- 1Department of Biomedical Sciences, Heritage College of Osteopathic Medicine, Ohio University, Athens, OH, United States
- 2Ohio Musculoskeletal and Neurological Institute, Ohio University, Athens, OH, United States
- 3School of Chemistry and Biosciences, Faculty of Life Sciences, University of Bradford, Bradford, United Kingdom
Chronic alcohol-related myopathy (CAM), characterized by muscle atrophy and weakness, arises from prolonged excessive ethanol (EtOH) intake. The precise mechanisms by which EtOH induces skeletal muscle atrophy are not fully understood. This article posits that the pathophysiology of CAM may be significantly influenced by how EtOH modifies lipid profiles and alters lipid composition and content in skeletal muscle. We review existing literature on lipid alterations in CAM-afflicted individuals and analogous animal models, discuss EtOH’s direct and indirect effects on skeletal muscle lipids, and present specific instances where lipids contribute to muscle atrophy. This article advocates for a novel viewpoint, suggesting that lipid dysregulation may be the principal factor in EtOH-induced muscle wasting, offering a different angle to approach CAM research and treatment strategies.
Introduction
Chronic consumption of alcohol leads to various histological, biochemical, and physiological changes in skeletal muscle (Lang et al., 2005). These changes can result in chronic alcohol-related myopathy (CAM), a disorder marked by muscle wasting and weakness, particularly in fast-twitch muscles (Preedy et al., 2001). The degree of skeletal muscle atrophy correlates with the amount of alcohol consumed over a lifetime, potentially eroding up to 20% of total muscle mass and significantly reducing strength under extreme conditions (Ekbom et al., 1964; Rossouw et al., 1976; Martin et al., 1985; Estruch et al., 1998; Aagaard et al., 2003). Affecting approximately 40%–60% of chronic alcoholics, CAM is more prevalent than alcohol-induced liver cirrhosis yet remains under-researched (Preedy et al., 2003). The intricate pathophysiological mechanisms that contribute to the development and progression of CAM are still to be fully elucidated.
Preclinical studies in rodents have enhanced our understanding of CAM. Rodent studies, utilizing various methods of ethanol (EtOH) administration (such as liquid diet or drinking water), consistently demonstrate significant muscle atrophy and weakness following prolonged EtOH exposure (Lang et al., 1999; Crowell et al., 2019; Moser et al., 2022; Moser et al., 2023; Ganjayi et al., 2023). These effects are observed independently of caloric intake and other influential factors like diet and lifestyle, suggesting an obvious link between EtOH and CAM. Historically, research has concentrated on EtOH’s role in diminishing muscle mass via impeding protein synthesis via mTORC1 signaling (Preedy et al., 1991; Lang et al., 2001; Steiner and Lang, 2015; Simon et al., 2023). However, these studies narrow the focus on this single mechanism, potentially neglecting other contributing or upstream factors. For this article, we suggest that the onset and progression of CAM might also be attributed to EtOH’s direct or indirect effects on skeletal muscle lipids.
Impact of chronic EtOH consumption on skeletal muscle lipid profiles
Chronic EtOH consumption has a profound impact on lipid composition and metabolism in skeletal muscle, as evidenced by a series of studies spanning several decades. To our knowledge, the earliest known investigation by Sunnasy et al. (1983) revealed that chronic alcoholics (at least 100 g of EtOH daily for 3 years) with myopathy had a 53% increase in total lipid content in the quadriceps muscle, primarily due to triglycerides. This was characterized by elevated levels of palmitate (16:0), oleate (18:1), and arachidate (20:0), and lower levels of myristate (14:0), stearate (18:0), and linoleate (18:3).
Further research in male Wistar rats demonstrated that 6 weeks of EtOH consumption (75 mmol/kg body weight) altered fatty acid composition in soleus and plantaris muscles, with linoleic (18:2) and oleic (18:1) fatty acids increasing and decreasing, respectively (Salem et al., 2006). This was complemented by others (Kulagina et al., 2018) who demonstrated a duration-dependent response in the gastrocnemius muscle of male Wistar rats that consumed a 10% aqueous EtOH solution plus a 30% EtOH solution in agar blocks. After 12 weeks, myristic, vaccinic, dihomo-γ-linolenic, ω-6-decosapentaenoic, palmitic, palmitoleic, oleic, and linoleic fatty acids increased (or tended to increase), whereas at 24 weeks total fatty acid content was lower, with myristic (14:0), oleic (C18:1, ω-9), linoleic (C18:2, ω-6), α and γ linolenic (c18:3, ω-6, ω-3), eicosadienoic (C20:2), and polyunsaturated fatty acids all decreasing.
A more recent study using the gastrocnemius muscle from male C57BL/6 mice consuming EtOH that accounted for 27.5% of total calories for 4 weeks provided additional insights (Zhao et al., 2011). Zhao et al. (2011) found that while total lipids remained unchanged, individual lipids containing 18:3, 18:2, 18:1, and/or 18:0 fatty acids increased 41%–152%, whereas levels of 16:0/20:4 phosphatidylcholines (PC) and 16:0/22:6 PC decreased 29%–35%. Furthermore, Koh et al. (2020) reported that triglycerides accumulated in skeletal muscle after 4 weeks of 5% EtOH intake (specific muscle and sex of the mice were unspecified). These clinical and preclinical findings collectively underscore the significant regulatory effects of EtOH on the skeletal muscle lipid profile, which has implications for understanding the pathophysiology of alcohol-related muscle disorders and the development of therapeutic strategies.
Mechanisms by which EtOH may be altering skeletal muscle lipids
Chronic EtOH consumption appears to affect muscle tissue lipid profiles through direct and indirect pathways (Figure 1). Directly, EtOH can alter lipid profiles via several interrelated mechanisms: generation of reactive oxygen species (ROS), triggering of a proinflammatory response, and disrupting mitochondrial function. For instance, the activity of the glutathione peroxidase system is known to decrease in chronic alcoholics and in rodent models of excessive EtOH consumption (Guerri and Grisolía, 1980; Fernández-Solà et al., 2002; Otis and Guidot, 2009). Concomitantly, ROS production in skeletal muscle is increased after chronic EtOH intake, as evidenced by elevated protein carbonylation and lipid peroxidation (Adachi et al., 2003a; Otis et al., 2007; Kumar et al., 2019; Ganjayi et al., 2023). Persistent EtOH-induced ROS production can lead to membrane damage due to lipid modifications (Simon et al., 2023), which in turn stimulates the production of proinflammatory cytokines such as TNF-alpha (Patel and Patel, 2017) and IL-6 (Steiner et al., 2015). These cytokines further increase free radical production and levels of oxidative stress (Otis and Guidot, 2009). Prolonged exposure to ROS and proinflammatory cytokines impairs mitochondrial function (Kumar et al., 2019), favoring metabolic inflexibility. Short-term EtOH consumption has also been shown to alter cholesterol metabolism in skeletal muscle, leading to increased levels of oxysterols (Adachi et al., 2000), which persist even after several months of intake (Adachi et al., 2003b). Oxysterols and cholesterol-derived hydroperoxides found in skeletal muscle following excessive EtOH intake have been suggested to signify perturbations in membrane lipids (Adachi et al., 2000; Fujita et al., 2002), including the mitochondrial membranes. Chronic EtOH exposure in muscle cells, specifically cardiac cells, has been reported to decrease mitochondrial membrane potential, reducing mitochondrial function, mitochondrial content, and fatty acid oxidation (Hwang et al., 2023). Taken together, these data demonstrate how EtOH can directly impact skeletal muscle lipids (Figure 1), such as phospholipids, and influence muscle lipid concentrations by reducing mitochondrial function and content.
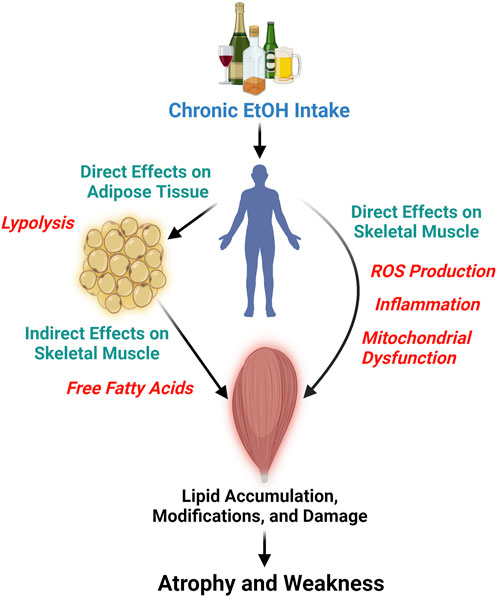
Figure 1. This diagram illustrates the detrimental effects of chronic and persistent alcohol (ethanol, EtOH) consumption on skeletal muscle, leading to atrophy and weakness. It highlights the direct impact of EtOH on skeletal muscle lipids through the generation of reactive oxygen species (ROS), triggering inflammation, and mitochondrial dysfunction, which are likely interconnected processes. Furthermore, the figure depicts the indirect effects of EtOH on skeletal muscle lipids, mediated by its action on other tissues, such as adipose tissue. Here, EtOH induces lipolysis in adipocytes, elevating the levels of free fatty acids that may subsequently infiltrate skeletal muscle. Figure was created using BioRender.
Indirectly, chronic EtOH exposure dysregulates the lipid profile of other bodily tissues and organs, which in turn can impact skeletal muscle (Figure 1). EtOH specifically has been shown to cause lipolysis in adipose tissue through EtOH-induced secretion of adipokines and activation of adipose triglyceride, hormone sensitive, and monoglyceride lipases (Kang et al., 2007b; Zhong et al., 2012). This tissue-specific lipolysis causes a surge of excess free fatty acids into the bloodstream, which is seen as dyslipidemia in chronic alcoholics and pre-clinical rodent models of excessive EtOH intake (Kang et al., 2007a; Kema et al., 2015). Free fatty acids and other circulating lipids can then enter skeletal muscle causing lipid accumulation, lipid modifications, and influence cellular metabolism (Rubin et al., 1976; Jensen, 2002; Schwenk et al., 2010; Watt and Hoy, 2012; van Hall, 2015; Lipina and Hundal, 2017). Indeed, high fat feeding plus chronic EtOH intake increased lipid peroxidation in mouse skeletal muscle beyond that of EtOH alone (Ismaeel et al., 2022). Here, we provide an example of an indirect pathway that specifically involves adipose-skeletal muscle crosstalk but also acknowledge, that due to EtOH’s widespread effect, other tissue interactions also exist (e.g., liver-skeletal muscle) (Welch et al., 2020).
Lipids can cause skeletal muscle atrophy
The relationship between EtOH-induced changes in skeletal muscle lipid and muscle atrophy is complex and incompletely defined. It has been reported that downregulation of cardiolipin synthase (crucial for cardiolipin production) reduces myofiber cross-sectional area, muscle mass, and force in the tibialis anterior muscle of young mice (Yoo et al., 2024). Furthermore, others have demonstrated that reductions in lysophospholipids content (particularly lyso-PC) in young mice made the extensor digitorum longus muscle 20% weaker (Ferrara et al., 2021). Additionally, treating muscle cells with palmitate, which leads to ceramide accumulation, increased expression of pro-atrophic genes expression and reduced protein synthesis rates (Woodworth-Hobbs et al., 2014). Similarly, in drosophila, muscle-specific knockdown of phosphatidylserine synthase increased rates of apoptosis and autophagy, reduced muscle mass, and impaired motor function (Kim et al., 2024). Though more correlative, several preclinical studies using denervation, immobilization, and high fat feeding demonstrated that intramyocellular lipids (including triglycerides and diglycerides) increased in skeletal muscle and were associated with changes in muscle mass (Kumar and Sharma, 2009; Vigelsø et al., 2016; Fan and Xiao, 2020; Kimura et al., 2021). In humans, unfavorable changes in muscle PC and phosphatidylethanolamine (PE) content also correlate with reduced insulin sensitivity (Newsom et al., 2016) and age-related declines in muscle size and strength (Hinkley et al., 2020).
Based on the lipid species reported to change due to chronic alcohol consumption, we posit that alcohol-induced alterations in lipid metabolism and transport (e.g., skeletal muscle lipid uptake, storage, and oxidation) result in lipotoxicity, leading to apoptosis. Briefly, changes in lipid metabolism can alter membrane compositions, protein distribution and function, and gene expression. Free fatty acids play vital roles, including energy generation and reserve, components of the cell membrane, and ligands for nuclear receptors (de Vries et al., 1997; Turner et al., 2014; Al Saedi et al., 2022). However, disturbances in fatty acid homeostasis, such as inefficient metabolism or intensified release from storage sites, may result in increased free fatty acid levels, leading to an unfavorable accumulation of intracellular lipids (Figure 1). Cells can adjust to free fatty acid intake to a limited extent, yet prolonged exposure to free fatty acids can become deleterious, impairing mitochondrial function, generating ROS, and producing proinflammatory cytokines. Indeed, overloading cells with palmitate, palmitic acid, or ceramides can cause lipotoxicity and apoptosis in otherwise healthy cells (de Vries et al., 1997; Lin et al., 2012; Shan et al., 2013; Yuan et al., 2013; Zorov et al., 2014; Zhang et al., 2017; Li et al., 2019; Mansuri et al., 2021). In the presence of alcohol, apoptosis has been implicated as a mechanism leading to cellular damage in cardiomyocytes, hepatocytes, endothelial cells, thymocytes, lymphocytes, and neural cells (Ewald and Shao, 1993; Beckemeier and Bora, 1998; Baker et al., 1999; Spyridopoulos et al., 2001; Yin and Ding, 2003; Hwang et al., 2005; Pasala et al., 2015; Jan and Chaudhry, 2019). We are aware of only a few studies that assessed apoptosis in chronic alcohol myopathy (CAM) (Fernández-Solà et al., 2002; FernÁndez-SolÀ et al., 2003; Nakahara et al., 2003). The most well-designed and in-depth was conducted in skeletal muscle biopsies of 30 male high-dose well-nourished chronic alcohol consumers and 12 nonalcoholic controls, with apoptosis being assessed by TUNEL, BAX, and BCL-2 immunohistochemical assays (FernÁndez-SolÀ et al., 2003). Chronic alcoholics had significantly higher apoptotic indices in TUNEL, BAX, and BCL-2 muscle assays, and apoptotic indices were higher in alcoholics with skeletal myopathy compared to those without skeletal myopathy (FernÁndez-SolÀ et al., 2003). It can therefore be speculated that alcohol-induced lipoapoptosis could be occurring in skeletal muscle, causing atrophy and weakness in individuals and animals that consume alcohol chronically. These findings collectively underscore the complex relationship between lipid composition and muscle health and offer valuable insights into underlying mechanisms by which lipids may contribute to alcohol-induced tissue dysfunction.
Future directions
The current article highlights the significance of lipids as a primary factor influencing CAM. While the existing descriptive data provides valuable insights, it lacks a definitive causal link. To advance our understanding, comprehensive omics studies that focus on the skeletal muscle lipidome are essential. Identifying specific lipids altered by chronic EtOH consumption will pave the way for targeted isolation of lipids that affect muscle size. This approach will enable more focused mechanistic studies to investigate the precise impact of lipids on protein anabolic and catabolic pathways. Such data is crucial for the field, as it extends the literature base beyond what is typically studied in CAM, by aiming to clarify the role of lipids in modulating specific gene and protein alterations.
Conclusion
We suggest that the development and progression of CAM may be due, in part, to the direct or indirect influence of EtOH on skeletal muscle lipids (Figure 1). Chronic EtOH consumption significantly alters lipid composition and metabolism within skeletal muscle, a fact supported by numerous studies published over several decades. These lipid alterations can significantly impact muscle size and function. Recent research has shown that specific changes in lipids can modulate anabolic and catabolic signaling pathways in conditions such as aging, diabetes, and cancer cachexia (Das et al., 2011; Gilbert, 2021; Al Saedi et al., 2022). In summary, this article posits that lipids play a key role in the pathogenesis of CAM, with further research necessary to substantiate this hypothesis.
Author contributions
MG: Conceptualization, Writing–original draft, Writing–review and editing. TK: Writing–original draft, Writing–review and editing. CW: Writing–original draft, Writing–review and editing. CB: Conceptualization, Funding acquisition, Resources, Supervision, Writing–original draft, Writing–review and editing.
Funding
The author(s) declare that financial support was received for the research, authorship, and/or publication of this article. CB acknowledges the support of the Osteopathic Heritage Foundation through funding for the Ralph S. Licklider, D.O., Endowed Faculty Fellowship in the Heritage College of Osteopathic Medicine.
Conflict of interest
The authors declare that the research was conducted in the absence of any commercial or financial relationships that could be construed as a potential conflict of interest.
The handling editor JO declared a past collaboration with the author CB.
Publisher’s note
All claims expressed in this article are solely those of the authors and do not necessarily represent those of their affiliated organizations, or those of the publisher, the editors and the reviewers. Any product that may be evaluated in this article, or claim that may be made by its manufacturer, is not guaranteed or endorsed by the publisher.
Abbreviations
CAM, chronic alcohol-related myopathy; EtOH, ethanol; PC, phosphatidylcholines; PE, phosphatidylethanolamine; ROS, reactive oxygen species.
References
Aagaard N. K., Andersen H., Vilstrup H., Clausen T., Jakobsen J., Dørup I. (2003). Decreased muscle strength and contents of Mg and Na,K-pumps in chronic alcoholics occur independently of liver cirrhosis. J. Intern Med. 253, 359–366. doi:10.1046/j.1365-2796.2003.01100.x
Adachi J., Asano M., Ueno Y., Niemelä O., Ohlendieck K., Peters T. J., et al. (2003a). Alcoholic muscle disease and biomembrane perturbations (review). J. Nutr. Biochem. 14, 616–625. doi:10.1016/s0955-2863(03)00114-1
Adachi J., Asano M., Ueno Y., Reilly M., Mantle D., Peters T. J., et al. (2000). 7alpha- and 7beta-hydroperoxycholest-5-en-3beta-ol in muscle as indices of oxidative stress: response to ethanol dosage in rats. Alcohol Clin. Exp. Res. 24, 675–681. doi:10.1097/00000374-200005000-00012
Adachi J., Fujita T., Kudo R., Asano M., Nurhantari Y., Ueno Y. (2003b). 7-hydroperoxycholesterol and oxysterols as indices of oxidative stress: chronic ethanol feeding and rat skeletal muscle. Leg. Med. (Tokyo) 5 (Suppl. 1), S105–S109. doi:10.1016/s1344-6223(02)00077-9
Al Saedi A., Debruin D. A., Hayes A., Hamrick M. (2022). Lipid metabolism in sarcopenia. Bone 164, 116539. doi:10.1016/j.bone.2022.116539
Baker K. G., Harding A. J., Halliday G. M., Kril J. J., Harper C. G. (1999). Neuronal loss in functional zones of the cerebellum of chronic alcoholics with and without Wernicke’s encephalopathy. Neuroscience 91, 429–438. doi:10.1016/S0306-4522(98)90664-9
Beckemeier M. E., Bora P. S. (1998). Fatty acid ethyl esters: potentially toxic products of myocardial ethanol metabolism. J. Mol. Cell Cardiol. 30, 2487–2494. doi:10.1006/jmcc.1998.0812
Crowell K. T., Laufenberg L. J., Lang C. H. (2019). Chronic alcohol consumption, but not acute intoxication, decreases in vitro skeletal muscle contractile function. Alcohol Clin. Exp. Res. 43, 2090–2099. doi:10.1111/acer.14179
Das S. K., Eder S., Schauer S., Diwoky C., Temmel H., Guertl B., et al. (2011). Adipose triglyceride lipase contributes to cancer-associated cachexia. Science 333, 233–238. doi:10.1126/science.1198973
de Vries J. E., Vork M. M., Roemen T. H., de Jong Y. F., Cleutjens J. P., van der Vusse G. J., et al. (1997). Saturated but not mono-unsaturated fatty acids induce apoptotic cell death in neonatal rat ventricular myocytes. J. Lipid Res. 38, 1384–1394. doi:10.1016/s0022-2275(20)37421-6
Ekbom K., Hed R., Kirstein L., Astrom K. E. (1964). Muscular affections in chronic alcoholism. Arch. Neurol. 10, 449–458. doi:10.1001/archneur.1964.00460170019003
Estruch R., Sacanella E., Fernández-Solá J., Nicolás J. M., Rubin E., Urbano-Márquez A. (1998). Natural history of alcoholic myopathy: a 5-year study. Alcohol Clin. Exp. Res. 22, 2023–2028. doi:10.1111/j.1530-0277.1998.tb05911.x
Ewald S. J., Shao H. (1993). Ethanol increases apoptotic cell death of thymocytes in vitro. Alcohol Clin. Exp. Res. 17, 359–365. doi:10.1111/j.1530-0277.1993.tb00776.x
Fan Z., Xiao Q. (2020). Impaired autophagic flux contributes to muscle atrophy in obesity by affecting muscle degradation and regeneration. Biochem. Biophys. Res. Commun. 525, 462–468. doi:10.1016/j.bbrc.2020.02.110
Fernández-Solà J., García G., Elena M., Tobías E., Sacanella E., Estruch R., et al. (2002). Muscle antioxidant status in chronic alcoholism. Alcohol Clin. Exp. Res. 26, 1858–1862. doi:10.1111/j.1530-0277.2002.tb02493.x
FernÁndez-SolÀ J., NicolÁs J.-M., FatjÓ F., GarcÍa G., Sacanella E., Estruch R., et al. (2003). Evidence of apoptosis in chronic alcoholic skeletal myopathy. Hum. Pathol. 34, 1247–1252. doi:10.1016/j.humpath.2003.07.017
Ferrara P. J., Verkerke A. R. P., Maschek J. A., Shahtout J. L., Siripoksup P., Eshima H., et al. (2021). Low lysophosphatidylcholine induces skeletal muscle myopathy that is aggravated by high-fat diet feeding. FASEB J. 35, e21867. doi:10.1096/fj.202101104R
Fujita T., Adachi J., Ueno Y., Peters T. J., Preedy V. R. (2002). Chronic ethanol feeding increases 7-hydroperoxycholesterol and oxysterols in rat skeletal muscle. Metabolism 51, 737–742. doi:10.1053/meta.2002.32803
Ganjayi M. S., Brown A. M., Baumann C. W. (2023). Longitudinal assessment of strength and body composition in a mouse model of chronic alcohol-related myopathy. Alcohol, Clin. and Exp. Res. 47, 1653–1664. doi:10.1111/acer.15149
Gilbert M. (2021). Role of skeletal muscle lipids in the pathogenesis of insulin resistance of obesity and type 2 diabetes. J. Diabetes Investig. 12, 1934–1941. doi:10.1111/jdi.13614
Guerri C., Grisolía S. (1980). Changes in glutathione in acute and chronic alcohol intoxication. Pharmacol. Biochem. Behav. 13, 53–61. doi:10.1016/S0091-3057(80)80009-8
Hinkley J. M., Cornnell H. H., Standley R. A., Chen E. Y., Narain N. R., Greenwood B. P., et al. (2020). Older adults with sarcopenia have distinct skeletal muscle phosphodiester, phosphocreatine, and phospholipid profiles. Aging Cell 19, e13135. doi:10.1111/acel.13135
Hwang H., Liu R., Eldridge R., Hu X., Forghani P., Jones D. P., et al. (2023). Chronic ethanol exposure induces mitochondrial dysfunction and alters gene expression and metabolism in human cardiac spheroids. Alcohol, Clin. and Exp. Res. 47, 643–658. doi:10.1111/acer.15026
Hwang J.-T., Park I.-J., Shin J.-I., Lee Y. K., Lee S. K., Baik H. W., et al. (2005). Genistein, EGCG, and capsaicin inhibit adipocyte differentiation process via activating AMP-activated protein kinase. Biochem. Biophys. Res. Commun. 338, 694–699. doi:10.1016/j.bbrc.2005.09.195
Ismaeel A., Laudato J. A., Fletcher E., Papoutsi E., Tice A., Hwa L. S., et al. (2022). High-fat diet augments the effect of alcohol on skeletal muscle mitochondrial dysfunction in mice. Nutrients 14, 1016. doi:10.3390/nu14051016
Jan R., Chaudhry G.-S. (2019). Understanding apoptosis and apoptotic pathways targeted cancer therapeutics. Adv. Pharm. Bull. 9, 205–218. doi:10.15171/apb.2019.024
Jensen M. D. (2002). Fatty acid oxidation in human skeletal muscle. J. Clin. Invest. 110, 1607–1609. doi:10.1172/JCI17303
Kang L., Chen X., Sebastian B. M., Pratt B. T., Bederman I. R., Alexander J. C., et al. (2007a). Chronic ethanol and triglyceride turnover in white adipose tissue in rats: inhibition of the anti-lipolytic action of insulin after chronic ethanol contributes to increased triglyceride degradation. J. Biol. Chem. 282, 28465–28473. doi:10.1074/jbc.M705503200
Kang L., Sebastian B. M., Pritchard M. T., Pratt B. T., Previs S. F., Nagy L. E. (2007b). Chronic ethanol-induced insulin resistance is associated with macrophage infiltration into adipose tissue and altered expression of adipocytokines. Alcohol Clin. Exp. Res. 31, 1581–1588. doi:10.1111/j.1530-0277.2007.00452.x
Kema V. H., Mojerla N. R., Khan I., Mandal P. (2015). Effect of alcohol on adipose tissue: a review on ethanol mediated adipose tissue injury. Adipocyte 4, 225–231. doi:10.1080/21623945.2015.1017170
Kim S., Heo H., Kwon S.-H., Park J. H., Lee G., Jeon S.-H. (2024). Loss of function of phosphatidylserine synthase causes muscle atrophy in Drosophila. Dev. Biol. 511, 1–11. doi:10.1016/j.ydbio.2024.03.006
Kimura K., Morisasa M., Mizushige T., Karasawa R., Kanamaru C., Kabuyama Y., et al. (2021). Lipid dynamics due to muscle atrophy induced by immobilization. J. Oleo Sci. 70, 937–946. doi:10.5650/jos.ess21045
Koh J. H., Kim K. H., Park S. Y., Kim Y. W., Kim J. Y. (2020). PPARδ attenuates alcohol-mediated insulin resistance by enhancing fatty acid-induced mitochondrial uncoupling and antioxidant defense in skeletal muscle. Front. Physiol. 11, 749. doi:10.3389/fphys.2020.00749
Kulagina T. P., Gritsyna Yu. V., Aripovsky A. V., Zhalimov V. K., Vikhlyantsev I. M. (2018). Fatty acid levels in striated muscles of chronic alcohol-fed rats. Biophys. (Oxf) 63, 805–813. doi:10.1134/S0006350918050135
Kumar A., Davuluri G., Welch N., Kim A., Gangadhariah M., Allawy A., et al. (2019). Oxidative stress mediates ethanol-induced skeletal muscle mitochondrial dysfunction and dysregulated protein synthesis and autophagy. Free Radic. Biol. Med. 145, 284–299. doi:10.1016/j.freeradbiomed.2019.09.031
Kumar R., Sharma S. (2009). Lipid profile changes in mouse gastrocnemius muscle after denervation and beta-adrenoceptor stimulation. Indian J. Exp. Biol. 47, 314–319.
Lang C. H., Frost R. A., Summer A. D., Vary T. C. (2005). Molecular mechanisms responsible for alcohol-induced myopathy in skeletal muscle and heart. Int. J. Biochem. Cell Biol. 37, 2180–2195. doi:10.1016/j.biocel.2005.04.013
Lang C. H., Kimball S. R., Frost R. A., Vary T. C. (2001). Alcohol myopathy: impairment of protein synthesis and translation initiation. Int. J. Biochem. Cell Biol. 33, 457–473. doi:10.1016/s1357-2725(00)00081-9
Lang C. H., Wu D., Frost R. A., Jefferson L. S., Kimball S. R., Vary T. C. (1999). Inhibition of muscle protein synthesis by alcohol is associated with modulation of eIF2B and eIF4E. Am. J. Physiol. 277, E268–E276. doi:10.1152/ajpendo.1999.277.2.E268
Li X., Wei X., Sun Y., Du J., Li X., Xun Z., et al. (2019). High-fat diet promotes experimental colitis by inducing oxidative stress in the colon. Am. J. Physiology-Gastrointestinal Liver Physiology 317, G453–G462. doi:10.1152/ajpgi.00103.2019
Lin N., Chen H., Zhang H., Wan X., Su Q. (2012). Mitochondrial reactive oxygen species (ROS) inhibition ameliorates palmitate-induced INS-1 beta cell death. Endocrine 42, 107–117. doi:10.1007/s12020-012-9633-z
Lipina C., Hundal H. S. (2017). Lipid modulation of skeletal muscle mass and function. J. Cachexia Sarcopenia Muscle 8, 190–201. doi:10.1002/jcsm.12144
Mansuri M. L., Sharma G., Parihar P., Dube K. T., Sharma T., Parihar A., et al. (2021). Increased oxidative stress and mitochondrial impairments associated with increased expression of TNF-α and caspase-3 in palmitic acid-induced lipotoxicity in myoblasts. J. Biochem. Mol. Toxicol. 35, e22744. doi:10.1002/jbt.22744
Martin F., Ward K., Slavin G., Levi J., Peters T. J. (1985). Alcoholic skeletal myopathy, a clinical and pathological study. Q. J. Med. 55, 233–251.
Moser S. E., Brown A. M., Clark B. C., Arnold W. D., Baumann C. W. (2022). Neuromuscular mechanisms of weakness in a mouse model of chronic alcoholic myopathy. Alcohol Clin. Exp. Res. 46, 1636–1647. doi:10.1111/acer.14907
Moser S. E., Brown A. M., Ganjayi M. S., Otis J. S., Baumann C. W. (2023). Excessive ethanol intake in mice does not impair recovery of torque after repeated bouts of eccentric contractions. Med. Sci. Sports Exerc 55, 873–883. doi:10.1249/MSS.0000000000003118
Nakahara T., Hashimoto K., Hirano M., Koll M., Martin C. R., Preedy V. R. (2003). Acute and chronic effects of alcohol exposure on skeletal muscle c-myc, p53, and Bcl-2 mRNA expression. Am. J. Physiology-Endocrinology Metabolism 285, E1273–E1281. doi:10.1152/ajpendo.00019.2003
Newsom S. A., Brozinick J. T., Kiseljak-Vassiliades K., Strauss A. N., Bacon S. D., Kerege A. A., et al. (2016). Skeletal muscle phosphatidylcholine and phosphatidylethanolamine are related to insulin sensitivity and respond to acute exercise in humans. J. Appl. Physiol. (1985) 120, 1355–1363. doi:10.1152/japplphysiol.00664.2015
Otis J. S., Brown L. A. S., Guidot D. M. (2007). Oxidant-induced atrogin-1 and transforming growth factor-beta1 precede alcohol-related myopathy in rats. Muscle Nerve 36, 842–848. doi:10.1002/mus.20883
Otis J. S., Guidot D. M. (2009). Procysteine stimulates expression of key anabolic factors and reduces plantaris atrophy in alcohol-fed rats. Alcohol Clin. Exp. Res. 33, 1450–1459. doi:10.1111/j.1530-0277.2009.00975.x
Pasala S., Barr T., Messaoudi I. (2015). Impact of alcohol abuse on the adaptive immune system. Alcohol Res. 37, 185–197.
Patel H. J., Patel B. M. (2017). TNF-α and cancer cachexia: molecular insights and clinical implications. Life Sci. 170, 56–63. doi:10.1016/j.lfs.2016.11.033
Preedy V. R., Adachi J., Ueno Y., Ahmed S., Mantle D., Mullatti N., et al. (2001). Alcoholic skeletal muscle myopathy: definitions, features, contribution of neuropathy, impact and diagnosis. Eur. J. Neurol. 8, 677–687. doi:10.1046/j.1468-1331.2001.00303.x
Preedy V. R., Edwards P., Peters T. J. (1991). Ethanol-induced reductions in skeletal muscle protein synthesis: use of the inhibitors of alcohol and aldehyde dehydrogenase. Biochem. Soc. Trans. 19, 167S. doi:10.1042/bst019167s
Preedy V. R., Ohlendieck K., Adachi J., Koll M., Sneddon A., Hunter R., et al. (2003). The importance of alcohol-induced muscle disease. J. Muscle Res. Cell Motil. 24, 55–63. doi:10.1023/a:1024842817060
Rossouw J. E., Keeton R. G., Hewlett R. H. (1976). Chronic proximal muscular weakness in alcoholics. S Afr. Med. J. 50, 2095–2098.
Rubin E., Katz A. M., Lieber C. S., Stein E. P., Puszkin S. (1976). Muscle damage produced by chronic alcohol consumption. Am. J. Pathol. 83, 499–516.
Salem R. O., Laposata M., Rajendram R., Cluette-Brown J. E., And Preedy V. R. (2006). The total body mass of fatty acid ethyl esters in skeletal muscles following ethanol exposure greatly exceeds that found in the liver and the heart. Alcohol Alcohol. 41, 598–603. doi:10.1093/alcalc/agl069
Schwenk R. W., Holloway G. P., Luiken J. J. F. P., Bonen A., Glatz J. F. C. (2010). Fatty acid transport across the cell membrane: regulation by fatty acid transporters. Prostagl. Leukot. Essent. Fat. Acids (PLEFA) 82, 149–154. doi:10.1016/j.plefa.2010.02.029
Shan X., Miao Y., Fan R., Song C., Wu G., Wan Z., et al. (2013). Suppression of Grb2 expression improved hepatic steatosis, oxidative stress, and apoptosis induced by palmitic acid in vitro partly through insulin signaling alteration. Vitro Cell Dev. Biol. Anim. 49, 576–582. doi:10.1007/s11626-013-9646-9
Simon L., Bourgeois B. L., Molina P. E. (2023). Alcohol and skeletal muscle in health and disease. Alcohol Res. 43, 04. doi:10.35946/arcr.v43.1.04
Spyridopoulos I., Wischhusen J., Rabenstein B., Mayer P., Axel D. I., Frohlich K.-U., et al. (2001). Alcohol enhances oxysterol-induced apoptosis in human endothelial cells by a calcium-dependent mechanism. Arterioscler. Thromb. Vasc. Biol. 21, 439–444. doi:10.1161/01.ATV.21.3.439
Steiner J. L., Lang C. H. (2015). Dysregulation of skeletal muscle protein metabolism by alcohol. Am. J. Physiol. Endocrinol. Metab. 308, E699–E712. doi:10.1152/ajpendo.00006.2015
Steiner J. L., Pruznak A. M., Navaratnarajah M., Lang C. H. (2015). Alcohol differentially alters extracellular matrix and adhesion molecule expression in skeletal muscle and heart. Alcohol Clin. Exp. Res. 39, 1330–1340. doi:10.1111/acer.12771
Sunnasy D., Cairns S. R., Martin F., Slavin G., Peters T. J. (1983). Chronic alcoholic skeletal muscle myopathy: a clinical, histological and biochemical assessment of muscle lipid. J. Clin. Pathol. 36, 778–784. doi:10.1136/jcp.36.7.778
Turner N., Cooney G. J., Kraegen E. W., Bruce C. R. (2014). Fatty acid metabolism, energy expenditure and insulin resistance in muscle. J. Endocrinol. 220, T61–T79. doi:10.1530/JOE-13-0397
van Hall G. (2015). The physiological regulation of skeletal muscle fatty acid supply and oxidation during moderate-intensity exercise. Sports Med. 45, 23–32. doi:10.1007/s40279-015-0394-8
Vigelsø A., Gram M., Wiuff C., Hansen C. N., Prats C., Dela F., et al. (2016). Effects of immobilization and aerobic training on proteins related to intramuscular substrate storage and metabolism in young and older men. Eur. J. Appl. Physiol. 116, 481–494. doi:10.1007/s00421-015-3302-x
Watt M. J., Hoy A. J. (2012). Lipid metabolism in skeletal muscle: generation of adaptive and maladaptive intracellular signals for cellular function. Am. J. Physiology-Endocrinology Metabolism 302, E1315–E1328. doi:10.1152/ajpendo.00561.2011
Welch N., Dasarathy J., Runkana A., Penumatsa R., Bellar A., Reen J., et al. (2020). Continued muscle loss increases mortality in cirrhosis: impact of aetiology of liver disease. Liver Int. 40, 1178–1188. doi:10.1111/liv.14358
Woodworth-Hobbs M. E., Hudson M. B., Rahnert J. A., Zheng B., Franch H. A., Price S. R. (2014). Docosahexaenoic acid prevents palmitate-induced activation of proteolytic systems in C2C12 myotubes. J. Nutr. Biochem. 25, 868–874. doi:10.1016/j.jnutbio.2014.03.017
Yin X.-M., Ding W.-X. (2003). Death receptor activation-induced hepatocyte apoptosis and liver injury. Curr. Mol. Med. 3, 491–508. doi:10.2174/1566524033479555
Yoo Y., Yeon M., Kim W.-K., Shin H.-B., Lee S.-M., Yoon M.-S., et al. (2024). Age-dependent loss of Crls1 causes myopathy and skeletal muscle regeneration failure. Exp. Mol. Med. 56, 922–934. doi:10.1038/s12276-024-01199-x
Yuan Q., Zhao S., Wang F., Zhang H., Chen Z.-J., Wang J., et al. (2013). Palmitic acid increases apoptosis of neural stem cells via activating c-Jun N-terminal kinase. Stem Cell Res. 10, 257–266. doi:10.1016/j.scr.2012.11.008
Zhang Y., Xia G., Zhang Y., Liu J., Liu X., Li W., et al. (2017). Palmitate induces VSMC apoptosis via toll like receptor (TLR)4/ROS/p53 pathway. Atherosclerosis 263, 74–81. doi:10.1016/j.atherosclerosis.2017.06.002
Zhao Z., Yu M., Crabb D., Xu Y., Liangpunsakul S. (2011). Ethanol-induced alterations in fatty acid-related lipids in serum and tissues in mice. Alcohol Clin. Exp. Res. 35, 229–234. doi:10.1111/j.1530-0277.2010.01338.x
Zhong W., Zhao Y., Tang Y., Wei X., Shi X., Sun W., et al. (2012). Chronic alcohol exposure stimulates adipose tissue lipolysis in mice: role of reverse triglyceride transport in the pathogenesis of alcoholic steatosis. Am. J. Pathol. 180, 998–1007. doi:10.1016/j.ajpath.2011.11.017
Keywords: adipose tissue, atrophy, metabolism, skeletal muscle, weakness
Citation: Ganjayi MS, Krauss TA, Willis CRG and Baumann CW (2024) Chronic alcohol-related myopathy: a closer look at the role of lipids. Front. Physiol. 15:1492405. doi: 10.3389/fphys.2024.1492405
Received: 06 September 2024; Accepted: 08 November 2024;
Published: 18 November 2024.
Edited by:
Jeff Otis, Georgia State University, United StatesReviewed by:
Kate Kosmac, Augusta University, United StatesCopyright © 2024 Ganjayi, Krauss, Willis and Baumann. This is an open-access article distributed under the terms of the Creative Commons Attribution License (CC BY). The use, distribution or reproduction in other forums is permitted, provided the original author(s) and the copyright owner(s) are credited and that the original publication in this journal is cited, in accordance with accepted academic practice. No use, distribution or reproduction is permitted which does not comply with these terms.
*Correspondence: Cory W. Baumann, YmF1bWFubkBvaGlvLmVkdQ==