 1) as queries in The Arabidopsis Information Resource (TAIR)1 and the Phytozome database (release version 12.02) as previously described (Gupta and Sankararamakrishnan, 2009; Zhang et al., 2013), with an E-value of 1e–10 and a minimum amino acid identity of 50%. The hidden Markov model (HMM) data of the MIP domain (PF00230) were downloaded from the Sanger database3. The PF00230 sequence information was then used to query the P. armeniaca protein database via HMMER 3.0 software4 with default parameters. Thereafter, the predicted AQP protein sequences were submitted to the National Center for Biotechnology Information (NCBI) Conserved Domain Database (CDD)5 (Marchler-Bauer et al., 2002, 2015) and SMART (Simple Modular Architecture Research Tool6) (Letunic et al., 2012) to confirm the presence and completeness of the MIP domain, with an E-value threshold of 1e–2.
Sequence Alignments and Phylogenetic and Synthetic Analyses
The PaAQP sequences were aligned with those of AtAQPs from A. thaliana7 (Johanson et al., 2001) and those of PpeAQPs from P. persica (Deshmukh et al., 2015) via the ClustalW program8 (Thompson et al., 1994). A phylogenetic tree was constructed by Molecular Evolutionary Genetics Analysis (MEGA) software 7.0 (Kumar et al., 2016; Qu et al., 2018) with the maximum likelihood method and 1,000 bootstrap resamplings. PaAQPs were named and confirmed by sequence homology and phylogenetic analyses. The syntenic relationships between the P. armeniaca, A. thaliana, and P. persica genomes were constructed by MCScan (Tang et al., 2008a,b, 2009) following the protocol for the Plant Genome Duplication Database (Lee et al., 2012). To identify the syntenic regions among P. armeniaca, initially, potential homologous gene pairs were detected using the BLASTP algorithm (E < 1e–5, top five matches) between every two genomes. Second, the BLASTP results and the gene location information were input into MCScanX software (Wang et al., 2012b). P. armeniaca whole genome/segmental and tandem duplications were subsequently identified. All syntenic relationship and gene location data were presented using TBtools software (Chen et al., 2018).
Structural Features of Putative PaAQPs
The molecular weight (Mw), isoelectric point (pI), and grand average of hydropathy (GRAVY) values were predicted via the ExPASy database (Swiss Institute of Bioinformatics, Lausanne, Switzerland) and ProtParam tool9 (Gasteiger et al., 2003). Transmembrane helical domains (TMHs) were assessed via TMHMM Sever 2.010 (Kong et al., 2017). The subcellular localization of the proteins was predicted using WoLF PSORT11 (Horton et al., 2007) and PlantmPLoc12 (Chou and Shen, 2010). Functional prediction was performed on the basis of two NPA motifs, the ar/R filter (H2, H5, LE1 and LE2), and Froger’s positions (P1-P5), from the multiple sequence alignment results from ClustalW.
Transient Expression of PaAQPs in A. thaliana Protoplasts
The GFP DNA fragment was amplified by PCR and subcloned into the pBI221 vector (Clontech, Mountain View, CA, United States). The coding regions of PaPIP1-1, PaPIP2-3, and PaSIP1-3 cDNA were amplified and fused in frame to the 5′ end of the GFP DNA sequence. The six PaAQP-GFP constructs and the GFP-only control construct were introduced into A. thaliana protoplasts via a polyethylene glycol-mediated method described previously with some modifications (Sheen, 2001). For plasma membrane localization, the membrane-specific dye FM4-64 was used to stain the transfected protoplasts (Nelson et al., 2007). The fluorescence of FM4-64 and GFP in the cells was analyzed with a 551-nm helium-neon laser and a 488-nm argon laser, respectively, via confocal scanning microscopy (TCS SP8, Leica, Germany; GFP excitation at 488 nm and emission at 505–525 nm; FM4-64 excitation at 551 nm and emission at 498–588 nm).
Exon-Intron Structure, 3D Structure, and Conserved Motif Distribution
The exon-intron organization was assessed via the Gene Structure Display Server13 based on annotated coding sequences and genome sequences. Conserved domains and motifs were analyzed with MEME14. The three-dimensional (3D) structures of the PaAQPs were generated from protein sequences submitted to the Phyre2 server15. The transmembrane helices and topology of the PaAQPs were analyzed via the MEMSAT-SVM prediction method on the Phyre2 server.
In silico Analysis of Promoter Sequences and Protein–Protein Interaction Analysis
The promoter sequences (2.0 kb upstream region from the transcription start site) were extracted from the genomic DNA sequences of the PaAQP genes. Putative cis-acting regulatory elements of the promoter sequences were identified with the program PlantCARE online16. The prediction of interacting networks of proteins was generated from the STRING database17.
cDNA-Library Preparation and Illumina Sequencing for Transcriptome Analysis
Total RNA was extracted from the ED, SP, and GS of the flower buds of two Youyi plants using a Plant RNA Extraction Kit (Autolab, China) following the manufacturer’s protocol. The concentration and quality of each RNA sample were determined using a NanoDrop 2000TM microvolume spectrophotometer (Thermo Scientific, Waltham, MA, United States) and gel electrophoresis. Poly(A) mRNA was isolated from 10 mg of total RNA using magnetic oligo (dT) beads and then divided into short fragments by fragmentation buffer (Ambion, Austin, TX, United States). First-strand cDNA was synthesized with random hexamer primers, and then second-strand cDNA was synthesized using DNA polymerase I (New England Biolabs), RNase H (Invitrogen), buffer, and dNTPs. Short DNA fragments were purified with a QIAquick PCR Purification Kit (Qiagen, Inc., Valencia, CA, United States), subjected to end repair and poly(A) addition and ligated to sequencing adaptors. Suitable fragments (350–450 bp) were purified by agarose gel electrophoresis and gathered by PCR amplification. Six cDNA libraries (ED, SP, and GS of the flower buds of two Youyi plants) were sequenced by using the Illumina HiSeqTM 2000 platform.
To facilitate assembly, the following criteria were used to filter low-quality reads:
1. Filter reads with adapter contamination.
2. Filter reads with unknown nucleotides >5%.
3. Filter reads in which more than 20% of bases showed a Q-value less than 20.
High-quality clean reads was mapped to the reference genome to calculate the expression level in Fragments Per Kilobase of transcript per Million fragments mapped (FPKM).
Yeast Two-Hybrid Assays
Yeast two-hybrid (Y2H) assays were performed using the Matchmaker Gold Yeast Two Hybrid System (Clontech). The coding regions of the PaAQP genes (Supplementary Table 1) and predicted interacting proteins (Supplementary Table 2) were cloned into pGBKT7 and pGADT7 vectors to create different baits and prey, respectively. The different pairs of bait and prey constructs were then cotransformed into yeast strain Gold Y2H, and yeast cells were grown on plates lacking leucine and tryptophan (SD/–Leu/–Trp) for 3 days. Transformed colonies were plated onto quadruple dropout medium (SD/–Leu/–Trp/–His/–Ade) containing 100 μM aureobasidin A and 3 mg mL–1 X-α-Gal at 30°C to test for interactions between PaAQPs and predicted interacting proteins.
Gene Expression Analysis and Quantitative Real-Time-PCR
To study the gene expression profiles of the PaAQPs in three periods of dormancy and germination in P. armeniaca ‘Youyi,’ RNA sequencing (RNA-seq) data were collected from the transcriptome sequencing data from flower buds collected during the ED stage, SP, and GS of the of two Youyi plants grown under the same conditions.
The bud and stem samples at each stage were collected according to Song et al. (2017). Flower buds were checked and did not burst under forced conditions on the sampling day of PD or ED period, and could burst under forced conditions on the sampling day of SP or GS period. When flower buds are in the SP period, flower buds are not covered by scales, and petals are not visible. While flower buds are in the GS period, they are not covered by scales, and petals are visible. The RNA-seq data of P. armeniaca ‘Youyi’ in this study were deposited in the NCBI Sequence Read Archive (SRA) database under number SRS1042411. On the basis of fragments per kilobase of transcript per million mapped reads (FPKM) values, heatmaps and hierarchical clusters were analyzed with HemI 1.018. Quantitative real-time (qRT)-PCR detection was chosen to analyze the expression of PaAQP genes in flower buds and stems of three P. armeniaca plants exhibiting similar growth during the PD stage, ED stage, SP, and GS. Total RNA was extracted from flower buds and stems using the RNeasy Plant Mini Kit (Qiagen, Valencia, CA, United States), and reverse transcription reactions were performed using Superscript II reverse transcriptase (Invitrogen, Grand Island, NY, United States) according to the manufacturer’s protocols. The primers used for qRT-PCR were designed using Primer Premier 5.0 software (Premier Biosoft Int., Palo Alto, CA, United States), and the specific primer sequences are listed in Supplementary Table 3. qRT-PCR was performed with an ABI Prism 7500 sequence detector (Applied Biosystems, Foster City, CA, United States) and the SYBR ® Premix Ex TaqTM Kit (TaKaRa, Tokyo, Japan) to measure transcript levels. PaElf was used as a reference gene in qRT-PCR detection. qRT-PCR analyses were performed in triplicate for each biological sample, and quantitative results were analyzed according to the 2–ΔΔCT method (Livak and Schmittgen, 2001).
Coexpression Network Analysis
The coexpression data for PaAQPs were obtained from RNA-Seq data (SRA database number SRS1042411). For P. armeniaca genome-wide coexpression network construction, transcriptome data from the flower buds of two P. armeniaca plants were used. Genes with Pearson correlation coefficients >0.876 were selected, and 1,500 genes were screened preliminarily. Among the 1,500 genes coexpressed with PaAQPs, 14 genes related to cold resistance and 16 genes associated with cold stress were selected to build the coexpression network of PaAQPs. A network diagram was generated according to Cytoscape software (The Cytoscape 1.1 Core was downloaded from19).
Yeast Transformants and Low-Temperature Treatment
The coding DNA sequences (CDSs) of PaPIP1-1, PaPIP1-3, PaPIP2-3, or PaTIP1-1 were inserted into the yeast expression vector pGAPZA. The recombinant vector pGAPZA:PaAQP was introduced into yeast strains. After identifying the positive colonies by PCR, the low-temperature treatment was conducted in solid and liquid Simmons Citrate-Ura (SC-U) media as described previously (Liu et al., 2015). Briefly, colonies of positive clones harboring the PaAQP gene or empty-vector (pGAPZA vector) were shaken and cultured at 200 rpm and 30°C for 24 h to induce PaAQP protein expression. A 1-mL aliquot of yeast culture liquid (containing the pGAPZA empty or pGAPZA:PaAQP recombinant vectors) was added to the strains under the low-temperature treatment (at an OD600 value of 1.0), which was then cultured at −20°C for 24 h. Afterward, the yeast liquid was diluted 1:10, 100, 1,000, and 10,000, followed by the sequential addition of 4 μL of the original yeast liquid and the diluted liquid to solid SC-U media (consisting of 2% galactose). After 48 h of culture at 30°C, the growth rate of the transformed yeast cells was observed and recorded. In addition, 1 mL of the aliquot yeast (containing the pGAPZA empty vector or pGAPZA:PaAQP recombinant vector) liquid at an OD600 value of 1.0 was removed and added to 10 mL of liquid SC-U medium (containing 2% galactose). After 24 h of −20°C treatment and 200 rpm at 30°C, the OD600 value of the transformed yeast cells was measured. A control group that was not stressed was also included.
Plant Transformation and Low-Temperature Resistance Analysis
The PaPIP1-3 and PaTIP1-1 CDSs were cloned into a pBI121 plant expression vector. The pBI121:PaPIP1-3 and pBI121:PaTIP1-1 recombinant plasmids were introduced into Agrobacterium tumefaciens (LBA4404). Transformation into Arabidopsis was carried out via the floral-dip method (Clough and Bent, 2010). To test the effect of cold stress on seedling growth, the seeds were allowed to germinate under normal growth conditions and then transferred to growth chambers at 22, 16, and 16°C 16 h/4°C 8 h. After 10 days of treatment, the physiological indexes of transgenic Arabidopsis and wild type plants were determined. Superoxide dismutase (SOD) activity (Wang et al., 2012a) and malondialdehyde (MDA) and proline levels were measured as previously described (Bates et al., 1973). Each sample comprised at least three individual plants, and all physiological analysis experiments were conducted in triplicate.
Statistical Analyses
The data concerning plant height, gene expression, OD600 values, etc., were assessed by one-way analysis of variance (ANOVA) followed by Tukey’s HSD post hoc analysis. All the tests were conducted in triplicate. In the figures, the lowercase letters indicate statistical significance based on one-way ANOVA (P < 0.05). Statistical analyses were performed with SPSS 16.0 software (SPSS, Inc., Chicago, IL, United States).
Results
Identification, Classification, and Properties of PaAQP Genes in P. armeniaca
Based on an extensive analysis of AQP-homologous sequences, a total of 33 putative full-length cDNA sequences of AQPs were identified from P. armeniaca. To investigate the phylogenetic relationship of AQP proteins among P. armeniaca, A. thaliana, and P. persica, a phylogenetic tree was constructed based on 33 protein sequences of AQP genes obtained from P. armeniaca, 35 sequences from A. thaliana, and 29 sequences from P. persica using the maximum likelihood method. The PaAQPs were classified into five subfamilies: NIPs (10 members), SIPs (4 members), TIPs (10 members), XIPs (2 members), and PIPs (7 members). The NIP subfamily included seven subgroups (one NIP1, one NIP2, two NIP3s, two NIP4s, two NIP5s, one NIP6, and one NIP7). The TIP subfamily was divided into five subgroups (three TIP1s, three TIP2s, two TIP3s, one TIP4, and one TIP5). The SIP subfamily was divided into two subgroups (three SIP1s and one SIP2s). The PIP subfamily was further clustered into three PIP1 and four PIP2 subgroups, and two members (XIP1s) belonged to the XIP subfamily (Figure 1A and Supplementary Table 1). All of these genes were grouped with A. thaliana and P. persica AQPs, confirming that they belonged to the AQP family.
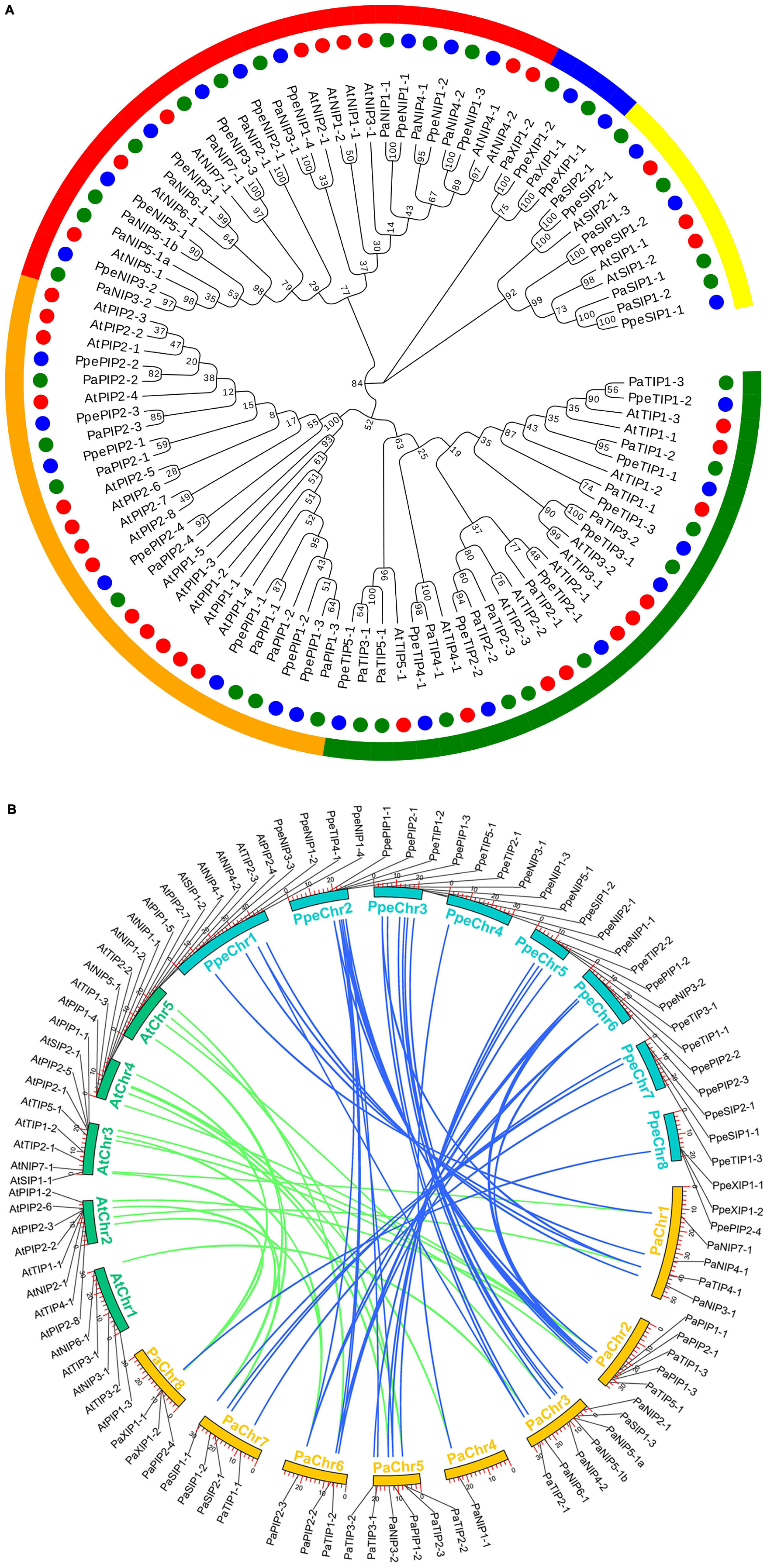
Figure 1. Phylogenetic relationships and microsynteny analyses of AQP genes among the P. armeniaca, A. thaliana, and P. persica genomes. (A) Deduced amino acid sequences were aligned using default parameters in ClustalW, and the phylogenetic tree was constructed via the maximum likely hood method with 1,000 bootstrap replicates with MEGA 7.0 software. Red, green, and blue circles represent the A. thaliana, P. armeniaca, and P. persica AQP gene family members. Yellow, blue, red, orange, and green arches represent the SIP, XIP, NIP, PIP, and TIP AQP gene family subgroups of A. thaliana, P. armeniaca, and P. persica. The scale bar indicates the distance calculated by way of multiple alignment. (B) The chromosome numbers of all three species are specified by different colors: blue, green, and yellow represent the P. armeniaca, A. thaliana, and P. persica chromosomes, respectively. The chromosome number is indicated on the inside with the chromosome sequence lengths in megabases. Gene pairs with syntenic relationships are linked by blue and green lines representing the microsyntenic regions between the P. armeniaca and A. thaliana chromosomes and the P. armeniaca and P. persica chromosomes, respectively.
Synteny analysis was carried out using MCScanX software, and syntenic relationships and whole genome sequences to visualize the locations of orthologous genes were presented using TBtools software. Microsynteny analysis was performed across the P. armeniaca, A. thaliana, and P. persica genomes. Between P. armeniaca and A. thaliana, 14 collinear blocks were identified, while 22 orthologous gene pairs were found (Figure 1B). Between P. armeniaca and P. persica, 30 collinear blocks were identified, with 41 orthologous gene pairs (Figure 1B). These results suggest that P. armeniaca and P. persica are more closely related than P. armeniaca and A. thaliana.
Bioinformatics analysis, CDS and amino acid sequence analysis, and ExPaSy database analysis showed that the Mw of the PaAQPs ranged from 21.94 to 35.37 kDa and that their pIs ranged from 5.32 to 10.00 (Table 1). The SIPs and TIPs were smaller (<28 kDa) than the NIPs, XIPs, and PIPs. The TIPs were acidic (except for PaTIP2-3), while the majority of the other subfamilies were alkaline. Most of the AQPs were predicted to have TMHs, while PaTIP2-2 and PaTIP2-3 had only five, PaNIP3-1 had seven, and PaNIP5-1b had eight TMHs (Table 1). The GRAVY scores of the PaAQP proteins ranged from 0.291 to 0.990, and among the subfamilies, the PIPs presented the lowest average GRAVY value (0.409) (Table 1).
Chromosomal Distribution, Gene Structure, and Microsynteny Analysis
The PaAQPs showed less variation in transcript length (ranging from 657 to 987 bp) than in gene length (ranging from 1075 to 12,213 bp). The number of amino acids in the identified PaAQPs ranged from 219 (PaTIP2-2) to 329 (PaNIP2-1) (Table 1). The 33 identified PaAQPs were unevenly mapped on the eight chromosomes of P. armeniaca. Chromosome 3 contained the largest number (7; 21.21%) of PaAQP genes, followed by chromosomes 5 and 2, which contained six members (18.18%) and five members (15.15%), respectively. Both chromosomes 1 and 7 contained four members (12.12%). Chromosome 8 contained three members (9.09%), and chromosome 4 contained only one member (3.03%) (Supplementary Figure 1).
Gene duplication has long been considered one of the main forces in the evolution and expansion of a gene family (Crow and Wagner, 2006). Therefore, we investigated the different types of gene duplications in the PaAQP gene family. We found that 6 AQP genes clustered in 3 tandem repeat event regions in the P. armeniaca genome (Supplementary Figure 1). There were three pairs of tandem duplicated genes (PaNIP5-1a and PaNIP5-1b, PaTIP2-2 and PaTIP2-3, PaXIP1-1, and PaXIP1-2) within the 1 Mb chromosomal region of chromosomes 3, 5, and 8. In addition, 6 pairs of WGD/segmental duplication genes (PaTIP4-1 and PaTIP2-1, PaTIP1-2 and PaTIP1-3, PaSIP1-1, and PaSIP1-2, triangle WGD/segmental duplication among PaPIP2-1, PaPIP2-2, and PaPIP2-3) were found within the PaAQP family (Supplementary Figure 1). Notably, all the identified segmental duplication gene pairs were distributed on different chromosomes in the P. armeniaca genome. However, the distribution of segmental replication genes on the chromosomes was uneven; there were three genes on chromosome 6, two on chromosomes 2 and 7, and only one on chromosome 1. Moreover, we also found that PaPIP2-1 was the common duplicated gene between PaPIP2-2 and PaPIP2-3. This particular gene replication event involved three genes: PaPIP2-1, which was located on chromosome 2, and PaPIP2-2 and PaPIP2-3 on chromosome 6. Additionally, the analysis revealed one particular WGD/segmental duplication event between chromosomes 2 and 6 involving four genes. PaPIP2-1 combined with PaTIP1-3 was reverse duplicated to PaPIP2-2 combined with PaTIP1-2.
Exon-intron organization plays key roles in phylogenetic relationships and plant evolution (Wang et al., 2014; Jue et al., 2015). As shown in Supplementary Figure 2, the distribution of introns in the PaAQP genes ranged from one to five introns per gene. All PaPIPs presented four exons: PaXIP1-1 had two exons, and PaXIP1-2 had three exons. The majority of PaSIPs exhibited three exons; an exception involved PaSIP1-3, which had only one exon and was therefore intronless. Most PaTIPs had three exons, while PaTIP1-1 had two and PaTIP2-3 had five. A relatively diverse exon-intron pattern was detected for the PaNIP subfamily. The majority of PaNIPs had five exons, with the exceptions of PaNIP3-2, which had six exons, PaNIP5-1a, which had four exons, and PaNIP3-1, which had three exons. Exon-intron organization analysis revealed a conserved pattern of gene structure within the subfamilies of PaAQPs. The conserved exon-intron structure supports the phylogenetic relationships of P. armeniaca (Supplementary Figure 2).
Characterization of Asn-Pro-Ala Motifs, Transmembrane Domains, and Conserved Motifs of PaAQPs
To better understand the possible physiological mechanisms of the PaAQPs, all 33 sequences were aligned, and the conserved residues (NPA motifs, ar/R selectivity filter, and Froger’s position) were analyzed (Figure 2, Table 2, and Supplementary Figures 3–6). The P. armeniaca PaAQPs displayed differences in NPA motifs and residues at the ar/R selectivity filters and Froger’s positions compared with those of other plant species. Most of the AQPs showed dual NPA motifs, with the exception of PaSIP2-1, which was found to harbor a single NPA motif. The majority of members from the PIP and TIP subfamilies contained a typical NPA motif, as observed for the Beta vulgaris counterpart (Kong et al., 2017), with the exception of PaTIP2-3, which showed an alanine (A)-to-serine (S) substitution in the first NPA motif and asparagine (N)-to-A and A-to-lysine (K) substitutions in the secondary NPA motif. The LPK (PaTIP2-3) motifs detected in P. armeniaca have not been reported in any other plant species. These changes may alter the substrate specificity of the AQPs in P. armeniaca. In the NIP subfamily, some members of the NIPs, such as PaNIP5-1a, PaNIP3-2, PaNIP5-1b, and PaNIP6-1, showed replacement of alanine (A) at the third residue of the first NPA motif by serine (S) and replacement of other alanine (A) residues by isoleucine (I) or valine (V). In the XIP subfamily, the first NPA motif showed an N-to-S substitution in PaXIP1-1 and a valine (V)-to-A substitution in PaXIP1-2, while the second NPA motif was conserved. All PIP subfamily members showed conserved ar/R filter residues, with phenylalanine (F) at H2, histidine (H) at H5, threonine (T) at LE1, and arginine (R) at LE2, which is typical of water-transporting AQPs. They also showed Q/E-S-A-F-W residues at Froger’s positions, as observed in species such as flax (Linum usitatissimum) (Shivaraj et al., 2017) and chickpea (Cicer arietinum L.) (Deokar and Bunyamin, 2016). In the TIP subfamily, the H2 position of the ar/R filter contained histidine (H), and the H5 position contained isoleucine (I), except in PaTIP1-1, where this position was occupied by valine (V), and PaTIP2-3, which did not exhibit a residue at this position. The LE1 and LE2 positions were found to be specific to each subgroup of PaTIP. The PaTIP1 subgroup was characterized by alanine (LE1) and valine (LE2) levels. The PaTIP2 subgroup was characterized by glycine (LE1) and arginine (LE2), except for PaTIP2-2, which contained glycine (LE2), and PaTIP2-3, which contained alanine (LE1) and leucine (LE2). The PaTIP3 and PaTIP4 subgroups were characterized by alanine (LE1) and arginine (LE2), with the exception of PaTIP3-1, which contained glycine (LE1) and cystine (LE2). PaTIP5 was also characterized by glycine (LE1) and cystine (LE2) and showed a conserved relationship with chickpea (Deokar and Bunyamin, 2016) and flax (Shivaraj et al., 2017). Among the NIPs, the ar/R selectivity filter and Froger’s positions presented multiple types. PaNIP1-1, PaNIP3-1, PaNIP4-1, and PaNIP4-2 showed W-R-A-F/V/I in the ar/R selectivity filter and F/L-S-A-Y-I/T/F residues at Froger’s positions, and PaNIP5-1a and PaNIP5-1b showed S-R/Q-G-V in the ar/R selectivity filter and F-T-A-Y-L residues at Froger’s positions. PaNIP2-1, PaNIP3-2, PaNIP6-1, and PaNIP7-1 showed G/A/T-R-G/A-F/I in the ar/R selectivity filter. The SIP family members showed A-V-P-N residues in the ar/R selectivity filter and M-A-A-Y-W at Froger’s positions, with the exception of PaSIP1-3, which contained isoleucine (H2), and PaSIP2-1, which showed T/H/G/S in the ar/R selectivity filter and F-V-A-Y-W at Froger’s positions. The ar/R selectivity filter of PaXIP1-1 and PaXIP1-2 was I/V/V/R, and Froger’s positions consisted of V-C-A-F-W, showing a conserved relationship with flax (Shivaraj et al., 2017).
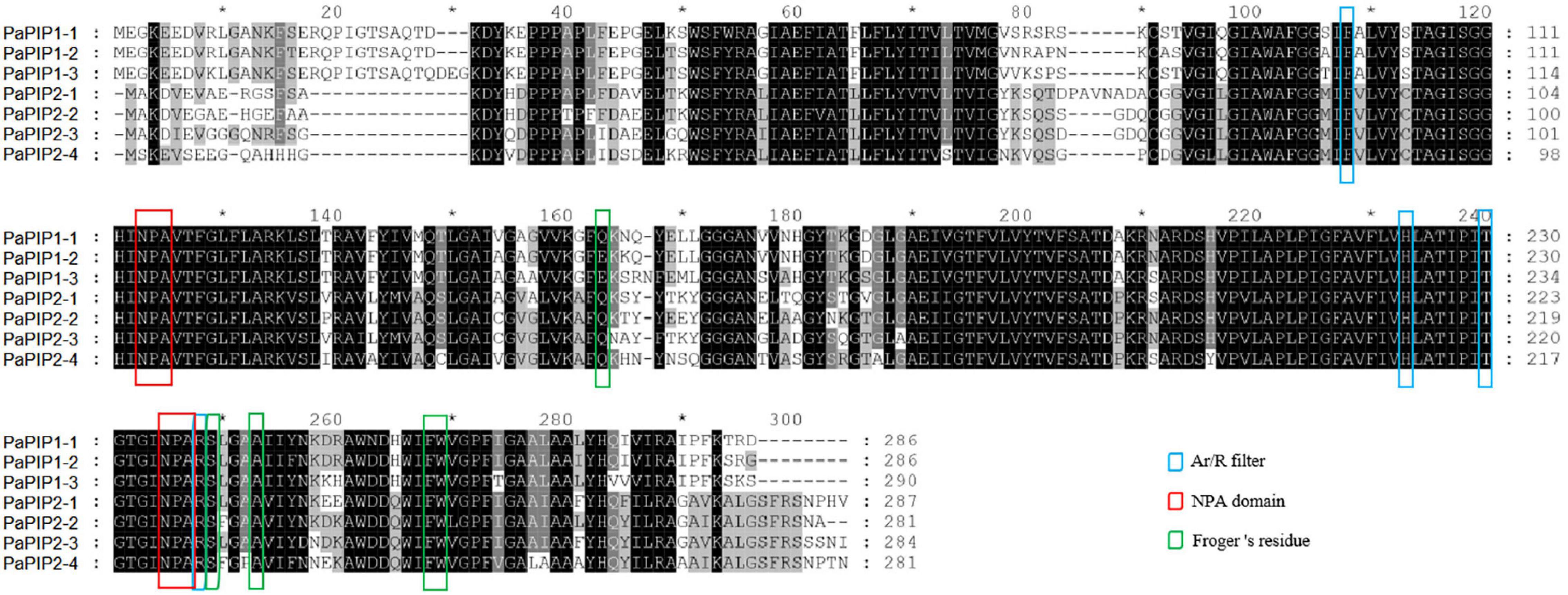
Figure 2. Multiple sequence alignment of the deduced amino acid sequences of PaPIPs. Amino acids at NPA domains, ar/R selectivity filters, and Froger’s residues were identified in seven PIP family members (3 PIP1s and 4 PIP2s) in P. armeniaca.
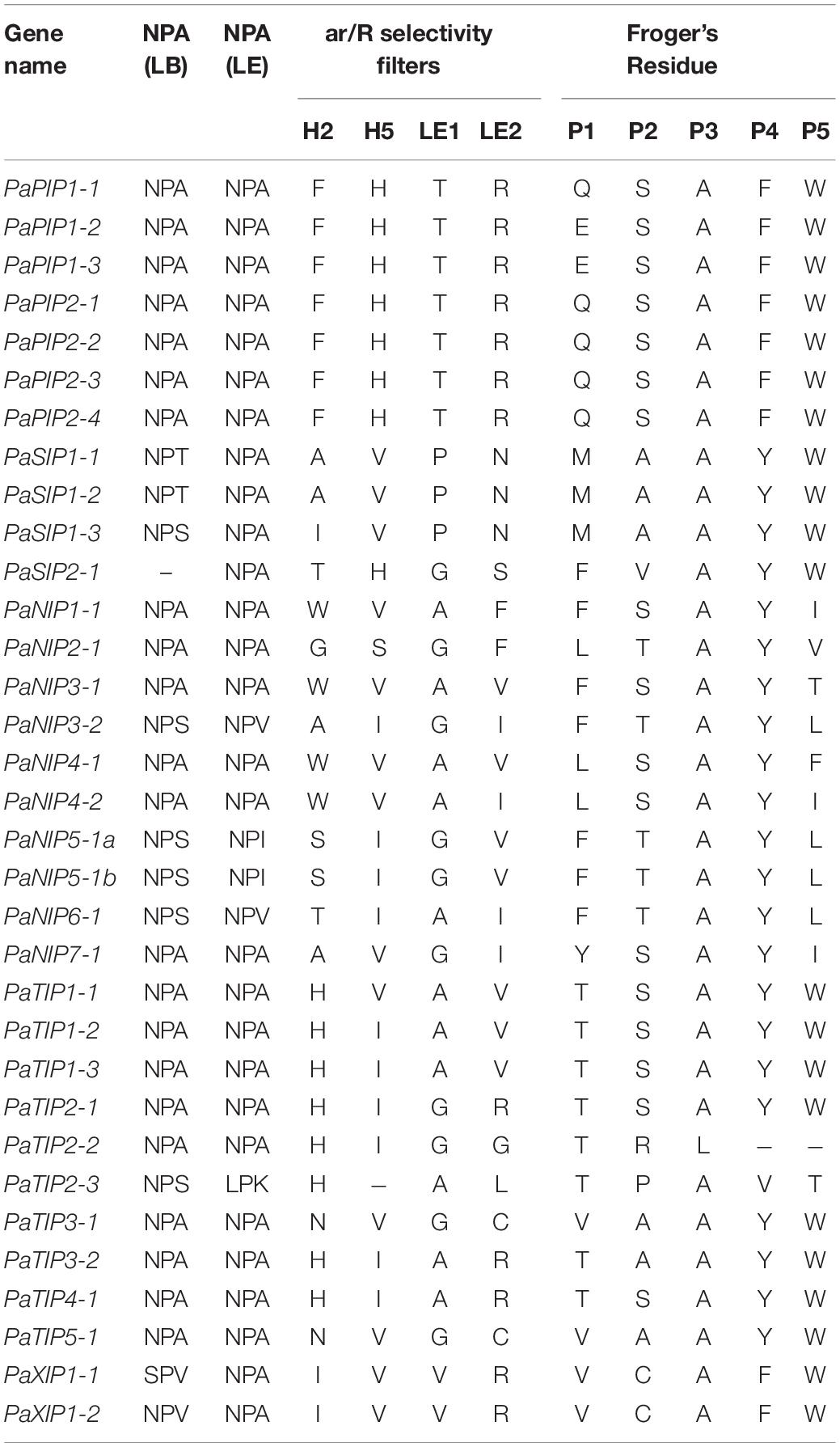
Table 2. Amino acid composition of the NPA motifs, ar/R selectivity filter, and Froger’s residues of PaAQP.
To further analyze the conservation, diversity, and relationship in each subfamily, motif scan analysis was conducted via the MEME program. Seventeen conserved motifs were identified in the PaAQP family, and most of the members in the same subfamily shared a similar number of motifs (Supplementary Figure 7). For example, all the members of PaPIPs had thirteen motifs, except for PaPIP1-2. All the members of PaTIP1s had eleven motifs, all the members of PaSIP1s had nine motifs, and all the members of PaNIP4s had eleven motifs. The results also showed that PaAQPs had similar functional motifs (motif 2) consisting of highly conserved regions (channel proteins of the major intrinsic protein (MIP) superfamily) with motif 4 from B. vulgaris BvAQP proteins. These results implied that PaAQPs may have a similar function to AQPs from other plants.
Promoter Cis-Element Analysis
Promoter sequence analysis indicated that the PaAQP genes contained several development-related, stress-, and hormone-responsive cis-acting regulatory elements, including low-temperature-responsive elements (LTEs), light-responsive elements (GT1s, MREs, TCT-motifs and G-boxes), auxin-responsive elements (TGAs and AuxRR-cores), abscisic acid (ABA)-response elements (ABREs), dehydration-responsive elements (MBS), endosperm expression elements (AACA motifs and GCN4 motifs), and wound-responsive elements (WUN motifs) (Supplementary Figure 8 and Supplementary Table 4). Among the stress-related cis-acting elements, all PaAQPs (33 genes) exhibited AREs (essential for anaerobic induction). There were 17 low temperature-responsive (LTR) and 16 MBS (drought inducible) elements within 14 and 12 PaAQP promoters, respectively. This finding indicated that AQP proteins may play an important functional role in the stress response and tolerance in P. armeniaca. Among the hormone-related cis-acting elements, 76 ABREs (ABA responsive), 38 CGTCA motifs (methyl jasmonate (MeJA) responsive), and 37 TGACG motifs (MeJA responsive) were identified in the promoters of 23, 22, and 21 PaAQPs, respectively. Furthermore, large numbers of elements related to light responsiveness, zein metabolism regulation (O2 sites), meristem expression (CAT-boxes) and some elements related to flavonoid biosynthesis (MBSIs) and endosperm expression were detected, implying that PaAQPs might participate in developmental processes in P. armeniaca.
Molecular Modeling of PaAQP
3D proteins of plant AQPs can be effectively used for understanding the substrate specificity and solute permeation rate of AQP genes, which will help to improve the understanding of the cold resistance of PaAQPs (Groot and Grubmüller, 2005). The structural properties of all 33 PaAQPs were displayed in homology-based tertiary (3D) protein models, which were predicted via the Phyre2 server display, and the results are shown in Supplementary Table 5. All 3D protein models were constructed with 100% confidence, and the residue coverage varied from 68 to 98%. The PaAQP 3D protein model contained a conserved hourglass-like structure consisting of an a-helical bundle forming five to eight TM helices (H1 to H8) and two short helices (HE and HB). Loops HE and HB were located close to each in the center of the membrane. These predicted 3D models showed the boron (B) and silicon (Si) substrate selectivity of the PaAQPs and provided an important basis for the protein functional analysis of the AQP genes of P. armeniaca (Deshmukh et al., 2013; Tombuloglu et al., 2016).
Protein–Protein Interaction Network of PaAQPs
To further reveal the function of PaAQPs during the interaction with other proteins, PaTIP1-1, PaPIP1-3, PaSIP1-3, and PaNIP6-1, which showed high expression in each subfamily (Figure 3), were used to construct a protein-protein interaction network. As shown in Figures 4A–D and Supplementary Table 6, none of these tested PaAQPs shared the same interacting proteins. In addition, various proteins specifically interacted with TIP separately, such as zinc finger proteins, calcium-dependent protein kinases, and NAC domain-containing proteins. In particular, the interacting zinc finger proteins and NAC transcription factors can enhance cold tolerance in transgenic plants (Jung et al., 2013). Interestingly, TIP2 could interact with TIP and form homodimers. The proteins that interact with PIP include AGD2-like defense response protein, methylenetetrahydrofolate reductase family proteins, and Delta 1-pyrroline 1–5-carboxylate reductase. Specifically, SIP and NIP members could interact with each other. For example, NIP6-1 specifically interacted with SIP1-3, SIP2-1, and SIP1A, while four NIP members (NIP1-2, NIP2-1, NIP4-1, and NIP6-1) and three PIP members (PIP1B, PIP1B, and PIP2-5) could interact with SIP1A. Notably, NIP6-1 specifically interacted with WRKY6, which plays important roles in cold resistance, stress tolerance, growth and development (Wang et al., 2014). Y2H assays showed that yeast cells cotransformed with constructs PaNIP6-1-pGBKT7 and WRKY6-pGADT7, PaTIP1-1-pGBKT7 and TSPO-pGADT7, and PaPIP2-1-pGBKT7 and ATHATPLC1G-pGADT7 could support growth in the selection medium and b-galactosidase staining (Figure 4E). Thus, Y2H assays revealed the protein interaction between PaNIP6-1 and WRKY6, PaTIP1-1 and TSPO, and PaPIP2-1 and ATHATPLC1G. In particular, the A. thaliana gene encoding a phosphatidylinositol-specific phospholipase C AtPLC1 (ATHATPLC1G) was induced by low-temperature, dehydration, and salt stress, which might contribute to the adaptation of the plant to cold stresses (Hirayama et al., 1995).
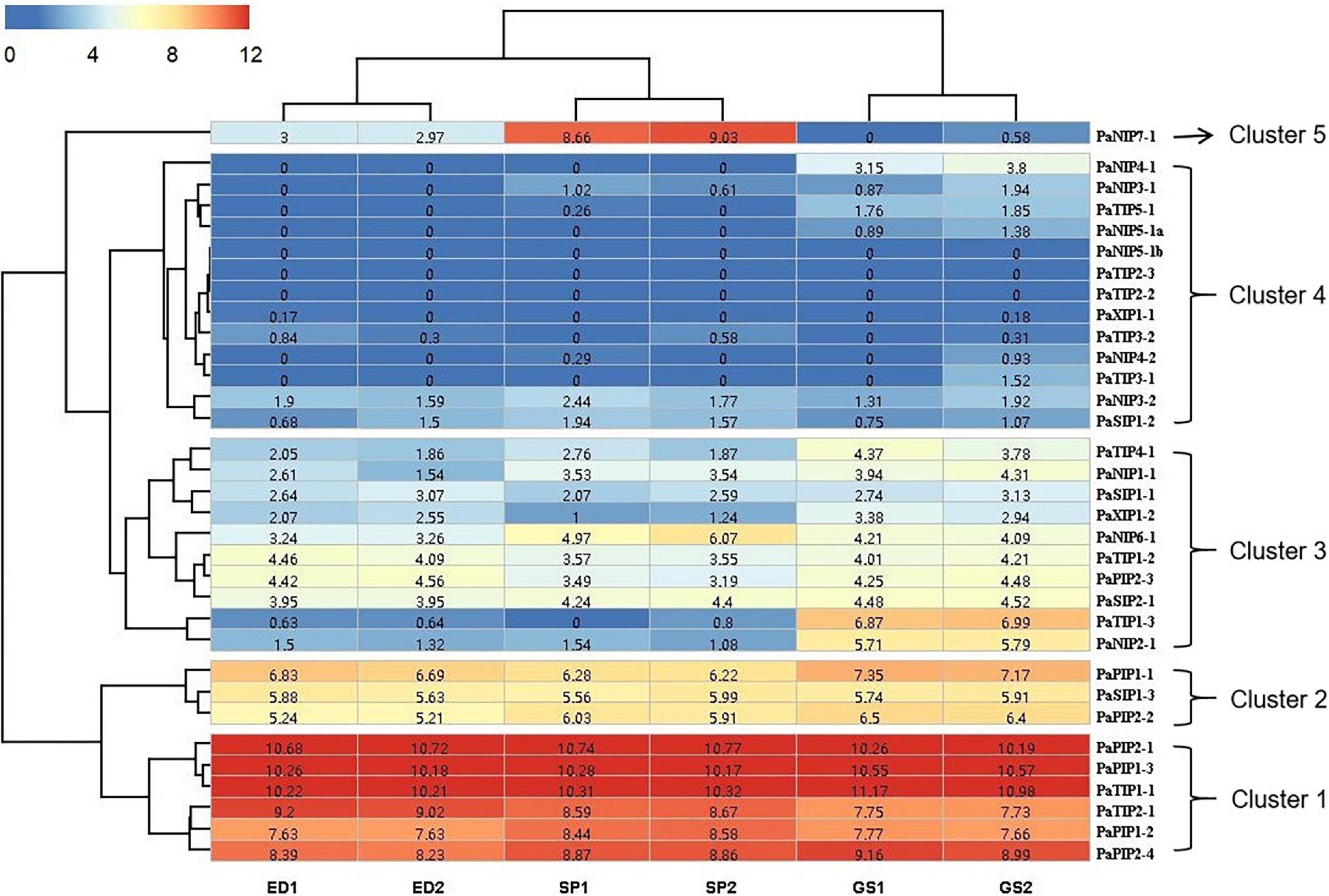
Figure 3. Expression profiles of PaAQPs at different developmental stages based on RNA-seq data. ED, SP, and GS indicate that the tested materials were collected during ecological dormancy, the sprouting period, and germination stage of P. armeniaca, respectively. The number in the middle of each box represents the FPKM.
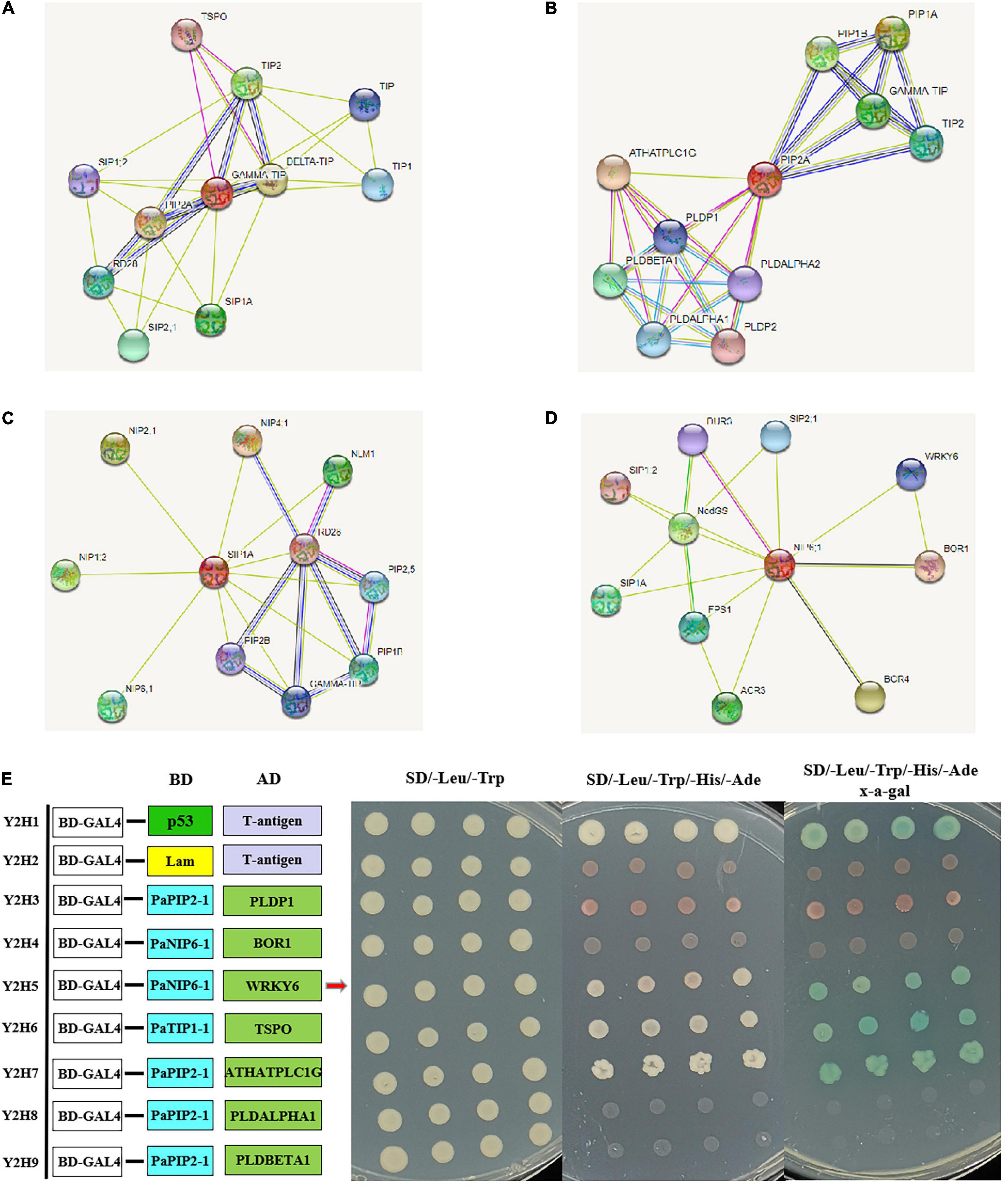
Figure 4. Prediction of the interaction network of PaAQP proteins based on the interactions of their orthologs in A. thaliana. (A) The interaction network of TIP. (B) The interaction network of PIP. (C) The interaction network of SIP. (D) The interaction network of NIP. (E) Y2H assays showing the protein interaction between PaNIP6-1 and WRKY6, PaTIP1-1 and TSPO, and PaPIP2-1 and ATHATPLC1G. The networks were generated from the STRING database. The red circles represent the queried protein, while the other circles are the interacting proteins. The annotation of the predicted interacting protein was derived from UniProtKB (https://www.uniprot.org/). The line thickness indicates the strength of the data support.
Expression Profiling of PaAQPs in the Dormancy and Sprouting Stages of P. armeniaca
To gain more information about the potential role of PaAQP proteins in P. armeniaca, we analyzed the expression of PaAQPs in the ED, SP, and GS by transcriptome sequencing. The RNA-seq data can be found in the NCBI SRA database under number SRS1042411. The expression levels of 33 PaAQP genes were clustered in a heatmap (Figure 3). We observed the expression of 30 PaAQPs (90.90%) in at least one of the developmental stages, and all of these PaAQPs showed differential expression in three stages of dormancy and sprouting periods. Among these genes, 23 PaAQP genes (75%) were expressed in all three periods of dormancy and germination, among which PaPIP2-1, PaPIP1-3, and PaTIP1-1 exhibited the greatest expression levels in different periods. In addition, PaTIP1-2 showed greater expression in the ED period than in the SP and GS periods, which may suggest that the P. armeniaca plant was in the ED period. Similarly, PaNIP7-1 showed greater expression in the SP period than in the ED and GS periods and may be useful as a marker gene of entry into the SP. PaTIP1-3 and PaNIP2-1 exhibited greater expression levels in the GS than in the ED and SP, so these two genes might be useful for suggesting that the P. armeniaca plant is entering the GS. Interestingly, PaNIP5-1b, PaTIP2-3, and PaTIP2-2 were not expressed in any of the three periods of dormancy and germination. In addition to these three genes, PaNIP3-1, PaNIP4-1, PaNIP4-2, PaNIP5-1a, PaTIP3-1, and PaTIP5-1 were not expressed in the ED period of flower buds; and PaNIP5-1a, PaNIP4-1, and PaTIP3-1 were not expressed in the SP of flower buds.
The heatmap demonstrated the clustering of four main clades. The PaAQP genes from clade 1 corresponded to 2 TIP members (PaTIP1-1 and PaTIP2-1) and 4 PIP members (PaPIP2-1, PaPIP1-3, PaPIP1-2, and PaPIP2-4) displaying the greatest expression in three periods of dormancy and germination. The genes from clade 2 included 2 PIP members (PaPIP1-1 and PaPIP2-2) and 1 SIP member (PaSIP1-3) that exhibited relatively high expression in these three periods, while those from clade 3 included 3 NIP members (PaNIP1-1, PaNIP2-1, and PaNIP6-1), 3 TIP members (PaTIP1-2, PaTIP1-3, and PaTIP4-1), 2 SIP members (PaSIP1-1 and PaSIP2-1), 1 PIP member (PaPIP2-3), and 1 XIP member (PaXIP1-2) showing an intermediate level of expression, and clade 4 included 7 NIP members (PaNIP3-1, PaNIP3-2, PaNIP4-1, PaNIP4-2, PaNIP5-1a, PaNIP5-1b, and PaNIP7-1), 5 TIP members (PaTIP2-2, PaTIP2-3, PaTIP3-1, PaTIP3-2, and PaTIP5-1), 1 SIP member (PaSIP1-2), and 1 XIP member (PaXIP1-1) displaying the lowest expression in three developmental periods of flower buds.
Expression Patterns of PaAQP Genes in Different Tissues During the Dormancy and Sprouting Stages of P. armeniaca
To gain insight into potential functions, qRT-PCR was employed to determine the expression patterns of PaAQP genes in two tissues: flower buds and stems (Figure 5 and Supplementary Figure 9). All 20 key PaAQP genes were expressed in two tested tissues with different expression patterns. Nine genes (PaPIP2-3, PaSIP1-1, PaSIP1-3, PaSIP2-1, PaTIP1-2, PaNIP1-1, PaNIP3-2, PaXIP1-2, and PaTIP4-1) with stable expression patterns in all stages of dormancy and germination, five genes (PaPIP1-1, PaPIP2-2, PaNIP2-1, PaNIP6-1, and PaTIP1-3) with differential expression patterns, three highly expressed genes (PaTIP1-1, PaTIP2-1, and PaPIP2-4), and three genes with low expression (PaNIP3-1, PaTIP5-1, and PaSIP1-2) were selected for qRT-PCR analysis. The qPCR results for the 20 selected PaAQPs were generally consistent with the transcript-level changes determined by RNA-seq analysis in the dormancy and sprouting stages of flower buds, suggesting that the transcriptomic profiling data were likely reliable. However, moderate discrepancies in the transcript levels were detected in two PaAQPs compared with the RNA-seq data: PaPIP2-3 and PaSIP1-3 (Figure 5). The expression of PaPIP2-3 and PaSIP1-3 in the stems showed almost the same trends as in the flower buds in the four examined stages of P. armeniaca. PaPIP1-1, PaPIP2-2, PaPIP2-3, and PaSIP1-1 showed the greatest expression during PD in the stem, and PaPIP2-4, PaSIP2-1, PaXIP1-2, PaNIP1-1, PaNIP2-1, PaNIP3-1, PaTIP1-1, PaTIP1-3, PaTIP4-1, and PaTIP5-1 showed the greatest expression in the GS in the flower buds (Figure 5). These results suggested that the differential expression of PaAQP members during the dormancy and sprouting stages in different tissues could predict the different biological functions of these genes.
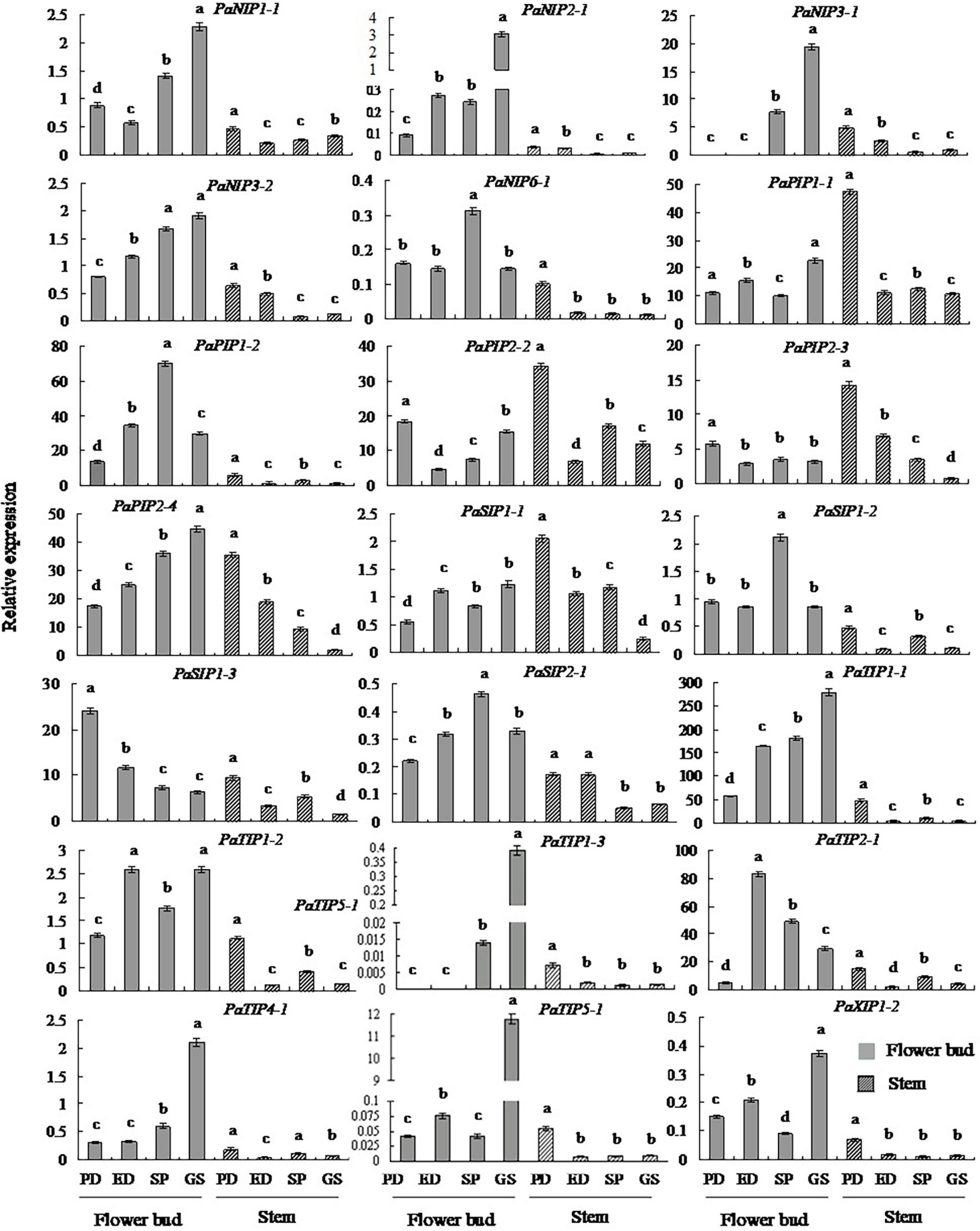
Figure 5. Changes in the transcript levels of 20 selected genes in different tissues during the dormancy and sprouting stages of P. armeniaca. The stem and flower buds were collected during the PD stage, ED stage, SP stage, and GS stage of P. armeniaca plants. All expression levels of PaAQP genes were normalized to the expression levels of PaElf (elongation factor-1α). The 2–ΔΔCT method was used to analyze the relative gene expression. The data are means of three replicates ± SDs. The lowercase letters indicate statistical significance based on one-way ANOVA with Tukey’s HSD post hoc analysis.
Coexpression Network of PaAQPs in P. armeniaca
To elucidate the molecular mechanism of PaAQPs in the dormancy and sprouting stages of P. armeniaca, a PaAQP coexpression network was constructed (Figure 6A and Supplementary Table 7). A total of 1,500 genes were identified as coexpressed with PaAQPs. Among these, 14 genes (0.93%) were associated with cold resistance. For example, C3HC4-type RING zinc finger proteins are involved in cold stress (Jung et al., 2013). MYB transgenic rice enhances tolerance to chilling stress and mediates ectopic expression of stress genes (Ma et al., 2009). bZIP transcription factors regulate freezing tolerance in Arabidopsis (Yao et al., 2020). Additionally, overexpression of the NAC transcription factor enhances the cold tolerance of Medicago truncatula (Qu et al., 2016). Serine/threonine protein kinases are involved in the cold stress response in the cyanobacterium Synechocystis (Zorina et al., 2014). In our research, zinc finger proteins, MYBs, bZIPs, NACs, and serine/threonine protein kinases, among others, were highly coexpressed with PaAQPs in different tissues during the dormancy and sprouting stages of P. armeniaca and may be involved in cold resistance in P. armeniaca.
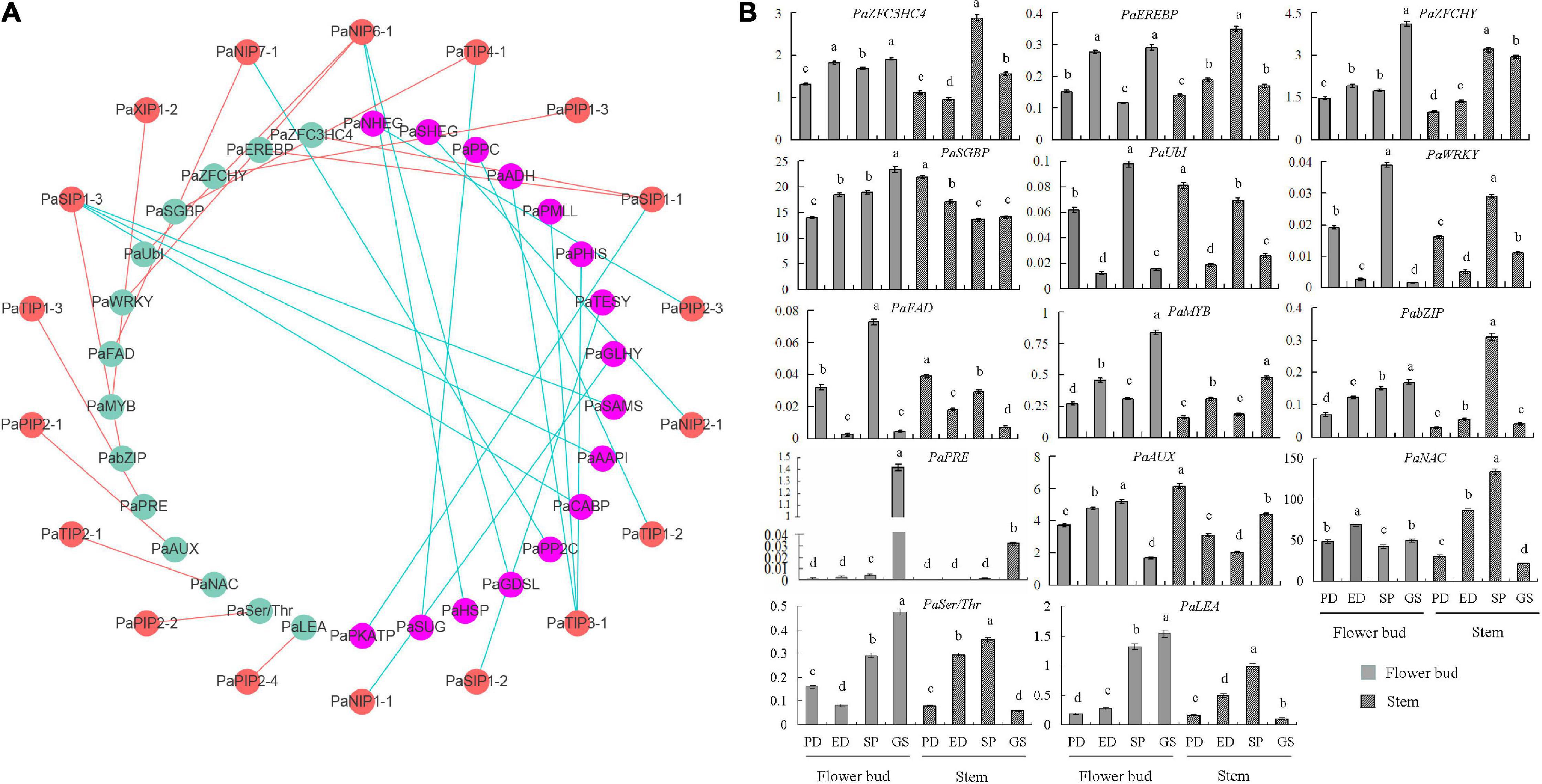
Figure 6. PaAQP coexpression network. (A) Among the 1,500 genes coexpressed with PaAQPs, 14 (green nodes) are involved in cold resistance based on previous research. In addition, the anticold ability of 16 genes (pink nodes) is not very clear. The red node is the PaAQP genes. Cyan lines represent PaAQPs coexpressed with 14 cold resistance genes, and red lines represent PaAQPs coexpressed with 16 cold stress-associated genes. (B) Transcript levels of 14 genes involved in cold resistance in stem and flower buds during the PD stage, ED stage, SP stage, and GS stage of P. armeniaca. All expression levels of the PaAQP genes were normalized to those of PaElf. Each bar represents the mean ± SD of three technical replicates. The different letters represent significant differences at P < 0.05 (one-way ANOVA).
To validate the network constructed by the core PaAQPs, changes in the expression of the 14 genes related to cold resistance in the PaAQP coexpression network were detected in the PD, ED, SP, and GS of flower buds and stems of P. armeniaca plants (Figure 6B). Among the 14 pairs of coexpressed genes, the expression trends of PaSIP1-1 and PaZFC3HC4, PaSIP1-1 and PaEREBP were consistent in the four periods (PD, ED, SP, and GS) of flower buds of P. armeniaca, whereas those of PaTIP4-1 and PaSGBP were rarely consistent. Similarly, the expression was similar between PaNIP6-1 and PaUbI and between PaNIP6-1 and PaWRKY. Additionally, three pairs exhibited no differences in expression patterns: PaXIP1-2 and PaMYB, PaTIP1-3 and PaPRE, PaPIP2-4 and PaLEA.
Subcellular Localization of PaPIP1-1, PaPIP2-3, PaSIP1-3, PaXIP1-2, PaNIP6-1, and PaTIP1-1 Proteins
WoLF PSORT and Plant-mPLoc prediction were used to predict the subcellular localization of the PaAQPs. Almost all members of the PaPIP subfamilies were localized to the plasma membrane according to WoLF PSORT and Plant-mPLoc prediction (Table 1). However, the subcellular localizations predicted by Plant-mPLoc indicated that PaPIP2-2 was localized to the cell wall. The PaTIPs were predicted to localize to the vacuoles, and PaNIPs (except for PaNIP5-1a),PaSIPs, and PaXIPs were predicted to localize to the plasma membrane according to Plant-mPLoc prediction. However, the results predicted by WoLF PSORT were diverse and included localization to the nucleus, Golgi, cytosol, mitochondrion, chloroplast, and endoplasmic reticulum (Table 1). Genes with high expression in different periods of low temperature may play important roles in cold resistance during the dormancy and sprouting stages of P. armeniaca. To identify and gain insight into the subcellular localization of these highly expressed PaAQP genes in plant cells, chimeric EGFP-PaPIP1-1, EGFP-PaPIP2-3, EGFP-PaSIP1-3, EGFP-PaXIP1-2, EGFP-PaNIP6-1, and EGFP-PaTIP1-1 cDNAs (representing genes with the greatest expression in each subgroup in flower buds in the dormancy and germination of P. armeniaca) were constructed and inserted downstream of the CaMV35S promoter. Transient expression analyses were performed using A. thaliana leaf mesophyll cells.
Analyses of fusion protein localization by confocal microscopy showed that the green fluorescence of these proteins was confined to the plasma membrane (Figure 7). This membrane localization of the PaPIP1-1, PaPIP2-3, and PaSIP1-3 proteins was consistent with the location of PIP and SIP gene expression in other plants (Zou et al., 2015; Deokar and Bunyamin, 2016).
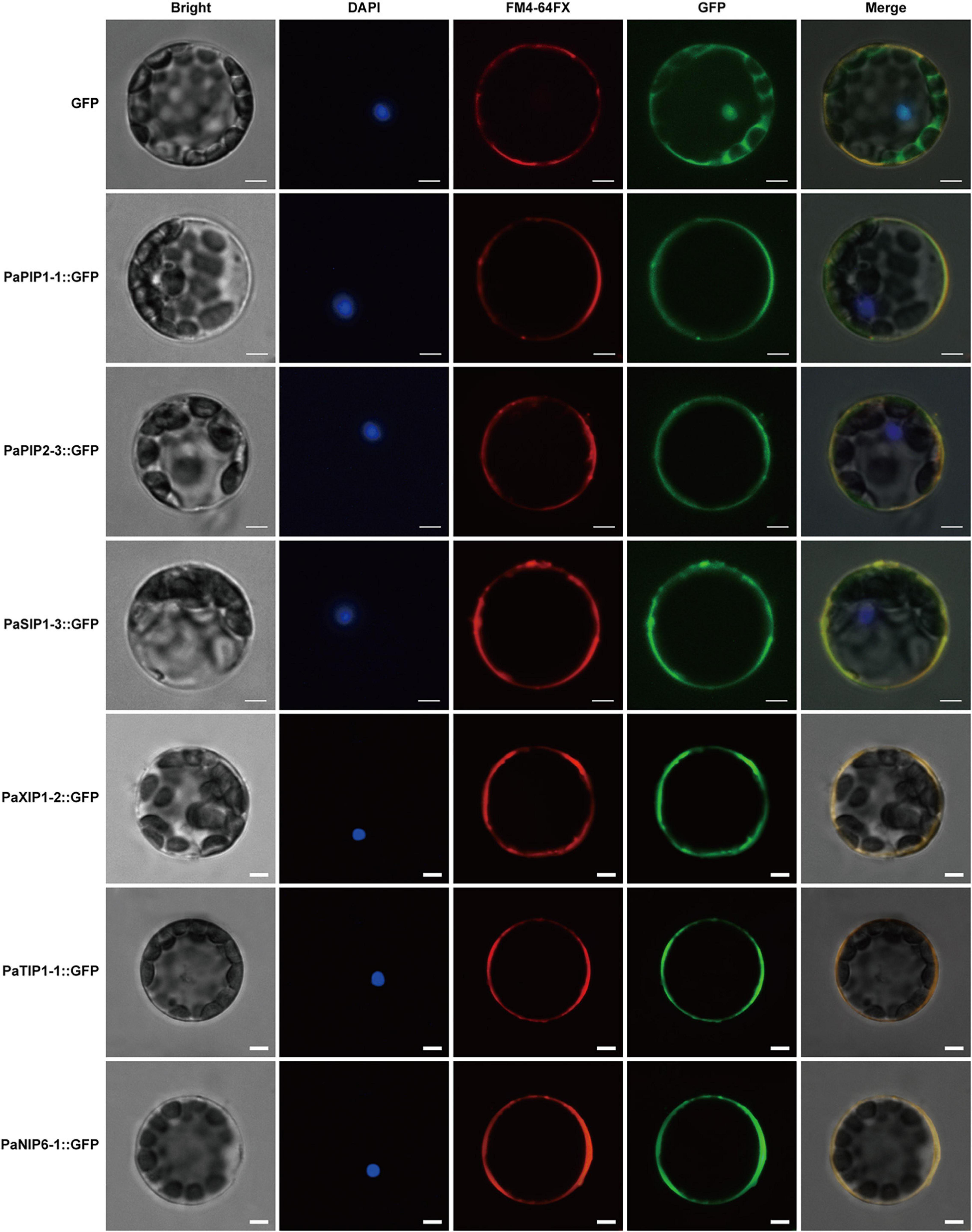
Figure 7. Localization of PaPIP1-1, PaPIP2-3, PaSIP1-3, PaXIP1-2, PaNIP6-1, and PaTIP1-1 in the cell plasma membrane. Bright, Bright-field images. DAPI, nuclei counterstained with 4′,6-diamidino-2-phenylindole (DAPI), a nuclear marker; FM4-64FX, FM4-64FX dye images, a plasma membrane-specific vital dye. Merge, overlap of GFP (green) and FM4-64FX (red) fluorescence. Row 1 shows the protoplasts expressing GFP alone, which was used as a control. Row 2 shows protoplasts expressing the PaPIP1-1:GFP fusion protein with FM4-64 fluorescence. Row 3 shows the protoplasts expressing the PaPIP2-3:GFP fusion protein with FM4-64 dye. Row 4 shows protoplasts expressing the PaSIP1-3:GFP fusion protein with FM4-64 fluorescence. Row 5 shows the protoplasts expressing the PaXIP1-2:GFP fusion protein with FM4-64 dye. Row 6 shows the protoplasts expressing the PaNIP6-1:GFP fusion protein with FM4-64 dye. Row 7 shows protoplasts expressing the PaTIP1-1:GFP fusion protein with FM4-64 dye. FM4-64FX shows a plasma membrane-specific dye. Bars = 5 μm.
The PaPIP1-3 and PaTIP1-1 Genes Conferred Low-Temperature Resistance to Yeast
To analyze the function of PaAQP genes involved in low-temperature tolerance, four genes that showed high expression in flower buds and stems during the dormancy and sprouting stages of P. armeniaca were expressed in yeast strain GS115 to check their low-temperature stress resistance. The yeast strains transformed with the pGAPZA empty vector or various pGAPZA:PaAQP recombinant expression vectors were incubated in solid and liquid SC-U medium (2% galactose) for low-temperature stress treatment. The growth of yeast cells transformed with pGAPZA:PaPIP1-3 and pGAPZA:PaTIP1-1 on solid SC-U media consisting of 2 M sorbitol after −20°C low-temperature treatment grew much more than did the cells transformed with empty vector. Moreover, the colonies of yeast cells harboring the PaPIP1-3 and PaTIP1-1 genes were larger than those harboring empty vectors strains (Figure 8A). In 2 M liquid SC-U sorbitol media, the mean OD value of the empty-vector strains was 2.33, whereas the positive strains harboring PaPIP1-3 and PaTIP1-1 had OD values of 2.73 and 2.55, respectively. After the −20°C low-temperature treatment, the mean OD values were 1.99 vs. 2.54 and 2.48 (Figure 8B). These results suggested that the PaPIP1-3 and PaTIP1-1 genes improved the low-temperature stress resistance of yeast cells.
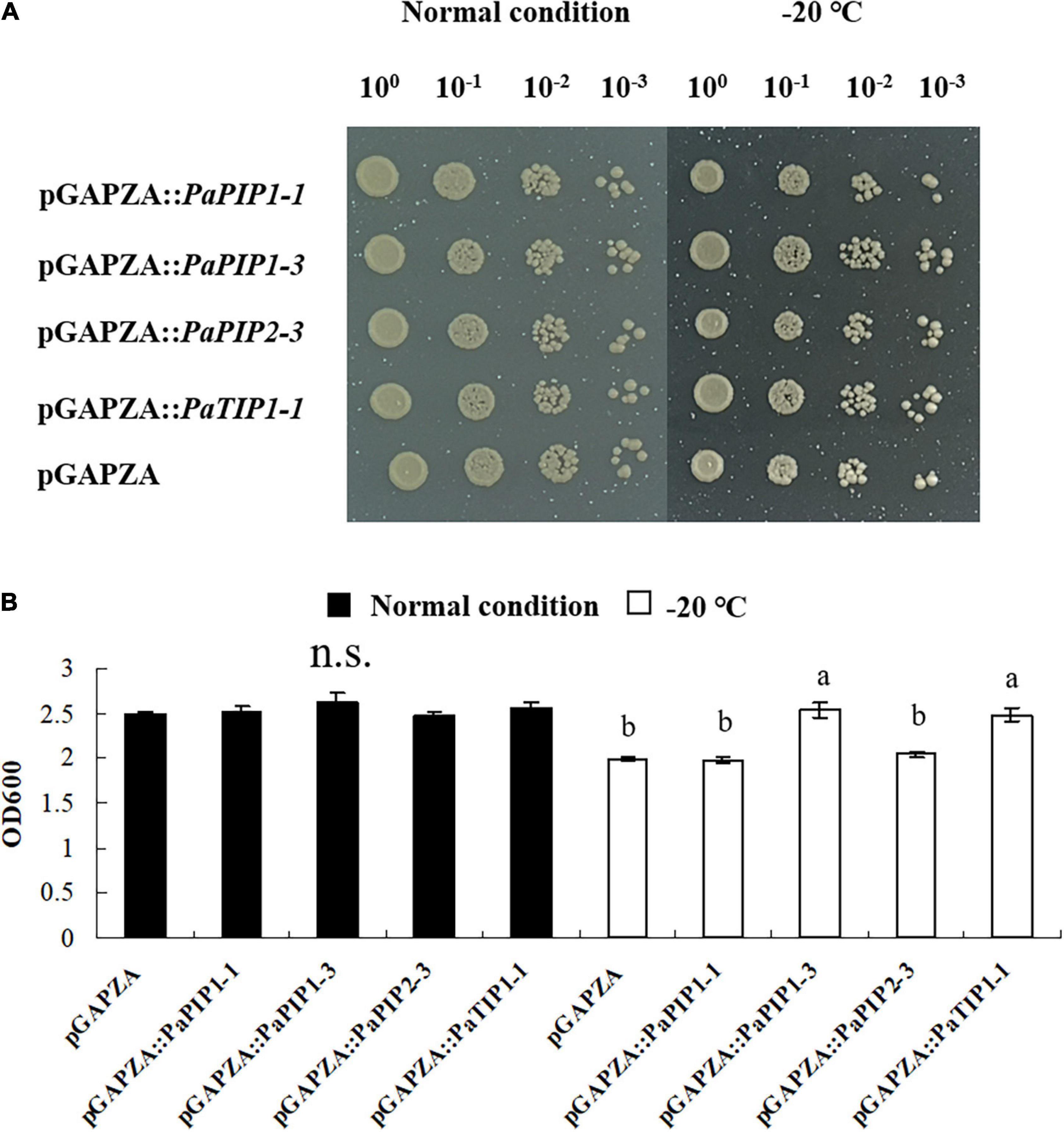
Figure 8. Overexpression of PaPIP1-3 and PaTIP1-1 increases cold tolerance and accumulation in yeast. (A) Growth of the GS115 yeast strain transformed with the empty vector pGAPZA or with pGAPZA harboring PaPIP1-1, PaPIP1-3, PaPIP2-3, or PaTIP1-1. (B) OD600 value of yeast transformants in response to low-temperature stress. The data are the means ± SDs of three replications. n.s. means not significant. The different lowercase letters indicate significant differences at P < 0.05 by ANOVA.
Ectopic Expression of PaPIP1-3 and PaTIP1-1 in A. thaliana Conferred Cold Tolerance
To study the function of the PaAQP genes in cold stress, two genes (PaPIP1-3 and PaTIP1-1) exhibiting cold stress resistance in yeast were introduced individually into A. thaliana plants. Normally growing wild-type Arabidopsis and PaPIP1-3 or PaTIP1-1 gene-carrying Arabidopsis were transferred to growth chambers at 22, 16, or 16°C 16 h/4°C 8 h. After 10 days of treatment, the wild-type plants showed significantly high sensitivity to cold stress with yellow leaves. The growth of PaPIP1-3- or PaTIP1-1-overexpressing (OE) Arabidopsis lines (PIPOE-1, PIPOE-2, TIPOE-1, and TIPOE-2) was better than that of the wild-type plants, demonstrating good cold resistance (Figure 9A). After low temperature treatment, two independent PaPIP1-3 transgenic lines (PIPOE-1 and PIPOE-2) and two independent PaTIP1-1 transgenic lines (TIPOE-1 and TIPOE-2), which with higher relative expression level than untreated transgenic lines, were identified by qRT-PCR (Figure 9B). We further examined the activities of SOD, the major ROS antioxidant enzyme in plants. The PIPOE-1 and PIPOE-2 lines and the TIPOE-1 and TIPOE-2 lines had significantly greater SOD activity than the wild-type line (Figure 9C). The superior performance of the PaPIP1-3- and PaTIP1-1-transformed plants was further validated by analyzing the proline and MDA contents subsequent to cold stress. The proline level was significantly greater in the transgenic leaves than in the wild-type leaves under the stress conditions, indicating superior biochemical capabilities of the transgenic plants (Figure 9D). Similarly, the MDA content was greatest in wild-type leaves of cold-stressed plants, showing that the greatest membrane damage had occurred in untransformed plants (Figure 9E). This research indicated that the target genes PaPIP1-3 or PaTIP1-1 were directly related to cold resistance, and the increase in PaPIP1-3 or PaTIP1-1 expression in PaPIP1-3- or PaTIP1-1-transformed plants could improve the physiological condition and cold resistance of transgenic plants.
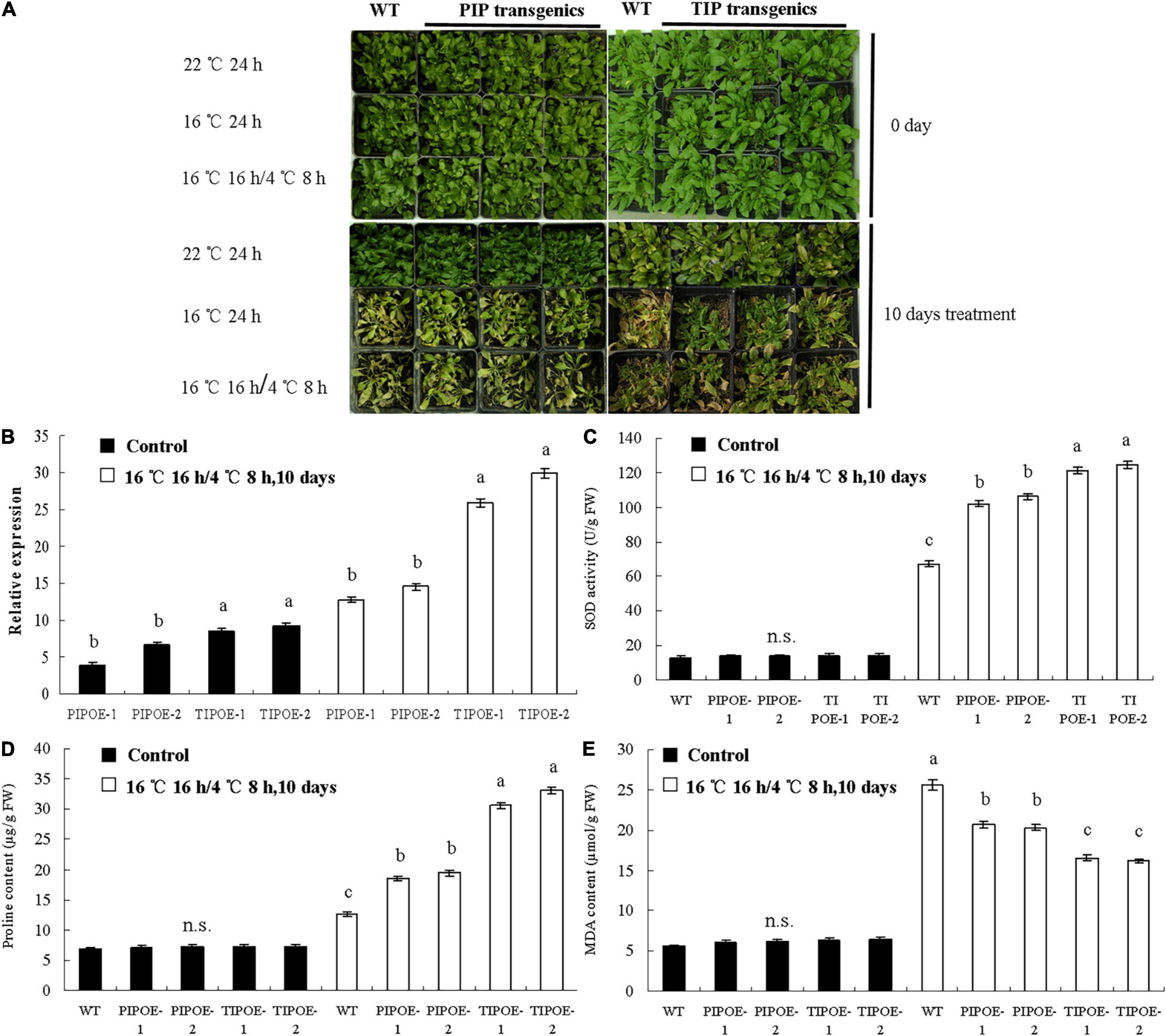
Figure 9. Cold tolerance analysis of wild-type and PaPIP1-3- or PaTIP1-1-OE plants. (A) Image of the wild-type and transgenic plants in growth chambers at 22, 16, and 16°C for 16 h/4°C 8 h. Two independent PaPIP1-3 transgenic lines (PIPOE-1and PIPOE-2) and two independent PaTIP1-1 transgenic lines (TIPOE-1and TIPOE-2), were identified by qRT-PCR. (B). After 10 days of treatment, the SOD activity (C), proline content (D), and MDA content (E) of PaPIP1-3 or PaTIP1-1 transgenic Arabidopsis and wild-type Arabidopsis were determined. PIPOE- 1-, PIPOE- 2-, and PaPIP1-3-OE transgenic lines; TIPOE- 1-, TIPOE- 2-, and PaTIP1-1-OE transgenic lines. Actin was used as a control gene. The error bars represent the SEs of three replicates. n.s. means not significant. The different letters represent significant differences at P < 0.05 (one-way ANOVA).
Discussion
Aquaporins are varied and are widely distributed and functionally diverse in plant genomes. These proteins play important roles in the transport of water and substrates in plants, in regulating plant development and growth, and in responses to drought, cold, or salt stresses (Prado and Maurel, 2013; Kong et al., 2017). A majority of the genome-wide analyses and functional studies of AQP genes conducted to date have focused on model plants such as Z. mays, A. thaliana, O. sativa, N. tabacum, and Populus (Chaumont et al., 2001; Gupta and Sankararamakrishnan, 2009; Nguyen et al., 2013). Very little is known about the AQPs of economic forest species such as P. armeniaca. To determine the evolutionary relationships and functional mechanisms of PaAQPs, a phylogenetic tree was built using AQPs from A. thaliana (in which a full set of AtAQP genes is well known) and Prunus persica (Deshmukh et al., 2015), which are closely related species of P. armeniaca. The number of AQPs identified in this study was the same as that reported in Brachypodium distachyon (Deshmukh et al., 2015), being slightly greater than the 30 AQP genes reported in Elaeis guineensis (Deshmukh et al., 2015) and Vitis vinifera (Deshmukh et al., 2015) and lower than the 33 genes found in rice (Sakurai et al., 2005), 35 genes found in A. thaliana (Zhang et al., 2013), and 37 genes found in Ricinus communis (Deshmukh et al., 2015). The number of P. armeniaca AQPs in the five subfamilies was also similar to their distribution in Cajanus cajan (Deshmukh et al., 2015). However, the numbers of PIPs and TIPs varied in the two genomes compared with those of the other subfamilies. Twelve and thirteen members of PIPs and TIPs have been reported in C. cajan (Deshmukh et al., 2015), while seven SIPs and ten TIPs have been detected in P. armeniaca. PaTIPs are divided into five subgroups, TIP1, TIP2, TIP3, TIP4, and TIP5, and this classification is consistent with those previously reported in species such as barley, sugar beet, rice, and maize (Tombuloglu et al., 2016; Kong et al., 2017).
The GRAVY score is an important property of AQPs that facilitates high water permeability (Murata et al., 2000), and the GRAVY scores of the PaAQP proteins can reflect their hydrophobic nature. The lowest average GRAVY value was found in the PIP subfamily, indicating good hydrophilicity of PaPIPs toward water molecules. Topological analysis predicted that almost all PaAQPs had six transmembrane helical domains (Table 2), which is consistent with the structure identified in other plants, such as flax (L. usitatissimum) (Shivaraj et al., 2017), Hevea brasiliensis (Zhi et al., 2016), and B. vulgaris (Kong et al., 2017). However, PaTIP2-2 and PaTIP2-3 showed a partial loss of TM6, and the effect of the missing protein domains on protein functions must be confirmed. The absence of the TM6 domain may affect the protein folding, transport activity, and subcellular localization of PaTIPs (Hu et al., 2012). Almost all PaTIPs were located in vacuoles, while PaTIP2-3 was localized in the plasma membrane based on Plant-mPLoc prediction.
An unrooted phylogenetic tree was constructed to analyze the evolutionary relationships based on the protein sequences of the PaAQPs, in which one PaSIP (PaSIP1-3), three PaNIPs (PaNIP3-2, PaNIP5-1a, and PaNIP5-1b), and one PaXIP (PaXIP1-2) aroused our attention and caused confusion in the classification and cluster analysis. SIP1-3 and NIP3-2 were not found in A. thaliana; PaSIP1-3 shared the greatest similarity of 61.41% with PtSIP1-3 (Supplementary Table 8), while for PaNIP3-2 and PaXIP1-2, the closest homolog of PaNIP3-2 was PpeNIP3-2 (Figure 1A), and PaXIP1-2 clustered closer to PpeXIP1-2 of P. persica. PaNIP5-1a and PaNIP5-1b are tandemly duplicated genes located at the same site on chromosome 3.
The number of PaAQP genes (33) in the P. armeniaca genome is lower than that in A. thaliana (35) and greater than that in P. persica (29). However, while the genome size of P. armeniaca (222 Mb) is slightly smaller than that of P. persica (226 Mb) (Verde et al., 2013), it is larger than that of A. thaliana (164 Mb). The 33 PaAQP proteins were categorized into five clades. We further observed that most of the PaAQP genes were closely related to the AQP genes in P. persica, consistent with the finding that P. armeniaca and P. persica diverged from a recent common ancestor. It is well known that gene duplication mechanisms (WGD/segmental duplication, tandem duplication) have a significant role in biological evolution (Panchy et al., 2016). In the present study, we observed that orthologous genes developed through segmental duplication and tandem duplication. Both styles of duplication have played a significant role in the expansion of P. armeniaca AQP family genes. The identification of orthologous PaAQP genes will further benefit the evolutionary history of this gene family.
Asn-Pro-Ala motifs, the ar/R selectivity filter, and Froger’s positions are considered to participate in transport activity and substrate selection. PaAQP functions can be speculated based on the amino acid residues of the proteins compared with the AQPs of other plants. The PaPIPs showed typical NPA motifs, F-H-T-R residues in the ar/R selectivity filter, and Q/E-S-A-F-W at Froger’s positions, which are highly conserved in the PIPs of other plants, such as flax (L. usitatissimum) (Shivaraj et al., 2017), chickpea (C. arietinum L.) (Deokar and Bunyamin, 2016) and H. brasiliensis (Murata et al., 2000). This composition of PIPs is considered to play a vital role in facilitating CO2 diffusion, regulating leaf and root hydraulics, and influencing plant photosynthesis (Gupta and Sankararamakrishnan, 2009). Therefore, the homologous PIPs of P. armeniaca may play similar roles in regulating plant growth and development, such as in photosynthetic physiology, plant hydraulics, and water and solute transport. Plant TIPs have been suggested to function as water transporters as efficiently as PIPs (Murata et al., 2000). For example, PaTIP1-1, BvTIP1-2, and BvTIP1-3 contain dual conserved NPA motifs, H-I/V-A-V at the ar/R selectivity filter and T-S-A-Y-W at Froger’s positions, which is the same composition observed for the Citrus sinensis AQPs CsTIP1s and CsTIP3s. Additionally, these proteins are predicted to function in the transport of urea and H2O2 (Hove and Bhave, 2011). Compared with other TIPs, PaTIP1-2, PaTIP1-3, and PaTIP2-1 showed H-I-A-V or H-I-G-R at the ar/R selectivity filter and at Froger’s positions and harbored T-S-A-Y-W residues at the P1-P5 positions; this structure type is considered to transport substances such as NH4, urea, and H2O2 (Hove and Bhave, 2011). These analyses suggest that PaTIPs play an important role in the transport of a wide range of molecules.
WoLF PSORT predicted that all the PaPIP members were localized in the plasma membrane, and plant-mPLoc prediction indicated that the PaNIPs (except for PaNIP5-1a) were also localized in the plasma membranes. These results were consistent with experimental findings for PIPs and NIPs of banana, flax, and other plant species (Maurel et al., 2008; Shivaraj et al., 2017). However, some tobacco NtPIP members show dual localization in the plasma membrane and inner chloroplast membrane (Uehlein and Kaldenhoff, 2008), and different localizations of a maize PIP gene (ZmPIP1-2) have been found in the endoplasmic reticulum and plasma membrane (Chaumont et al., 2000). NIPs also exhibit different subcellular localizations in the peribacteroid membrane, endoplasmic reticulum, or plasma membrane in other plants (Takano et al., 2006; Deokar and Bunyamin, 2016). According to our predictions, both PaNIP1-1 and PaNIP4-2 were localized to the plasma membrane and vacuoles. The reasons for the different localizations of these genes are not clear. The majority of SIPs have been shown to localize to the vacuoles and plasma membrane; however, grapevine and A. thaliana SIPs are predicted to be localized to the endoplasmic reticulum (Ishikawa et al., 2005; Noronha et al., 2014; Kong et al., 2017). In addition to differences between species and protein sequences, many experiments have shown that external environmental conditions such as drought and salinity, but not cold, influence the characteristics of the subcellular localization of PaAQPs (Boursiac et al., 2008; Deokar and Bunyamin, 2016). These results indicate that the subcellular localization of plant AQPs is complex and regulated by environmental conditions.
Aquaporin genes are expressed in different plant tissues and organs, and their spatiotemporally specific expression is highly correlated with different developmental stages (Alexandersson et al., 2005; Giorgio et al., 2016). The expression patterns of AQPs in various tissues as well as under different stresses may provide an efficient basis for identifying their molecular functions. qRT-PCR results showed that PaAQPs were expressed in all tissues tested, but their transcript levels largely differed in various organs (Figure 5), suggesting that the functions of PaAQPs might vary. For example, the expression levels during the endodormancy stage (PaPIP2-3, PaPIP2-2, and PaSIP1-3 are high) indicated that the effects of growth inhibition were controlled by other internal factors resulting from insufficient low-temperature accumulation. High expression levels during the ecodormancy stage (PaTIP1-2 and PaTIP2-1) could reflect the activation of growth through release from endogenous inhibitors but maintained suppression due to unsuitable conditions (insufficient high temperature accumulation). Expression during SP (PaSIP1-2, PaNIP3-2, and PaNIP6-1 are high) and GS (PaPIP1-1, PaPIP2-4, PaSIP1-1, PaSIP2-1, PaXIP1-2, PaNIP2-1, PaNIP1-1, PaNIP3-1, PaTIP1-1, PaTIP1-3, and PaTIP4-1 are high) showed morphological growth resumption, not (endo)dormancy release. Moreover, PaPIP1-1, PaPIP2-2, PaPIP2-3, and PaSIP1-1 were highly expressed in stems, where they may contribute to the storage of energy and materials needed for growth, development, and germination.
In the PaAQP coexpression network, similar expression patterns of gene pairs (such as PaSIP1-1 and PaZFC3HC4, PaSIP1-1 and PaEREBP, PaNIP6-1 and PaUbI and PaNIP6-1 and PaWRKY) during the dormancy and sprouting stages suggested that PaAQPs cooperated with various vital cold stress- or flower bud dormancy/germination-related genes to regulate cold tolerance, bud dormancy, and bud break. For example, a novel zinc-finger protein (homologous gene of PaZFC3HC4) mediates ABA-regulated seed dormancy in Arabidopsis (He and Gan, 2004), and an ubiquitin-conjugating enzyme (homologous gene of PaUbI) has been found to be involved in tuber dormancy breaking (Liu et al., 2012). A total of 68 AP2/ERF genes were first identified in dormant Chinese cherry flower buds. Some PpcAP2/ERF TFs (homologous genes of PaEREBP) participate in and influence the dormancy transition through their responses to low temperature (Zhu et al., 2021). Chen et al. (2016) found that the expression levels of six WRKY genes increased during endodormancy and decreased during ecodormancy, indicating that these six WRKY genes may play a role in dormancy in peach. However, several coexpression pairs, such as PaSIP1-3 and PabZIP and PaTIP2-1 and PaNAC, exhibited different expression regularities, implying that these gene pairs may have different roles and functions in different periods during the dormancy and sprouting stages of P. armeniaca.
Aquaporins regulate long-distance water transport between cells and can mediate the osmotic pressure of the cytoplasm to appropriately regulate plant growth and development, responses to environmental stress, and stomatal movement, among others. PaTIPs can mediate rapid water transport between cells, remove accumulated H2O2, and improve water transport activity, which is necessary to end dormancy (Erez et al., 1998; Yooyongwech et al., 2008). In our study, several PaTIPs, such as PaTIP1-1, PaTIP1-2, and PaTIP4-1, showed greater transcript levels in all four dormancy and sprouting stages of P. armeniaca. PaTIP1-2 showed greater expression in the ED period than SP and GS, which may represent a response to cold stress to aid in the removal of the accumulated induced H2O2, thereby promoting the end of dormancy. In particular, overexpression of PaTIP1-1 increased cold tolerance and accumulation in yeast (Figure 8), which may help to improve water transport activity and promote normal growth under low-temperature stress. Previous research has indicated that AtPIP2-5 and AtPIP2-6 are upregulated by cold stress in A. thaliana plants (Alexandersson et al., 2005). Transgenic banana plants overexpressing MusaPIP1-2 show a high tolerance to cold conditions (Shekhawat and Ganapathi, 2013). A. thaliana plants overexpressing AtPIP1-4 or AtPIP2-5 display greater cold tolerance than wild-type plants (Afzal et al., 2016). In our study, PaPIP1-2 and PaPIP2-4 exhibited high expression in the dormancy and sprouting stages in the flower buds of P. armeniaca. Interestingly, yeast cells expressing PaPIP1-3 and PaTIP1-1 exhibited markedly enhanced growth compared with that of yeast cells transformed with the empty vector on SG-U medium with low-temperature treatment (Figure 8), and PaPIP1-3 or PaTIP1-1 overexpressed in Arabidopsis showed enhanced cold tolerance by improving antioxidative enzyme activities (Figure 9). These results may suggest that PaPIPs present stronger cold resistance and play a vital role in the maintenance of normal growth and development under cold conditions.
Conclusion
Overall, this work presents the first genome-wide study of the P. armeniaca AQP gene family, in which 33 non-redundant PaAQP genes were identified in the P. armeniaca genome and phylogenetically clustered into five distinct subfamilies. Synteny analysis identified 14 collinear blocks containing AQP genes between P. armeniaca and A. thaliana and 30 collinear blocks between P. armeniaca and P. persica. The chromosome localization, gene structure, protein characteristics, conserved functional motifs, and homology-based 3D models of the PaAQP genes were further examined. Cis-motif analysis of the 2.0-kb sequences upstream of the AQP genes indicated the existence of several light-, hormone-, and stress-responsive and developmental stage-specific elements in the PaAQPs. The subcellular localization of PaPIP1-1, PaPIP2-3, PaSIP1-3, PaXIP1-2, PaNIP6-1, and PaTIP1-1 was examined, and the green fluorescence of the recombinant proteins was observed in the plasma membrane of isolated protoplasts. In silico analysis of protein-protein interactions suggested the association of PaAQP proteins with specific cold tolerance and development pathways. Gene expression profiling of PaAQP showed that PaPIP1-3, PaPIP2-1, and PaTIP1-1 were highly expressed in flower buds during the dormancy and sprouting stages of P. armeniaca. Significantly, PaPIP1-3 and PaTIP1-1 conferred low-temperature stress resistance in a yeast expression system. Overexpression of PaPIP1-3 and PaTIP1-1 in Arabidopsis plants increased antioxidative enzyme activities and improved transgenic plant tolerance to cold stress. This study provides an important resource for the future functional analysis of PaAQPs and a theoretical basis for the breeding of new varieties via the genetic engineering of P. armeniaca.
Data Availability Statement
The datasets presented in this study can be found in online repositories. The names of the repository/repositories and accession number(s) can be found in the article/Supplementary Material.
Author Contributions
SL and T-NW conceived and designed the research. SL, YX, and SW performed all the experiments. YZ, GZ, XZ, and JL contributed reagents, materials, and analysis tools. JZ, XG, LW, and WM analyzed the data. SL wrote the manuscript. All authors read and approved the final manuscript.
Funding
This work was supported by the Fundamental Research Funds of CAF (CAFYBB2018MA003), the National Natural Science Foundation of China (31770705 and 31400570), and the National Key R&D Program of China (2019YFD1001200).
Conflict of Interest
The authors declare that the research was conducted in the absence of any commercial or financial relationships that could be construed as a potential conflict of interest.
Publisher’s Note
All claims expressed in this article are solely those of the authors and do not necessarily represent those of their affiliated organizations, or those of the publisher, the editors and the reviewers. Any product that may be evaluated in this article, or claim that may be made by its manufacturer, is not guaranteed or endorsed by the publisher.
Supplementary Material
The Supplementary Material for this article can be found online at: https://www.frontiersin.org/articles/10.3389/fpls.2021.690040/full#supplementary-material
Supplementary Figure 1 | Distribution of PaAQP genes on the eight P. armeniaca chromosomes. Thirty-three PaAQP genes are distributed across all eight P. armeniaca chromosomes. The right side of the chromosomal bar represents the PaAQP genes, and the corresponding positions on the chromosome (megabase pairs; Mb) are given on the left side. The PaNIP genes are in black, the PaPIP genes are in red, the PaSIP genes are in purple, the PaXIP genes are in green, and the PaTIP genes are in magenta. Tandemly duplicated genes are underlined. Whole genome/segmental duplications are lined and underlined.
Supplementary Figure 2 | Exon-intron structures of the 33 PaAQP genes. The yellow boxes denote exons, and the lines denote introns. The exon-intron structure was determined via GSDS 2.0 (http://gsds.cbi.pku.edu.cn/) on the basis of gene and CDSs.
Supplementary Figure 3 | Protein sequence alignment of PaNIPs identified in P. armeniaca showing the amino acids at NPA domains, ar/R filters, and Froger’s residues.
Supplementary Figure 4 | Protein sequence alignment of PaTIPs identified in P. armeniaca showing the amino acids at NPA domains, ar/R filters, and Froger’s residues.
Supplementary Figure 5 | Protein sequence alignment of PaSIPs identified in P. armeniaca showing the amino acids at NPA domains, ar/R filters, and Froger’s residues.
Supplementary Figure 6 | Protein sequence alignment of PaXIPs identified in P. armeniaca showing the amino acids at NPA domains, ar/R filters, and Froger’s residues.
Supplementary Figure 7 | Distribution of conserved motifs in the PaAQP proteins. The motifs were identified via the online MEME program. The different colored boxes indicate different motifs at the corresponding positions.
Supplementary Figure 8 | Various cis-acting elements in the promoter of PaAQP genes. The numbers of ARE (AAACCA), ABRE (ACGTG), MBS (CAACTG), MRE (AACCTAA), and other cis-acting elements in the promoter region of P. armeniaca PaAQP genes from each AQP family. The cis-acting elements were determined in the 2-kb promoter region upstream of the translation initiation site using the PLACE database.
Supplementary Figure 9 | qRT-PCR analysis of the relative expression of 20 genes during the dormancy and sprouting stages of P. armeniaca.
Supplementary Table 1 | Complete coding sequences and corresponding amino acid sequences of the AQP genes of P. armeniaca.
Supplementary Table 2 | The coding regions of WRKY6, TSPO, and ATHATPLC1G can interact with PaAQP genes.
Supplementary Table 3 | Primers used in the qRT-PCR analysis of the PaAQP genes, and cold resistance genes coexpressed with PaAQP genes.
Supplementary Table 4 | Analysis of cis-acting elements in the 2,000-bp sequence upstream of the translation initiation codon of PaAQP genes.
Supplementary Table 5 | Predicted 3D structure and transmembrane region of the 33 PaAQP proteins.
Supplementary Table 6 | Predicted functional partners of TIP, PIP, SIP, and NIP. Function of the top 10 highest scoring interacting proteins in each subfamily.
Supplementary Table 7 | Genes related to cold resistance in the PaAQP coexpression network.
Supplementary Table 8 | Amino acid sequences of AQP genes in poplar.
Footnotes
- ^ http://www.arabidopsis.org
- ^ https://phytozome.jgi.doe.gov/pz/portal.html
- ^ http://pfam.xfam.org/family/PF00230
- ^ http://hmmer.org/
- ^ http://www.ncbi.nlm.nih.gov/Structure/cdd/wrpsb.cgi
- ^ http://smart.embl-heidelberg.de/
- ^ https://www.arabidopsis.org/
- ^ http://www.ebi.ac.uk/clustalw/
- ^ http://web.expasy.org/protparam/
- ^ http://www.cbs.dtu.dk/services/TMHMM/
- ^ http://www.genscript.com/wolf-psort.html
- ^ http://www.csbio.sjtu.edu.cn/bioinf/plant-multi/
- ^ http://gsds.cbi.pku.edu.cn/
- ^ http://meme-suite.org/tools/meme
- ^ http://www.sbg.bio.ic.ac.uk/phyre2/html/page.cgi?id=index
- ^ http://bioinformatics.psb.ugent.be/webtools/plantcare/html/
- ^ https://string-db.org/?tdsourcetag=s_pctim_aiom
- ^ http://hemi.biocuckoo.org/down.php
- ^ http://www.cytoscape.org/
References
Afzal, Z., Howton, T. C., Sun, Y., and Mukhtar, M. S. (2016). The Roles of aquaporins in plant stress responses. J. Dev. Biol. 4:9. doi: 10.3390/jdb4010009
Alexandersson, E., Danielson, J. A., Rade, J., Moparthi, V. K., Fontes, M., Kjellbom, P., et al. (2010). Transcriptional regulation of aquaporins in accessions of Arabidopsis in response to drought stress. Plant J. 61, 650–660. doi: 10.1111/j.1365-313X.2009.04087.x
Alexandersson, E., Fraysse, L., Sjövall-Larsen, S., Gustavsson, S., Fellert, M., Karlsson, M., et al. (2005). Whole gene family expression and drought stress regulation of Aquaporins. Plant Mol. Biol. 59, 469–484. doi: 10.1007/s11103-005-0352-1
Anderberg, H. I., Kjellbom, P., and Johanson, U. (2012). Annotation of Selaginella moellendorffii major intrinsic proteins and the evolution of the protein family in terrestrial plants. Front. Plant Sci. 3:33. doi: 10.3389/fpls.2012.00033
Ariani, A., and Gepts, P. (2015). Genome-wide identification and characterization of aquaporin gene family in common bean (Phaseolus vulgaris L.). Mol. Genet Genome 290, 1771–1785. doi: 10.1007/s00438-015-1038-2
Bansal, A., and Sankararamakrishnan, R. (2007). Homology modeling of major intrinsic proteins in rice, maize and Arabidopsis: comparative analysis of transmembrane helix association and aromatic/arginine selectivity filters. BMC Struct. Biol. 7:27. doi: 10.1186/1472-6807-7-27
Bates, L. S., Waldren, R. P., and Teare, J. D. (1973). Rapid determination of free proline for water stress studies. Plant Soil. 39, 205–207. doi: 10.1007/BF00018060
Boursiac, Y., Prak, S., Boudet, J., Postaire, O., Luu, D. T., Tournaire-Roux, C., et al. (2008). The response of Arabidopsis root water transport to a challenging environment implicates reactive oxygen species- and phosphorylation dependent internalization of aquaporins. Plant Signal. Behav. 3, 1096–1098. doi: 10.4161/psb.3.12.7002
Chaumont, F., Barrieu, F., Jung, R., and Chrispeels, M. J. (2000). Plasma membrane intrinsic proteins from maize cluster in two sequence subgroups with differential aquaporin activity. Plant Physiol. 122, 1025–1034. doi: 10.1104/pp.122.4.1025
Chaumont, F., Barrieu, F., Wojcik, E., Chrispeels, M. J., and Jung, R. (2001). Aquaporins constitute a large and highly divergent protein family in maize. Plant Physiol. 125, 1206–1215. doi: 10.1104/pp.125.3.1206
Chen, C., Chen, H., Zhang, Y., Thomas, H. R., Frank, M. H., He, Y., et al. (2018). TBtools-an integrative toolkit developed for interactive analyses of big biological data. Mol. Plant 13, 1194–1202. doi: 10.1016/j.molp.2020.06.009
Cheng, F., Mandáková, T., Wu, J., Xie, Q., Lysak, M. A., and Wang, X. (2013). Deciphering the diploid ancestral genome of the mesohexaploid Brassica rapa. Plant Cell 25, 1541–1554. doi: 10.1105/tpc.113.110486
Chen, M., Tan, Q., Sun, M., Li, D., Fu, X., Chen, X., et al. (2016). Genome-wide identification of WRKY family genes in peach and analysis of WRKY expression during bud dormancy. Mol. Genet. Genomics 291, 1319–1332. doi: 10.1007/s00438-016-1171-6
Chou, K. C., and Shen, H. B. (2010). Plant-mPLoc: a top-down strategy to augment the power for predicting plant protein subcellular localization. PLoS One 5:e11335. doi: 10.1371/journal.pone.0011335
Clough, S. J., and Bent, A. F. (2010). Floral dip: a simplified method for Agrobacterium-mediated transformation of Arabidopsis thaliana. Plant J. 16, 735–743. doi: 10.1046/j.1365-313x.1998.00343.x
Crow, K. D., and Wagner, G. P. (2006). What is the role of genome duplication in the evolution of complexity and diversity. Mol. Biol. Evol. 23, 887–892. doi: 10.1093/molbev/msj083
Danielson, J. A., and Johanson, U. (2008). Unexpected complexity of the aquaporin gene family in the moss Physcomitrella patens. BMC Plant Biol. 8:45. doi: 10.1186/1471-2229-8-45
Deokar, A. A., and Bunyamin, T. (2016). Genome-wide analysis of the aquaporin gene family in Chickpea (Cicer arietinum L.). Front. Plant Sci. 7:1802. doi: 10.3389/fpls.2016.01802
Deshmukh, R. K., Vivancos, J., Guérin, V., Sonah, H., Labbé, C., Belzile, F., et al. (2013). Identification and functional characterization of silicon transporters in soybean using comparative genomics of major intrinsic proteins in Arabidopsis and rice. Plant Mol. Biol. 83, 303–315. doi: 10.1007/s11103-013-0087-3
Deshmukh, R. K., Vivancos, J., Ramakrishnan, G., Guerin, V., Carpentier, G., Sonah, H., et al. (2015). A precise spacing between the NPA domains of aquaporins is essential for silicon permeability in plants. Plant J. 83, 489–500. doi: 10.1111/tpj.12904
Erez, A., Faust, M., and Line, M. J. (1998). Changes in water status in peach buds on induction development and release from dormancy. Sci. Hortic. 73, 111–123. doi: 10.1016/S0304-4238(97)00155-6
Froger, A., Tallur, B., Thomas, D., and Delamarche, C. (1998). Prediction of functional residues in water channels and related proteins. Protein Sci. 7, 1458–1468. doi: 10.1002/pro.5560070623
Gao, Y., Zhu, M., Ma, Q., and Li, S. (2020). Dormancy breakage in Cercis chinensis seeds. Can. J. Plant Sci. 100, 666–673. doi: 10.1139/CJPS-2019-0229
Gasteiger, E., Gattiker, A., Hoogland, C., Ivanyi, I., Appel, R. D., and Bairoch, A. (2003). ExPASy: the proteomics server for in-depth protein knowledge and analysis. Nucleic Acids Res. 31, 3784–3788. doi: 10.1093/nar/gkg563
Giorgio, J. A. P. D., Bienert, G. P., Ayub, N. D., Yaneff, A., Barberini, M. L., Mecchia, M. A., et al. (2016). Pollen-specific aquaporins NIP4;1 and NIP4;2 are required for pollen development and pollination in Arabidopsis thaliana. Plant Cell 28, 1053–1077. doi: 10.1105/tpc.15.00776
Groot, B. L. D., and Grubmüller, H. (2005). The dynamics and energetics of water permeation and proton exclusion in aquaporins. Curr. Opin. Struct. Biol. 15, 176–183. doi: 10.1016/j.sbi.2005.02.003
Gupta, A. B., and Sankararamakrishnan, R. (2009). Genome-wide analysis of major intrinsic proteins in the tree plant Populus trichocarpa: characterization of XIP subfamily of aquaporins from evolutionary perspective. BMC Plant Biol. 9:134. doi: 10.1186/1471-2229-9-134
He, Y., and Gan, S. (2004). A novel zinc-finger protein with a proline-rich domain mediates ABA-regulated seed dormancy in Arabidopsis. Plant Mol. Biol. 54, 1–9. doi: 10.1023/b:plan.0000028730.10834.e3
Heinen, R. B., and Chaumont, F. (2009). Role of aquaporins in leaf physiology. J. Exp. Bot. 60, 2971–2985. doi: 10.1093/jxb/erp171
Hirayama, T., Ohto, C., Mizoguchi, T., and Shinozaki, K. (1995). A gene encoding a phosphatidylinositol-specific phospholipase C is induced by dehydration and salt stress in Arabidopsis thaliana. Proc. Natl. Acad. Sci. U S A. 92, 3903–3907. doi: 10.1073/pnas.92.9.3903
Horton, P., Park, K. J., Obayashi, T., Fujita, N., Harada, H., Adams-Collier, C. J., et al. (2007). WoLF PSORT: protein localization predictor. Nucleic Acids Res. 35, W585–W587. doi: 10.1093/nar/gkm259
Hove, R. M., and Bhave, M. (2011). Plant aquaporins with non-aqua functions: deciphering the signature sequences. Plant Mol. Biol. 75, 413–430. doi: 10.1007/s11103-011-9737-5
Hu, W., Yuan, Q., Wang, Y., Cai, R., Deng, X., Wang, J., et al. (2012). Overexpression of a wheat aquaporin gene, TaAQP8, enhances salt stress tolerance in transgenic tobacco. Plant Cell Physiol. 53, 2127–2141. doi: 10.1093/pcp/pcs154
Ishikawa, F., Suga, S., Uemura, T., Sato, M. H., and Maeshima, M. (2005). Novel type aquaporin SIPs are mainly localized to the ER membrane and show cell specific expression in Arabidopsis thalina. FEBS Lett. 579, 5814–5820. doi: 10.1016/j.febslet.2005.09.076
Jiang, F., Zhang, J., Wang, S., Yang, L., Luo, Y., Gao, S., et al. (2019). The apricot (Prunus armeniaca L.) genome elucidates Rosaceae evolution and beta-carotenoid synthesis. Hortic. Res-England 6:128. doi: 10.1038/s41438-019-0215-6
Johanson U., Karlsson, M., Johansson, I., Gustavsson, S., Sjövall, S., Fraysse, L., et al. (2001). The complete set of genes encoding major intrinsic proteins in Arabidopsis provides a framework for a new nomenclature for major intrinsic proteins in plants. Plant Physiol. 126, 1358–1369. doi: 10.1104/pp.1264.1358
Jue, D., Sang, X., Lu, S., Dong, C., Zhao, Q., Chen, H., et al. (2015). Genome-wide identification, phylogenetic and expression analyses of the Ubiquitin-conjugating enzyme gene family in Maize. PLoS One 10:e0143488. doi: 10.1371/journal.pone.0143488
Jung, Y. J., Lee, I. H., Nou, I. S., Lee, K. D., Rashotte, A. M., and Kang, K. K. (2013). BrRZFP1 a Brassica rapa C3HC4-type RING zinc finger protein involved in cold, salt and dehydration stress. Plant Biol. 15, 274–283. doi: 10.1111/j.1438-8677.2012.00631.x
Kaldenhoff, R., and Fischer, M. (2006). Functional aquaporin diversity in plants. Biochim. Biophys. Acta 1758, 1134–1141. doi: 10.1016/j.bbamem.2006.03.012
Kayum, M. A., Park, J. I., Nath, U. K., Biswas, M. K., Kim, H. T., and Nou, I. S. (2017). Genome-wide expression profiling of aquaporin genes confer responses to abiotic and biotic stresses in Brassica rapa. BMC Plant Biol. 17:23. doi: 10.1186/s12870-017-0979-5
Kong, W., Yang, S., Wang, Y., Bendahmane, M., and Fu, X. (2017). Genome-wide identification and characterization of aquaporin gene family in Beta vulgaris. Peer J. 5:e3747. doi: 10.7717/peerj.3747
Kumar, S., Stecher, G., and Tamura, K. (2016). MEGA7, molecular evolutionary genetics analysis version 7.0 for bigger datasets. Mol. Biol. Evol. 33:1870. doi: 10.1093/molbev/msw054
Lee, T. H., Tang, H., Wang, X., and Paterson, A. H. (2012). PGDD: a database of gene and genome duplication in plants. Nucleic Acids Res. 41, 1152–1158. doi: 10.1093/nar/gks1104
Letunic, I., Doerks, T., and Bork, P. (2012). SMART 7: recent updates to the protein domain annotation resource. Nucleic Acids Res. 40, 302–305. doi: 10.1093/nar/gkr931
Liu, Q., Umeda, M., and Uchimiya, H. (1994). Isolation and expression analysis of two rice genes encoding the major intrinsic protein. Plant Mol. Biol. 26, 2003–2007. doi: 10.1007/BF00019511
Liu, Y., Li, X., Liang, X., and Zhang, S. (2015). CpLEA5, the Late embryogenesis abundant protein gene from Chimonanthus praecox, possesses low temperature and osmotic resistances in Prokaryote and Eukaryotes. Int. J. Mol. Sci. 16, 26978–26990. doi: 10.3390/ijms161126006
Liu, B., Zhang, N., Wen, Y., Si, H., and Wang, D. (2012). Identification of differentially expressed genes in potato associated with tuber dormancy release. Mol. Biol. Rep. 39, 11277–11287. doi: 10.1007/s11033-012-2037-6
Livak, K. J., and Schmittgen, T. D. (2001). Analysis of relative gene expression data using real-time quantitative PCR. Method 25, 402–408. doi: 10.1006/meth.2001
Ma, Q., Dai, X., Xu, Y., Guo, J., Liu, Y., Chen, N., et al. (2009). Enhanced tolerance to chilling stress in OsMYB3R-2 transgenic rice is mediated by alteration in cell cycle and ectopic expression of stress genes. Plant Physiol. 150, 244–256. doi: 10.1104/pp.108.133454
Marchler-Bauer, A., Derbyshire, M. K., Gonzales, N. R., Lu, S., Chitsaz, F., Geer, L. Y., et al. (2015). CDD: NCBI’s conserved domain database. Nucleic Acids Res. 43, D222–D226. doi: 10.1093/nar/gku1221
Marchler-Bauer, A., Panchenko, A. R., Shoemaker, B. A., Thiessen, P. A., Geer, L. Y., and Bryant, S. H. (2002). CDD: a database of conserved domain alignments with links to domain three-dimensional structure. Nucleic Acids Res. 30, 281–283. doi: 10.1093/nar/30.1.281
Maurel, C., Santoni, V., Luu, D. T., Wudick, M. M., and Verdoucq, L. (2009). The cellular dynamics of plant aquaporin expression and functions. Curr. Opin. Plant Biol. 12, 690–698. doi: 10.1016/j.pbi.2009.09.002
Maurel, C., Verdoucq, L., Luu, D. T., and Santoni, V. (2008). Plant aquaporins: membrane channels with multiple integrated functions. Annu. Rev. Plant Biol. 59, 595–624. doi: 10.1146/annurev.arplant.59.032607.092734
Maurel, C., Boursiac, Y., Luu, D. T., Santoni, V., Shahzad, Z., and Verdoucq, L. (2015). Aquaporins in plants. Physiol. Rev. 95, 1321–1358. doi: 10.1152/physrev.00008.2015
Mazzitelli, L., Hancock, R. D., Haupt, S., Walker, P. G., Pont, S. D. A., McNicol, J., et al. (2007). Co-ordinated gene expression during phases of dormancy release in raspberry (Rubus idaeus L.) buds. J. Exp. Bot. 58, 1035–1045. doi: 10.1093/jxb/erl266
Mitani-Ueno, N., Yamaji, N., Zhao, F. J., and Ma, J. F. (2011). The aromatic/arginine selectivity filter of NIP aquaporins plays a critical role in substrate selectivity for silicon, boron, and arsenic. J. Exp. Bot. 62, 4391–4398. doi: 10.1093/jxb/err158
Mitrakos, K., and Diamantoglou, S. (2006). Endosperm dormancy breakage in olive seeds. Physiol. Plantarum. 62, 8–10. doi: 10.1111/j.1399-3054.1984.tb05915.x
Murata, K., Mitsuoka, K., Hirai, T., Walz, T., Agre, P., Heymann, J. B., et al. (2000). Structural determinants of water permeation through aquaporin-1. Nature 407, 599–605. doi: 10.1038/35036519
Nelson, B. K., Cai, X., and Nebenführ, A. (2007). A multicolored set of in vivo organelle markers for co-localization studies in Arabidopsis and other plants. Plant J. 51, 1126–1136. doi: 10.1111/j.1365-313X.2007.03212.x
Nguyen, M. X., Moon, S., and Jung, K. H. (2013). Genome-wide expression analysis of rice aquaporin genes and development of a functional gene network mediated by aquaporin expression in roots. Planta 238, 669–681. doi: 10.1007/s00425-013-1918-9
Noronha, H., Agasse, A., Martins, A. P., Berny, M. C., Gomes, D., Zarrouk, O., et al. (2014). The grape aquaporin VvSIP1 transports water across the ER membrane. J. Exp. Bot. 65, 981–993. doi: 10.1093/jxb/ert448
Panchy, N., Lehti-Shiu, M., and Shiu, S. H. (2016). Evolution of gene duplication in plants. Plant Physiol. 171, 2294–2316. doi: 10.1104/pp.16.00523
Prado, K., and Maurel, C. (2013). Regulation of leaf hydraulics: from molecular to whole plant levels. Front. Plant Sci. 4:255. doi: 10.3389/fpls.2013.00255
Qu, J., Xu, S., Zhang, Z., Chen, G., Zhong, Y., Liu, L., et al. (2018). Evolutionary, structural and expression analysis of core genes involved in starch synthesis. Sci. Rep. 8:12736. doi: 10.1038/s41598-018-30411-y
Qu, Y., Duan, M., Zhang, Z., Dong, J., and Wang, T. (2016). Overexpression of the Medicago falcata NAC transcription factor MfNAC3 enhances cold tolerance in Medicago truncatula. Environ. Exp. Bot. 129, 67–76. doi: 10.1016/j.envexpbot.2015.12.012
Rohde, A., and Bhalerao, R. P. (2007). Plant dormancy in the perennial context. Trends Plant Sci. 12, 217–223. doi: 10.1016/j.tplants.2007.03.012
Sakurai, J., Ishikawa, F., Yamaguchi, T., Uemura, M., and Maeshima, M. (2005). Identification of 33 rice aquaporin genes and analysis of their expression and function. Plant Cell Physiol. 46, 1568–1577. doi: 10.1093/pcp/pci172
Sheen, J. (2001). Signal transduction in maize and Arabidopsis mesophyll protoplasts. Plant Physiol. 127, 1466–1475. doi: 10.1104/pp.010820
Shekhawat, U. K., and Ganapathi, T. R. (2013). Overexpression of a native plasma membrane aquaporin for development of abiotic stress tolerance in banana. Plant Biotechnol J. 11, 942–952. doi: 10.1371/journal.pone.0075506
Shivaraj, S. M., Deshmukh, R. K., Rai, R., Bélanger, R., Agrawal, P. K., and Dash, P. K. (2017). Genome-wide identification, characterization, and expression profile of aquaporin gene family in flax (Linum usitatissimum). Sci. Rep. 7:46137. doi: 10.1038/srep46137
Song, C., Yin, M., Jiang, Z., Li, H., Wuyun, T. N., and Song, J. (2017). Relationship between flower bud differentiation and change of endogenous hormone of ‘Youyi’ during dormancy period. J. Northwest A&F University (Nat. Sci. Ed.) 45, 170–176.
Takano, J., Wada, M., Ludewig, U., Schaaf, G., Wirén, N., and Fujiwara, T. (2006). The Arabidopsis major intrinsic protein NIP5;1 is essential for efficient boron uptake and plant development under boron limitation. Plant Cell 18, 1498–1509. doi: 10.1105/tpc.106.041640
Tang, H., Bowers, J. E., Wang, X., Ming, R., Alam, M., and Paterson, A. H. (2008a). Synteny and collinearity in plant genomes. Science 320, 486–488. doi: 10.1126/science.1153917
Tang, H., Wang, X., Bowers, J. E., Ming, R., Alam, M., and Paterson, A. H. (2008b). Unraveling ancient hexaploidy through multiply-aligned angiosperm gene maps. Genome Res. 18, 1944–1954. doi: 10.1101/gr.080978.108
Tang, H., Bowers, J. E., Wang, X., and Paterson, A. H. (2009). Angiosperm genome comparisons reveal early polyploidy in the monocot lineage. Proc. Natl. Acad. Sci. U S A. 107, 472–477. doi: 10.1073/pnas.0908007107
Tao, P., Zhong, X., Li, B., Wang, W., Yue, Z., Lei, J., et al. (2014). Genome-wide identification and characterization of aquaporin genes (AQPs) in Chinese cabbage (Brassica rapa ssp. pekinensis). Mol. Genet Genome 289, 1131–1145. doi: 10.1007/s00438-014-0874-9
Thompson, J. D., Higgins, D. G., and Gibson, T. J. (1994). CLUSTAL W: improving the sensitivity of progressive multiple sequence alignment through sequence weighting, position-specific gap penalties and weight matrix choice. Nucleic Acids Res. 22, 4673–4680. doi: 10.1093/nar/22.22.4673
Tombuloglu, H., Ozcan, I., Tombuloglu, G., Sakcali, S., and Unver, T. (2016). Aquaporins in boron-tolerant barley: identifification, characterization, and expression analysis. Plant Mol. Biol. Rep. 34, 374–386. doi: 10.1007/s11105-015-0930-6
Uehlein, N., and Kaldenhoff, R. (2008). Aquaporins and plant leaf movements. Ann. Bot. 101, 1–4. doi: 10.1093/aob/mcm278
Venkatesh, J., Yu, J. W., and Park, S. W. (2013). Genome-wide analysis and expression profiling of the Solanum tuberosum aquaporins. Plant Physiol. Biochem. 73, 392–404. doi: 10.1016/j.plaphy.2013.10.025
Verde, I., Abbott, A., Scalabrin, S., Jung, S., Shu, S., Marroni, F., et al. (2013). The high-quality draft genome of peach (Prunus persica) identifies unique patterns of genetic diversity, domestication and genome evolution. Nat. Genet. 45, 487–494. doi: 10.1038/ng.2586
Wang, L., Xu, C., Wang, C., and Wang, Y. (2012a). Characterization of aeukaryotic translation initiation factor 5A homolog from Tamarix androssowii involved in plant abiotic stress tolerance. BMC Plant Biol. 12:118. doi: 10.1186/1471-2229-12-118
Wang, Y., Tang, H., DeBarry, J. D., Tan, X., Li, J., Wang, X., et al. (2012b). MCScanX: a toolkit for detection and evolutionary analysis of gene synteny and collinearity. Nucleic Acids Res. 40:e49. doi: 10.1093/nar/gkr1293
Wang, L., Zhu, W., Fang, L., Sun, X., Su, L., Liang, Z., et al. (2014). Genome-wide identification of WRKY family genes and their response to cold stress in Vitis vinifera. BMC Plant Biol. 14:103. doi: 10.1186/1471-2229-14-103
Yao, L., Hao, X., Cao, H., Ding, C., Yang, Y., Wang, L., et al. (2020). ABA-dependent bZIP transcription factor, CsbZIP18, from Camellia sinensis negatively regulates freezing tolerance in Arabidopsis. Plant Cell Rep. 39, 553–565. doi: 10.1007/s00299-020-02512-4
Yooyongwech, S., Horigane, A. K., Yoshida, M., Yamaguchi, M., Sekozawa, Y., Sugaya, S., et al. (2008). Changes in aquaporin gene expression and magnetic resonance imaging of water status in peach tree flower buds during dormancy. Physiol. Plantarum. 134, 522–533. doi: 10.1111/j.1399-3054.2008.01143.x
Zhang, D. Y., Ali, Z., Wang, C. B., Xu, L., Yi, J. X., Xu, Z. L., et al. (2013). Genome-wide sequence characterization and expression analysis of major intrinsic proteins in soybean (Glycine max L.). PLoS One 8:e56312. doi: 10.1371/journal.pone.0056312
Zhi, Z., Lifu, Y., and Jun, G. (2016). Genome-wide identification of Jatropha curcas aquaporin genes and the comparative analysis provides insights into the gene family expansion and evolution in Hevea brasiliensis. Front. Plant Sci. 7:395. doi: 10.3389/fpls.2016.00395
Zhou, S., Hu, W., Deng, X., Ma, Z., Chen, L., Huang, C., et al. (2012). Overexpression of the wheat aquaporin gene, TaAQP7, enhances drought tolerance in transgenic tobacco. PLoS One 7:e52439. doi: 10.1371/journal.pone.0052439
Zhu, Y., Liu, X., Gao, Y., Li, K., and Guo, W. (2021). Transcriptome-based identification of AP2/ERF family genes and their cold-regulated expression during the dormancy phase transition of Chinese cherry flower buds. Sci. Hortic. 275:109666. doi: 10.1016/j.scienta.2020.109666
Zorina, A. A., Bedbenov, V. S., Novikova, G. V., Panichkin, V. B., and Los’, D. A. (2014). Involvement of serine/threonine protein kinases in the cold stress response in the cyanobacterium Synechocystis sp. PCC 6803: functional characterization of SpkE protein kinase. Mol. Biol. 48, 390–398. doi: 10.1134/S0026893314030212
Keywords: cold resistance, functional analysis, genome-wide analysis, aquaporin gene, kernel-using apricot (P. armeniaca L.)
Citation: Li S, Wang L, Zhang Y, Zhu G, Zhu X, Xia Y, Li J, Gao X, Wang S, Zhang J, Wuyun T-n and Mo W (2021) Genome-Wide Identification and Function of Aquaporin Genes During Dormancy and Sprouting Periods of Kernel-Using Apricot (Prunus armeniaca L.). Front. Plant Sci. 12:690040. doi: 10.3389/fpls.2021.690040
Received: 02 April 2021; Accepted: 27 July 2021;
Published: 04 October 2021.
Edited by:
Hisayo Yamane, Kyoto University, JapanReviewed by:
Jorge Lora, Consejo Superior de Investigaciones Científicas, Spanish National Research Council (CSIC), SpainYuto Kitamura, Setsunan University, Japan
Copyright © 2021 Li, Wang, Zhang, Zhu, Zhu, Xia, Li, Gao, Wang, Zhang, Wuyun and Mo. This is an open-access article distributed under the terms of the Creative Commons Attribution License (CC BY). The use, distribution or reproduction in other forums is permitted, provided the original author(s) and the copyright owner(s) are credited and that the original publication in this journal is cited, in accordance with accepted academic practice. No use, distribution or reproduction is permitted which does not comply with these terms.
*Correspondence: Shaoli Wang, d3NoYW9saUBpY2Nhcy5hYy5jbg==; Jianhui Zhang, amlhbmh1aS56aGFuZ0Btb250YW5hLmVkdQ==; Ta-na Wuyun, dGFuYXRhbmFuQDE2My5jb20=