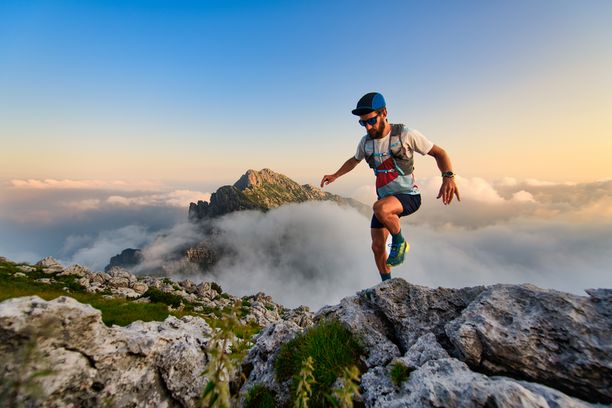
95% of researchers rate our articles as excellent or good
Learn more about the work of our research integrity team to safeguard the quality of each article we publish.
Find out more
MINI REVIEW article
Front. Physiol., 30 August 2023
Sec. Cell Physiology
Volume 14 - 2023 | https://doi.org/10.3389/fphys.2023.1188816
This article is part of the Research TopicKallikrein-Kinin System: Insights into a Multifunctional SystemView all 5 articles
Human plasma kallikrein (PKa) is obtained by activating its precursor, prekallikrein (PK), historically named the Fletcher factor. Human PKa and tissue kallikreins are serine proteases from the same family, having high- and low-molecular weight kininogens (HKs and LKs) as substrates, releasing bradykinin (Bk) and Lys-bradykinin (Lys-Bk), respectively. This review presents a brief history of human PKa with details and recent observations of its evolution among the vertebrate coagulation proteins, including the relations with Factor XI. We explored the role of Factor XII in activating the plasma kallikrein–kinin system (KKS), the mechanism of activity and control in the KKS, and the function of HK on contact activation proteins on cell membranes. The role of human PKa in cell biology regarding the contact system and KSS, particularly the endothelial cells, and neutrophils, in inflammatory processes and infectious diseases, was also approached. We examined the natural plasma protein inhibitors, including a detailed survey of human PKa inhibitors’ development and their potential market.
Human plasma kallikrein [E.C.3.4.21.34] (PKa), initially described by Hathaway et al. (1965), was later further characterized (Hathaway and Alsever, 1970) and then named the Fletcher factor (after the surname patient-family name). The detailed characterization and isolation of PKa were the first approaches (Moriya et al., 1965; Colman et al., 1969; McConnell and Mason, 1970; Sampaio et al., 1974).
Wuepper and Cochrane (1972) reported the effect of PKa on coagulation in vitro, and the studies with enzymes purified from human plasma established that PKa acts as a proteolytic activator of factor XII (FXII) (Revak et al., 1977).
Plasma deficiency in PKa presented alterations in coagulation tests, kinin release, fibrinolytic activity after plasma activation by kaolin, and chemotactic activity, all of them characterized by correction with plasma prekallikrein (PK), the zymogen form of PKa, that functions as a kinin releaser (Wuepper, 1973), chemotactic factor, and Hageman factor (FXII) activator (Weiss et al., 1974; Nakayasu and Nagasawa, 1979).
Detailed studies demonstrated the hepatic synthesis of PK and its secretion into the blood as a single polypeptide chain glycoprotein with two molecular species of molecular masses (MW) 88 kDa and 86 kDa (Nagase and Barrett, 1981), respectively, due to the differences in glycosylation extension. PK is activated to PKa by Factor XIIa (FXIIa), and the two species consist of a heavy chain (amino-terminal), with an MW of 43 kDa, and a light chain (carboxy-terminal), with two variants of MW 36 kDa or 33 kDa and occurring in equimolar amounts. The light-chain region contains the enzymatic active site, and hydrolyzes oligopeptide substrates, protein substrates, and the zymogen form of FXII. Nevertheless, the heavy chain participates in binding to high-molecular weight kininogen (HK), providing its optimal cleavage, and in the surface-dependent activation of coagulation (Mandle et al., 1976; Fisher et al., 1982; van der Graaf et al., 1982). Figure 1 shows the sequence and structure of PKa after activation by hydrolysis at the R371–I372 peptide bond, and a schematic structure from a high-resolution 1.3-Å structure of full-length PKa in the active conformation, including the apple domains, has been reported in detail (Li et al., 2019).
FIGURE 1. The sequence of PKa shows the apple domains A1 through A4 that constitute the heavy chain, linked to the catalytic domain (light chain) by a disulfide bound. The apple domains A1, A2, and A3 contain six cysteine residues linked by three disulfide bridges (C1-C6, C2-C5, and C3-C4; the numbers indicate the order of each cysteine in sequence). The apple domain A4 contains a fourth disulfide bridge. The connecting sequences of the apple domains are in italics.
Relevant observations about the physiological control of PKa are its clearance from circulation by hepatocytes (Borges et al., 1981; Borges and Kouyoumdjian, 1992; Kouyoumdjian et al., 2005), inhibition by the plasma-circulating protein inhibitors discussed in section 7.1, and inactivation by proteolysis (Motta et al., 1992). In addition to the contact system of the intrinsic coagulation pathway, PKa plays a role in pathophysiological processes as a potent pro-urokinase (pro-uPA) activator (Ichinose et al., 1986), in the renin–angiotensin system as a prorenin activator (Hsueh et al., 1981; Almeida et al., 2000), as an activator of C3 convertase (DiScipio, 1982; Irmscher et al., 2018), and as factor B of the complement system (Hiemstra et al., 1985).
The genomic structure of PK (also KLKB1) (Beaubien et al., 1991; Yu et al., 2000) comprises a single gene in the human genome that maps to chromosome 4 (q34-q35), and hepatocytes are the local center of the PK gene transcription. However, it also occurs at different levels in non-hepatic tissues, but circulating PK in plasma is obtained essentially from the liver. It would be reasonable to assume that PK synthesized in extrahepatic tissues presents special functions in or close to their synthesis locations (Ciechanowicz et al., 1993; Hermann et al., 1999; Neth et al., 2001; Neth et al., 2005).
The evolution of the plasma kallikrein–kinin system (KKS) and Factor XI (FXI) described by Ponczek et al. (2008) and later in more detail by Ponczek et al. (2020) showed the KKS appeared in lobe-finned fish, the ancestors of all land vertebrates. Duplication of the gene for PK occurred during mammalian evolution, resulting in FXI changing independently of the KKS in placental mammals.
A significant observation related to the evolution of the KKS was the molecular studies of kalliklectin from channel catfish (Ictalurus punctatus), a fish-specific lectin containing eight apple domains, with structures similar to those of mammalian PK/FXI but without proteolytic activity (Tsutsui et al., 2021). Recently, the same group reported genomic sequences encoding a protein with apple and serine protease domains in a few cartilaginous and bony fish species by bioinformatic analysis and also purified, by mannose-affinity chromatography. Two ∼70 kDa proteins from the blood plasma of Ictalurus punctatus containing internal amino acid sequences were mapped onto possible PK/FXI-like sequences, which contains the typical cleavage site of mammalian PK and FXI, indicating protease activity (Tsutsui et al., 2023). Another new finding in this report is that catfish PK/FXI-like proteins have lectin activity.
Table 1 shows the PK, FXI, FXII, and HK appearances in the representative classes in the evolution, as reported by Ponczek et al. (2020), in which we added the recent contribution of Tsutsui et al. (2023) that can provide alternative evolutionary scenarios.
TABLE 1. Evolution of vertebrate coagulation proteins. The symbols in the columns indicate if a gene for the respective proteins was identified (+) or not identified (−) in genomic analyses. Data from Ponczek et al. (2020); Tsutsui et al. (2023). Table 1 was reprinted from BlooEsterl W, Bhoola KDd Adv. 4(24) Dec 22. Ponczek MB, Shamanaev A, LaPlace A, Dickeson SK, Srivastava P, Sun MF, Gruber A, Kastrup C, Emsley J, Gailani D. The evolution of factor XI and the kallikrein–kinin system. Pages 6135-6147, 2020. Doi:10.1182/bloodadvances.2020002456, with permission from Elsevier, License 5600360083462, Copyright Clearance Center’s RightsLink®. RightsLink®.
Relevant evolution occurred in the A4 domain of tetrapod PKs, including humans, with the additional Cys321–Cys326 disulfide bond (Figure 1) (McMullen et al., 1991), which is also present in the lungfish. The cysteine C326 in PK-A4 resulted in an extra disulfide bridge in PK-A4, and the substitution by G326 in FXI-A4 resulted in a free -SH group of C321 responsible for FXI dimerization (Cheng et al., 2003). Another relevant acquisition of FXI is proline at position 368 (P368), close to the cleavage site (R369-I370), for the activation to FXIa, which turned out to be susceptible to thrombin that requires proline at the P2 position (Schechter and Berger, 1967; Gallwitz et al., 2012), that amplifies the coagulation process and integrates the extrinsic and intrinsic pathways, as reviewed in Mohammed et al. (2018) and recently revisited in Barroeta et al. (2023).
Two extensive and comprehensive reviews of biochemistry and interactions of the blood contact activation system (Colman and Schmaier, 1986) and bioregulation of kinins (Bhoola et al., 1992) stressed the structural and functional characterization of PKa and its chains, along with its role related to coagulation, fibrinolysis, complement, and inflammation. PKa potentiates platelet aggregation induced by ADP, collagen, and adrenaline (Cassaro et al., 1987; Ottaiano et al., 2017).
The study of the KKS proteins in cell biology was a landmark between contact and KKSs despite their participation in both processes (Colman and Schmaier, 1997). The outer face of the neutrophil membrane binds the contact-phase proteins (HK, PK, FXI, and FXII) (Figure 2), as demonstrated by immunolocalization techniques (Henderson et al., 1994).
FIGURE 2. Setting of the contact-phase factors HK, PK, FXI, and FXII on neutrophils, with the reciprocal activation of PK—HK with bradykinin (BK) and Lys-BK released by tissue kallikrein 1(KLK1), adapted with permission from Henderson et al.,1994. Figure 2 was reprinted from Blood 84(2) Jul 15. Henderson LM, Figueroa CD, Muller–Esterl W, Bhoola KD. Assembly of contact-phase factors on the surface of the human neutrophil membrane, pages 474-82, 1994. Doi: 10.1182/blood.V84.2.474.474, with permission from Elsevier, License #5597371352787, Copyright Clearance Center’s RightsLink®.
The endothelial cells and platelet membranes assemble HKs; however, on endothelial cells and the extracellular matrix, PK binds mainly through assembled HK, and this interaction allows PKa formation independent of the FXII assembly on the cell membrane (Motta et al., 1998; Schmaier, 1998; Motta et al., 2001; Motta and Tersariol, 2017). Heat-shock protein 90 (Hsp-90) (Joseph et al., 2002) and prolylcarboxypeptidase (PRCP) (Shariat-Madar et al., 2002) are two other activators of PK besides FXIIa.
PKa plays relevant roles in inflammation, as reviewed with appropriate citations (Schmaier, 2016). Thrombosis and inflammation are present in many tissue injuries with multiple crossed-ways and interfaces between them (Wu, 2015), and with extensive participation of neutrophils (Mortaz et al., 2018), including all types (Rosales, 2018).
Muller–Ester’s and Schmaier’s groups identified the portions of HK that bind to the cell surface using monoclonal antibodies against different parts of HK (Kaufmann et al., 1993; Hasan et al., 1994) and the discontinuous binding site for HK on the PK heavy chain (Hock et al., 1990).
The HK interaction with the cell surface has been well characterized (Schmaier and McCrae, 2007). The HK presents six domains from its N-terminal portion (D1-D6); each domain plays a particular function that characterizes the whole molecule as a multifunctional protein. The domains D5(His-Gly-Lys rich), D4(BK), and D3 (cysteine protease inhibitor) allow the molecule to interact with the cell membrane. Nevertheless, after HK cleavage and bradykinin (BK) release, the intact molecule turns to BK-free-HK (HKa) (Lalmanach et al., 2010).
The organization of HK in six domains, as described previously, with clearly defined functions, has been well-established and reviewed in many publications. Nonetheless, determining its tridimensional (3D) structure is still challenging. The size of the protein, its multi-structural organization, and heavy glycosylation seem to be barriers to obtaining this goal. The structural changes that probably follow BK release or the acquired conformation upon an interaction with PK or ligands in the endothelial surface should be equally challenging to obtain. As recently explained by Ponczek, 2021), neither crystallography nor NMR methods provided published structures of HK. As suggested, a probably helpful approach, given the recent advances in the technique, would be the utilization of cryo-electron microscopy (cryoEM) not only for the determination of the 3D structure of HK itself but also for its many known physiologically relevant complexes with protein or non-protein molecules. For example, the interaction of HK with glycosaminoglycans and Zn2+ (Gozzo et al., 2011), which changes the kinetics of BK release by the PK from HK, would be attractive structural targets for cryoEM.
PK is an unusual plasma protease as it does not recruit its principal substrate, HK, upon activation. However, it instead circulates as a zymogen in a tightly bound but inactive PK–HK complex (Mandle et al., 1976), whose structure consists of HK binding to discontinuous sites of PK at its N-terminal apple domain in the rank order of binding affinity for kininogen of A2 > A4 = A1 > A3, A2 domain being essential for binding (Renné et al., 1999). It is important to mention that regions of the A3 domain have increased surface exposure in PKa compared to PK, indicating conformational changes upon activation (Li et al., 2019).
PK and FXI, through their heavy chain, circulate bound to domain 6 of HK (Vogel et al., 1990; Kunapuli et al., 1993). Either FXI or PK interacts via the apple domain A2 on the HK sequence (F582NPISDFPDT591); nevertheless, in this interaction with HK, PK has the second exosite in the apple domain A1, but FXI interacts with a unique pocket formed between A2 and A3 domains (Li et al., 2023).
The membrane-binding proteins of both HK and HKa (activated kininogen) on endothelial cells include the globular domains of the complement factor C1q receptor (gC1qR), urokinase plasminogen activator receptor (uPAR), and cytokeratin 1 (CK1), and the affinity levels among them are gC1qR > CK1 > soluble uPAR, indicating that gC1qR is dominant for binding. uPAR is an essential membrane-binding protein for differential binding and signaling between HK and HKa (Pixley et al., 2011).
Proteoglycans (PGs) are molecules expressed by all cells and found in all extracellular matrices (ECMs), consisting of a protein core onto which one or more of the negatively charged polysaccharide compounds, glycosaminoglycan (GAG) chains, are covalently attached (Karamanos et al., 2018). Heparan sulfate works as a putative receptor of either HK or PK, alone or in complex with each other, mediating the endocytosis and activation of HK and PK (Motta and Tersariol, 2017).
The two forms of plasma kininogens, HK and LK (low-molecular weight kininogen), are the products of a single gene that maps to 3q26-qter. The single kininogen gene of 11 exons of 27 kb produces a unique mRNA for HK and LK by alternative splicing. HK and LK share the coding region of the first nine exons, a part of exon 10 containing the BK sequence, and the first 12 amino acids after the carboxy-terminal sequence of BK. Exon 11 codes for a unique 4-kD light chain of LK, and the complete exon 10 contains the entire coding sequence for the unique 56-kD light chain of HK. So, the splice variant LK is 409 amino acids in length, and the principal difference is the loss of HK domain 6 (Colman and Schmaier, 1997).
The tissue and PKa, KLK1 and PKa, belong to the same family of serine proteases and show high sequence similarity in the region encoding the trypsin-like domain. Interestingly, the comparative genomics and phylogenetic analysis argue for a previous origin of PKa than KLK1. Kallikreins exert their major physiological roles by acting as proteases. Still, the expression of KLK protein isoforms, without the protease activity, may indicate that KLKs have other functions, at least in humans (Koumandou and Scorilas, 2013).
The kinins, initially BK and, by the action of carboxypeptidases, desArg9BK, are the end active peptides generated after the activation of PK to PKa. Human KLK1, unlike PKa, cleaves LK to generate Lys-BK (also kallidin), which is converted to BK by a second aminopeptidase cleavage (Pathak et al., 2013). The pathophysiological and beneficial physiological effects of kinins in various cardiovascular disorders such as hypertension, ischemic heart disease, left ventricular hypertrophy, ventricular remodeling, congestive heart failure, and diabetic conditions occur by the stimulation of BK receptors (B2 and B1) (Heitsch, 2003; Sharma, 2003; Marceau et al., 2020).
Recently, comprehensive reviews have extensively dealt with many aspects of BK and desArg9BK generation; its metabolism by endo, carboxy, and amino peptidases; and the multitude of actions attributed to both peptides in many cell models, tissues, and organs (Lalmanach et al., 2010; Campbell, 2013; Motta et al., 2018). The role of BK in angioedema as the B2BK receptor agonist has been unequivocally established (Hofman et al., 2016; Schmaeir, 2018; Rex et al., 2022; Gailani, 2023) through the increase in vascular permeability, but also desArg9BK, the B1BK receptor agonist, seems to be relevant as these receptors modulate neutrophil trafficking (Araújo et al., 2001). Neutrophil elastase release, among other effects (Stuardo et al., 2004), is one of the factors able to inactivate the C1-esterase inhibitor (C1-INH) or cleave FXII, accelerating FXII activation by PKa (de Maat et al., 2019; Ferrara et al., 2021).
A recent review highlights multiple mechanisms for the increased generation of kinins or their slowed inactivation, resulting in angioedema (Gailani, 2023). Of significant interest is the recently described mutation on the Glu311-plasminogen that turns plasmin, which is able to release BK from HK and LK at considerably higher rates than wild-type plasmin. Considering that LK concentrations are two to four times higher in plasma than HK, all this evidence indicates that LK, previously a not-so-relevant player in BK generation in angioedema, can be an important actor.
FXII, the zymogen of the protease FXIIa, plays a role in BK-dependent angioedema and thrombosis by activating prekallikrein and factor XI zymogen (FXI). These activation processes are highly magnified when FXII binds to a surface through EGF1, gaining an open conformation that facilitates FXII activation. These processes are recently described and discussed in detail (Shamanaev et al., 2022; Shamanaev et al., 2023).
More recently, the crystal structure for analyzing factor XII, HK, or both binding to gC1qR has been solved. FXII-HK-gC1qR forms a ternary complex assembled in the presence of Zn2+, stimulating reciprocal FXII-PK activation by gC1qR (Kaira et al., 2020). The authors also compared the asymmetric binding onto gC1qR to multiple client proteins that can be colocalized by asymmetric binding onto the Hsp-90 dimer (Flynn et al., 2015). The extracellular Hsp-90 has been described as a chaperokine involved in inflammatory processes (Taha et al., 2019) and as a PK activator on the cell surface, as mentioned previously (Joseph et al., 2002).
It is relevant to emphasize that the initial contributions of the plasma KKS related to coagulation, fibrinolysis, and inflammation processes remain under investigation over the years (Kolte and Shariat-Madar, 2016; Schmaier, 2016; Simão and Feener, 2017; Oehmcke-Hecht et al., 2022). More recently, it has been shown that PKa could be a significant physiological activator of FIX (Kearney et al., 2021).
Genetic manipulation of the KKS in mice has allowed recognition of the physiological role of the KKS in health and disease (Girolami et al., 2014). Mice deficient in the PK gene (Klkb1−/−) show an alternative mechanism for thrombosis protection, in addition to reduced contact activation, mediated by increased receptor Mas, prostacyclin, Sirt1, and KLF4 (Stavrou et al., 2015).
In the central nervous system inflammatory processes, PKa acts as a neuromodulator by engaging PAR-2 and BK-B2 receptors (Jaffa et al., 2021), and the complex biology of kinins also affects cancer progression (Deepak et al., 2022). In liver injury, PKa cleaves the transforming growth factor (TGF)-beta 1 (Li et al., 2018; Ahmed et al., 2021).
In addition to neutrophils, endothelial cells, and platelets, the contact system proteins bind to vascular smooth muscle cells (VSMCs) (Fernando et al., 2005). In the aortic VSMC, PKa activates the ERK1/2 mitogen-activated protein kinase cascade with the stimulation of ADAM 17 (a disintegrin-metalloprotease) via a PAR1/2 receptor-dependent mechanism, without the involvement of BK receptors. The BK-independent PKa action may regulate vascular responses in pathophysiologic states, such as diabetes mellitus (Abdallah et al., 2010).
The connection of fibrinolysis and the KKS at several levels supports understanding hereditary angioedema and other forms of vascular permeability mediated by BK (Tomita et al., 2012; Kaplan and Maas, 2017).
In the eye, the consequences of diabetes are microvascular abnormalities, the proliferation of retinal vessels, and increased retinal vascular permeability. The involvement of either kallikrein–kinin or renin–angiotensin systems has been reported in diabetic retinopathy, glaucoma, uveitis, diabetic macular edema, and age-related macular degeneration. In this way, the breakdown of the blood–retinal barrier function, which leads to increased retinal vascular permeability, results in significant alterations in the biochemical components of intraocular fluids and diffusion of blood-circulating factors into the interstitial retinal space and vitreous (Abdulaal et al., 2016; Igić, 2018; Othman et al., 2021).
In vitreous patients, carbonic anhydrase-I (CA-I), an intracellular enzyme, suggests retinal hemorrhage and erythrocyte lysis (Gao et al., 2007). CA-I induces alkalinization of vitreous, increasing the PKa activity and generating factor XIIa, revealing a new pathway for contact system activation. In addition, these authors showed the presence of extracellular CA-I inside either the blood–retinal or blood–brain barrier that can induce vasogenic edema. The blood–brain barrier breakdown seems to exist in patients with temporal lobe epilepsy (TLE) due to KKS activation (Simões et al., 2019).
The fibrin frames generated within the microvessels by contact systems capture microorganisms, reducing their spread through the whole organism, and facilitate activated leukocytes’ functions (Stark and Massberg, 2021). This process results from converging platelet-generated thrombogenesis with the activated neutrophils and monocytes. This intrinsic path results from the autoactivation of factor XII (FXIIa) by negatively charged substances, such as platelet-derived polyphosphates and DNA, from neutrophil extracellular traps that are the contact platforms. Once functional, FXIIa cleaves PK, generating PKa, releasing BK from HK, and initiating inflammation.
BK induces vasodilation and increases microvascular permeability by activating the endothelial BK-B2 receptors. A second receptor for BK is BK-B1, which presents high affinity for des-Arg9-BK produced by GPI-linked carboxypeptidase M by removing the C-terminal arginine from BK.
Scharfstein and his group extensively reviewed these processes, focusing mainly on Trypanosome cruzi as the infectious agent and including extensive studies on the role of parasite cysteine proteases (Scharfstein et al., 2012; Scharfstein et al., 2017; Scharfstein, 2018). A similar interplay between parasite cysteine proteases of Leishmania donovani and Leishmania chagasi also critically modulates inflammation and innate immunity in visceral leishmaniasis using the host kinin/B2 receptor activation pathway (Svensjö et al., 2006; 2014).
Plasmodium chabaudi and Plasmodium falciparum internalize and process plasma kininogen by falcipain-2 and falcipain-3, both cysteine proteases, releasing Lys-BK, BK, and des-Arg9-BK and resulting in hemodynamic alterations during acute malaria (Bagnaresi et al., 2012; Cotrin et al., 2013), as well as increasing the blood–brain barrier permeability (Silva et al., 2019).
The extensive inflammation process is a characteristic of the clinical evolution of periodontitis due to gingipain, the cysteine protease complex from the Gram-negative bacteria Porphyromonas gingivalis, which efficiently activates the KKS with direct kinin release and PK activation with subsequent BK release (Imamura et al., 1994; Monteiro et al., 2009).
Regarding virus infection, it is noteworthy that contact/KKS activation followed by BK-induced enhancement of DENV replication in the endothelium seems to underlie microvascular pathology in dengue (Coelho et al., 2021). The contact/intrinsic pathway contributes to the pathogenesis of the prothrombotic state in COVID-19 (Carvalho et al., 2021; Alfaro et al., 2022; Henderson et al., 2022). In completing this theme, we mentioned an opinion article about the clinical repercussions of Prof. Dr. Sérgio Ferreira on COVID-19 (Nicolau et al., 2020).
Zanelatto et al. (2022) recently showed that cathepsin B and PKa measured in the serum can be used to discriminate different stages of liver damage in HCV-infected patients as biomarkers to exclude hepatic fibrosis in the population.
C1-esterase inhibitor (C1-INH), α2-macroglobulin, antithrombin-III (van der Graaf et al., 1983), protein C inhibitor (PCI) (España et al., 1989), and alpha-2 plasmin inhibitor (Saito et al., 1979) are reported as PKa inhibitors. All belong to the serine proteinase inhibitor family (serpins).
Serpins have been extensively studied, and excellent recent reviews deal with their action mechanism, improvements in specificity, and potential use as drugs for replacement therapies (Maas and de Maat, 2021; Bouton et al., 2023). Briefly, serpins are proteins acting as molecular traps to proteases, particularly but not exclusively serine proteases, whose “beacon” is their carboxy-terminal regions, usually called the reactive center loop (RCL) that keeps the serpin in a high-energy state. Once cleaved, the RCL, but before a water molecule promotes the deacylation of the enzyme, changes to a lower energy state, where the remaining RCL remains linked to the enzyme in a new, lower-energy state. This structure is then buried in the five-strand beta-sheet (ß sheet A) present in serpins. It forms a sixth strand that makes water access difficult, blocks deacylation, and distorts the enzyme’s active center, rendering it inactive.
C1-INH plays a fundamental role in PKa inhibition. As estimated from biochemical experiments, approximately 42% of PKa inhibition is due to C1-INH activity, and 50% could be linked to α2-macroglobulin (Schapira et al., 1982) in normal plasma. The remaining 8% of inactivation could be attributed to other protease inhibitors in the plasma. The complete framework is much more complex when considering other enzymes and inhibitors in the in vivo steady-state physiological or pathological conditions. Proteolytic enzymes from the immune cells, for example, neutrophil elastase, can be released from the polymorphonuclear and mast cells and alter C1-INH activity (Ferrara et al., 2021; Kajdácsi et al., 2021). Anionic glycosaminoglycans, like heparin, heparan sulfate, chondroitin sulfate, and even polyphosphates, can interact either with the inhibitors or with the enzymes and dramatically change the inhibition or interaction constants (Grover and Mackman, 2022), adding more complexity to these interactions, as classically demonstrated for the antithrombin, PKa, HK, and heparin interaction (Olson et al., 1993).
PKa inhibitor (PKi) development followed a parallel trajectory, which is typical in discovering new drugs. Many clues indicated the role of PKa in hereditary angioedema (HAE) at the end of the fifties and beginning of the sixties, in the last century. The work by Landerman et al. (1962) is not only an elegant set of experiments and conclusions but also demonstrates the role of PKa and suggests that a “permeability factor (PF)" released from the plasma of patients diagnosed with HAE was probably kallidin. Logical treatment of these patients would be with replacement therapy of the inhibitor to the proteinase or by an inhibitor to the proposed polypeptide.
This comprehensive review (Schmaier, 2018) clearly shows that, in 1963, the puzzle of the etiology of HA was solved (Donaldson and Evans, 1963). Still, the emergence of the complement system and the role of C1-inhibitor (C1-INH) in it postponed the recognition of Landerman’s suggestions to the early seventies (Gigli et al., 1970).
PKa inhibitors were the first drugs to treat PKa disbalance present in HA and also in other conditions related to uncontrolled PKa activity, such as diabetic retinopathy, macular edema (Bhatwadekar et al., 2020), sepsis, surgical intercurrences observed in cardiopulmonary bypass (CPB) surgery and other conditions related to inflammatory diseases, thrombotic events, and vascular alterations (Bryant and Shariat-Madar, 2009).
Nonetheless, attention should be given to the proper production of BK, as explained by Regoli and Gobeil (2015) and recent reports relating the reduced PKa activity to diabetic nephropathy (Härma et al., 2020). As with many other proteinase inhibitors, at first, molecules found in nature, like Kunitz and Bowman–Birk inhibitors, were purified and characterized by chromatographic and structural tools that evolved during the ‘70s, the ‘80s, and later, from plants (Oliva and Sampaio, 2009). As far as 1975, aprotinin (Trasylol®), a serine proteinase inhibitor of animal origin, was assayed during extracorporeal circulation in an open-heart surgery (Nagaoka and Katori, 1975).
However, most of these inhibitors have low specificity, potentially induce immune reactions, and cannot be patented. Nonetheless, the scaffolds of those inhibitors were used in the future, in the 90s and beyond, to select more effective molecules. Other contributions made to understand the PKa specificities and potentially add to the design of new inhibitors were obtained from many groups. The development of sensible substrates, especially with quenched fluorescence properties, accelerated the usually slow determination of kinetic constants (Chagas et al., 1991) and could combine this technique with known interactions in natural inhibitors (Nunes et al., 2003).
The group of Yoshio Okada, at Kobe University, during the ‘90s and early 2003, searched for small peptidomimetic inhibitors based on the known specificities of PKa. PSI-527 was one of the PKa inhibitors that reached the clinical trial but was discontinued (Hashimoto et al., 2003). Meanwhile, high-throughput methods for ligand selection became available. Phage display of interaction regions with PKa from known ligands or scFv immunoglobulin regions, peptide libraries, and chemical libraries was used to search for more effective inhibitors with potential clinical use.
In 1996, a specific and selective PKa inhibitor was obtained, later named ecallantide, from a phage display selection/maturation system, an inhibitor based on a Kunitz-type scaffold, identified from the first Kunitz domain of human lipoprotein-associated coagulation inhibitor (LAC1-D1), also known as tissue factor pathway inhibitor-I or TFPI-I (https://www.creative-biolabs.com/DX-88-Library-Construction.html) (Ley et al., 1996).
Later, in 2003, a paper entitled “DX-88 and HAE: a developmental perspective,” where DX-88 was the ecallantide molecule, reported the positive results of clinical phase I studies. In 2008, a summary of the DX-88 development was published and indicated the size of the potential uses of C1-INH activity replacement with molecules other than C1-INH itself (Lehmann, 2008). At this point, it became clear that a race between pharma companies had started. In 2009, ecallantide was approved by the Food and Drug Administration (FDA). From 2008 to 2010, concentrates of C1-INH (Berinert licensed in Germany since 1985 and Cynrise) were FDA-approved. A recombinant C1-INH (Ruconest, Rhucin) was approved in Europe (2010) and the USA (2014).
Phage display libraries also generated monoclonal antibodies that specifically inhibit PKa, like lanadelumab (Kenniston et al., 2014), approved by the FDA in 2018.
As stated previously, C1-INH is a serpin. Historically, phage display libraries based on the serpin scaffold have successfully found specific inhibitors for proteases belonging to the KLK family (Cloutier et al., 2004; Felber et al., 2006). However, we do not find reports of libraries based on the serpin scaffold that could select specific PKa inhibitors. Likewise, no other phage display libraries were found based on Kunitz or Bowman–Birk inhibitors for PKa despite a structure published about at least one of these inhibitors being effective for PKa inhibition (Zhou et al., 2015). These libraries have not been tried or have not shown effective results yet.
At the same time, phage display and other methods have originated protein structures that are able to inhibit PKa. Synthetic chemists have performed much work to create successful new inhibitory molecules. Berotralstat (BCX7353) (Kotian et al., 2021), sebetralstat (Davie et al., 2022), ATN-249 (Attune Pharmaceuticals), KVD824 (KalVista Pharmaceuticals), THR-149 (Oxurion NV), RZ402 (Rezolute Bio), and VE-3539 and VE-4840 (Verseon Corp) (Xie et al., 2020) were included.
Cyclic or bicyclic peptide libraries expressed in phage display systems have been employed to select new high-affinity and specificity inhibitors of PKa, whose interactions are extended to sites far from the active site, opening the possibility to explore new intermolecular interactions with PKa (Teufel et al., 2018).
Many options are on the way, including orally active inhibitors and protocols aimed at acute, chronic, and prophylactic treatments linked to PKa inhibition disbalance. These new developments of synthetic molecules, their properties, and their uses have been the subject of recent comprehensive reviews (Busse and Christiansen, 2020; Xie et al., 2020; Caballero, 2021; Busse and Kaplan, 2022). Meanwhile, although none of the 3D PKa structures were available on the Protein Data Bank database until 2005, 14 structures have been deposited (some from the same complex at different resolutions), as summarized in Table 2, indicating the increased interest of pharma companies, a race in new developments on this class of inhibitors and a sure indication of an exciting market. Ecallantide in the USA had an estimated market of U$ 150 million in 2010 (Zuraw et al., 2010). A recent report of 2010 estimated the global plasma protease C1-inhibitor treatment market at US$ 3,289.5 million in 2020, projected to be US$ 10,603.4 million in 2027 (https://www.coherentmarketinsights.com/market-insight/plasma-protease-c1-inhibitor-treatment-market-4262).
A patent application (WO2022197761A1) claims that the compounds of the invention may be therapeutically beneficial for treating or preventing various ophthalmic, cardiovascular, or cerebrovascular thromboembolic conditions in patients suffering from unstable angina, acute coronary syndrome, refractory angina, myocardial infarction, transient ischemic attacks, atrial fibrillation, strokes such as thrombotic stroke or embolic stroke, venous thrombosis, coronary and cerebral arterial thrombosis, cerebral and pulmonary embolism, atherosclerosis, deep vein thrombosis, disseminated intravascular coagulation, reocclusion or restenosis of recanalized vessels, hereditary angioedema, uveitis, posterior uveitis, wet age-related macular degeneration, diabetic macular edema, diabetic retinopathy, and retinal vein occlusion. These perspectives are well beyond the original purpose of C1_INH studies for treating HAE. Hopefully, in the face of such a potential range of diseases or pathologies and the consequent expected market, many innovations will be implemented in the field.
All authors listed have made a substantial, direct, and intellectual contribution to the work and approved it for publication.
This work received financial support from Coordenação de Aperfeiçoamento de Pessoal de Nível Superior-CAPES-Brasil; Conselho Nacional de Desenvolvimento Cientifico e Tecnológico (CNPq – 313324/2022-0)-Brasil and Fundação de Amparo à Pesquisa do Estado de São Paulo-Brasil (FAPESP- 2020/07065-4).
The authors declare that the research was conducted in the absence of any commercial or financial relationships that could be construed as a potential conflict of interest.
All claims expressed in this article are solely those of the authors and do not necessarily represent those of their affiliated organizations, or those of the publisher, the editors, and the reviewers. Any product that may be evaluated in this article, or claim that may be made by its manufacturer, is not guaranteed or endorsed by the publisher.
Abdallah, R. T., Keum, J. S., El-Shewy, H. M., Lee, M. H., Wang, B., Gooz, M., et al. (2010). Plasma kallikrein promotes epidermal growth factor receptor transactivation and signaling in vascular smooth muscle through direct activation of protease-activated receptors. J. Biol. Chem. 285 (45), 35206–35215. doi:10.1074/jbc.M110.171769
Abdulaal, M., Haddad, N. M., Sun, J. K., and Silva, P. S. (2016). The role of plasma kallikrein-kinin pathway in the development of diabetic retinopathy: pathophysiology and therapeutic approaches. Semin. Ophthalmol. 31 (1-2), 19–24. doi:10.3109/08820538.2015.1114829
Ahmed, I. A., Jaffa, M. A., Moussa, M., Hatem, D., El-Achkar, G. A., Al Sayegh, R., et al. (2021). Plasma kallikrein as a modulator of liver injury/remodeling. Front. Pharmacol. 12, 715111. doi:10.3389/fphar.2021.715111
Alfaro, E., Díaz-García, E., García-Tovar, S., Zamarrón, E., Mangas, A., Galera, R., et al. (2022). Impaired kallikrein-kinin system in COVID-19 patients' severity. Front. Immunol. 13, 909342. doi:10.3389/fimmu.2022.909342
Almeida, P. C., Chagas, J. R., Cezari, M. H., Juliano, M. A., and Juliano, L. (2000). Hydrolysis by plasma kallikrein of fluorogenic peptides derived from prorenin processing site. Biochim. Biophys. Acta 1479 (1-2), 83–90. doi:10.1016/s0167-4838(00)00049-2
Araújo, R. C., Kettritz, R., Fichtner, I., Paiva, A. C., Pesquero, J. B., and Bader, M. (2001). Alteredneutrophil homeostasis in kinin B1 receptor-deficient mice. Biol. Chem. 382 (1), 91–95. doi:10.1515/BC.2001.014
Bagnaresi, P., Barros, N. M., Assis, D. M., Melo, P. M., Fonseca, R. G., Juliano, M. A., et al. (2012). Intracellular proteolysis of kininogen by malaria parasites promotes release of active kinins. Malar. J. 11, 156. doi:10.1186/1475-2875-11-156
Barroeta, A. B., Albanese, P., Marquart, A., Meijers, J. C. M., and Scheltema, R. A. (2023). Thrombin activation of the factor XI dimer is a multi-staged process for each subunit. bioRxiv preprint. doi:10.1101/2023.02.11.528103
Beaubien, G., Rosinski-Chupin, I., Mattei, M. G., Mbikay, M., Chrétien, M., and Seidah, N. G. (1991). Gene structure and chromosomal localization of plasma kallikrein. Biochemistry 30 (6), 1628–1635. doi:10.1021/bi00220a027
Bhatwadekar, A. D., Kansara, V. S., and Ciulla, T. A. (2020). Investigational plasma kallikrein inhibitors for the treatment of diabetic macular edema: an expert assessment. Expert Opin. Investig. Drugs 29 (3), 237–244. doi:10.1080/13543784.2020.1723078
Bhoola, K. D., Figueroa, C. D., and Worthy, K. (1992). Bioregulation of kinins: kallikreins, kininogens, and kininases. Pharmacol. Rev. 44 (1), 1–80.
Borges, D. R., and Kouyoumdjian, M. (1992). The recognition site for hepatic clearance of plasma kallikrein is on its heavy chain and is latent on prokallikrein. J. Hepatol. 16 (1-2), 115–121. doi:10.1016/s0168-8278(05)80103-5
Borges, D. R., Webster, M. E., Guimarães, J. A., and Prado, J. L. (1981). Synthesis of prekallikrein and metabolism of plasma kallikrein by perfused rat liver. Biochem. Pharmacol. 30 (10), 1065–1069. doi:10.1016/0006-2952(81)90443-3
Bouton, M. C., Geiger, M., Sheffield, W. P., Irving, J. A., Lomas, D. A., Song, S., et al. (2023). The under-appreciated world of the serpin family of serine proteinase inhibitors. EMBO Mol. Med. 15 (6), e17144. doi:10.15252/emmm.202217144
Bryant, J. W., and Shariat-Madar, Z. (2009). Human plasma kallikrein-kinin system: physiological and biochemical parameters. Cardiovasc Hematol. Agents Med. Chem. 7 (3), 234–250. doi:10.2174/187152509789105444
Busse, P., and Kaplan, A. (2022). Specific targeting of plasma kallikrein for treatment of hereditary angioedema: a revolutionary decade. J. Allergy Clin. Immunol. Pract. 10 (3), 716–722. doi:10.1016/j.jaip.2021.11.011
Busse, P. J., and Christiansen, S. C. (2020). Hereditary angioedema. N. Engl. J. Med. 382 (12), 1136–1148. doi:10.1056/NEJMra1808012
Caballero, T. (2021). Treatment of hereditary angioedema. J. Investig. Allergol. Clin. Immunol. 31 (1), 1–16. doi:10.18176/jiaci.0653
Campbell, D. J. (2013). “Bradykinin peptides,” in Handbook of biologically active peptides. Editor A. J. Kastin, 1386–1393. Academic Press-Elservier. doi:10.1016/B978-0-12-385095-9.00188-3
Carvalho, P. R., Sirois, P., and Fernandes, P. D. (2021). The role of kallikrein-kinin and renin-angiotensin systems in COVID- 19 infection. Peptides 135, 170428. doi:10.1016/j.peptides.2020.170428
Cassaro, C. M., Sampaio, M. U., Maeda, N. Y., Chamone, D. F., and Sampaio, C. A. (1987). Human plasma kallikrein: effect on the induced platelet aggregation. Thromb. Res. 48 (1), 81–87. doi:10.1016/0049-3848(87)90348-3
Chagas, J. R., Juliano, L., and Prado, E. S. (1991). Intramolecularly quenched fluorogenic tetrapeptide substrates for tissue and plasma kallikreins. Anal. Biochem. 192 (2), 419–425. doi:10.1016/0003-2697(91)90558-b
Cheng, Q., Sun, M. F., Kravtsov, D. V., Aktimur, A., and Gailani, D. (2003). Factor XI apple domains and protein dimerization. J. Thromb. Haemost. 1 (11), 2340–2347. doi:10.1046/j.1538-7836.2003.00418.x
Ciechanowicz, A., Bader, M., Wagner, J., and Ganten, D. (1993). Extrahepatic transcription of plasma prekallikrein gene in human and rat tissues. Biochem. Biophys. Res. Commun. 197 (3), 1370–1376. doi:10.1006/bbrc.1993.2628
Cloutier, S. M., Kundig, C., Felber, L. M., Fattah, O. M., Chagas, J. R., Gygi, C. M., et al. (2004). Development of recombinant inhibitors specific to human kallikrein 2 using phage-display selected substrates. Eur. J. Biochem. 271 (3), 607–613. doi:10.1111/j.1432-1033.2003.03963.x
Coelho, S. V. A., Rust, N. M., Vellasco, L., Papa, M. P., Pereira, A. S. G., Silva Palazzo, M. F. D., et al. (2021). Contact system activation in plasma from dengue patients might harness endothelial virus replication through the signaling of bradykinin receptors. Pharm. (Basel). 14 (1), 56. doi:10.3390/ph14010056
Colman, R. W., Mattler, L., and Sherry, S. (1969). Studies on the prekallikrein (kallikreinogen)--kallikrein enzyme system of human plasma. I. Isolation and purification of plasma kallikreins. J. Clin. Invest. 48 (1), 11–22. doi:10.1172/JCI105959
Colman, R. W., and Schmaier, A. H. (1997). Contact system: A vascular biology modulator with anticoagulant, profibrinolytic, antiadhesive, and proinflammatory attributes. Blood 90 (10), 3819–3843. doi:10.1182/blood.v90.10.3819
Colman, R. W., and Schmaier, A. H. (1986). The contact activation system: biochemistry and interactions of these surface mediated defense reactions. Crit. Rev. Oncol. Hematol. 5 (1), 57–85. Select. doi:10.1016/s1040-8428(86)80053-1
Cotrin, S. S., Gouvêa, I. E., Melo, P. M., Bagnaresi, P., Assis, D. M., Araújo, M. S., et al. (2013). Substrate specificity studies of the cysteine peptidases falcipain-2 and falcipain-3 from Plasmodium falciparum and demonstration of their kininogenase activity. Mol. Biochem. Parasitol. 187 (2), 111–116. doi:10.1016/j.molbiopara.2013.01.002
Davie, R. L., Edwards, H. J., Evans, D. M., Hodgson, S. T., Stocks, M. J., Smith, A. J., et al. (2022). Sebetralstat (KVD900): A potent and selective small molecule plasma kallikrein inhibitor featuring a novel P1 group as a potential oral on-demand treatment for hereditary angioedema. J. Med. Chem. 65 (20), 13629–13644. doi:10.1021/acs.jmedchem.2c00921
de Maat, S., Clark, C. C., Boertien, M., Parr, N., Sanrattana, W., Hofman, Z. L. M., et al. (2019). Factor XII truncation accelerates activation in solution. Thromb. Haemost. 17 (1), 183–194. doi:10.1111/jth.14325
Deepak, K., Roy, P. K., Kola, P., Mukherjee, B., and Mandal, M. (2022). An overview of kinin mediated events in cancer progression and therapeutic applications. Biochim. Biophys. Acta Rev. Cancer 1877 (6), 188807. doi:10.1016/j.bbcan.2022.188807
DiScipio, R. G. (1982). The activation of the alternative pathway C3 convertase by human plasma kallikrein. Immunology 45 (3), 587–595.
Donaldson, V. H., and Evans, R. R. (1963). A biochemical abnormality in hereditary angioneurotic edema: absence of serum inhibitor of C1-esterase. Am. J. Med. 35, 37–44. doi:10.1016/0002-9343(63)90162-1
España, F., Berrettini, M., and Griffin, J. H. (1989). Purification and characterization of plasma protein C inhibitor. Thromb. Res. 55 (3), 369–384. doi:10.1016/0049-3848(89)90069-8
Felber, L. M., Kundig, C., Borgoño, C. A., Chagas, J. R., Tasinato, A., Jichlinski, P., et al. (2006). Mutant recombinant serpins as highly specific inhibitors of human kallikrein 14. FEBS J. 273 (11), 2505–2514. doi:10.1111/j.1742-4658.2006.05257.x
Fernando, A. N., Fernando, L. P., Fukuda, Y., and Kaplan, A. P. (2005). Assembly, activation, and signaling by kinin-forming proteins on human vascular smooth muscle cells. Am. J. Physiol. Heart Circ. Physiol. 289 (1), H251–H257. doi:10.1152/ajpheart.00206.2004
Ferrara, A. L., Cristinziano, L., Petraroli, A., Bova, M., Gigliotti, M. C., Marcella, S., et al. (2021). Roles of immune cells in hereditary angioedema. Clin. Rev. Allergy Immunol. 60 (3), 369–382. doi:10.1007/s12016-021-08842-9
Fisher, C. A., Schmaier, A. H., Addonizio, V. P., and Colman, R. W. (1982). Assay of prekallikrein in human plasma: comparison of amidolytic, esterolytic, coagulation, and immunochemical assays. Blood 59 (5), 963–970. doi:10.1182/blood.v59.5.963.963
Flynn, J. M., Mishra, P., and Bolon, D. N. A. (2015). Mechanistic asymmetry in Hsp90 dimers. J. Mol. Biol. 427 (18), 2904–2911. doi:10.1016/j.jmb.2015.03.017
Gailani, D. (2023). Hereditary angioedema and thrombosis. Blood 141 (19), 2295–2297. doi:10.1182/blood.2023019861
Gallwitz, M., Enoksson, M., Thorpe, M., and Hellman, L. (2012). The extended cleavage specificity of human thrombin. PLoS One 7 (2), e31756. doi:10.1371/journal.pone.0031756
Gao, B. B., Clermont, A., Rook, S., Fonda, S. J., Srinivasan, V. J., Wojtkowski, M., et al. (2007). Extracellular carbonic anhydrase mediates hemorrhagic retinal and cerebral vascular permeability through prekallikrein activation. Nat. Med. 13 (2), 181–188. doi:10.1038/nm1534
Gigli, I., Mason, J. W., Colman, R. W., and Austen, K. F. (1970). Interaction of plasma kallikrein with the C1 inhibitor. J. Immunol. 104 (3), 574–581. doi:10.4049/jimmunol.104.3.574
Girolami, J. P., Blaes, N., Bouby, N., and Alhenc-Gelas, F. (2014). Genetic manipulation and genetic variation of the kallikrein-kinin system: impact on cardiovascular and renal diseases. Prog. Drug Res. 69, 145–196. doi:10.1007/978-3-319-06683-7_6
Gozzo, A. J., Motta, G., Cruz-Silva, I., Nunes, V. A., Barros, N. M., Carmona, A. K., et al. (2011). Heparin affects the interaction of kininogen on endothelial cells. Biochimie 93 (10), 1839–1845. Epub 2011 Jul 26. doi:10.1016/j.biochi.2011.07.003
Grover, S. P., and Mackman, N. (2022). Anticoagulant SERPINs: endogenous regulators of hemostasis and thrombosis. Front. Cardiovasc Med. 9, 878199. doi:10.3389/fcvm.2022.878199
Härma, M. A., Dahlström, E. H., Sandholm, N., Forsblom, C., Groop, P. H., Lehto, M., et al. (2020). Decreased plasma kallikrein activity is associated with reduced kidney function in individuals with type 1 diabetes. Diabetologia 63 (7), 1349–1354. Epub. doi:10.1007/s00125-020-05144-1
Hasan, A. A., Cines, D. B., Zhang, J., and Schmaier, A. H. (1994). The carboxyl terminus of bradykinin and amino terminus of the light chain of kininogens comprise an endothelial cell binding domain. J. Biol. Chem. 269 (50), 31822–31830. doi:10.1016/s0021-9258(18)31769-1
Hashimoto, M., Oiwa, K., Matsuo, O., Ueshima, S., Okada, K., Okada, Y., et al. (2003). Suppression of argatroban-induced endogenous thrombolysis by PKSI-527, and antibodies to TPA and UPA, evaluated in a rat arterial thrombolysis model. Thromb. Haemost. 89 (5), 820–825. doi:10.1055/s-0037-1613467
Hathaway, W. E., and Alsever, J. (1970). The relation of 'Fletcher Factor' to factors XI and XII. Br. J. Haematol. 18 (2), 161–169. doi:10.1111/j.1365-2141.1970.tb01431.x
Hathaway, W. E., Belhasen, L. P., and Hathaway, H. S. (1965). Evidence for a new plasma thromboplastin factor. I. Case report, coagulation studies and physicochemical properties. Blood 26 (5), 521–532. select. doi:10.1182/blood.v26.5.521.521
Heitsch, H. (2003). The therapeutic potential of bradykinin B2 receptor agonists in the treatment of cardiovascular disease. Expert Opin. Investig. Drugs 12 (5), 759–770. doi:10.1517/13543784.12.5.759
Henderson, L. M., Figueroa, C. D., Muller-Esterl, W., and Bhoola, K. D. (1994). Assembly of contact-phase factors on the surface of the human neutrophil membrane. Blood 84 (2), 474–482. doi:10.1182/blood.V84.2.474.474
Henderson, M. W., Lima, F., Moraes, C. R. P., Ilich, A., Huber, S. C., Barbosa, M. S., et al. (2022). Contact and intrinsic coagulation pathways are activated and associated with adverse clinical outcomes in COVID-19. Blood Adv. 6 (11), 3367–3377. doi:10.1182/bloodadvances.2021006620
Hermann, A., Arnhold, M., Kresse, H., Neth, P., and Fink, E. (1999). Expression of components of the kallikrein-kinin system in human cell lines. Immunopharmacology 45 (1-3), 135–139. doi:10.1016/s0162-3109(99)00066-1
Hiemstra, P. S., Daha, M. R., and Bouma, B. N. (1985). Activation of factor B of the complement system by kallikrein and its light chain. Thromb. Res. 38 (5), 491–503. doi:10.1016/0049-3848(85)90182-3
Hock, J., Vogel, R., Linke, R. P., and Muller-Esterl, W. (1990). High molecular weight kininogen-binding site of prekallikrein probed by monoclonal antibodies. J. Biol. Chem. 265 (20), 12005–12011. doi:10.1016/s0021-9258(19)38500-x
Hofman, Z., de Maat, S., Hack, C. E., and Maas, C. (2016). Bradykinin: inflammatory product of the coagulation system. Clin. Rev. Allergy Immunol. 51 (2), 152–161. doi:10.1007/s12016-016-8540-0
Hsueh, W. A., Carlson, E. J., and Israel-Hagman, M. (1981). Mechanism of acid-activation of renin: role of kallikrein in renin activation. Hypertension 3 (3), I22–I29. doi:10.1161/01.hyp.3.3_pt_2.i22
Ichinose, A., Fujikawa, K., and Suyama, T. (1986). The activation of pro-urokinase by plasma kallikrein and its inactivation by thrombin. J. Biol. Chem. 261 (8), 3486–3489. doi:10.1016/s0021-9258(17)35674-0
Igić, R. (2018). Four decades of ocular renin-angiotensin and kallikrein-kinin systems (1977-2017). Exp. Eye Res. 166, 74–83. Epub 2017 May 23. doi:10.1016/j.exer.2017.05.007
Imamura, T., Pike, R. N., Potempa, J., and Travis, J. (1994). Pathogenesis of periodontitis: A major arginine-specific cysteine proteinase from Porphyromonas gingivalis induces vascular permeability enhancement through activation of the kallikrein/kinin pathway. J. Clin. Invest. 94 (1), 361–367. doi:10.1172/JCI117330
Irmscher, S., Döring, N., Halder, L. D., Jo, E. A. H., Kopka, I., Dunker, C., et al. (2018). Kallikrein cleaves C3 and activates complement. Innate Immun. 10 (2), 94–105. doi:10.1159/000484257
Jaffa, A. A., Jaffa, M. A., Moussa, M., Ahmed, I. A., Karam, M., Aldeen, K. S., et al. (2021). Modulation of neuro-inflammatory signals in microglia by plasma prekallikrein and neuronal cell debris. Front. Pharmacol. 12, 743059. doi:10.3389/fphar.2021.743059
Joseph, K., Tholanikunnel, B. G., and Kaplan, A. P. (2002). Activation of the bradykinin-forming cascade on endothelial cells: A role for heat shock protein 90. Int. Immunopharmacol. 2 (13-14), 1851–1859. doi:10.1016/s1567-5769(02)00186-8
Kaira, B. G., Slater, A., McCrae, K. R., Dreveny, I., Sumya, U., Mutch, N. J., et al. (2020). Factor XII and kininogen asymmetric assembly with gC1qR/C1QBP/P32 is governed by allostery. Blood 136 (14), 1685–1697. doi:10.1182/blood.2020004818
Kajdácsi, E., Veszeli, N., Mező, B., Jandrasics, Z., Kőhalmi, K. V., Ferrara, A. L., et al. (2021). Pathways of neutrophil granulocyte activation in hereditary angioedema with C1 inhibitor deficiency. Clin. Rev. Allergy Immunol. 60 (3), 383–395. doi:10.1007/s12016-021-08847-4
Kaplan, A. P., and Maas, C. (2017). The search for biomarkers in hereditary angioedema. Front. Med. (Lausanne) 4, 206. doi:10.3389/fmed.2017.00206
Karamanos, N. K., Piperigkou, Z., Theocharis, A. D., Watanabe, H., Franchi, M., Baud, S., et al. (2018). Proteoglycan chemical diversity drives multifunctional cell regulation and therapeutics. Chem. Rev. 118 (18), 9152–9232. doi:10.1021/acs.chemrev.8b00354
Kaufmann, J., Haasemann, M., Modrow, S., and Müller-Esterl, W. (1993). Structural dissection of the multidomain kininogens. Fine mapping of the target epitopes of antibodies interfering with their functional properties. J. Biol. Chem. 268 (12), 9079–9091. doi:10.1016/s0021-9258(18)52980-x
Kearney, K. J., Butler, J., Posada, O. M., Wilson, C., Heal, S., Ali, M., et al. (2021). Kallikrein directly interacts with and activates Factor IX, resulting in thrombin generation and fibrin formation independent of Factor XI. Proc. Natl. Acad. Sci. U. S. A. 118 (3), e2014810118. doi:10.1073/pnas.2014810118
Kenniston, J. A., Faucette, R. R., Martik, D., Comeau, S. R., Lindberg, A. P., Kopacz, K. J., et al. (2014). Inhibition of plasma kallikrein by a highly specific active site blocking antibody. J. Biol. Chem. 289 (34), 23596–23608. doi:10.1074/jbc.M114.569061
Kolte, D., and Shariat-Madar, Z. (2016). Plasma kallikrein inhibitors in cardiovascular disease: an innovative therapeutic approach. Cardiol. Rev. 24 (3), 99–109. doi:10.1097/CRD.0000000000000069
Kotian, P. L., Wu, M., Vadlakonda, S., Chintareddy, V., Lu, P., Juarez, L., et al. (2021). Berotralstat (BCX7353): structure-guided design of a potent, selective, and oral plasma kallikrein inhibitor to prevent attacks of hereditary angioedema (HAE). J. Med. Chem. 64 (17), 12453–12468. doi:10.1021/acs.jmedchem.1c00511
Koumandou, V. L., and Scorilas, A. (2013). Evolution of the plasma and tissue kallikreins, and their alternative splicing isoforms. PLoS ONE 8 (7), e68074. doi:10.1371/journal.pone.0068074
Kouyoumdjian, M., Nagaoka, M. R., and Borges, D. R. (2005). Kallikrein-kinin system in hepatic experimental models. Peptides 26 (8), 1301–1307. Epub 2005 Apr 26. doi:10.1016/j.peptides.2005.03.024
Kunapuli, S. P., DeLa Cadena, R. A., and Colman, R. W. J. (1993). Deletion mutagenesis of high molecular weight kininogen light chain. Identification of two anionic surface binding subdomains. Biol. Chem. 268 (4), 2486–2492. doi:10.1016/s0021-9258(18)53802-3
Lalmanach, G., Naudin, C., Lecaille, F., and Fritz, H. (2010). Kininogens: more than cysteine protease inhibitors and kinin precursors. Biochimie 92 (11), 1568–1579. doi:10.1016/j.biochi.2010.03.011
Landerman, N. S., Webster, M. E., Becker, E. L., and Ratcliffe, H. E. (1962). Hereditary angioneurotic edema. II. Deficiency of inhibitor for serum globulin permeability factor and/or plasma kallikrein. J. Allergy 33, 330–341. doi:10.1016/0021-8707(62)90032-1
Lehmann, A. (2008). Ecallantide (DX-88), a plasma kallikrein inhibitor for the treatment of hereditary angioedema and the prevention of blood loss in on-pump cardiothoracic surgery. Expert Opin. Biol. Ther. 8 (8), 1187–1199. doi:10.1517/14712598.8.8.1187
Ley, A. C., Markland, W., and Ladner, R. C. (1996). Obtaining a family of high-affinity, high-specificity protein inhibitors of plasmin and plasma kallikrein. Mol. Divers 2 (1-2), 119–124. doi:10.1007/BF01718709
Li, C., Barroeta, A. B., Wong, S. S., Kim, H. J., Pathak, M., Dreveny, I., et al. (2023). Structures of factor XI and prekallikrein bound to domain 6 of high-molecular weight kininogen reveal alternate domain 6 conformations and exosites. Thromb. Haemost. 21 (23), 00326–334. doi:10.1016/j.jtha.2023.03.042
Li, C., Voos, K. M., Pathak, M., Hall, G., McCrae, K. R., Dreveny, I., et al. (2019). Plasma kallikrein structure reveals apple domain disc rotated conformation compared to factor XI. J. Thromb. Haemost. 17 (5), 759–770. doi:10.1111/jth.14418
Li, M., Qin, X. Y., Furutani, Y., Inoue, I., Sekihara, S., Kagechika, H., et al. (2018). Prevention of acute liver injury by suppressing plasma kallikrein-dependent activation of latent TGF-β. Biochem. Biophys. Res. Commun. 504 (4), 857–864. Epub. doi:10.1016/j.bbrc.2018.09.026
Maas, C., and de Maat, S. (2021). Therapeutic SERPINs: improving on nature. Front. Cardiovasc Med. 8, 648349. doi:10.3389/fcvm.2021.648349
Mandle, R. J., Colman, R. W., and Kaplan, A. P. (1976). Identification of prekallikrein and high-molecular-weight kininogen as a complex in human plasma. Proc. Natl. Acad. Sci. U. S. A. 73 (11), 4179–4183. doi:10.1073/pnas.73.11.4179
Marceau, F., Bachelard, H., Bouthillier, J., Fortin, J. P., Morissette, G., Bawolak, M. T., et al. (2020). Bradykinin receptors: agonists, antagonists, expression, signaling, and adaptation to sustained stimulation. Int. Immunopharmacol. 82, 106305. doi:10.1016/j.intimp.2020.106305
McConnell, D. J., and Mason, B. (1970). The isolation of human plasma prekallikrein. Br. J. Pharmacol. 38 (3), 490–502. doi:10.1111/j.1476-5381.1970.tb10591.x
McMullen, B. A., Fujikawa, K., and Davie, E. W. (1991). Location of the disulfide bonds in human coagulation factor XI: the presence of tandem apple domains. Biochemistry 30 (8), 2056–2060. doi:10.1021/bi00222a008
Mohammed, B. M., Matafonov, A., Ivanov, I., Sun, M-F., Cheng, Q., Dickeson, S-K., et al. (2018). An update on factor XI structure and function. Thromb. Res. 161, 94–105. doi:10.1016/j.thromres.2017.10.008
Monteiro, A. C., Scovino, A., Raposo, S., Gaze, V. M., Cruz, C., Svensjö, E., et al. (2009). Kinin danger signals proteolytically released by gingipain induce Fimbriae-specific IFN-gamma- and IL-17-producing T cells in mice infected intramucosally with Porphyromonas gingivalis. J. Immunol. 183 (6), 3700–3711. doi:10.4049/jimmunol.0900895
Moriya, H., Yamazaki, K., and Fukushima, H. (1965). Biochemical studies on kallikreins and their related substances. 3. Preliminary experiment of studies on human plasma kallikrein. J. Biochem. 58 (4), 315–319. doi:10.1093/oxfordjournals.jbchem.a128207
Mortaz, E., Alipoor, S. D., Adcock, I. M., Mumby, S., and Koenderman, L. (2018). Update on neutrophil function in severe inflammation. Front. Immunol. 9, 2171. doi:10.3389/fimmu.2018.02171
Motta, G., Fink, E., Sampaio, M. U., and Sampaio, C. A. (1992). Human plasma kallikrein processing: proteolysis as an alternative control. Actions Suppl. 38 (2), 265–277.
Motta, G., Rojkjaer, R., Hasan, A. A., Cines, D. B., and Schmaier, A. H. (1998). High molecular weight kininogen regulates prekallikrein assembly and activation on endothelial cells: A novel mechanism for contact activation. Blood 91 (2), 516–528. doi:10.1182/blood.v91.2.516.516_516_528
Motta, G., Shariat-Madar, Z., Mahdi, F., Sampaio, C. A., and Schmaier, A. H. (2001). Assembly of high molecular weight kininogen and activation of prekallikrein on cell matrix. Thromb. Haemost. 86 (3), 840–847. doi:10.1055/s-0037-1616141
Motta, G., Tersariol, I. L. S., Calo, G., Gobeil, F., and Regoli, D. (2018). “Kallikrein–kinin system,” in eLS (Chichester: John Wiley and Sons, Ltd). doi:10.1002/9780470015902.a0000905.pub2
Motta, G., and Tersariol, I. L. S. (2017). Modulation of the plasma kallikrein-kinin system proteins performed by heparan sulfate proteoglycans. Front. Physiol. 8, 481. doi:10.3389/fphys.2017.00481
Nagaoka, H., and Katori, M. (1975). Inhibition of kinin formation by a kallikrein inhibitor during extracorporeal circulation in open-heart surgery. Circulation 52 (2), 325–332. doi:10.1161/01.cir.52.2.325
Nagase, H., and Barrett, A. J. (1981). Human plasma kallikrein. A rapid purification method with high yield. Biochem. J. 193 (1), 187–192. doi:10.1042/bj1930187
Nakayasu, T., and Nagasawa, S. (1979). Studies on human kininogens. I. Isolation, characterization, and cleavage by plasma kallikrein of high molecular weight (HMW)-kininogen. J. Biochem. 85 (1), 249–258. doi:10.1093/oxfordjournals.jbchem.a132318
Neth, P., Arnhold, M., Nitschko, H., and Fink, E. (2001). The mRNAs of prekallikrein, factors XI and XII, and kininogen, components of the contact phase cascade are differentially expressed in multiple non-hepatic human tissues. Thromb. Haemost. 85 (6), 1043–1047. doi:10.1055/s-0037-1615961
Neth, P., Arnhold, M., Sidarovich, V., Bhoola, K. D., and Fink, E. (2005). Expression of the plasma prekallikrein gene: utilization of multiple transcription start sites and alternative promoter regions. Biol. Chem. 386 (2), 101–109. doi:10.1515/BC.2005.013
Nicolau, L. A. D., Magalhães, P. J. C., and Vale, M. L. (2020). What would sérgio Ferreira say to your physician in this war against COVID-19: how about kallikrein/kinin system? Med. Hypotheses 143, 109886. doi:10.1016/j.mehy.2020.109886
Nunes, V. A., Gozzo, A. J., Sampaio, M. U., Juliano, M. A., Sampaio, C. A., and Araujo, M. S. (2003). Mapping of human plasma kallikrein active site by design of peptides based on modifications of a Kazal-type inhibitor reactive site. J. Protein Chem. 22 (6), 533–541. doi:10.1023/b:jopc.0000005503.20628.4e
Oehmcke-Hecht, S., Berlin, P., Muller-Hilke, B., Kreikemeyer, B., Vasudevan, P., Henze, L., et al. (2022). The versatile role of the contact system in cardiovascular disease, inflammation, sepsis and cancer. Biomed. Pharmacother. 145, 112429. doi:10.1016/j.biopha.2021.112429
Oliva, M. L., and Sampaio, M. U. (2009). Action of plant proteinase inhibitors on enzymes of physiopathological importance. Acad Bras Cienc 81 (3), 615–621. doi:10.1590/s0001-37652009000300023
Olson, S. T., Sheffer, R., and Francis, A. M. (1993). High molecular weight kininogen potentiates the heparin-accelerated inhibition of plasma kallikrein by antithrombin: role for antithrombin in the regulation of kallikrein. Biochemistry 32 (45), 12136–12147. doi:10.1021/bi00096a026
Othman, R., Cagnone, G., Joyal, J. S., Vaucher, E., and Couture, R. (2021). Kinins and their receptors as potential therapeutic targets in retinal pathologies. Cells 10 (8), 1913. doi:10.3390/cells10081913
Ottaiano, T. F., Andrade, S. S., de Oliveira, C., Silva, M. C. C., Buri, M. V., Juliano, M. A., et al. (2017). Plasma kallikrein enhances platelet aggregation response by subthreshold doses of ADP. Biochimie 135, 72–81. doi:10.1016/j.biochi.2017.01.010
Pathak, M., Wong, S. S., Dreveny, I., and Emsley, J. (2013). Structure of plasma and tissue kallikreins. Thromb. Haemost. 110 (3), 423–433. doi:10.1160/TH12-11-0840
Pixley, R. A., Espinola, R. G., Ghebrehiwet, B., Joseph, K., Kao, A., Bdeir, K., et al. (2011). Interaction of high-molecular-weight kininogen with endothelial cell binding proteins suPAR, gC1qR and cytokeratin 1 determined by surface plasmon resonance (BiaCore). Thromb. Haemost. 105 (6), 1053–1059. doi:10.1160/TH10-09-0591
Ponczek, M. B., Gailani, D., and Doolittle, R. F. (2008). Evolution of the contact phase of vertebrate blood coagulation. J. Thromb. Haemost. 6 (11), 1876–1883. doi:10.1111/j.1538-7836.2008.03143.x
Ponczek, M. B. (2021). High molecular weight kininogen: A review of the structural literature. Int. J. Mol. Sci. 22 (24), 13370. doi:10.3390/ijms222413370
Ponczek, M. B., Shamanaev, A., LaPlace, A., Dickeson, S. K., Srivastava, P., Sun, M. F., et al. (2020). The evolution of factor XI and the kallikrein-kinin system. Blood Adv. 4 (24), 6135–6147. doi:10.1182/bloodadvances.2020002456
Regoli, D., and Gobeil, F. (2015). Critical insights into the beneficial and protective actions of the kallikrein-kinin system. Vasc. Pharmacol. 64, 1–10. doi:10.1016/j.vph.2014.12.003
Renné, T., Dedio, J., Meijers, J. C., Chung, D., and Muller-Esterl, W. (1999). Mapping of the discontinuous H-kininogen binding site of plasma prekallikrein. Evidence for a critical role of apple domain-2. J. Biol. Chem. 274 (36), 25777–25784. doi:10.1074/jbc.274.36.25777
Revak, S. D., Cochrane, C. G., and Griffin, J. H. (1977). The binding and cleavage characteristics of human Hageman factor during contact activation. A comparison of normal plasma with plasmas deficient in factor XI, prekallikrein, or high molecular weight kininogen. J. Clin. Invest. 59 (6), 1167–1175. doi:10.1172/JCI108741
Rex, D. A. B., Deepak, K., Vaid, N., Dagamajalu, S., Kandasamy, R. K., Flo, T. H., et al. (2022). A modular map of Bradykinin-mediated inflammatory signaling network. J. Cell Commun. Signal 16 (2), 301–310. doi:10.1007/s12079-021-00652-0
Rosales, C. (2018). Neutrophil: A cell with many roles in inflammation or several cell types? Front. Physiol. 9, 113. doi:10.3389/fphys.2018.00113
Saito, H., Goldsmith, G. H., Moroi, M., and Aoki, N. (1979). Inhibitory spectrum of alpha 2-plasmin inhibitor. Proc. Natl. Acad. Sci. U. S. A. 76 (4), 2013–2017. doi:10.1073/pnas.76.4.2013
Sampaio, C., Wong, S. C., and Shaw, E. (1974). Human plasma kallikrein. Purification and preliminary characterization. Arch. Biochem. Biophys. 165 (1), 133–139. doi:10.1016/0003-9861(74)90150-7
Schapira, M., Scott, C. F., and Colman, R. W. (1982). Contribution of plasma protease inhibitors to the inactivation of kallikrein in plasma. J. Clin. Invest. 69 (2), 462–468. doi:10.1172/jci110470
Scharfstein, J., Andrade, D., Svensjö, E., Oliveira, A. C., and Nascimento, C. R. (2012). The kallikrein-kinin system in experimental chagas disease: A paradigm to investigate the impact of inflammatory edema on GPCR mediated pathways of host cell invasion by trypanosoma cruzi. Front. Immunol. 3, 396. doi:10.3389/fimmu.2012.00396
Scharfstein, J., Ramos, P. I. P., and Barral-Netto, M. G. (2017). G protein-coupled kinin receptors and immunity against pathogens. Adv. Immunol. 136, 29–84. doi:10.1016/bs.ai.2017.05.007
Scharfstein, J. (2018). Subverting bradykinin-evoked inflammation by co-opting the contact system: lessons from survival strategies of trypanosoma cruzi. Curr. Opin. Hematol. 25 (5), 347–357. doi:10.1097/MOH.0000000000000444
Schechter, I., and Berger, A. (1967). On the size of the active site in proteases. I. Papain. Biochem. Biophys. Res. Commun. 27 (2), 157–162. doi:10.1016/s0006-291x(67)80055-x
Schmaier, A. H., and McCrae, K. R. (2007). The plasma kallikrein-kinin system: its evolution from contact activation. J. Thromb. Haemost. 5 (12), 2323–2329. doi:10.1111/j.1538-7836.2007.02770.x
Schmaier, A. H. (1998). Plasma contact activation: A revised hypothesis. Biol. Res. 31 (3), 251–262.
Schmaier, A. H. (2018). Plasma prekallikrein: its role in hereditary angioedema and health and disease. Front. Med. (Lausanne) 5, 3. doi:10.3389/fmed.2018.00003
Schmaier, A. H. (2016). The contact activation and kallikrein/kinin systems: pathophysiologic and physiologic activities. J. Thromb. Haemost. 14 (1), 28–39. doi:10.1111/jth.13194
Shamanaev, A., Litvak, M., and Gailani, D. (2022). Recent advances in factor XII structure and function. Curr. Opin. Hematol. 29 (5), 233–243. doi:10.1097/MOH.0000000000000727
Shamanaev, A., Litvak, M., Ivanov, I., Srivastava, P., Sun, M. F., Dickeson, S. K., et al. (2023). Factor XII structure-function relationships. Semin. Thromb. Hemost. 2023. doi:10.1055/s-0043-1769509
Shariat-Madar, Z., Mahdi, F., and Schmaier, A. H. (2002). Identification and characterization of prolylcarboxypeptidase as an endothelial cell prekallikrein activator. J. Biol. Chem. 277 (20), 17962–17969. doi:10.1074/jbc.M106101200
Sharma, J. N. (2003). Does the kinin system mediate in cardiovascular abnormalities? An overview. J. Clin. Pharmacol. 43 (11), 1187–1195. doi:10.1177/0091270003258171
Silva, L. S., Pinheiro, A. S., Teixeira, D. E., Silva-Aguiar, R. P., Peruchetti, D. B., Scharfstein, J., et al. (2019). Kinins released by erythrocytic stages of Plasmodium falciparum enhance adhesion of infected erythrocytes to endothelial cells and increase blood brain barrier permeability via activation of bradykinin receptors. Front. Med. (Lausanne) 6, 75. doi:10.3389/fmed.2019.00075
Simão, F., and Feener, E. P. (2017). The effects of the contact activation system on hemorrhage. Front. Med. (Lausanne). 4, 121. doi:10.3389/fmed.2017.00121
Simões, P. S. R., Zanelatto, A. O., Assis, M. C., Varella, P. P. V., Yacubian, E. M., Carrete, H., et al. (2019). Plasma kallikrein-kinin system contributes to peripheral inflammation in temporal lobe epilepsy. J. Neurochem. 150 (3), 296–311. doi:10.1111/jnc.14793
Stark, K., and Massberg, S. (2021). Interplay between inflammation and thrombosis in cardiovascular pathology. Nat. Rev. Cardiol. 18 (9), 666–682. doi:10.1038/s41569-021-00552-1
Stavrou, E. X., Fang, C., Merkulova, A., Alhalabi, O., Grobe, N., Antoniak, S., et al. (2015). Reduced thrombosis in Klkb1-/- mice is mediated by increased Mas receptor, prostacyclin, Sirt1, and KLF4 and decreased tissue factor. Blood 125 (4), 710–719. doi:10.1182/blood-2014-01-550285
Stuardo, M., Gonzalez, C. B., Nualart, F., Boric, M., Corthorn, J., Bhoola, K. D., et al. (2004). Stimulated human neutrophils form biologically active kinin peptides from high and low molecular weight kininogens. J. Leukoc. Biol. 75 (4), 631–640. doi:10.1189/jlb.1103546
Svensjö, E., Batista, P. R., Brodskyn, C. I., Silva, R., Lima, A. P., Schmitz, V., et al. (2006). Interplay between parasite cysteine proteases and the host kinin system modulates microvascular leakage and macrophage infection by promastigotes of the Leishmania donovani complex. Microbes Infect. 8 (1), 206–220. doi:10.1016/j.micinf.2005.06.016
Svensjö, E., Nogueira de Almeida, L., Vellasco, L., Juliano, L., and Scharfstein, J. (2014). Ecotin-like ISP of L. major promastigotes fine-tunes macrophage phagocytosis by limiting the pericellular release of bradykinin from surface-bound kininogens: A survival strategy based on the silencing of proinflammatory G-protein coupled kinin B2 and B1 receptors. Mediat. Inflamm. 2014, 143450. doi:10.1155/2014/143450
Taha, E. A., Ono, K., and Eguchi, T. (2019). Roles of extracellular HSPs as biomarkers in immune surveillance and immune evasion. Int. J. Mol. Sci. 20 (18), 4588. doi:10.3390/ijms20184588
Teufel, D. P., Bennett, G., Harrison, H., van Rietschoten, K., Pavan, S., Stace, C., et al. (2018). Stable and long-lasting, novel bicyclic peptide plasma kallikrein inhibitors for the treatment of diabetic macular edema. J. Med. Chem. 61 (7), 2823–2836. doi:10.1021/acs.jmedchem.7b01625
Tomita, H., Sanford, R. B., Smithies, O., and Kakoki, M. (2012). The kallikrein-kinin system in diabetic nephropathy. Kidney Int. 81 (8), 733–744. doi:10.1038/ki.2011.499
Tsutsui, S., Yoshimura, A., Iwakuma, Y., and Nakamura, O. (2023). Discovery of teleost plasma kallikrein/coagulation factor XI-like gene from channel catfish (Ictalurus punctatus) and the evidence that the protein encoded by it acts as a lectin. J. Mol. Evol. 91 (4), 536–551. doi:10.1007/s00239-023-10113-4
Tsutsui, S., Yoshimura, A., Kawakami, Y., and Nakamura, O. (2021). Molecular evolution of kalliklectin in teleost and identification of the novel type with eight apple domains in channel catfish, Ictalurus punctatus. Mol. Biol. Rep. 48 (5), 4305–4318. doi:10.1007/s11033-021-06446-2
van der Graaf, F., Koedam, J. A., and Bouma, B. N. (1983). Inactivation of kallikrein in human plasma. J. Clin. Invest. 71 (1), 149–158. doi:10.1172/jci110743
van der Graaf, F., Tans, G., Bouma, B. N., and Griffin, J. H. (1982). Isolation and functional properties of the heavy and light chains of human plasma kallikrein. J. Biol. Chem. 257 (23), 14300–14305. doi:10.1016/s0021-9258(19)45380-5
Vogel, R., Kaufmann, J., Chung, D. W., Kellermann, J., and Müller-Esterl, W. J. (1990). Mapping of the prekallikrein-binding site of human H-kininogen by ligand screening of lambda gt11 expression libraries. Mimicking of the predicted binding site by anti-idiotypic antibodies. Biol. Chem. 265 (21), 12494–12502. doi:10.1016/s0021-9258(19)38373-5
Weiss, A. S., Gallin, J. I., and Kaplan, A. P. (1974). Fletcher factor deficiency. A diminished rate of Hageman factor activation caused by absence of prekallikrein with abnormalities of coagulation, fibrinolysis, chemotactic activity, and kinin generation. J. Clin. Invest. 53 (2), 622–633. doi:10.1172/JCI107597
Wu, Y. (2015). Contact pathway of coagulation and inflammation. Thromb. J. 13, 17. doi:10.1186/s12959-015-0048-y
Wuepper, K. D., and Cochrane, C. G. (1972). Effect of plasma kallikrein on coagulation in vitro. Proc. Soc. Exp. Biol. Med. 141 (1), 271–276. doi:10.3181/00379727-141-36757
Wuepper, K. D. (1973). Prekallikrein deficiency in man. J. Exp. Med. 138 (6), 1345–1355. doi:10.1084/jem.138.6.1345
Xie, Z., Li, Z., Shao, Y., and Liao, C. (2020). Discovery and development of plasma kallikrein inhibitors for multiple diseases. Eur. J. Med. Chem. 190, 112137. doi:10.1016/j.ejmech.2020.112137
Yu, H., Anderson, P. J., Freedman, B. I., Rich, S. S., and Bowden, D. W. (2000). Genomic Structure of the human plasma prekallikrein gene, identification of allelic variants, and analysis in end-stage renal disease. Genomics 69 (2), 225–234. doi:10.1006/geno.2000.6330
Zanelatto, A. C. O., Lacerda, G. S., Accardo, C. M., Rosário, N. F. D., Silva, A. A. D., Motta, G., et al. (2022). Cathepsin B and plasma kallikrein are reliable biomarkers to discriminate clinically significant hepatic fibrosis in patients with chronic hepatitis-C infection. Microorganisms 10 (9), 1769. doi:10.3390/microorganisms10091769
Zhou, D., Hansen, D., Shabalin, I. G., Gustchina, A., Vieira, D. F., de Brito, M. V., et al. (2015). Structure of BbKI, a disulfide-free plasma kallikrein inhibitor. Acta Crystallogr. F. Struct. Biol. Commun. 71 (8), 1055–1062. doi:10.1107/S2053230X15011127
Keywords: bradykinin, kininogen, kallikrein, proteolysis, cell biology, inflammation, inhibitors
Citation: Motta G, Juliano L and Chagas JR (2023) Human plasma kallikrein: roles in coagulation, fibrinolysis, inflammation pathways, and beyond. Front. Physiol. 14:1188816. doi: 10.3389/fphys.2023.1188816
Received: 17 March 2023; Accepted: 15 August 2023;
Published: 30 August 2023.
Edited by:
Pengcheng Shi, Southern Medical University, ChinaReviewed by:
Indranil Biswas, Oklahoma Medical Research Foundation, United StatesCopyright © 2023 Motta, Juliano and Chagas. This is an open-access article distributed under the terms of the Creative Commons Attribution License (CC BY). The use, distribution or reproduction in other forums is permitted, provided the original author(s) and the copyright owner(s) are credited and that the original publication in this journal is cited, in accordance with accepted academic practice. No use, distribution or reproduction is permitted which does not comply with these terms.
*Correspondence: Guacyara Motta, Z3VhY3lkYW1vdHRhQGdtYWlsLmNvbQ==
Disclaimer: All claims expressed in this article are solely those of the authors and do not necessarily represent those of their affiliated organizations, or those of the publisher, the editors and the reviewers. Any product that may be evaluated in this article or claim that may be made by its manufacturer is not guaranteed or endorsed by the publisher.
Research integrity at Frontiers
Learn more about the work of our research integrity team to safeguard the quality of each article we publish.