- 1Institute of Physiology, Institute for Clinical and Biomedical Research, Faculty of Medicine, University of Coimbra, Coimbra, Portugal
- 2College of Sciences, One UTSA Circle, University of Texas at San Antonio, San Antonio, TX, United States
Oxidative stress has been defined as an imbalance between oxidants and antioxidants and more recently as a disruption of redox signaling and control. It is generally accepted that oxidative stress can lead to cell and tissue injury having a fundamental role in vascular dysfunction. Physiologically, reactive oxygen species (ROS) control vascular function by modulating various redox-sensitive signaling pathways. In vascular disorders, oxidative stress instigates endothelial dysfunction and inflammation, affecting several cells in the vascular wall. Vascular ROS are derived from multiple sources herein discussed, which are prime targets for therapeutic development. This review focuses on oxidative stress in vascular physiopathology and highlights different strategies to inhibit ROS production.
Introduction
Oxidative stress is an important underlying factor in health and disease. It is originated by an alteration in the balance of reactive oxygen species (ROS) production and antioxidant defense mechanisms (Halliwell, 1994; Betteridge, 2000). Homeostatic ROS concentrations play a crucial role as secondary messengers in many intracellular signaling pathways in both innate and adaptive immune responses (Schieber and Chandel, 2014; Sies, 2017). The problem occurs when ROS bioavailability overtakes the antioxidant defenses. In these conditions, ROS act as destructive agents affecting proteins, lipids and DNA, leading to cellular damage, tissue injury, and inflammation (Halliwell and Gutteridge, 1999). On the other hand, from a mechanistic point of view, oxidative stress may be better defined as a disruption of redox signaling and control. This concept could redirect research to identify crucial perturbations of redox signaling and control and lead to new treatments for oxidative stress-related disease processes (Jones, 2006).
Oxidative stress has been associated with the pathogenesis of several chronic disorders such as neurodegenerative diseases, diabetes, hypercholesterolemia, and atherosclerosis and is a contributory pathogenic factor in obesity-related disorders (Sayre et al., 2001; Schleicher and Friess, 2007; DeMarco et al., 2010; Giacco and Brownlee, 2010; Sena et al., 2013; Bhatti et al., 2017). In addition, vascular oxidative stress is a leading cause in cardiovascular diseases (Li et al., 2014; Förstermann et al., 2017). Elevated oxidative stress leads to vasoconstriction, vascular remodeling, inflammation, and fibrosis (Rodriguez-Porcel et al., 2017). Insufficient cellular protection against oxidative stress has been described as a major factor for the development of vascular diseases (Li et al., 2014). Oxidative stress is also the principal cause of epigenetic changes that occur during aging (Guillaumet-Adkins et al., 2017).
The involvement of reactive oxygen and nitrogen species has been extensively studied in various pathological conditions. Its etiology is diverse.
This review focuses on the oxidative processes that occur mainly in the vasculature and lead to vascular dysfunction.
Vascular Oxidative Stress
Regulation of vascular tone is critical for the homeostatic function of blood vessels and blood supply to peripheral organs. In physiological conditions, maintenance of appropriate endothelial function provides vasorelaxant properties through release of vasoactive substances (Sena et al., 2013). An imbalance between production of vasoprotective and vasorelaxant factors and vasoconstrictor substances by the endothelium is a hallmark of endothelial dysfunction, which precedes numerous vascular pathologies (Sena et al., 2013). In this context, oxidative stress has a crucial role in the initiation and progression of endothelial dysfunction and vascular disease affecting several cells in the vascular wall (Giacco and Brownlee, 2010; Sena et al., 2013; Rodriguez-Porcel et al., 2017).
In addition, vascular oxidative stress promotes systemic inflammation via immune activation. Activated immune cells migrate into the vasculature, and release several factors including ROS, metalloproteinases, cytokines, and chemokines that promote dysfunction and cause vascular damage promoting vasoconstriction and remodeling of blood vessels (Zhou et al., 2017; Norlander et al., 2018). Vascular remodeling, stiffness, structural elastin abnormalities, and increased oxidative stress are hallmarks of vascular damage in hypertension (Martínez-Revelles et al., 2017).
Endothelium
Endothelial cells regulate vascular tone having a crucial role in the control of organ vascular resistance. These cells, through their secretome, influence vascular smooth muscle cells (VSMCs) and circulating cells such as platelets and monocytes (Sena et al., 2013; Rodriguez-Porcel et al., 2017).
The arterial endothelium is subjected to various injurious stimuli such as oscillatory shear stress, disturbed turbulent flow and oxidative stress among others. The major ROS produced in response to several stimuli (such as hyperglycemia, hyperlipidemia, and hypertension) is superoxide anion () that quickly combines with NO to produce peroxynitrite decreasing NO bioavailability and leading to endothelial dysfunction (Sena et al., 2008, 2011). In response, endothelial cells became activated, produce vasoconstrictor agents (thromboxane A2, endothelin-1, or prostaglandin H2) and an inflammatory response is initiated. The endothelium starts expressing adhesion molecules and secretes chemokines such as chemokine (C-C motif) ligand 2 (CCL2) to attract immune cells (Sena et al., 2013). The increased expression of chemokines and proteases in endothelial cells creates a vicious circle perpetuating the inflammatory response (Campbell et al., 2015). During prolonged vascular inflammation, several changes such as an increment in apoptotic cells, remodeling of the extracellular matrix, breakdown of elastic lamella, and endothelial dysfunction, emerge in atheroprone vessels and accelerate atherosclerosis-related complications (Weber and Noels, 2011). Dysfunctional endothelium is crucial to initiate vascular dysfunction leading to several pathologic conditions including macro (atherosclerosis) and microvascular diseases.
Vascular Smooth Muscle Cells
Vasoactive substances exert their vasorelaxant or vasoconstrictive properties through effects on receptors of VSMCs, which are central for the regulation of vascular tone. These substances derive from endothelial cells, vasoactive nerves, and perivascular tissue (Giacco and Brownlee, 2010; Majesky, 2015).
Vascular smooth muscle cells can also be sources of ROS promoting oxidative stress (Lim and Park, 2014). Various stimuli including increased cyclic stretch can promote oxidative stress in VSMCs. It was recently described that lysyl oxidase (LOX), an elastin crosslinking enzyme, is as a novel source of vascular ROS. LOX-derived ROS activate p38 mitogen-activated protein kinase critically influencing elastin structure and vessel stiffness in hypertension (Martínez-Revelles et al., 2017).
Activation of oxidative stress and inflammation occurs via receptors leading to changes in the balance between vasodilators and vasoconstrictors affecting vascular tone and ultimately lead to vascular dysfunction (Bhatt et al., 2014; Lim and Park, 2014). Vascular ROS effects are mediated through redox-sensitive signaling pathways. ROS regulate protein kinases, phosphatases, mitogen-activated protein kinases, and transcription factors; playing an important role as modulators of [Ca2+]i, rho-associated coiled-coil protein kinase (ROCK), and the contractile machinery. Oxidative stress will activate protein kinase C (PKC) leading to the activation of nicotinamide adenine dinucleotide phosphate (NADPH) oxidases and in turn increment oxidative stress. PKC activation will phosphorylate and activate multiple mechanisms that ultimately promote VSMCs contraction. Increased Nox-derived ROS generation enhances calcium signaling, up-regulates ROCK and modulates the actin cytoskeleton, thereby promoting vascular contraction and increasing vascular tone. PKC and ROCK activity play a role in exacerbated vasoconstriction associated with vascular dysfunction (Liu and Khalil, 2018).
Adventitia and Perivascular Adipose Tissue
The adventitia surrounds the tunica media containing many different cell types in close continuity with perivascular tissue (Majesky, 2015), particularly in large arteries, influencing both endothelial (through vasa vasorum) and VSMCs. In this context, perivascular adipose tissue (PVAT) is an important regulator of vasculature, with much more than a supportive and mechanical role it has also endocrine and paracrine functions. Visceral adipose tissue is a known source of adipokines but PVAT is also an active producer of both adipokines and inflammatory cytokines (Omar et al., 2014).
Under physiological conditions, PVAT has anti-contractile and anti-inflammatory properties that protect blood vessels. Recent studies have suggested that aortic PVAT is protective for endothelial dysfunction in hypercholesterolemic LDL receptor knockout mice. Elevated eNOS-derived NO production in aortic PVAT of this animal model is an adaptive mechanism that may protect endothelial function and maintain normal endothelium-dependent relaxation in the early stages of atherosclerotic disease (Baltieri et al., 2018).
In pathologic conditions such as obesity and atherosclerosis, PVAT changes its phenotype and can contribute to vascular oxidative stress because they have an increment in NADPH oxidases in adipocytes with increase activity in obesity-related conditions (Gao et al., 2006; Gil-Ortega et al., 2014; Padilla et al., 2015). In fact, an increment in ROS has been previously described (DeMarco et al., 2010; Salgado-Somoza et al., 2010; Meijer et al., 2011; Sacks and Fain, 2011). In addition, oxidative stress in adipocytes subsequently stimulates recruitment of immune cells (Chatterjee et al., 2009). Perivascular adipocytes also express angiotensinogen (Serazin et al., 2004) and PVAT produces and releases angiotensin II and related peptides (Bujak-Gizycka et al., 2007).
In obesity, PVAT displays hypoxia, inflammation, and oxidative stress culminating in a dysregulated production/secretion of adipokines and cytokines and leading to PVAT changes toward a proinflammatory phenotype. In animal models and in patients with obesity, PVAT phenotype has been described by up-regulation of pro-inflammatory adipokines/cytokines (e.g., leptin, interleukin-6, tumor necrosis factor-α) and down-regulation of anti-inflammatory adipokines/cytokines (e.g., adiponectin and Interleukin-10) (Henrichot et al., 2005; Chatterjee et al., 2009; Marchesi et al., 2009; Ketonen et al., 2010; Meijer et al., 2011; Aghamohammadzadeh et al., 2016; Xia et al., 2016; Sena et al., 2017). In addition, an increment in esterified fatty acids has also been observed (Ghorbani et al., 1997).
The oxidative stress and inflammation in PVAT have a major impact on endothelial function (Wimalasundera et al., 2003; Payne et al., 2010; Sena et al., 2017), vascular stiffness (Tsioufis et al., 2007; Wu et al., 2016), smooth muscle migration (Barandier et al., 2005) and ultimately lead to vascular disease (Marchesi et al., 2009; Ketonen et al., 2010; Ma et al., 2010; Almabrouk et al., 2014; Gil-Ortega et al., 2014; Omar et al., 2014; Molica et al., 2015) reinforcing the “vasocrine” effects of PVAT in obesity-related diseases.
Sources of Reactive Oxygen Species
In biological systems most ROS are generated from mitochondria (Cadenas and Davies, 2000). Mitochondrial dysfunction is an important cause in the development and progression of several diseases: energy surplus and oxidative stress causes the mitochondrial dysfunction fostering ROS production and oxidative stress. Other sources include NADPH oxidase, xanthine oxidase, cytochrome P450, uncoupled endothelial nitric oxide synthase (eNOS enzyme produces instead of NO), myeloperoxidases and lipoxygenases (Madamanchi et al., 2005; Santilli et al., 2015) (Figure 1).
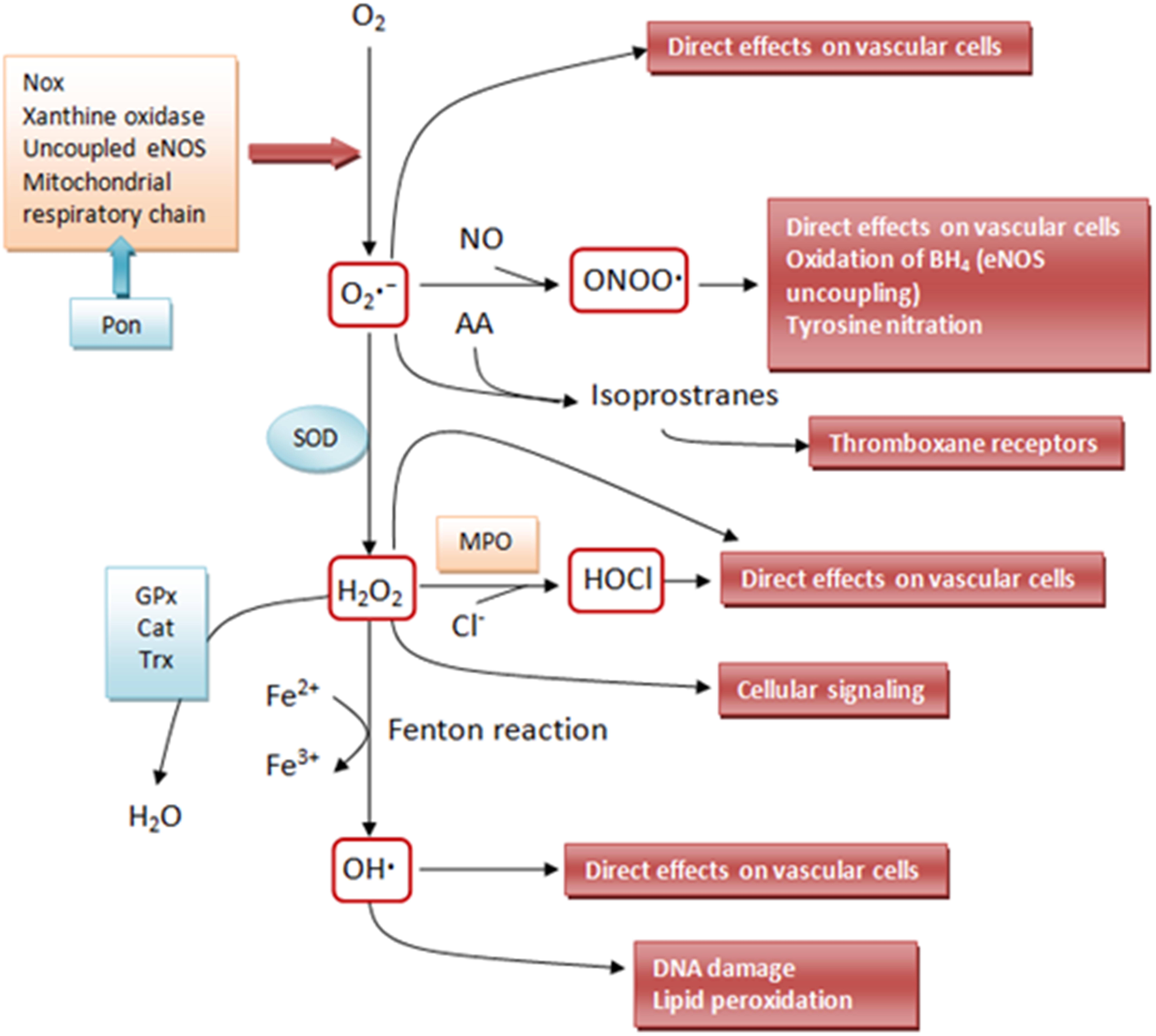
FIGURE 1. Schematic outline of the interrelationships between some of the more relevant reactive oxygen species (ROS) that affect the vascular wall. Superoxide (O2-∙) is produced from molecular oxygen (O2) by different sources such as nicotinamide adenine dinucleotide phosphate – NADPH oxidase (Nox), mitochondrial respiratory chain, xanthine oxidase, uncoupled endothelial nitric oxide synthase (eNOS) and lipoxygenases. Superoxide can directly affect vascular cells but can also be converted by superoxide dismutases (SOD) to hydrogen peroxide (H2O2). H2O2 can undergo spontaneous conversion to hydroxyl radical (OH∙, extremely reactive – attacks most cellular components) in the presence of iron (Fe2+) via the Fenton reaction. H2O2 produces direct effects on the vascular wall or can be detoxified via glutathione peroxidase (GPx), catalase (Cat), or thioredoxin (Trx) peroxidase to H2O and O2. Superoxide can also react with nitric oxide (NO) or arachidonic acid to form peroxynitrite (ONOO⋅-) or isoprostanes, respectively. In addition to other signaling effects, H2O2 can activate Nox, resulting in further production of superoxide. The enzyme myeloperoxidase (MPO) can use H2O2 to oxidize chloride to the strong-oxidizing agent hypochlorous acid (HOCl). HOCl can chlorinate and thereby inactivate various biomolecules including lipoproteins and the eNOS substrate L-arginine. Besides HOCl generation, myeloperoxidase can oxidize (and thus inactivate) NO to nitrite (NO2-) in the vasculature. Paraoxonase (PON) isoforms 2 and 3 can prevent mitochondrial O2∙– generation (Adapted with modifications from De Silva and Faraci, 2017).
Pro-Oxidant Pathways
NADPH Oxidase
NADPH oxidases (Nox) are a crucial contributor to oxidative stress in vascular cells, including endothelial cells, VSMCs, fibroblasts, and perivascular adipocytes (Griendling et al., 2000; Cai et al., 2003). Nox expression and activity are closely linked with clinical risk factors for atherosclerosis (Guzik et al., 2000). There are at least seven variations of the Nox, characterized by their different catalytic subunits (Guzik and Touyz, 2017).
In blood vessels, Nox1 expression is residual under basal conditions and increases considerably after stimuli (Lassègue and Clempus, 2003). Nox2 generates both and H2O2 directly affecting both NO bioavailability and contractile properties of the vasculature (Judkins et al., 2010; Guzik and Touyz, 2017). Nox4 is expressed in all vascular and some perivascular cell types. Nox4 possesses vasorelaxant properties via eNOS activation. It predominantly produces H2O2 and only small amounts of . In the context of atherosclerosis, Nox4 expression appears to exert vasoprotective effects (Ellmark et al., 2005; Guzik and Touyz, 2017). Nox4 was shown to exert both a beneficial as well as detrimental effect (Lener et al., 2009; Kozieł et al., 2013), depending on the cell context and stimuli that influence its activity. Indeed, Nox4 is a source of ROS, which changes the redox-state of numerous proteins, and further research is needed to clarify its role in the vasculature. Nox 5 is also expressed in blood vessels. It is a Nox sensitive calcium isoform that produces (Montezano et al., 2018). Nox 5 is a pro-contractile Nox isoform important in redox-sensitive contraction. It was recently described a novel function for vascular Nox5, linking calcium and ROS to the pro-contractile molecular machinery in VSMCs (Montezano et al., 2018). Further studies are necessary to clarify Nox5 functions.
The renin-angiotensin system also stimulates NADPH oxidase activity contributing to oxidative stress, endothelial dysfunction, and structural vascular changes typical of hypertension and atherosclerosis (Dzau, 1987; Heart Outcomes Prevention Evaluation Study Investigators et al., 2000; Guzik and Touyz, 2017).
The Mitochondrial Respiratory Chain
Mitochondrial oxidative phosphorylation produces , which is transformed to H2O2 by the manganese-dependent superoxide dismutase and subsequently to water by glutathione peroxidase 1 (Boveris et al., 1976). Under pathological conditions, due to insufficient ROS detoxification or excessive ROS production mitochondrial oxidative stress arises (Madamanchi et al., 2005; Yu et al., 2012; Murphy et al., 2016). Several diseases including atherosclerosis in human have been linked with mitochondrial dysfunction and subsequent oxidative stress (Corral-Debrinski et al., 1992).
Xanthine Oxidase
Endothelium dysfunction is linked with an increment in endothelial xanthine oxidase expression (Landmesser et al., 2002; Spiekermann et al., 2003) due to the increased production of superoxide and H2O2. The activity of this enzyme is increased in patients with coronary artery disease (Landmesser et al., 2007) and inhibitors of this enzyme decrement endothelial dysfunction in both humans and animal models (Guthikonda et al., 2003; Schröder et al., 2006; Nomura et al., 2014).
Uncoupled eNOS
Uncoupled eNOS generates instead of NO due to low levels of its cofactor tetrahydrobiopterin (BH4) or its substrate L-arginine (Alp and Channon, 2004). ROS, in particular peroxynitrite, promote eNOS “uncoupling” (Sena et al., 2013; Santilli et al., 2015). Superoxide reacts with NO forming peroxynitrite that further oxidizes BH4 to dihydrobiopterin (BH2), creating a vicious circle and more eNOS uncoupling (Li and Forstermann, 2014). Under physiological conditions, PVAT prevents eNOS uncoupling (Ebrahimian et al., 2009).
Myeloperoxidase
Myeloperoxidase (MPO) is an enzyme that belongs to the mammalian heme peroxidase superfamily, present in polymorphonuclear neutrophils and in monocytes/macrophages (Lefkowitz et al., 2010). MPO produces various compounds with pro-oxidant properties contributing to oxidative stress by oxidizing LDL and lowering NO bioavailability (Pitanga et al., 2014). This enzyme is involved in the formation of products derived from the oxidation of arachidonic acid that are involved in the inflammatory response and in lipid peroxidation (Zhang et al., 2002; Kubala et al., 2010). In addition, MPO promotes atherogenesis through the production of modified subtypes of LDL and HDL lipoproteins (Daugherty et al., 1994; Nicholls and Hazen, 2009; Kettle et al., 2014).
Lipoxygenases
Lipoxygenases (LOXs) are intracellular enzymes that peroxidize polyunsaturated fatty acids into bioactive lipids with a potential important role in the pathogenesis of atherosclerosis. LOXs, in particular 5-LOX and 12/15 LOX were found to be overexpressed in advanced atherosclerotic lesions. 5-LOX converts arachidonic acid into leukotriene B4, a potent chemo-attractant and leukocyte activator (Rådmark et al., 2015). However, inconclusive data were obtained with respect to the pathophysiological relevance of this leukotriene signaling in atherosclerosis. Thus, more studies are necessary to clarify this matter (Kuhn et al., 2015).
Antioxidant Defenses
In the vascular wall, the primary antioxidant defense systems to neutralize ROS production are enzymatic detoxifiers such as superoxide dismutases (MnSOD, CuZnSOD, EcSOD), catalase, glutathione peroxidase, paraoxonase, thioredoxin peroxidase, and heme oxygenases (Santilli et al., 2015). In addition, the transcription factor nuclear factor erythroid-2 related factor 2 (Nrf2) has also been shown to play a key role in establishing a cellular anti-oxidant defense mechanism against oxidative stress (Bryan et al., 2013; Lee, 2017) and is consider an important therapeutic target to manage vascular dysfunction (Förstermann, 2008).
Therapeutics
Current pharmacological approaches for prevalent diseases, such as obesity, diabetes, and cardiovascular diseases are limited in efficacy. Many studies with antioxidants have proven unsuccessful in clinical trials (Steinhubl, 2008). Hence, the search for new therapies is very important an emergent in order to improve the health status and increase lifespan of the patients.
Lifestyle Approaches
Lifestyle interventions are capable of reducing body weight through an increment in physical exercise and a reduction in caloric intake. Weight loss by calorie restriction and/or exercise can improve the global health state reducing oxidative stress (Imayama et al., 2012).
Mitochondrial-Targeted Therapies
An important and potentially useful therapeutic approach for diseases associated with an increment in oxidative stress is to target antioxidants (as ubiquinol or α-tocopherol) to the mitochondria (Milagros Rocha and Victor, 2007; Murphy and Smith, 2007) with lipophilic cations such as mitoquinone (MitoQ) or MitoE2 (Murphy and Smith, 2007; Smith et al., 2008). However, some studies revealed that MitoQ may be prooxidant and proapoptotic because its quinone group can participate in redox cycling and superoxide production. In light of these results, studies using mitoquinone as an antioxidant should be interpreted with caution (Doughan and Dikalov, 2007).
In addition, enzymatic systems or cell-permeable cationic peptides (Szeto-Schiller peptides) directed to the mitochondria can also be an alternative therapeutic approach (Szeto, 2006).
The mitochondrial protein p66Shc is fundamental in the homeostasis of mitochondria. Targeting this adaptor is another form of decreasing mitochondrial oxidative stress. P66Shc leads to ROS generation (Camici et al., 2015) and its inactivation may be essential in oxidative stress related diseases (Francia et al., 2004). Previous studies have demonstrated that the expression of p66Shc is reduced by SIRT1 activation, which in turn decrements oxidative stress and endothelial dysfunction (Chen et al., 2013). SIRT mimetics are therefore promising tools to mitigate vascular disease progression.
Nrf2 Activators
Nrf2 is a transcription factor ubiquitously expressed in various tissues (including the vasculature) of human and animal models (Pereira et al., 2017; Suzuki and Yamamoto, 2017). It regulates the expression of several antioxidant and detoxification enzymes by binding to upstream antioxidant response elements (Ungvari et al., 2011; Juurlink, 2012).
This transcriptional factor is crucial in the prevention of oxidative stress related-diseases (Kobayashi and Yamamoto, 2006; Lee, 2017). Nrf2 interacts with antioxidant response element sequences of genes coding for antioxidant enzymes include γ-glutamyl cysteine ligase, NAD(P)H quinone oxidoreductase-1, glutathione S-transferase, heme oxygenase-1, uridine diphosphate glucuronosyl transferase, superoxide dismutase, catalase, and glutathione peroxidase-1 having a major role in cellular responses to oxidative stress (Alam et al., 1999).
Nrf2 activators can act through different mechanisms (Niture et al., 2014): they can directly prevent the interacting of Nrf2 with the Nrf2-binding site of Kelch-ECH-associated protein-1 (KEAP1) (as, for instance, ML334) (Jiang et al., 2016), they enhance transcription of Nrf2-targeted antioxidant genes (as berberine) (Imenshahidi and Hosseinzadeh, 2016) or they specifically and reversibly increment Nrf2 half-life (as MG-132) (Dreger et al., 2009). Other compounds promote the release of Nrf2 from KEAP1 through interaction with its cysteine thiol residues (as sulforaphane) (Hong et al., 2005).
Nox Inhibitors and Recouplers of eNOS
Triazolopyrimidines are efficient and selective inhibitors of NADPH oxidase activity. They specifically inhibit NADPH oxidase-derived ROS in vitro (ten Freyhaus et al., 2006; Drummond et al., 2011; Santilli et al., 2015) and have great potential in the field of atherosclerosis.
GKT137831, an inhibitor of NOx 1 and Nox4, reduces oxidative stress and diabetic vasculopathy (Gray et al., 2017) and is currently under clinical trial. GLX351322 has been suggested as a therapeutic approach in type 2 diabetes through its selective inhibition of NOX4 (Anvari et al., 2015). In addition, rutin, a glycoside of quercetin, exhibits anti-oxidant and anti-inflammatory properties protecting endothelial dysfunction through inhibition of NOx4 and the ROS-sensitive NLRP3 inflammasome (Wang et al., 2017).
The aldehyde dehydrogenase 2 and its activator Alda-1 are novel molecules capable of inhibiting toxic aldehydes, decreasing oxidative stress and NADPH oxidase activity. These molecules are able to reverse mitochondria dysfunction having a potential role in atherogenesis (Stachowicz et al., 2014; Yang et al., 2018). Nebivolol inhibits the activity of NADPH oxidase and, in addition, reverses eNOS uncoupling (Munzel and Gori, 2009).
Inhibiting eNOS uncoupling is another strategy capable of reducing ROS. Among the drugs used in the clinical practice, inhibitors of the renin–angiotensin–aldosterone system, statins, metformin and pentaerythritol tetranitrate have been described to prevent or reverse eNOS uncoupling. Other compounds, such as resveratrol, sepiapterin, BH4, folic acid, α-lipoic acid and AVE3085, improve endothelial function through eNOS recoupling although further studies are needed to validate these compounds (Li and Forstermann, 2014; Xia et al., 2017). Recently, thioredoxin was shown to reverse age-related hypertension and arterial stiffness by improving vascular redox and restoring eNOS function (Hilgers et al., 2017).
Targeting Hyperglycemic Memory
Inhibiting hyperglycemic memory concomitantly with a reduction in oxidative stress is crucial under diabetic conditions. The hyperglycemic memory needs to be reduced in diabetes in order to prevent the progression of diabetic complications (Bianchi et al., 2013). Otherwise, this occurrence will result in a vicious cycle continuously producing ROS and leading to vascular dysfunction. Targeting the incretin pathway with dipeptidyl peptidase inhibitors or glucagon-like peptide receptor-1 agonists was able to reduce advanced glycation end products (AGEs) and ROS downstream events that promote cellular damage (Berezin, 2016). Therapies associated with reversion of hyperglycemic memory deserve further investigation in this field and include soluble RAGE/RAGE antagonists, AGE/methylglyoxal inhibitors, S100 inhibitors, targeting vascular protein lysine acetylation (Carrillo-Sepulveda, 2017; Kraakman et al., 2017), among others.
Anti-diabetic Drugs
Studies both in humans and in animal models revealed that anti-diabetic drugs (such as metformin) are able to decrement oxidative stress and inflammation and ameliorate endothelial function reducing the progression toward atherosclerosis (Sena et al., 2009, 2011; Jenkins et al., 2018). Incretin mimetics, in particular GLP1 agonists and dipeptidyl peptidase-4 (DPP4) inhibitors, are currently anti-diabetic agents with a wide range of beneficial effects that include antioxidant effects. An example is linagliptin, an inhibitor of DPP4 that reduces obesity-related insulin resistance and inflammation by regulating M1/M2 macrophage status (Zhuge et al., 2016). It was recently shown that saxagliptin, an inhibitor of DPP4, prevented coronary vascular stiffness through a mechanism that involved a decrement in AGEs, NF-κB, and nitrotyrosine levels in aortic-banded mini swine (Fleenor et al., 2018).
In obesity, diabetes and hypertension mineralocorticoid receptor antagonism improves endothelial function and seems to be a mediator of the switch from vascular health to disease. In endothelial cells, the mineralocorticoid receptor exerts a protective role on endothelial function. In the presence of cardiovascular risk factors, endothelial mineralocorticoid receptor contributes to endothelial dysfunction through NOX activation, eNOS uncoupling, increased epithelial sodium channel expression, and ICAM1/VCAM1-mediated inflammation. Further studies are necessary to clarify these mechanisms (Bakris et al., 2015; Davel et al., 2017).
PCSK-9 Inhibitors
PCSK9 inhibitors are monoclonal antibodies that bind to and inactivate proprotein convertase subtilisinkexin 9 (PCSK9), a liver enzyme that promotes the lysosomal degradation of LDL receptors in hepatocytes thus increasing the number of LDL receptors in the membrane and LDL-cholesterol uptake. In recent studies, evolocumab for 52 weeks significantly decreased the level of vitamin E in LDL-C and increased vitamin E level in HDL (Blom et al., 2015) revealing antioxidant properties (Sabatine et al., 2017).
Other Pharmacological Approaches
Serotonin in peripheral blood reflects oxidative stress and plays an important role in atherosclerosis highlighting the novel anti-atherothrombotic strategy to mitigate vascular disease (Sugiura et al., 2016). Tropisetron, a 5-HT3 receptor antagonist, can attenuate early diabetes through calcineurin inhibition and by suppressing oxidative stress and some inflammatory cytokines in streptozotocin-induced diabetic rats (Barzegar-Fallah et al., 2015).
More recently, cell-permeable peptides mimicking the kinase inhibitory region of suppressor of cytokine signaling- 1 (SOCS1) regulatory protein emerged as an important antioxidant and anti-inflammatory strategy to limit the progression of diabetic complications (Lopez-Sanz et al., 2018). SOCS-targeted therapies have recently been suggested as potential therapeutic approaches in atherosclerosis and deserve further investigation.
Other approach includes the inhibition of selenoprotein P (SeP). SeP is a liver derived secretory protein that promotes insulin resistance (Misu et al., 2010) and is upregulated in the liver of type 2 diabetic patients. This hepatokine downregulates the metabolic switch, AMP-activated protein kinase (AMPK) (Misu et al., 2010). Recent studies have suggested that SeP regulates cellular metabolism and the development of vascular diseases (Ishikura et al., 2014; Cetindaǧlı et al., 2017; Mita et al., 2017; Kikuchi et al., 2018). SeP promotes vascular smooth cell proliferation through increased oxidative stress and mitochondrial dysfunction in an autocrine/paracrine manner. Sanguinarine, an orally active small molecule, reduces SeP expression and smooth muscle proliferation, and ameliorates pulmonary arterial hypertension in mice and rats. Thus, it seems that SeP could be a novel and realistic therapeutic target (Kikuchi et al., 2018).
The clinical benefit of these compounds awaits further confirmation. Moreover, novel nanotherapeutic approaches are being developed to target oxidative stress and inflammation (Wang et al., 2018).
Traditional medicinal plants have been used by several civilizations through the years contributing to the notion that natural products are relevant sources of new pharmaceutical compounds. Technological advances are now capable of unravel the value of natural products as novel sources for new drug discovery including their role as antioxidants.
Conclusion
Vascular oxidative stress promotes endothelial dysfunction and atherosclerosis progression. In the vasculature, several sources promote an increment in oxidative stress.
Preventing vascular oxidative stress and incrementing NO bioavailability may represent the future therapeutic strategy to mitigate the cardiovascular burden and reduce associated risk factors.
Author Contributions
CS wrote the article. AL did bibliographic research. LA did bibliographic research. RS revised the manuscript. GP revised the manuscript.
Funding
This study was supported by Fundação para a Ciência e a Tecnologia (Award IDs: PTDC/BIM-MET/4447/2014 and COMPETE:POCI-01-0145-FEDER-016784).
Conflict of Interest Statement
The authors declare that the research was conducted in the absence of any commercial or financial relationships that could be construed as a potential conflict of interest.
References
Aghamohammadzadeh, R., Unwin, R. D., Greenstein, A. S., and Heagerty, A. M. (2016). Effects of obesity on perivascular adipose tissue vasorelaxant function: nitric oxide, inflammation and elevated systemic blood pressure. J. Vasc. Res. 52, 299–305. doi: 10.1159/000443885
Alam, J., Stewart, D., Touchard, C., Boinapally, S., Choi, A. M., and Cook, J. L. (1999). Nrf2, a Cap‘n’Collar transcription factor, regulates induction of the heme oxygenase-1 gene. J. Biol. Chem. 274, 26071–26078. doi: 10.1074/jbc.274.37.26071
Almabrouk, T. A., Ewart, M. A., Salt, I. P., and Kennedy, S. (2014). Perivascular fat, AMP-activated protein kinase and vascular diseases. Br. J. Pharmacol. 171, 595–617. doi: 10.1111/bph.12479
Alp, N. J., and Channon, K. M. (2004). Regulation of endothelial nitric oxide synthase by tetrahydrobiopterin in vascular disease. Arterioscler. Thromb. Vasc. Biol. 24, 413–420. doi: 10.1161/01.ATV.0000110785.96039.f6
Anvari, E., Wikström, P., Walum, E., and Welsh, N. (2015). The novel NADPH oxidase 4 inhibitor GLX351322 counteracts glucose intolerance in high-fat diet-treated C57BL/6 mice. Free Radic. Res. 49, 1308–1318. doi: 10.3109/10715762.2015.1067697
Bakris, G. L., Agarwal, R., Chan, J. C., Cooper, M. E., Gansevoort, R. T., Haller, H., et al. (2015). Effect of finerenone on albuminuria in patients with diabetic nephropathy: a randomized clinical trial. JAMA 314, 884–894. doi: 10.1001/jama.2015.10081
Baltieri, N., Guizoni, D. M., Victorio, J. A., and Davel, A. P. (2018). Protective role of perivascular adipose tissue in endothelial dysfunction and insulin-induced vasodilatation of hypercholesterolemic LDL receptor-deficient mice. Front. Physiol. 9:229. doi: 10.3389/fphys.2018.00229
Barandier, C., Montani, J.-P., and Yang, Z. (2005). Mature adipocytes and perivascular adipose tissue stimulate vascular smooth muscle cell proliferation: effects of aging and obesity. Am. J. Physiol. 289, H1807–H1813. doi: 10.1152/ajpheart.01259.2004
Barzegar-Fallah, A., Alimoradi, H., Asadi, F., Dehpour, A. R., Asgari, M., and Shafiei, M. (2015). Tropisetron ameliorates early diabetic nephropathy in streptozotocin-induced diabetic rats. Clin. Exp. Pharmacol. Physiol. 42, 361–368. doi: 10.1111/1440-1681.12373
Berezin, A. (2016). Metabolic memory phenomenon in diabetes mellitus: achieving and perspectives. Diabetes Metab. Syndr. 10, S176–S183. doi: 10.1016/j.dsx.2016.03.016
Bhatt, S. R., Lokhandwala, M. F., and Banday, A. A. (2014). Vascular oxidative stress upregulates angiotensin II type I receptors via mechanisms involving nuclear factor kappa B. Clin. Exp. Hypertens. 1963, 367–373. doi: 10.3109/10641963.2014.943402
Bhatti, J. S., Kumar, S., Vijayan, M., Bhatti, G. K., and Reddy, P. H. (2017). Therapeutic strategies for mitochondrial dysfunction and oxidative stress in age-related metabolic disorders. Prog. Mol. Biol. Transl. Sci. 146, 13–46. doi: 10.1016/bs.pmbts.2016.12.012
Bianchi, C., Miccoli, R., and Del Prato, S. (2013). Hyperglycemia and vascular metabolic memory: truth or fiction? Curr. Diab. Rep. 13, 403–410. doi: 10.1007/s11892-013-0371-2
Blom, D. J., Djedjos, C. S., Monsalvo, M. L., Bridges, I., Wasserman, S. M., Scott, R., et al. (2015). Effects of evolocumab on Vitamin E and steroid hormone levels: results from the 52-week, phase 3, double-blind, randomized, placebo-controlled DESCARTES study. Circ. Res. 117, 731–741. doi: 10.1161/CIRCRESAHA.115.307071
Boveris, A., Cadenas, E., and Stoppani, A. O. (1976). Role of ubiquinone in the mitochondrial generation of hydrogen peroxide. Biochem. J. 156, 435–444. doi: 10.1042/bj1560435
Bryan, H. K., Olayanju, A., Goldring, C. E., and Park, B. K. (2013). The Nrf2 cell defence pathway: keap1-dependent and -independent mechanisms of regulation. Biochem. Pharmacol. 85, 705–717. doi: 10.1016/j.bcp.2012.11.016
Bujak-Gizycka, B., Madej, J., Wołkow, P. P., Olszanecki, R., Drabik, L., Rutowski, J., et al. (2007). Measurement of angiotensin metabolites in organ bath and cell culture experiments by liquid chromatography-electrospray ionization-mass spectrometry (LC-ESI-MS). J. Physiol. Pharmacol. 58,529–540.
Cadenas, E., and Davies, K. J. (2000). Mitochondrial free radical generation, oxidative stress, and aging. Free Radic. Biol. Med. 29, 222–230. doi: 10.1016/S0891-5849(00)00317-8
Cai, H., Griendling, K. K., and Harrison, D. G. (2003). The vascular NAD(P)H oxidases as therapeutic targets in cardiovascular diseases. Trends Pharmacol. Sci. 24, 471–478. doi: 10.1016/S0165-6147(03)00233-5
Camici, G. G., Savarese, G., Akhmedov, A., and Lüscher, T. F. (2015). Molecular mechanism of endothelial and vascular aging: implications for cardiovascular disease. Eur. Heart J. 36, 3392–3403. doi: 10.1093/eurheartj/ehv587
Campbell, N. R., Lackland, D. T., Lisheng, L., Niebylski, M. L., Nilsson, P. M., and Zhang, X. H. (2015). Using the Global Burden of Disease study to assist development of nation-specific factsheets to promote prevention and control of hypertension and reduction in dietary salt: a resource from the World Hypertension League. J. Clin. Hypertens. 17, 165–167. doi: 10.1111/jch.12479
Carrillo-Sepulveda, M. A. (2017). Vascular protein lysine acetylation: potential epigenetic mechanisms mediating diabetic vascular dysfunction and metabolic memory. FASEB J. 31(Suppl. 1):1014.19.
Cetindaǧlı, I., Kara, M., Tanoglu, A., Ozalper, V., Aribal, S., Hancerli, Y., et al. (2017). Evaluation of endothelial dysfunction in patients with nonalcoholic fatty liver disease: association of selenoprotein P with carotid intima-media thickness and endothelium-dependent vasodilation. Clin. Res. Hepatol. Gastroenterol. 41, 516–524. doi: 10.1016/j.clinre.2017.01.005
Chatterjee, T. K., Stoll, L. L., Denning, G. M., Harrelson, A., Blomkalns, A. L., Idelman, G., et al. (2009). Proinflammatory phenotype of perivascular adipocytes: influence of high-fat feeding. Circ Res. 104, 541–549. doi: 10.1161/CIRCRESAHA.108.182998
Chen, H. Z., Wan, Y. Z., and Liu, D. P. (2013). Cross-talk between SIRT1 and p66Shc in vascular diseases. Trends Cardiovasc. Med. 23, 237–241. doi: 10.1016/j.tcm.2013.01.001
Corral-Debrinski, M., Shoffner, J. M., Lott, M. T., and Wallace, D. C. (1992). Association of mitochondrial DNA damage with aging and coronary atherosclerotic heart disease. Mutat. Res. 275, 169–180. doi: 10.1016/0921-8734(92)90021-G
Daugherty, A., Dunn, J. L., Rateri, D. L., and Heinecke, J. W. (1994). Myeloperoxidase, a catalyst for lipoprotein oxidation, is expressed in human atherosclerotic lesions. J. Clin. Invest. 94, 437–444. doi: 10.1172/JCI117342
Davel, A. P., Anwar, I. J., and Jaffe, I. Z. (2017). The endothelial mineralocorticoid receptor: mediator of the switch from vascular health to disease. Curr. Opin. Nephrol. Hypertens. 26, 97–104. doi: 10.1097/MNH.0000000000000306
DeMarco, V., Johnson, M., Whaley-Connell, A., and Sowers, J. (2010). Cytokine abnormalities in the etiology of the cardiometabolic syndrome. Curr. Hypertens. Rep. 12, 93–98. doi: 10.1007/s11906-010-0095-5
De Silva, T. M., and Faraci, F. M. (2017). “Reactive oxygen species and the regulation of cerebral vascular tone,” in Studies on Atherosclerosis. Oxidative Stress in Applied Basic Research and Clinical Practice, eds M. Rodriguez-Porcel, A. Chade, and J. Miller (Boston, MA: Humana Press),89–112.
Doughan, A. K., and Dikalov, S. I. (2007). Mitochondrial redox cycling of mitoquinone leads to superoxide production and cellular apoptosis. Antioxid. Redox Signal. 9, 1825–1836. doi: 10.1089/ars.2007.1693
Dreger, H., Westphal, K., Weller, A., Baumann, G., Stangl, V., Meiners, S., et al. (2009). Nrf2-dependent upregulation of antioxidative enzymes: a novel pathway for proteasome inhibitor-mediated cardioprotection. Cardiovasc. Res. 83, 354–361. doi: 10.1093/cvr/cvp107
Drummond, G. R., Selemidis, S., Griendling, K. K., and Sobey, C. G. (2011). Combating oxidative stress in vascular disease: NADPH oxidases as therapeutic targets. Nat. Rev. Drug Discov. 10, 453–471. doi: 10.1038/nrd3403
Dzau, V. J. (1987). Implications of local angiotensin production in cardiovascular physiology and pharmacology. Am. J. Cardiol. 59, A59–A65. doi: 10.1016/0002-9149(87)90178-0
Ebrahimian, T., Angulo, O., Paradis, P., and Schiffrin, E. L. (2009). Endothelial nitric oxide synthase uncoupling and perivascular adipose oxidative stress and inflammation contribute to vascular dysfunction in a rodent model of metabolic syndrome. Hypertension 54, 1384–1392. doi: 10.1161/HYPERTENSIONAHA.109.138305
Ellmark, S. H., Dusting, G. J., Fui, M. N., Guzzo-Pernell, N., and Drummond, G. R. (2005). The contribution of Nox4 to NADPH oxidase activity in mouse vascular smooth muscle. Cardiovasc. Res. 65, 495–504. doi: 10.1016/j.cardiores.2004.10.026
Fleenor, B. S., Ouyang, A., Olver, T. D., Hiemstra, J. A., Cobb, M. S., Minervini, G., et al. (2018). Saxagliptin prevents increased coronary vascular stiffness in aortic-banded mini swine. Hypertension 72, 466–475. doi: 10.1161/HYPERTENSIONAHA.118.10993
Förstermann, U. (2008). Oxidative stress in vascular disease: causes, defense mechanisms and potential therapies. Nat. Clin. Pract. Cardiovasc. Med. 5, 338–349. doi: 10.1038/ncpcardio1211
Förstermann, U., Xia, N., and Li, H. (2017). Roles of vascular oxidative stress and nitric oxide in the pathogenesis of atherosclerosis. Circ. Res. 120, 713–735. doi: 10.1161/CIRCRESAHA.116.309326
Francia, P., delli Gatti, C., Bachschmid, M., Martin-Padura, I., Savoia, C., Migliaccio, E., et al. (2004). Deletion of p66shc gene protects against age-related endothelial dysfunction. Circulation 110, 2889–2895. doi: 10.1161/01.CIR.0000147731.24444.4D
Gao, Y. J., Takemori, K., Su, L. Y., An, W. S., Lu, C., Sharma, A. M., et al. (2006). Perivascular adipose tissue promotes vasoconstriction: the role of superoxide anion. Cardiovasc. Res. 71, 363–373. doi: 10.1016/j.cardiores.2006.03.013
Ghorbani, M., Claus, T. H., and Himms-Hagen, J. (1997). Hypertrophy of brown adipocytes in brown and white adipose tissues and reversal of diet-induced obesity in rats treated with a 3-adrenoceptor agonist. Biochem. Pharmacol. 54, 121–131. doi: 10.1016/S0006-2952(97)00162-7
Giacco, F., and Brownlee, M. (2010). Oxidative stress and diabetic complications. Circ. Res. 107, 1058–1070. doi: 10.1161/CIRCRESAHA.110.223545
Gil-Ortega, M., Condezo-Hoyos, L., García-Prieto, C. F., Arribas, S. M., González, M. C., Aranguez, I., et al. (2014). Imbalance between pro and anti-oxidant mechanisms in perivascular adipose tissue aggravates long-term high-fat diet-derived endothelial dysfunction. PLoS One 9:e95312. doi: 10.1371/journal.pone.0095312
Gray, S. P., Jha, J. C., Kennedy, K., van Bommel, E., Chew, P., Szyndralewiez, C., et al. (2017). Combined NOX1/4 inhibition with GKT137831 in mice provides dose-dependent Reno- and atheroprotection even in established micro- and macrovascular disease. Diabetologia 60, 927–937. doi: 10.1007/s00125-017-4215-5
Griendling, K. K., Sorescu, D., and Ushio-Fukai, M. (2000). NAD(P)H oxidase: role in cardiovascular biology and disease. Circ. Res. 86, 494–501. doi: 10.1161/01.RES.86.5.494
Guillaumet-Adkins, A., Yañez, Y., Peris-Diaz, M. D., Calabria, I., Palanca-Ballester, C., and Sandoval, J. (2017). Epigenetics and oxidative stress in aging. Oxid. Med. Cell. Longev. 2017:9175806. doi: 10.1155/2017/9175806
Guthikonda, S., Sinkey, C., Barenz, T., and Haynes, W. G. (2003). Xanthine oxidase inhibition reverses endothelial dysfunction in heavy smokers. Circulation 107, 416–421. doi: 10.1161/01.CIR.0000046448.26751.58
Guzik, T. J., and Touyz, R. M. (2017). Vascular physiology of hypertension. ESC Textbook Vasc. Biol. 291, 291–307.
Guzik, T. J., West, N. E., Black, E., McDonald, D., Ratnatunga, C., Pillai, R., et al. (2000). Vascular superoxide production by NAD(P)H oxidase: association with endothelial dysfunction and clinical risk factors. Circ Res. 86, e85–e90. doi: 10.1161/01.RES.86.9.e85
Halliwell, B. (1994). Free radicals, antioxidants and human disease: curiosity, cause or consequence. Lancet 344, 721–724. doi: 10.1016/S0140-6736(94)92211-X
Halliwell, B., and Gutteridge, J. M. C. (1999). Free Radicals in Biology and Medicine, 3rd Edn. NewYork, NY: Oxford University Press.
Heart Outcomes Prevention Evaluation Study Investigators, Yusuf, S., Sleight, P., Pogue, J., Bosch, J., Davies, R., et al. (2000). Effects of an angiotensin-converting-enzyme inhibitor, ramipril, on cardiovascular events in high-risk patients. New Engl. J. Med. 342, 145–153. doi: 10.1056/NEJM200001203420301
Henrichot, E., Juge-Aubry, C. E., Pernin, A., Pache, J. C., Velebit, V., Dayer, J. M., et al. (2005). Production of chemokines by perivascular adipose tissue: a role in the pathogenesis of atherosclerosis? Arterioscler. Thromb. Vasc. Biol. 25, 2594–2599. doi: 10.1161/01.ATV.0000188508.40052.35
Hilgers, R. H., Kundumani-Sridharan, V., Subramani, J., Chen, L. C., Cuello, L. G., Rusch, N. J., et al. (2017). Thioredoxin reverses age related hypertension by chronically improving vascular redox and restoring eNOS function. Sci. Transl. Med. 9:eaaf6094. doi: 10.1126/scitranslmed.aaf6094
Hong, F., Freeman, M. L., and Liebler, D. C. (2005). Identification of sensor cysteines in human Keap1 modified by the cancer chemopreventive agent sulforaphane. Chem. Res. Toxicol. 18, 1917–1926. doi: 10.1021/tx0502138
Imayama, I., Ulrich, C. M., Alfano, C. M., Wang, C., Xiao, L., Wener, M. H., et al. (2012). Effects of a caloric restriction weight loss diet and exercise on inflammatory biomarkers in overweight/obese postmenopausal women: a randomized controlled trial. Cancer Res. 72, 2314–2326. doi: 10.1158/0008-5472.CAN-11-3092
Imenshahidi, M., and Hosseinzadeh, H. (2016). Berberis vulgaris and berberine: an update review. Phytother. Res. 30, 1745–1764. doi: 10.1002/ptr.5693
Ishikura, K., Misu, H., Kumazaki, M., Takayama, H., Matsuzawa-Nagata, N., Tajima, N., et al. (2014). Selenoprotein P as a diabetes-associated hepatokine that impairs angiogenesis by inducing VEGF resistance in vascular endothelial cells. Diabetologia 57, 1968–1976. doi: 10.1007/s00125-014-3306-9
Jenkins, A. J., Welsh, P., and Petrie, J. R. (2018). Metformin, lipids and atherosclerosis prevention. Curr. Opin. Lipidol. 29, 346–353. doi: 10.1097/MOL.0000000000000532
Jiang, Z. Y., Lu, M. C., and You, Q. D. (2016). Discovery and development of Kelch-like ECH-associated protein 1. Nuclear factor erythroid 2-related factor 2 (KEAP1:NRF2) protein-protein interaction inhibitors: achievements, challenges, and future directions. J. Med. Chem. 59, 10837–10858. doi: 10.1021/acs.jmedchem.6b00586
Jones, D. P. (2006). Redefining oxidative stress. Antioxid. Redox Signal. 8, 1865–1879. doi: 10.1089/ars.2006.8.1865
Judkins, C. P., Diep, H., Broughton, B. R., Mast, A. E., Hooker, E. U., Miller, A. A., et al. (2010). Direct evidence of a role for Nox2 in superoxide production, reduced nitric oxide bioavailability, and early atherosclerotic plaque formation in ApoE-/- mice. Am. J. Physiol. Heart Circ. Physiol. 298, H24–H32. doi: 10.1152/ajpheart.00799.2009
Juurlink, B. H. (2012). Dietary Nrf2 activators inhibit atherogenic processes. Atherosclerosis 225, 29–33. doi: 10.1016/j.atherosclerosis.2012.08.032
Ketonen, J., Shi, J., Martonen, E., and Mervaala, E. (2010). Periadventitial adipose tissue promotes endothelial dysfunction via oxidative stress in diet-induced obese C57Bl/6 mice. Circ. J. 74, 1479–1487. doi: 10.1253/circj.CJ-09-0661
Kettle, A. J., Albrett, A. M., Chapman, A. L., Dickerhof, N., Forbes, L. V., Khalilova, I., et al. (2014). Measuring chlorine bleach in biology and medicine. Biochim. Biophys. Acta 1840, 781–793. doi: 10.1016/j.bbagen.2013.07.004
Kikuchi, N., Satoh, K., Kurosawa, R., Yaoita, N., Elias-Al-Mamun, M., Siddique, M. A. H., et al. (2018). Selenoprotein P promotes the development of pulmonary arterial hypertension. Circulation 138, 600–623. doi: 10.1161/CIRCULATIONAHA.117.033113
Kobayashi, M., and Yamamoto, M. (2006). Nrf2-Keap1 regulation of cellular defense mechanisms against electrophiles and reactive oxygen species. Adv. Enzyme Regul. 46, 113–140. doi: 10.1016/j.advenzreg.2006.01.007
Kozieł, R., Pircher, H., Kratochwil, M., Lener, B., Hermann, M., Dencher, N. A., et al. (2013). Mitochondrial respiratory chain complex I is inactivated by NADPH oxidase Nox4. Biochem. J. 452, 231–239. doi: 10.1042/BJ20121778
Kraakman, M. J., Lee, M. K., Al-Sharea, A., et al. (2017). Neutrophil-derived S100 calcium binding proteins A8/A9 promote reticulated thrombocytosis and atherogenesis in diabetes. J. Clin. Invest. 127, 2133–2147. doi: 10.1172/JCI92450
Kubala, L., Schmelzer, K. R., Klinke, A., Kolarova, H., Baldus, S., Hammock, B. D., et al. (2010). Modulation of arachidonic and linoleic acid metabolites in myeloperoxidase deficient mice during acute inflammation. Free Radic. Biol. Med. 48, 1311–1320. doi: 10.1016/j.freeradbiomed.2010.02.010
Kuhn, H., Banthiya, S., and van Leyen, K. (2015). Mammalian lipoxygenases and their biological relevance. Biochim. Biophys. Acta 1851, 308–330. doi: 10.1016/j.bbalip.2014.10.002
Landmesser, U., Cai, H., Dikalov, S., McCann, L., Hwang, J., Jo, H., et al. (2002). Role of p47(phox) in vascular oxidative stress and hypertension caused by angiotensin II. Hypertension 40, 511–515. doi: 10.1161/01.HYP.0000032100.23772.98
Landmesser, U., Spiekermann, S., Preuss, C., Sorrentino, S., Fischer, D., Manes, C., et al. (2007). Angiotensin II induces endothelial xanthine oxidase activation: role for endothelial dysfunction in patients with coronary disease. Arterioscler. Thromb. Vasc. Biol. 27, 943–948. doi: 10.1161/01.ATV.0000258415.32883.bf
Lassègue, B., and Clempus, R. E. (2003). Vascular NAD(P)H oxidases: specific features, expression, and regulation. Am. J. Physiol. Regul. Integr. Comp. Physiol. 285, R277–R297. doi: 10.1152/ajpregu.00758.2002
Lee, C. (2017). Collaborative power of Nrf2 and PPAR γ activators against metabolic and drug-induced oxidative injury. Oxid. Med. Cell. Longev. 2017:1378175. doi: 10.1155/2017/1378175
Lefkowitz, D. L., Mone, J., and Lefkowitz, S. S. (2010). Myeloperoxidase: the good, the bad, and the ugly. Curr. Immunol. Rev. 6, 123–129. doi: 10.1111/apha.12515
Lener, B., Kozieł, R., Pircher, H., Hütter, E., Greussing, R., Herndler-Brandstetter, D., et al. (2009). The NADPH oxidase Nox4 restricts the replicative lifespan of human endothelial cells. Biochem. J. 423, 363–374. doi: 10.1042/BJ20090666
Li, H., and Forstermann, U. (2014). Pharmacological prevention of eNOS uncoupling. Curr. Pharm. Des. 20, 3595–3606.
Li, H., Horke, S., and Förstermann, U. (2014). Vascular oxidative stress, nitric oxide and atherosclerosis. Atherosclerosis 237, 208–219. doi: 10.1016/j.atherosclerosis.2014.09.001
Lim, S., and Park, S. (2014). Role of vascular smooth muscle cell in the inflammation of atherosclerosis. BMB Rep. 47, 1–7. doi: 10.5483/BMBRep.2014.47.1.285
Liu, Z., and Khalil, R. A. (2018). Evolving mechanisms of vascular smooth muscle contraction highlight key targets in vascular disease. Biochem. Pharmacol. 153, 91–122. doi: 10.1016/j.bcp.2018.02.012
Lopez-Sanz, L., Bernal, S., Recio, C., Lazaro, I., Oguiza, A., Melgar, A., et al. (2018). SOCS1-targeted therapy ameliorates renal and vascular oxidative stress in diabetes via STAT1 and PI3K inhibition. Lab. Invest. 98, 1276–1290. doi: 10.1038/s41374-018-0043-6
Ma, L., Ma, S., He, H., Yang, D., Chen, X., Luo, Z., et al. (2010). Perivascular fat-mediated vascular dysfunction and remodeling through the AMPK/mTOR pathway in high-fat diet-induced obese rats. Hypertens. Res. 33, 446–453. doi: 10.1038/hr.2010.11
Madamanchi, N. R., Vendrov, A., and Runge, M. S. (2005). Oxidative stress and vascular disease. Arterioscler. Thromb. Vasc. Biol. 25, 29–38. doi: 10.1161/01.ATV.0000150649.39934.13
Majesky, M. W. (2015). Adventitia and perivascular cells. Arterioscler. Thromb. Vasc. Biol. 35, e31–e35. doi: 10.1161/ATVBAHA.115.306088
Marchesi, C., Ebrahimian, T., Angulo, O., Paradis, P., and Schiffrin, E. L. (2009). Endothelial nitric oxide synthase uncoupling and perivascular adipose oxidative stress and inflammation contribute to vascular dysfunction in arodent model of metabolic syndrome. Hypertension 54, 1384–1392. doi: 10.1161/HYPERTENSIONAHA.109.138305
Martínez-Revelles, S., García-Redondo, A. B., Avendaño, M. S., Varona, S., Palao, T., Orriols, M., et al. (2017). Lysyl oxidase induces vascular oxidative stress and contributes to arterial stiffness and abnormal elastin structure in hypertension: role of p38MAPK. Antioxid. Redox Signal. 27, 379–397. doi: 10.1089/ars.2016.6642
Meijer, R. I., Serne, E. H., Smulders, Y. M., van Hinsbergh, V. W. M., Yudkin, J. S., and Eringa, E. C. (2011). Perivascular adipose tissue and its role in type 2 diabetes and cardiovascular disease. Curr. Diab. Rep. 11, 211–217. doi: 10.1007/s11892-011-0186-y
Milagros Rocha, M., and Victor, V. M. (2007). Targeting antioxidants to mitochondria and cardiovascular diseases: the effects of mitoquinone. Med. Sci. Monit. 13, RA132–RA145.
Misu, H., Takamura, T., Takayama, H., Hayashi, H., Matsuzawa-Nagata, N., Kurita, S., et al. (2010). A liver-derived secretory protein, selenoprotein P, causes insulin resistance. Cell Metab. 12, 483–495. doi: 10.1016/j.cmet.2010.09.015
Mita, Y., Nakayama, K., Inari, S., Nishito, Y., Yoshioka, Y., Sakai, N., et al. (2017). Selenoprotein P-neutralizing antibodies improve insulin secretion and glucose sensitivity in type 2 diabetes mouse models. Nat. Commun. 8:1658. doi: 10.1038/s41467-017-01863-z
Molica, F., Morel, S., Kwak, B. R., Rohner-Jeanrenaud, F., and Steffens, S. (2015). Adipokines at the crossroad between obesity and cardiovascular disease. Thromb. Haemost. 113, 553–566. doi: 10.1160/TH14-06-0513
Montezano, A. C., De Lucca Camargo, L., Persson, P., Rios, F. J., Harvey, A. P., Anagnostopoulou, A., et al. (2018). NADPH oxidase 5 is a pro-contractile nox isoform and a point of cross-talk for calcium and redox signaling-implications in vascular function. J. Am. Heart Assoc. 7:e009388. doi: 10.1161/JAHA.118.009388
Munzel, T., and Gori, T. (2009). Nebivolol: the somewhat-different beta adrenergic receptor blocker. J. Am. Coll. Cardiol. 54, 1491–1499. doi: 10.1016/j.jacc.2009.05.066
Murphy, E., Ardehali, H., Balaban, R. S., DiLisa, F., Dorn, G. W. II, Kitsis, R. N., et al. (2016). Mitochondria function, biology, and role in disease. Circ. Res. 118, 1960–1991. doi: 10.1161/RES.0000000000000104
Murphy, M. P., and Smith, R. A. (2007). Targeting antioxidants to mitochondria by conjugation to lipophilic cations. Annu. Rev. Pharmacol. Toxicol. 47, 629–656. doi: 10.1146/annurev.pharmtox.47.120505.105110
Nicholls, S. L., and Hazen, S. L. (2009). Myeloperoxidase, modified lipoproteins, and atherogenesis. J. Lipid Res. 50, S346–S351. doi: 10.1194/jlr.R800086-JLR200
Niture, S. K., Khatri, R., and Jaiswal, A. K. (2014). Regulation of Nrf2-an update. Free Radic. Biol. Med. 66, 36–44. doi: 10.1016/j.freeradbiomed.2013.02.008
Nomura, J., Busso, N., Ives, A., Matsui, C., Tsujimoto, S., Shirakura, T., et al. (2014). Xanthine oxidase inhibition by febuxostat attenuates experimental atherosclerosis in mice. Sci. Rep. 4:4554. doi: 10.1038/srep04554
Norlander, A. E., Madhur, M. S., and Harrison, D. G. (2018). The immunology of hypertension. J. Exp. Med. 215, 21–33. doi: 10.1084/jem.20171773
Omar, A., Chatterjee, T. K., Tang, Y., Hui, D. Y., and Weintraub, N. L. (2014). Proinflammatory phenotype of perivascular adipocytes. Arterioscler. Thromb. Vasc. Biol. 34, 1631–1636. doi: 10.1161/ATVBAHA.114.303030
Padilla, J., Vieira-Potter, V. J., Jia, G., and Sowers, J. R. (2015). Role of perivascular adipose tissue on vascular reactive oxygen species in type 2 diabetes: a give-and-take relationship. Diabetes 64, 1904–1906. doi: 10.2337/db15-0096
Payne, G. A., Borbouse, L., Kumar, S., Neeb, Z., Alloosh, M., Sturek, M., et al. (2010). Epicardial perivascular adipose-derived leptin exacerbates coronary endothelial dysfunction in metabolic syndrome via a protein kinase C-beta pathway. Arterioscler. Thromb. Vasc. Biol. 30, 1711–1717. doi: 10.1161/ATVBAHA.110.210070
Pereira, A., Fernandes, R., Crisóstomo, J., Seiça, R. M., and Sena, C. M. (2017). The Sulforaphane and pyridoxamine supplementation normalize endothelial dysfunction associated with type 2 diabetes. Sci. Rep. 7:14357. doi: 10.1038/s41598-017-14733-x
Pitanga, T. N., de Aragão França, L., Rocha, V. C., Meirelles, T., Borges, V. M., Gonçalves, M. S., et al. (2014). Neutrophil-derived microparticles induce myeloperoxidase-mediated damage of vascular endothelial cells. BMC Cell Biol. 15:21. doi: 10.1186/1471-2121-15-21
Rådmark, O., Werz, O., Steinhilber, D., and Samuelsson, B. (2015). 5-Lipoxygenase, a key enzyme for leukotriene biosynthesis in health and disease. Biochim. Biophys. Acta 1851, 331–339. doi: 10.1016/j.bbalip.2014.08.012
Rodriguez-Porcel, M., Chade, A. R., and Miller, J. D. (2017). Studies on Atherosclerosis. Oxidative Stress in Applied Basic Research and Clinical Practice, 1st Edn. Berlin: Springer. doi: 10.1007/978-1-4899-7693-2
Sabatine, M. S., Leiter, L. A., Wiviott, S. D., Giugliano, R. P., Deedwania, P., De Ferrari, G. M., et al. (2017). Cardiovascular safety and efficacy of the PCSK9 inhibitor evolocumab in patients with and without diabetes and the effect of evolocumab on glycaemia and risk of new-onset diabetes: a prespecified analysis of the FOURIER randomised controlled trial. Lancet Diabetes Endocrinol. 5, 941–950. doi: 10.1016/S2213-8587(17)30313-3
Sacks, H. S., and Fain, J. N. (2011). Human epicardial fat: what is new and what is missing? Clin. Exp. Pharmacol. Physiol. 38, 879–887. doi: 10.1111/j.1440-1681.2011.05601.x
Salgado-Somoza, A., Teijeira-Fernandez, E., Fernandez, A. L., Gonzalez-Juanatey, J. R., and Eiras, S. (2010). Proteomic analysis of epicardial and subcutaneous adipose tissue reveals differences in proteins involved in oxidative stress. Am. J. Physiol. 299, H202–H209. doi: 10.1152/ajpheart.00120.2010
Santilli, F., D’Ardes, D., and Davì, G. (2015). Oxidative stress in chronic vascular disease: from prediction to prevention. Vascul. Pharmacol. 74, 23–37. doi: 10.1016/j.vph.2015.09.003
Sayre, L. M., Smith, M. A., and Perry, G. (2001). Chemistry and biochemistry of oxidative stress in neurodegenerative disease. Curr. Med. Chem. 8, 721–738. doi: 10.2174/0929867013372922
Schieber, M., and Chandel, N. S. (2014). ROS function in redox signaling and oxidative stress. Curr. Biol. 24, R453–R462. doi: 10.1016/j.cub.2014.03.034
Schleicher, E., and Friess, U. (2007). Oxidative stress, AGE, and atherosclerosis. Kidney Int. 72(Suppl. 106), S17–S26. doi: 10.1038/sj.ki.5002382
Schröder, K., Vecchione, C., Jung, O., Schreiber, J. G., Shiri-Sverdlov, R., van Gorp, P. J., et al. (2006). Xanthine oxidase inhibitor tungsten prevents the development of atherosclerosis in ApoE knockout mice fed a Western-type diet. Free Radic. Biol. Med. 41, 1353–1360. doi: 10.1016/j.freeradbiomed.2006.03.026
Sena, C. M., Louro, T., Matafome, P., Nunes, E., Monteiro, P., and Seiça, R. (2009). Antioxidant and vascular effects of gliclazide in type 2 diabetic rats fed high-fat diet. Physiol. Res. 58, 203–209.
Sena, C. M., Matafome, P., Louro, T., Nunes, E., Fernandes, R., and Seiça, R. M. (2011). Metformin restores endothelial function in aorta of diabetic rats. Br. J. Pharmacol. 163, 424–437. doi: 10.1111/j.1476-5381.2011.01230.x
Sena, C. M., Nunes, E., Louro, T., Proença, T., Fernandes, R., Boarder, M. R., et al. (2008). Effects of alpha-lipoic acid on endothelial function in aged diabetic and high-fat fed rats. Br. J. Pharmacol. 153, 894–906. doi: 10.1038/sj.bjp.0707474
Sena, C. M., Pereira, A., Fernandes, R., Letra, L., and Seiça, R. M. (2017). Adiponectin improves endothelial function in mesenteric arteries of rats fed a high-fat diet: role of perivascular adipose tissue. Br. J. Pharmacol. 174, 3514–3526. doi: 10.1111/bph.13756
Sena, C. M., Pereira, A. M., and Seiça, R. (2013). Endothelial dysfunction- A major mediator of diabetic vascular disease. Biochim. Biophys. Acta 1832, 2216–2231. doi: 10.1016/j.bbadis.2013.08.006
Serazin, V., Dos Santos, E., Morot, M., and Giudicelli, Y. (2004). Human adipose angiotensinogen gene expression and secretion are stimulated by cyclic AMP via increased DNA cyclic AMP responsive element binding activity. Endocrine 25, 97–104. doi: 10.1385/ENDO:25:2:097
Sies, H. (2017). Hydrogen peroxide as a central redox signaling molecule in physiological oxidative stress: oxidative eustress. Redox Biol. 11, 613–619. doi: 10.1016/j.redox.2016.12.035
Smith, R. A., Adlam, V. J., Blaikie, F. H., Manas, A. R., Porteous, C. M., James, A. M., et al. (2008). Mitochondria-targeted antioxidants in the treatment of disease. Ann. N. Y. Acad. Sci. 1147, 105–111. doi: 10.1196/annals.1427.003
Spiekermann, S., Landmesser, U., Dikalov, S., Bredt, M., Gamez, G., Tatge, H., et al. (2003). Electron spin resonance characterization of vascular xanthine and NAD(P) H oxidase activity in patients with coronary artery disease: relation to endothelium-dependent vasodilation. Circulation 107, 1383–1389. doi: 10.1161/01.CIR.0000056762.69302.46
Stachowicz, A., Olszanecki, R., Suski, M., Wiśniewska, A., Totoń-Żurańska, J., Madej, J., et al. (2014). Mitochondrial aldehyde dehydrogenase activation by Alda-1 inhibits atherosclerosis and attenuates hepatic steatosis in apolipoprotein E-knockout mice. J. Am. Heart Assoc. 3:e001329. doi: 10.1161/JAHA.114.001329
Steinhubl, S. R. (2008). Why have antioxidants failed in clinical trials? Am. J. Cardiol. 101, 14D–19D. doi: 10.1016/j.amjcard.2008.02.003
Sugiura, T., Dohi, Y., Yamashita, S., Hirowatari, Y., Fujii, S., and Ohte, N. (2016). Serotonin in peripheral blood reflects oxidative stress and plays a crucial role in atherosclerosis: novel insights toward holistic anti-atherothrombotic strategy. Atherosclerosis 246, 157–160. doi: 10.1016/j.atherosclerosis.2016.01.015
Suzuki, T., and Yamamoto, M. (2017). Stress-sensing mechanisms and the physiological roles of the Keap1-Nrf2 system during cellular stress. J. Biol. Chem. 292, 16817–16824. doi: 10.1074/jbc.R117.800169
Szeto, H. H. (2006). Cell-permeable, mitochondrial-targeted, peptide antioxidants. AAPS J. 8, E277–E283. doi: 10.1007/BF02854898
ten Freyhaus, H., Huntgeburth, M., Wingler, K., Schnitker, J., Bäumer, A. T., Vantler, M., et al. (2006). Novel Nox inhibitor VAS2870 attenuates PDGF-dependent smooth muscle cell chemotaxis, but not proliferation. Cardiovasc. Res. 71, 331–341. doi: 10.1016/j.cardiores.2006.01.022
Tsioufis, C., Dimitriadis, K., Selima, M., Thomopoulos, C., Mihas, C., Skiadas, I., et al. (2007). Low-grade inflammation and hypoadiponectinaemia have an additive detrimental effect on aortic stiffness in essential hypertensive patients. Eur. Heart J. 28, 1162–1169. doi: 10.1093/eurheartj/ehm089
Ungvari, Z., Bailey-Downs, L., Sosnowska, D., Gautam, T., Koncz, P., Losonczy, G., et al. (2011). Vascular oxidative stress in aging: a homeostatic failure due to dysregulation of NRF2-mediated antioxidant response. Am. J. Physiol. Heart Circ. Physiol. 301, H363–H372. doi: 10.1152/ajpheart.01134.2010
Wang, W., Wu, Q. H., Sui, Y., Wang, Y., and Qiu, X. (2017). Rutin protects endothelial dysfunction by disturbing Nox4 and ROS-sensitive NLRP3 inflammasome. Biomed. Pharmacother. 86, 32–40. doi: 10.1016/j.biopha.2016.11.134
Wang, Y., Li, L., Zhao, W., Dou, Y., An, H., Tao, H., et al. (2018). Targeted therapy of atherosclerosis by a broad-spectrum reactive oxygen species scavenging nanoparticle with intrinsic anti-inflammatory activity. ACS Nano 12, 8943–8960. doi: 10.1021/acsnano.8b02037
Weber, C., and Noels, H. (2011). Atherosclerosis: current pathogenesis and therapeutic options. Nat. Med. 17, 1410–1422. doi: 10.1038/nm.2538
Wimalasundera, R., Fexby, S., Regan, L., Thom, S., and Hughes, A. (2003). Effect of tumor necrosis factor-αand interleukin-1βon endothelium dependent relaxation in rat mesenteric resistance arteries in vitro. Br. J. Pharmacol. 138, 1285–1294. doi: 10.1038/sj.bjp.0705168
Wu, J., Saleh, M. A., Kirabo, A., Itani, H. A., Montaniel, K. R., Xiao, L., et al. (2016). Immune activation caused by vascular oxidation promotes fibrosis and hypertension. J. Clin. Invest. 126, 50–67. doi: 10.1172/JCI80761
Xia, N., Daiber, A., Förstermann, U., and Li, H. (2017). Antioxidant effects of resveratrol in the cardiovascular system. Br. J. Pharmacol. 174, 1633–1646. doi: 10.1111/bph.13492
Xia, N., Horke, S., Habermeier, A., Closs, E. I., Reifenberg, G., Gericke, A., et al. (2016). Uncoupling of endothelial nitric oxide synthase in perivascular adipose tissue of diet-induced obese mice. Arterioscler. Thromb. Vasc. Biol. 36, 78–85. doi: 10.1161/ATVBAHA.115.306263
Yang, M. Y., Wang, Y. B., Han, B., Yang, B., Qiang, Y. W., Zhang, Y., et al. (2018). Activation of aldehyde dehydrogenase 2 slows down the progression of atherosclerosis via attenuation of ER stress and apoptosis in smooth muscle cells. Acta Pharmacol. Sin. 39, 48–58. doi: 10.1038/aps.2017.81
Yu, E. P., Mercer, J. A., and Bennett, M. C. (2012). Mitochondria in vascular disease. Cardiovasc. Res. 95, 173–182. doi: 10.1093/cvr/cvs111
Zhang, R., Brennan, M. L., Shen, Z., MacPherson, J. C., Schmitt, D., Molenda, C. E., et al. (2002). Myeloperoxidase functions as a major enzymatic catalyst for initiation of lipid peroxidation at sites of inflammation. J. Biol. Chem. 277, 46116–46122. doi: 10.1074/jbc.M209124200
Zhou, N., Lee, J. J., Stoll, S., Ma, B., Costa, K. D., and Qiu, H. (2017). Rho kinase regulates aortic vascular smooth muscle cell stiffness via actin/SRF/myocardin in hypertension. Cell. Physiol. Biochem. 44, 701–715. doi: 10.1159/000485284
Keywords: oxidative stress, vasculature, reactive oxygen species, therapies, antioxidants
Citation: Sena CM, Leandro A, Azul L, Seiça R and Perry G (2018) Vascular Oxidative Stress: Impact and Therapeutic Approaches. Front. Physiol. 9:1668. doi: 10.3389/fphys.2018.01668
Received: 24 May 2018; Accepted: 06 November 2018;
Published: 04 December 2018.
Edited by:
Michael A. Hill, University of Missouri, United StatesReviewed by:
James Sowers, University of Missouri, United StatesRoy Sutliff, Emory University, United States
Copyright © 2018 Sena, Leandro, Azul, Seiça and Perry. This is an open-access article distributed under the terms of the Creative Commons Attribution License (CC BY). The use, distribution or reproduction in other forums is permitted, provided the original author(s) and the copyright owner(s) are credited and that the original publication in this journal is cited, in accordance with accepted academic practice. No use, distribution or reproduction is permitted which does not comply with these terms.
*Correspondence: Cristina M. Sena, csena@ci.uc.pt; cmts33@sapo.pt