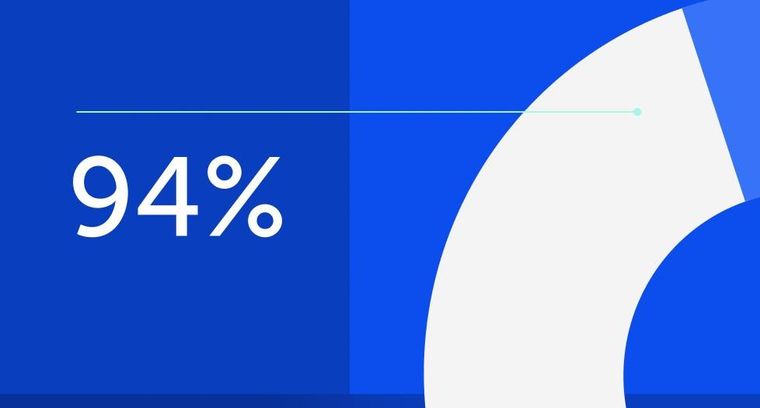
94% of researchers rate our articles as excellent or good
Learn more about the work of our research integrity team to safeguard the quality of each article we publish.
Find out more
ORIGINAL RESEARCH article
Front. Physiol., 08 January 2019
Sec. Invertebrate Physiology
Volume 9 - 2018 | https://doi.org/10.3389/fphys.2018.01890
This article is part of the Research TopicPhysiological Adaptations of Insects Exposed to Different Stress Conditions, Volume IView all 38 articles
Sogatella furcifera, an important migratory pest of rice, has substantial detrimental effects on rice production. To clarify the mechanism whereby S. furcifera responds to insecticide stress, we measured the activity of its protective [superoxide dismutase (SOD); peroxidase (POD); catalase (CAT)] and detoxifying [carboxylesterase (CarE); glutathione S-transferase (GST); mixed-function oxidase (MFO)] enzymes and the expression levels of its ATP-binding cassette subfamily G (ABCG) transporter genes in response to sublethal concentrations (LC10 and LC25) of the insecticides thiamethoxam, buprofezin, and abamectin. On the bases of the transcriptome data and the ABCG genes of Laodelphax striatellus, we obtained 14 full-length ABCG sequences for S. furcifera. RT-qPCR results showed that 13, 12, and 9 sfABCG genes were upregulated in the presence of thiamethoxam, buprofezin, and abamectin, respectively, at LC10. Moreover, 13 and 7 sfABCG genes were upregulated following treatment with thiamethoxam and abamectin, respectively, at LC25. Enzyme activity assays showed that although thiamethoxam, buprofezin, and abamectin induced GST, CarE, CAT, POD, and SOD activity, they did so at different concentrations and exposure times. The activity of MFO was generally inhibited with prolonged exposure to the three insecticides, with the inhibitory effect being most significant at 72 h. These results indicate that S. furcifera differs in its response to different types or concentrations of insecticides. Taken together, our results lay the foundations for gaining a deeper understanding of the mechanisms underlying the adaptation of S. furcifera to different types of insecticides, which would be of considerable significance for the development of effective pest management strategies.
Sogatella furcifera, an important pest of rice, causes serious problems in rice production by sucking phloem sap from the rice plant, inflicting damage through oviposition, and transmitting viral diseases (Zhou et al., 2008). Although the use of insecticides has traditionally been an important means of control for this rice pest (Endo and Tsurumachi, 2001; Nizamani et al., 2002), recent research has shown that sublethal concentrations of insecticides can affect the reproduction, development, and chemical susceptibility of insects in such a way that it could potentially result in the resurgence of pests (Zhou et al., 2017).
In general, the detoxification process in insects can be divided into three phases: phase I, phase II (involving metabolizing enzymes), and phase III (involving transporters) (Xu et al., 2005). The main enzymes involved in the phase I and phase II detoxification processes are P450 monooxygenase, glutathione S-transferase (GST), and carboxylesterase (CarE) (Xiao et al., 2018), whereas the ATP-binding cassette (ABC) transporters are the main components of phase III (Ferreira et al., 2014). In this regard, it has previously been observed that when the nymphs of Locusta migratoria were treated with chlorantraniliprole at LC50, only the activities of esterase (EST) and GST increased on the first day of treatment, whereas mixed-function oxidase (MFO) activity increased only at 3 days after treatment (Cao et al., 2017). In addition, superoxide dismutase (SOD), peroxidase (POD), and catalase (CAT) are three important protective enzymes in insects that play roles in immunity, preventing free-radical-associated damage, and protecting cells from adverse environmental effects (Dubovskiy et al., 2008; Bi et al., 2010). It has previously been reported that in response to treatment with abamectin at LC10 and LC20 for 12 h, the activities of SOD, POD, and CAT in Harmonia axyridis were higher relative to those in the untreated control group, although these activities gradually returned to normal levels as time progressed (Yang et al., 2015). Furthermore, in response to treatment with imidacloprid at LC10 and LC20, the SOD and POD activities in Aphidius gifuensis initially appeared to be inhibited but were subsequently stimulated, with the highest activity occurring at 36 h. Moreover, it has been found that with an increase in insecticide concentration, SOD, POD, and CAT activities show a decreasing trend (Zhu et al., 2015).
The ABC transporters comprise a large family of proteins that mediate the transport of inorganic ions, sugars, amino acids, lipids, lipopolysaccharides, peptides, metals, xenobiotics, and chemotherapeutic drugs (Higgins, 1992). In insects, this family can be subdivided into eight major subfamilies (A–H) (Dean et al., 2001). Studies of the ABC transporters in eukaryotes have revealed that they are capable of transporting structurally unrelated compounds (Dassa and Bouige, 2001; Dean et al., 2001), and researchers are thus increasingly focusing on the roles of these proteins in the transport of exogenous substances and in insecticide resistance in insects. Recent studies have shown that the expression of ABC transporters is directly related to the development of insecticide resistance (Silva et al., 2012a; Dermauw and Van Leeuwen, 2014). After treatment of Bactrocera dorsalis with malathion, abamectin, and beta-cypermethrin at an LD50 concentration, 4, 10, and 14 bdABC genes were significantly upregulated, respectively (Xiao et al., 2018). Quantitative polymerase chain reaction (qPCR) analysis has revealed that eight ABC transporters in the ABCB/C/D/G subfamilies were upregulated in strains of Laodelphax striatellus resistant to chlorpyrifos, deltamethrin, and imidacloprid, compared with those in a susceptible strain (Sun et al., 2017). In Plutella xylostella, RNA sequencing (RNA-seq) analysis showed that ABC transporters from the ABCA/C/G/H/F subfamilies were overexpressed in chlorpyrifos-resistant strains (You et al., 2013). Nevertheless, despite the insights gained from these studies, our current understanding of the role of ABC transporters in insect resistance to insecticides remains limited.
At present, little is known regarding the effects of insecticides on the activities of the detoxifying and protective enzymes and ABC transporters of S. furcifera. Accordingly, in this study, we sought to gain insights into the roles of these enzymes and the sfABCG subfamily genes in the response of S. furcifera to insecticide-induced stress. To this end, we exposed this insect to sublethal concentrations of three insecticides (thiamethoxam, abamectin, and buprofezin) and subsequently monitored the changes in enzyme activity and gene expression levels.
In 2013, S. furcifera individuals were collected from a rice field in Huaxi, Guiyang, Guizhou, China (26°31.302″ N, 106°62.294″ E) and maintained on rice seedlings in the laboratory at 25 ± 1°C and 70 ± 10% relative humidity under a 16:8 h (light:dark) photoperiod, without exposure to insecticides. For the purposes of this study, we used third-instar nymphs. Thiamethoxam (96%: technical formulation) was obtained from PFchem, Co., Ltd. (Nanjing, China); abamectin (96.4%: technical formulation) was obtained from Shandong Qilu King-Phar Pharmaceutical, Co., Ltd. (Shandong, China); and buprofezin (97%: technical formulation) was obtained from the Guangxi Pingle Pesticide Factory (Guangxi, China).
For the insecticide treatments, we used the rice stem dipping method (Zhou et al., 2017). Three 100 third-instar nymphs were transferred to and reared separately in glass tubes (300 mm high × 30 mm diameter) that were open at both ends and contained rice seedlings dipped in a sublethal concentration (LC10 or LC25) of thiamethoxam, abamectin, or buprofezin. Rice stems treated with distilled water were used as a control. The insects exposed to each treatment were maintained at 25 ± 1°C and 70 ± 10% relative humidity under a 16:8 h (light:dark) photoperiod in an artificial climate box. After 48 h, 15 surviving insects from each treatment were randomly collected for extraction of RNA for a quantitative reverse-transcription PCR (RT-qPCR) assay. In addition, samples were taken at 6, 12, 24, 48, and 72 h after the treatment to determine the activity of the target enzymes. The LC10 and LC25 values (Supplementary Table S1) of thiamethoxam, abamectin, and buprofezin for S. furcifera were based on previously presented results (Liu et al., 2015).
The RNA-seq transcriptome database of S. furcifera was sequenced and annotated as described previously (Zhou et al., 2018). With the reported ABCG gene of L. striatellus as a reference, Geneious R9 software (Kearse et al., 2012) was used to assemble the transcriptome data to obtain the corresponding sequences for S. furcifera. In addition, each of the putative ABCG sequences was used as a query to search the NCBI protein database1 to further validate their identity.
Specific primers were designed and used to amplify the internal cDNA fragments. PCRs were carried out using Sangon Biotech (Shanghai, China) Taq polymerase, under the following conditions: initial denaturation at 94°C for 3 min; 30 cycles of denaturation at 94°C for 30 s, annealing at 55–60°C for 30 s, and elongation at 72°C for 1–2 min; with a final elongation at 72°C for 10 min. Specific primers for amplification of the 3′ and 5′ ends were designed using Primer Premier 6.0 (Premier Biosoft International, Palo Alto, CA, United States). Using a SMARTer® RACE 5′/3′ Kit (Clontech, Mountain View, CA, United States), 3′ and 5′ rapid amplification of cDNA ends (RACE) were performed. Total RNA was extracted from 10 fifth-instar nymphs according to the instructions of an HP Total RNA Kit (Omega Bio-Tek, Norcross, GA, United States). Synthesis of the first-strand cDNA and PCR amplifications were carried out according to the instructions of a SMARTer® RACE 5′/3′ Kit. SeqAmp DNA Polymerase (a SMARTer® RACE 5′/3′ Kit component) was used for the RACE PCR, under the following conditions: 25 cycles of 94°C for 30 s, 60–70°C (depending on the primer) for 30 s, and 72°C for 3 min. The overlapping PCR products were purified using an E.Z.N.A® Gel Extraction Kit, cloned into a linearized pRACE vector (a SMARTer® RACE 5′/3′ Kit component), and sequenced by Sangon Biotech (Shanghai, China). The RACE sequences were assembled on the basis of the partial cDNA sequences corresponding to each fragment.
Using ORF finder2, we identified the open reading frames (ORFs) of the sfABCG genes and determined the amino acid sequences of the encoded proteins. The Pfam program3 and a search of the NCBI Conserved Domain Database4 were used to identify the conserved domains (nucleotide-binding and transmembrane domains) of all putative ABCG genes. The ABCG gene sequences were then subjected to phylogenetic analysis, using the neighbor-joining method and a bootstrap test with 1,000 replicates in the MEGA program package, v. 6.0 (Tamura et al., 2011).
The mRNA levels of the ABC transporter genes under different insecticide treatments were measured by RT-qPCR using FastStart Essential DNA Green Master Mix (Roche, Indianapolis, IN, United States) in a CFX96TM real-time quantitative PCR system (BioRad, Hercules, CA, United States). Total RNA was extracted as described above and quantified using a NanoDrop 2000 spectrophotometer (Thermo Fisher Scientific, Waltham, MA, United States) according to the manufacturer’s protocols. The RNA concentration was adjusted to 0.8 μg/μL with diethyl pyrocarbonate-treated H2O, and 0.8 μg of RNA was then reverse transcribed in a 20-μL reaction volume, using the PrimeScript RT Reagent Kit and gDNA Eraser (TaKaRa, Shiga, Japan), with ribosomal protein L9 (GenBank Accession No. KM885285) as an internal control. Specific primer pairs for each gene were designed using Primer Premier 6 (Supplementary Table S2). Each RT-qPCR was conducted in a 20-μL mixture containing 1 μL of sample cDNA, 1 μL of each primer (10 μM), 7 μL of diethyl pyrocarbonate-treated H2O, and 10 μL of FastStart Essential DNA Green Master Mix. The qPCR cycling parameters were as follows: 95°C for 10 min, followed by 40 cycles of 95°C for 30 s and 60°C for 30 s. Melting curve generation was performed from 65 to 95°C. To check the reproducibility of the assay results, the qPCR for each sample was performed using three technical replicates and three biological replicates. The comparative 2-ΔΔCT method (Livak and Schmittgen, 2001) was used to calculate the relative quantification.
In this study, we performed the following enzyme activity assays: the nitroblue tetrazolium reduction method for SOD; the guaiacol method for POD; a spectrophotometric method for CAT (based on the ultraviolet absorption of peroxide released from the activity of CAT on hydrogen peroxide); a colorimetric method for GST (based on the GST-catalyzed reaction between glutathione and 1-chloro-2,4-dinitrobenzene); and a colorimetric method for CarE (based on the CarE-catalyzed transformation of 1-naphthyl acetate to naphthyl ester, which then reacts with the Fast Blue RR salt to form an azo dye). These assays were conducted using respective commercial assay kits (Comin Biotechnology, Co., Ltd., Suzhou, China). MFO activity was measured according to the method reported by Qian et al. (2008). To check the reproducibility of the results, the enzyme activity assays for each insecticide treatment were performed using four biological replicates.
All data were analyzed using Bonferroni corrections for multiple comparisons when the variance was homogeneous. When the variance was non-homogeneous, the Wilcoxon signed-rank test was used. In addition, the Kruskal–Wallis test was used to verify the temporal shifts within the effects of the same insecticide. All analyses were performed using SPSS version 22.0 (SPSS, Chicago, IL, United States) and the data are presented as the mean ± standard error (SE) of three or four biological replicates.
Using the reported ABCG gene of L. striatellus as a reference, Geneious R9 software was used to assemble the transcriptome data to obtain the corresponding sequences for S. furcifera. We verified 14 sfABCG genes by RT-qPCR and RACE (Supplementary Table S3). The designation, accession number, length, ORF size, theoretical isoelectric point, and molecular weight of all the sfABCG genes are summarized in Table 1. The ORFs of all gene sequences ranged from 603 to 967 bp. We initially identified the characteristic nucleotide-binding domains of ABC transporters using Pfam. The nucleotide-binding and transmembrane domains of all genes were similar to those of L. striatellus (Supplementary Figure S2). As determined from the neighbor-joining tree generated from phylogenetic analysis of the ABCG genes of S. furcifera, L. striatellus, Tribolium castaneum, and B. dorsalis, the corresponding genes of each subfamily are clustered together (Supplementary Figure S1).
Table 1. Full-length ATP-binding cassette subfamily G (ABCG) transporter genes identified from Sogatella furcifera.
After exposing third-instar nymphs of S. furcifera to different concentrations of thiamethoxam for 48 h, we examined the relative expression levels of the 14 sfABCG genes. The results showed that the expression of only sfABCG7 was significantly downregulated (2.4-fold) after treatment with the insecticide at LC10 (Figure 1), whereas the other 13 sfABCG genes were significantly upregulated. Among these 13 genes, sfABCG5 (766.6-fold) and sfABCG9 (5.8-fold) showed the highest and lowest upregulation, respectively. Responses to the LC25 treatment were similar to those observed for the LC10 treatment, with only sfABCG7 being significantly downregulated (3.5-fold) and the remaining 13 genes being significantly upregulated by 4.5- to 643.8-fold. However, we found that the expression levels of the upregulated genes showed a decreasing trend with increasing insecticide concentration, with sfABCG3 showing significantly different expression levels in response to the LC10 and LC25 treatments (Wilcoxon signed-rank test P < 0.05).
Figure 1. Relative expression levels of 14 putative ABC subfamily G (ABCG) transporter genes in Sogatella furcifera under treatment with sublethal concentrations (LC10 and LC25) of thiamethoxam, buprofezin, and abamectin. The mean value ± SE was used to analyze the relative expression levels under different insecticide concentrations using the ΔΔCt method, with non-insecticide treatment (CK) as a reference. Different letters indicate significant differences among treatments at the same time. (A) Thiamethoxam; (B) buprofezin; and (C) abamectin.
After treatment with buprofezin at LC10, the relative expression levels of the 14 sfABCG genes showed trends similar to those observed for thiamethoxam at LC10, with only the sfABCG7 gene being significantly downregulated (3.0-fold), and the other 13 genes all being upregulated (significantly in the case of 12), from 6.0- to 924.0-fold. Although the expression of sfABCG9 was upregulated relative to that in the control, the difference was not significant (Figure 1). Buprofezin treatment at LC25 resulted in a significant upregulation of sfABCG5 relative to the control, whereas sfABCG7 was significantly downregulated by 2.2-fold (Bonferroni-corrected P = 0.02) compared with the control level.
After treatment with abamectin at LC10, nine sfABCG genes (sfABCG1, sfABCG3, sfABCG4, sfABCG5, sfABCG6, sfABCG8, sfABCG10, sfABCG11, and sfABCG14) were significantly upregulated in the range of 3.2- to 97.4-fold (Figure 1). In contrast, compared with the control levels, the expression levels of sfABCG7, sfABCG9, sfABCG12, and sfABCG13 were significantly downregulated in response to abamectin treatment at LC10 and LC25 concentrations, with sfABCG13 being the most downregulated by 6.0-fold (LC10) and 13.3-fold (LC25). In response to abamectin exposure at the LC25 concentration, sfABCG1, sfABCG4, sfABCG5, sfABCG6, sfABCG8, and sfABCG14 were significantly upregulated by 17.1-, 8.1-, 73.1-, 10.98-, 7.4-, and 2.7-fold, respectively, compared with the control levels. Interestingly, the expression levels of both sfABCG1 and sfABCG4 were upregulated with increasing abamectin concentration, with the difference being significant in the case of sfABCG4 (Bonferroni-corrected P = 0.04; Figure 1).
To gain a more intuitive understanding of the gene upregulation pattern in response to insecticide exposure, a Venn diagram was generated for the 13 significantly upregulated sfABCG genes after treatment with the three insecticides at LC10 (Figure 2). Among these, sfABCG9 was upregulated by thiamethoxam only; sfABCG2, sfABCG12, and sfABCG13 were upregulated by thiamethoxam and buprofezin; and sfABCG1, sfABCG3, sfABCG4, sfABCG5, sfABCG6, sfABCG8, sfABCG10, sfABCG11, and sfABCG14 were upregulated by all three insecticides. Among the latter group, the sfABCG5 gene showed the highest upregulation responses, with expression levels 766.6-, 924.0-, and 97.4-fold higher than those of the control in response to thiamethoxam, buprofezin, and abamectin treatments, respectively.
Figure 2. Summary of the significantly upregulated genes in Sogatella furcifera under treatment with the insecticides thiamethoxam, buprofezin, and abamectin. The Venn diagram shows the putative ABC subfamily G (ABCG) transporter genes found to be significantly upregulated in the insecticide-treated insects compared with the untreated controls.
Changes in the activity of the detoxifying enzymes in S. furcifera were examined after treatment with sublethal concentrations of the test insecticides for 6, 12, 24, 48, and 72 h (Figures 3–5). Compared with control levels, the activity of CarE was significantly increased after 6 and 12 h of treatment with thiamethoxam, buprofezin, and abamectin at the LC10 and LC25 levels, showing the same trend for all three insecticides and with the activity being highest at 6 h (Figure 3). It is worth noting that after treatment with the three insecticides at LC10 and LC25, there was an initial increase in the overall activity of CarE with time, followed by a decrease, and then subsequently a further increase.
Figure 3. Effects of sublethal concentrations of insecticides on the carboxylesterase (CarE) activity of Sogatella furcifera. Enzyme activities are shown as the mean ± SE. Different letters indicate significant differences among treatments at the same time. (A) Thiamethoxam; (B) buprofezin; and (C) abamectin.
Figure 4. Effects of sublethal concentrations of insecticides on the glutathione S-transferase (GST) activity of Sogatella furcifera. Enzyme activities are shown as the mean ± SE. Different letters indicate significant differences among treatments at the same time. (A) Thiamethoxam; (B) buprofezin; and (C) abamectin.
Figure 5. Effects of sublethal concentrations of insecticides on the mixed-function oxidase (MFO) activity of Sogatella furcifera. Enzyme activities are shown as the mean ± SE. Different letters indicate significant differences among treatments at the same time. (A) Thiamethoxam; (B) buprofezin; and (C) abamectin.
Glutathione S-transferase activity increased gradually and then decreased after treatment with thiamethoxam at LC10, peaking at 24 h (2.8-fold higher than that of the control). However, in response to treatment with thiamethoxam at LC25, there was no significant difference between the GST treatment and control groups after 24 h, and activity of the enzyme returned to normal levels at 72 h (Figure 4A). After treatment with buprofezin at LC10 and LC25, GST activity showed an overall increasing trend, being highest at 6 h after the LC25 treatment, and subsequently decreasing with the prolongation of treatment time, albeit at levels significantly higher than that of the control. In contrast, we observed a significant reduction in GST activity in response to treatment with buprofezin at LC10 for 24 h (Bonferroni-corrected P = 0.04) compared with that of the control, although again the levels had returned to normal at 72 h (Figure 4B). In response to treatment with abamectin at LC10 and LC25, the activity of GST increased significantly at 6 h, reached a maximum at 12 h, and then gradually decreased. Compared with control levels, the activity of this enzyme was significantly higher in response to the LC25 treatment. However, similar to the response to buprofezin treatment at LC10, GST activity following abamectin treatment at LC10 was not significantly different from that of the control at 24 and 72 h (Figure 4C).
Compared with the control, the activity of MFO showed a decreasing trend in response to treatment with thiamethoxam at LC10, with the difference being significant at 6, 24, and 72 h. In contrast, in response to treatment with thiamethoxam at LC25, although the activity of MFO had decreased at 6 and 72 h, we observed a significant increase at 12 h (Bonferroni-corrected P = 0.001) relative to the control level (Figure 5A). In response to buprofezin exposure at LC10, MFO activity was significantly increased at 6 and 12 h compared with that of the control, and reached a peak at 12 h (1.8-fold higher than that of the control). However, at 24, 48, and 72 h, the activity of MFO was significantly reduced. In addition, after treatment with buprofezin at LC25, we detected no significant difference between treatment and control MFO activities at 6 and 12 h, whereas there was a significant increase in activity in response to treatment at 24 h, which thereafter gradually decreased (Figure 5B). In response to treatment with abamectin at LC10 and LC25, MFO activity showed a decreasing trend compared with the control levels, with the difference being significant at 48 and 72 h. However, in response to abamectin treatment at LC10, MFO activity was significantly higher than that of the control after 12 and 24 h (Figure 5C).
The activities of CAT, POD, and SOD were measured at 6, 12, 24, 48, and 72 h after exposure to sublethal concentrations of the test insecticides (Figures 6–8). Although at 6 h after treatment with thiamethoxam at LC10 and LC25, we observed an inhibition of CAT activity, at 12 and 24 h the activity had increased significantly, respectively, but thereafter returned to normal levels (Figure 6A). Following treatment with buprofezin at LC10 and LC25, CAT activity had increased significantly by 1.8- and 2.1-fold at 12 and 6 h, respectively, compared with the control, and in the LC25 treatment group thereafter gradually returned to a normal level (Figure 6B). Similarly, after treatment with abamectin at LC10 and LC25, CAT activity was 2.4- and 1.9-fold higher, respectively, than that of the control at 6 h, and in the LC25 treatment group subsequently underwent a gradual return to normal levels. However, after 48 h of LC25 treatment, the activity of this enzyme had increased significantly to a level 1.6-fold higher than that of the control (Figure 6C). Interestingly, in response to treatment with both buprofezin and abamectin at LC10, CAT activity initially increased, then decreased, and subsequently increased again with a prolongation of exposure time.
Figure 6. Effects of sublethal concentrations of insecticides on the catalase (CAT) activity of Sogatella furcifera. Enzyme activities are shown as the mean ± SE. Different letters indicate significant differences among treatments at the same time. (A) Thiamethoxam; (B) buprofezin; and (C) abamectin.
Figure 7. Effects of sublethal concentrations of insecticides on the peroxidase (POD) activity of Sogatella furcifera. Enzyme activities are shown as the mean ± SE. Different letters indicate significant differences among treatments at the same time. (A) Thiamethoxam; (B) buprofezin; and (C) abamectin.
Figure 8. Effects of sublethal concentrations of insecticides on the superoxide dismutase (SOD) activity of Sogatella furcifera. Enzyme activities are shown as the mean ± SE. Different letters indicate significant differences among treatments at the same time. (A) Thiamethoxam; (B) buprofezin; and (C) abamectin.
In response to treatment with thiamethoxam at both LC10 and LC25, POD activity showed a tendency to initial increase and subsequently return to a normal level (Figure 7A). In the case of the LC10 treatment, POD activity peaked at 24 h (53.7-fold higher than that of the control) and then gradually decreased, albeit at levels still significantly higher than those of the control. In the LC25 treatment group, POD activity peaked at 12 h (45.9-fold higher than that of the control) and then decreased gradually until reaching the normal level at 72 h (Figure 7A). After treatment with buprofezin at LC10, POD activity began to increase significantly at 6 h (Bonferroni-corrected P = 0.004), peaked at 12 h (55.7-fold higher than that of the control), and then decreased gradually to a normal level after 72 h. Similarly, after treatment with buprofezin at LC25, POD activity showed a significant increase at 12 h (53.9-fold higher than that of the control) (Wilcoxon signed-rank test P < 0.05) and then gradually decreased to a normal level after 72 h (Figure 7B). The responses of POD activity following exposure to abamectin at LC10 and LC25 showed similar patterns to those following buprofezin treatment at LC10 and LC25, whereby activity peaked at 12 h (70.3- and 97.7-fold higher than that of the control, respectively) and returned to a normal level after 72 h (Figure 7C).
Compared with the control level, the SOD activity levels following thiamethoxam treatment at LC10 and LC25 were significantly increased at 6 h (1.4- and 2.5-fold higher than that of the control, respectively) and returned to normal levels at 12 h. Subsequently, however, the SOD activity showed a secondary significant increase at 24 h, before eventually returning to a normal level thereafter (Figure 8A). In response to treatment with buprofezin at LC10, SOD activity increased significantly at 6 h (3.4-fold higher than that of the control), and then underwent a gradual decrease (Figure 8B), whereas following treatment at LC25, the activity of this enzyme increased significantly at 12 h to a level 1.6-fold higher than that of the control. After 48 h of exposure to buprofezin at LC10 and LC25, SOD activity had decreased by 59.9 and 26.5%, respectively, compared with the control level, but had returned to a normal level at 72 h (Figure 8B). The responses of SOD activity to treatment with abamectin at LC10 and LC25 showed trends similar to those following buprofezin treatment at LC10 and LC25; however, after 24 h of abamectin treatment at both sublethal concentrations, SOD activity showed a tendency to return to a normal level (Figure 8C).
Previous studies on insects have shown that the protective enzymes SOD, POD, and CAT are related to resistance and the response to insecticide-induced stress. In this regard, it has been reported that sublethal concentrations (LC10 and LC25) of abamectin can promote upregulation of the SOD, POD, and CAT activities in Diadegma semiclausum adults, with activity increasing with increasing insecticide concentration (Jia et al., 2016). In third-instar H. axyridis nymphs exposed to LC10 abamectin, the highest levels of SOD, POD, and CAT activity were recorded at 24, 12, and 24 h, respectively, and were significantly higher than those in the control group (Yang et al., 2015). In the present study, the overall levels of SOD, POD, and CAT activity in abamectin-treated (LC10 and LC25) S. furcifer tended to undergo an initial increase and thereafter gradually return to normal levels, reaching their highest levels at 12, 12, and 6 h, respectively. Interestingly, at 12 and 24 h, POD and CAT activities showed an increase in response to increasing abamectin concentration, which is consistent with the observations on D. semiclausum previously reported by Jia et al. (2016). In contrast, the levels of SOD, POD, and CAT activity in third-instar H. axyridis nymphs were shown to decrease with an increase in abamectin concentration (Yang et al., 2015). In the present study, we found that exposure to thiamethoxam initially tended to promote upregulation of the overall activities of POD and SOD and then inhibit them with an increase in the insecticide concentration from LC10 to LC25, which contrasts with the observations for buprofezin (LC10 and LC25), which initially inhibited and then upregulated POD and SOD activities with increasing sublethal concentration. For CAT, buprofezin initially upregulated and then inhibited enzyme activity with increase in concentration, whereas thiamethoxam tended to initially inhibit and then upregulate CAT activity with increase in concentration. Similar observations have previously be made in Aphidius gifuensis, in which the levels of SOD, POD, and CAT activity tended to decrease with an increase in imidacloprid concentration (LC10, LC20, LC30, and LC50) (Zhu et al., 2015). Such studies indicate that, in insects, SOD, POD, and CAT activities are related to insect resistance and the response to insecticide-induced stress, although the effects of these enzymes may be species, concentration, and time dependent.
The detoxifying enzymes CarE, GST, and MFO are also important components of insect resistance mechanisms, an increase in the activities of which is necessary during insecticide metabolism (Qi et al., 2016). Previously, it has been found that the levels of GST and MFO activity in two color morphs of the pea aphid Acyrthosiphon pisum increased in response to increasing sublethal concentrations of abamectin (LC5, LC10, and LC20) following exposure for over 24 h (Wang and Liu, 2014). Similarly, the activities of CarE, GST, and MFO in Tetranychus urticae were significantly upregulated at 12 h following exposure to abamectin (LC10 and LC25) (Ru et al., 2017). In the present study, avermectin (LC10 and LC25) resulted in a similar significant induction of CarE, GST, and MFO activities in S. furcifera, at 6, 12, and 24 h, respectively. These findings indicate that insects can adapt to the stress induced by avermectin by activating their detoxifying enzymes. In addition, after treatment with thiamethoxam and buprofezin (LC10 and LC25), CarE activity showed an overall trend of initial upregulation and subsequent inhibition, with the activity being highest at 6 h. Thiamethoxam and buprofezin also significantly induced GST activity in S. furcifera, whereas these insecticides were found to have a generally inhibitory effect on the activity of MFO. Previously, it was found that GST and P450 activities in Aphis craccivora were significantly induced after treatment with cycloxaprid and imidacloprid (LC50) for 48 h, whereas in contrast, the activity of the CarE activity was inhibited, although the observed difference was not significant (Wu et al., 2016). In addition, after treating Cydia pomonella with imidacloprid (LC20), Shang et al. (2017) observed a significant induction of CarE and GST activity, whereas MFO activity was significantly inhibited. These findings suggest that MFO may not play a major role in the insect response to stress induced by neonicotinoid insecticides, and that the primary detoxifying enzymes are CarE and GST. The aforementioned findings indicate that detoxifying enzymes enable insects to respond to low levels of insecticide-induced stress; however, similar to protective enzymes, CarE, GST, and MFO are induced at different times in different insects. Moreover, the main enzymes involved in detoxification appear to be species dependent.
The ABC transporters are important participants in the third stage of detoxification and have been widely reported to be involved in insecticide resistance (Qi et al., 2016). In this regard, it has previously been found that the expression levels of an ABCG gene and an ABCC gene were upregulated in S. furcifera treated with a high concentration (LC85) of cycloxaprid, whereas the expression levels of two ABCG genes were upregulated at a low concentration (LC15) of this insecticide (Yang et al., 2016). Transcriptome sequencing has revealed that the ABCB, ABCC, and ABCG subfamily genes are expressed at high levels in a pyrethroid-resistant strain of Aedes aegypti (Bariami et al., 2012). Similarly, results of microarray experiments have shown that genes of the ABCG and ABCH subfamilies are expressed at high levels in resistant strains of Myzus persicae (Silva et al., 2012b), and that the expression levels of ABCG subfamily genes are increased in DDT-resistant strains of Anopheles arabiensis (Jones et al., 2012). Given that ABCG subfamily genes play a role in insecticide resistance in many insects (You et al., 2013; Yang et al., 2016; Sun et al., 2017; Xiao et al., 2018), we decided to study the expression of 14 ABCG subfamily genes in S. furcifera in response to thiamethoxam, buprofezin, and abamectin. We accordingly found that 13 of these 14 sfABCG genes were significantly upregulated after treatment with at least one sublethal concentration of insecticide. On exposure to these insecticides at the LC10 level, 13 sfABCG genes were significantly upregulated by thiamethoxam, 12 were significantly upregulated by thiamethoxam and buprofezin, and nine were upregulated by all three insecticides. Furthermore, 13 and seven sfABCG genes were significantly upregulated after treatment with LC25 concentrations of thiamethoxam and abamectin, respectively. These findings provide further evidence that ABC transporters probably participate in the transport of various substrates related to the resistance to different types of insecticides. Moreover, it is conceivable that, in addition to enhancing the metabolism of S. furcifera, these highly expressed sfABCG genes are associated with cross-resistance in this insect. However, these inferences need to be verified with functional experiments.
The sublethal effects of insecticides on insects are multifaceted, including their effects on insect behavior, reproduction, development, and insecticide resistance. In addition, insect adaptation to insecticide stress is a complex metabolic detoxification process involving the activity of multiple enzymes. The results of our study show that S. furcifera can eliminate insecticides in the body by activating detoxifying enzymes and ABC transporters, and also activate the protective enzyme system to prevent injury to the body. Taken together, our research results lay the foundations for gaining a deeper understanding of the mechanisms contributing to the adaptation of S. furcifera to different types of insecticides, which is of considerable significance with regards to the development of effective pest management strategies.
The gene sequences obtained have been submitted to the NCBI database (Accession Nos. MH481837–MH481850). Other datasets for this study are included in the manuscript and the Supplementary Files.
HY conceived and designed the experiments. ZW and G-YL measured the detoxifying and protective enzyme activities. CZ examined the ABCG gene expression levels and prepared the manuscript. CZ, HY, ZW, D-CJ, and G-YL finalized the manuscript. All authors read and approved the final manuscript.
This research was supported by the National Natural Science Foundation of China (Grant No. 31560522), the Provincial Key Project for Agricultural Science and Technology of Guizhou (Grant Nos. NY20133006 and NY20103064), the International Cooperation Base for Insect Evolutionary Biology and Pest Control (Grant No. [2016]5802), and the Graduate Education Innovation Project of Guizhou Province (Qian Jiao He YJSCXJH, Grant No. [2018] 043).
The authors declare that the research was conducted in the absence of any commercial or financial relationships that could be construed as a potential conflict of interest.
The Supplementary Material for this article can be found online at: https://www.frontiersin.org/articles/10.3389/fphys.2018.01890/full#supplementary-material
Bariami, V., Jones, C. M., Poupardin, R., Vontas, J., and Ranson, H. (2012). Gene amplification, ABC transporters and cytochrome P450s: unraveling the molecular basis of pyrethroid resistance in the dengue vector, Aedes aegypti. PLoS Negl. Trop. Dis. 6:e1692. doi: 10.1371/journal.pntd.0001692
Bi, M. J., Xue, M., Li, Q. L., Wang, H. T., and Liu, A. H. (2010). Effects of feeding on tobacco plants preinfested by Bemisia tabaci (Homoptera: Aleyrodidae) B-biotype on activities of protective enzymes and digestive enzymes in B. tabaci and Myzus persicae (Homoptera: Aphididae). Acta Entomol. Sin. 53, 139–146. doi: 10.16380/j.kcxb.2010.02.015
Cao, G., Jia, M., Zhao, X., Wang, L., Tu, X. B., Wang, G. J., et al. (2017). Effects of chlorantraniliprole on detoxification enzymes activities in Locusta migratoria L. J. Asia Pac. Entomol. 20, 741–746. doi: 10.1016/j.aspen.2017.04.013
Dassa, E., and Bouige, P. (2001). The ABC of ABCs: a phylogenetic and functional classification of ABC systems in living organisms. Res. Microbial. 152, 211–229. doi: 10.1016/s0923-2508(01)01194-9
Dean, M., Rzhetsky, A., and Allikmets, R. (2001). The human ATP-binding cassette (ABC) transporter superfamily. Genome Res. 42, 1007–1017. doi: 10.1101/gr.184901
Dermauw, W., and Van Leeuwen, T. (2014). The ABC gene family in arthropods: comparative genomics and role in insecticide transport and resistance. Insect Biochem. Mol. Biol. 45, 89–110. doi: 10.1016/j.ibmb.2013.11.001
Dubovskiy, I. M., Martemyanov, V. V., Vorontsova, Y. L., Rantala, M. J., Gryzanova, E. V., and Glupov, V. V. (2008). Effect of bacterial infection on antioxidant activity and lipid peroxidation in the midgut of Galleria mellonella L. larvae (Lepidoptera, Pyralidae). Comp. Biochem. Physiol. C Toxicol. Pharmacol. 148, 1–5. doi: 10.1016/j.cbpc.2008.02.003
Endo, S., and Tsurumachi, M. (2001). Insecticide susceptibility of the brown planthopper and the white-backed planthopper collected from Southeast Asia. J. Pestic. Sci. 26, 82–86. doi: 10.1584/jpestics.26.82
Ferreira, M., Costa, J., and Reis-Henriques, M. A. (2014). ABC transporters in fish species: a review. Front. Physiol. 2014:266. doi: 10.3389/fphys.2014.00266
Higgins, C. F. (1992). ABC transporters: from microorganisms to man. Annu. Rev. Cell Biol. 8, 67–113. doi: 10.1146/annurev.cb.08.110192.000435
Jia, B. T., Hong, S. S., Zhang, Y. C., and Cao, Y. W. (2016). Effect of sublethal concentrations of abamectin on protective and detoxifying enzymes in Diagegma semclausum. J. Environ. Entomol. 38, 990–995. doi: 10.3969/j.issn.1674-0858.2016.05.17
Jones, C. M., Toé, H. K., Sanou, A., Namountougou, M., Hughes, A., Diabaté, A., et al. (2012). Additional Selection for insecticide resistance in urban malaria vectors: ddt resistance in Anopheles arabiensis from Bobo-Dioulasso Burkina Faso. PLoS One 7:e45995. doi: 10.1371/journal.pone.0045995
Kearse, M., Moir, R., Wilson, A., Stones-Havas, S., Cheung, M., Sturrock, S., et al. (2012). Geneious basic: an integrated and extendable desktop software platform for the organization and analysis of sequence data. Bioinformatics 28, 1647–1649. doi: 10.1093/bioinformatics/bts199
Liu, L. L., Yang, H., and Jin, D. C. (2015). Sensitivity of Sogatella furcifera (Horváth) to frequently-used insecticides in rice field. Agrochemicals 54, 227–230. doi: 10.16820/j.cnki.1006-0413.2015.03.021
Livak, K. J., and Schmittgen, T. D. (2001). Analysis of relative gene expression data using real–time quantitative PCR and the 2-ΔΔCT method. Methods 25, 402–408. doi: 10.1006/meth.2001.1262
Nizamani, I. A., Talpur, M. A., and Qureshi, K. H. (2002). Effectiveness of different insecticides against white-backed plant hopper, Sogatella furcifera (Horv.) on rice crop. Asian J. Plant Sci. 1, 199–200. doi: 10.3923/ajps.2002.199.200
Qi, W., Ma, X., He, W., Chen, W., Zou, M., Gurr, G. M., et al. (2016). Characterization and expression profiling of ATP-binding cassette transporter genes in the diamondback moth, Plutella xylostella, (L.). BMC Genomics 17:760. doi: 10.1186/s12864-016-3096-1
Qian, L., Cao, G., Song, J., Yin, Q., and Han, Z. (2008). Biochemical mechanisms conferring cross-resistance between tebufenozide and abamectin in Plutella xylostella. Pestic. Biochem. Phys. 91, 175–179. doi: 10.1016/j.pestbp.2008.03.011
Ru, Y., Chen, Y., Shang, S., and Zhang, X. (2017). Effects of sublethal dose of avermectin on the activities of detoxifying enzymes in Teranychus urticae. J. Gansu Agric. Univ. 52, 87–91. doi: 10.13432/j.cnki.jgsau.2017.01.015
Shang, S., Liu, Y., Liu, L., and Wang, D. (2017). Effect of sublethal concentrations of imidacloprid on activities of detoxifying enzymes in Cydia pomonella. J. N. A. F. Univ. 45, 131–137. doi: 10.13207/j.cnki.jnwafu.2017.03.019
Silva, A. X., Bacigalupe, L. D., Luna-Rudloff, M., and Figueroa, C. C. (2012a). Insecticide resistance mechanisms in the green peach aphid Myzus persicae (Hemiptera: Aphididae) II: costs and benefits. PLoS One 7:e36810. doi: 10.1371/journal.pone.0036810
Silva, A. X., Jander, G., Samaniego, H., Ramsey, J. S., and Figueroa, C. C. (2012b). Insecticide resistance mechanisms in the green peach aphid Myzus persicae (Hemiptera: Aphididae) I: a transcriptomic survey. PLoS One 7:e36366. doi: 10.1371/journal.pone.0036366
Sun, H., Pu, J., Chen, F., Wang, J., and Han, Z. (2017). Multiple ATP-binding cassette transporters are involved in insecticide resistance in the small brown planthopper, Laodelphax striatellus. Insect Mol. Boil. 26, 343–355. doi: 10.1111/imb.12299
Tamura, K., Peterson, D., Peterson, N., Stecher, G., Nei, M., and Kumar, S. (2011). MEGA5: molecular evolutionary genetics analysis using maximum likelihood, evolutionary distance, and maximum parsimony methods. Mol. Biol. Evol. 28, 2731–2739. doi: 10.1093/molbev/msr121
Wang, X. Q., and Liu, C. Z. (2014). Detoxification enzymes activities in two color morphs of pea aphid (Acyrthosiphon pisum) treated with different sublethal concentrations of avermectins. Chin. J. Eco Agri. 22, 675–681. doi: 10.3724/SP.J.1011.2014.40093
Wu, Y. C., Xu, Z., Shao, X., Cheng, J., and Li, Z. (2016). Toxicity of cycloxaprid to Aphis craccivora (Koch) and its effects on detoxification enzymes. Chin. J. Pestic. Sci. 18, 710–716.
Xiao, L. F., Zhang, W., Jing, T. X., Zhang, M. Y., Miao, Z. Q., Wei, D. D., et al. (2018). Genome-wide identification, phylogenetic analysis, and expression profiles of ATP-binding cassette transporter genes in the oriental fruit fly, Bactrocera dorsalis (Hendel) (Diptera: Tephritidae). Comp. Biochem. Physiol. Part D Genomics Proteomics 25, 1–8. doi: 10.1016/j.cbd.2017.10.001
Xu, C., Li, C. Y., and Kong, A. T. (2005). Induction of phase I, II and III drug metabolism/transport by xenobiotics. Arch. Pharm. Res. 28, 249–268. doi: 10.1007/bf02977789
Yang, Q., Wang, S., Zhang, W., Yang, T., and Liu, Y. (2015). Toxicity of commonly used insecticides and their influences on protective enzyme activity of multicolored Asian lady beetle Harmonia axyridis (Pallas). J. Plant Prot. 42, 258–263. doi: 10.13802/j.cnki.zwbhxb.2015.02.017
Yang, Y., Zhang, Y., Yang, B., Fang, J., and Liu, Z. (2016). Transcriptomic responses to different doses of cycloxaprid involved in detoxification and stress response in the whitebacked planthopper, Sogatella furcifera. Entomol. Exp. Appl. 158, 248–257. doi: 10.1111/eea.12406
You, M., Yue, Z., He, W., Yang, X., Yang, G., Xie, M., et al. (2013). A heterozygous moth genome provides insights into herbivory and detoxification. Nat. Genet. 45, 220–225. doi: 10.1038/ng.2524
Zhou, C., Liu, L. L., Yang, H., Wang, Z., Long, G. Y., and Jin, D. C. (2017). Sublethal effects of imidacloprid on the development, reproduction, and susceptibility of the white-backed planthopper, Sogatella furcifera (Hemiptera: Delphacidae). J. Asia Pac. Entomol. 20, 996–1000. doi: 10.1016/j.aspen.2017.07.002
Zhou, C., Yang, H., Wang, Z., Long, G. Y., and Jin, D. C. (2018). Comparative transcriptome analysis of Sogatella furcifera (Horváth) exposed to different insecticides. Sci. Rep. 8:8773. doi: 10.1038/s41598-018-27062-4
Zhou, G. H., Wen, J. J., Cai, D. J., Li, P., Xu, D. L., and Zhang, S. G. (2008). Southern rice black-streaked dwarf virus: a new proposed Fijivirus species in the family Reoviridae. Chin. Sci. Bull. 53, 3677–3685. doi: 10.1007/s11434-008-0467-2
Keywords: white-backed planthopper, detoxifying enzyme, protective enzyme, ATP-binding cassette transporter, insecticide stress, response mechanism
Citation: Zhou C, Yang H, Wang Z, Long G-Y and Jin D-C (2019) Protective and Detoxifying Enzyme Activity and ABCG Subfamily Gene Expression in Sogatella furcifera Under Insecticide Stress. Front. Physiol. 9:1890. doi: 10.3389/fphys.2018.01890
Received: 17 August 2018; Accepted: 13 December 2018;
Published: 08 January 2019.
Edited by:
Bin Tang, Hangzhou Normal University, ChinaReviewed by:
Jose Eduardo Serrão, Universidade Federal de Viçosa, BrazilCopyright © 2019 Zhou, Yang, Wang, Long and Jin. This is an open-access article distributed under the terms of the Creative Commons Attribution License (CC BY). The use, distribution or reproduction in other forums is permitted, provided the original author(s) and the copyright owner(s) are credited and that the original publication in this journal is cited, in accordance with accepted academic practice. No use, distribution or reproduction is permitted which does not comply with these terms.
*Correspondence: Hong Yang, YXh5cmlkaXNAMTYzLmNvbQ==
Disclaimer: All claims expressed in this article are solely those of the authors and do not necessarily represent those of their affiliated organizations, or those of the publisher, the editors and the reviewers. Any product that may be evaluated in this article or claim that may be made by its manufacturer is not guaranteed or endorsed by the publisher.
Research integrity at Frontiers
Learn more about the work of our research integrity team to safeguard the quality of each article we publish.