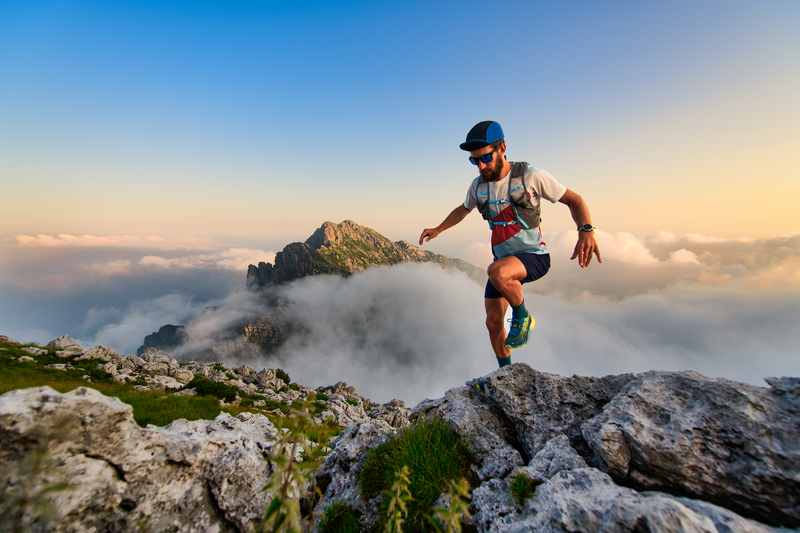
95% of researchers rate our articles as excellent or good
Learn more about the work of our research integrity team to safeguard the quality of each article we publish.
Find out more
SYSTEMATIC REVIEW article
Front. Psychiatry , 20 November 2020
Sec. Molecular Psychiatry
Volume 11 - 2020 | https://doi.org/10.3389/fpsyt.2020.561998
Autism spectrum disorder (ASD) is a pervasive neurodevelopmental disorder with limited available treatments and diverse causes. In ASD patients, numerous researches demonstrated various alterations in inflammation/immune, oxidative stress, and mitochondrial dysfunction, and these alterations could be regulated by Nrf2. Hence, we aimed to systematically review the current evidence about the effects of Nrf2 activator supplementation on ASD objects from in vitro studies, animal studies, and clinical studies. Relevant articles were retrieved through searching for the Cochrane Library, PubMed, Web of Science, Scope, Embase, and CNKI databases (through September 23, 2020). Ultimately, we identified 22 preclinical studies, one cell culture study, and seven clinical studies, covering a total of five Nrf2 activators. For each Nrf2 activator, we focused on its definition, potential therapeutic mechanisms, latest research progress, research limitations, and future development directions. Our systematic review provided suggestive evidence that Nrf2 activators have a potentially beneficial role in improving autism-like behaviors and abnormal molecular alterations through oxidant stress, inflammation, and mitochondrial dysfunction. These dietary phytochemicals are considered to be relatively safer and effective for ASD treatment. However, there are few clinical studies to support the Nrf2 activators as dietary phytochemicals in ASD, even though several preclinical studies. Therefore, caution should be warranted in attempting to extrapolate their effects in human studies, and better design and more rigorous research are required before they can be determined as a therapeutic option.
Autism spectrum disorder (ASD) is a pervasive neurodevelopmental disorder characterized by impaired communication and social interaction, as well as repetitive/stereotyped behaviors (1, 2). The world health organization (WHO) reported in 2017 that one in 160 children worldwide has ASD (3), currently affected 1 in 54 children in the United States (4, 5), predominantly males (6). ASDs greatly limit the capacity of individuals to perform daily activities and participate in society, which imposes a significant emotional and economic burden on affected individuals and their families (3). Many factors may cause ASD, however, no definite pathogenesis have been established yet (5). There is currently no cure for ASD. Current drugs can only be used to control symptoms related to ASD, including anxiety, hyperactivity, epilepsy, obsessive behavior, and gastrointestinal problems (7).
Admittedly, numerous publications have indicated that the pathogenesis of ASD was affected by immune dysregulation/inflammation, oxidative stress, and mitochondrial dysfunction (8–10). Specifically, compared with normal children, children with ASD showed abnormal oxidative stress (i.e., glutathione (GSH) and the major intracellular antioxidant decreased, oxidized glutathione (GSSG) increased, and GSH/GSSG redox ratios decreased) in the peripheral and brain tissues (10, 11). Furthermore, the activity of several enzymes related to GSH synthesis and metabolic pathways in the brain tissue of ASD patients is reduced (11). Increasing researches have speculated that there may be an interaction between oxidative stress and mitochondrial function, which together affected the pathogenesis of ASD, although this remains somewhat controversial (11). Interestingly, the mitochondrial electron transport chain is not only a source of free radicals but also a target of free radicals. Consequently, oxidative stress may damage mitochondrial function, conversely, the abnormal mitochondrial function may cause further oxidative stress (12, 13). Moreover, biomarkers of mitochondrial dysfunction are associated with autistic behavior or severity (9). On the whole, mitochondrial dysfunction may contribute to ASD pathogenesis. Indeed, researchers found mitochondria dysfunction in different types of ASD objects, from ASD animal models, to cell lines (e.g., lymphocytes and granulocytes) derived from children with ASD (14, 15), to brain tissues of ASD patients (9, 16, 17). Finally, multiple studies evidenced inflammation/immune dysregulation in individuals with ASD both within the brain and the periphery. Specifically, researchers identified abnormal alterations in microglial cell activation, atypical pro-inflammatory cytokine production, immune-related gene expression, and other inflammatory biomarkers, where occurs in the central neural system (CNS) and peripheral immune system (18). Above all, those alterations may contribute to the pathophysiology of ASD.
Strikingly, existing evidence has indicated that Nrf2 plays an important role in immune dysregulation/inflammation, oxidative stress, and mitochondrial dysfunction. Inhibition of Nrf2 activity in human brains can increase the risk of chronic diseases such as Parkinson's disease (PD), Alzheimer's disease (AD), and amyotrophic lateral sclerosis (ALS) (19). Nrf2 is a transcription factor that regulates cellular oxidative stress responses. Under physiological conditions, Nrf2 is coupled with Kelch-like ECH-associated protein 1 (Keap1) and exists in the cytoplasm in an inactive form, which is then rapidly degraded by the ubiquitin-proteasome system, thereby maintaining stable expression of Nrf2 (20). Under pathological conditions, Nrf2 dissociates from Keap1, then translocated into the nucleus. In the nucleus, Nrf2 will bind to Maf protein to form a heterodimer, which interacts with antioxidant reaction elements (ARE) to regulate the expression of antioxidant proteins and phase II enzymes (21) (Figure 1).
Figure 1. The mechanism of Nrf2 activation for ASD treatment by regulating oxidative stress, inflammation, and mitochondrial function by activating the Nrf2-ARE pathway (see main text). Besides, there is an interaction between the Nrf2/ARE pathway and the NF-κB pathway. On the one hand, free Keap1 prevents degradation of IkBα, thereby inhibiting NF-κB pathway. On the other hand, the p65 subunit of NF-κB also inhibits Keap1 from interfering with nuclear transcription of Nrf2. Dietary phytochemicals like sulforaphane (SFN), resveratrol (RSV), curcumin (CUR), naringenin (NGN), and agmatine (AGM) activated Nrf2/ARE pathway by interacting with Keap1.
Nuclear factor kappa-light-chain-enhancer of activated B cells (NF-κB) is a key regulator of inflammation (22). Under the physiological condition, NF-κB is coupled with an inhibitor of nuclear factor κ-B kinase subunit beta (IKK-β) protein and exits in the cytoplasm (23). Under pathological conditions (e.g., enhanced oxidative stress), IKKβ was activated, causing phosphorylation of NF-kappa-B inhibitor alpha (IkBα). Subsequently, NF-κB will migrate to the nucleus and bind to its region, which leads to transcription of pro-inflammatory cytokines such as IL-1β, IL-6, IL-12, and TNF-α (23, 24). Interestingly, there is an interaction between the Nrf2/ARE pathway and the NF-κB pathway. On the one hand, free Keap1 prevents degradation of IkBα, thereby inhibiting NF-κB pathway. On the other hand, the p65 subunit of NF-κB also inhibits Keap1 from interfering with the nuclear transcription of Nrf2 (24, 25) (Figure 1). Compared with normal animals, Nrf2-deficient mice have more pronounced effects on lipopolysaccharide (LPS)-induced microglial activation and inflammatory response, with lower levels of IL-1β, IL-6, IL-12, and TNF-α in the brain (23). Accordingly, the functional Nrf2 system is very important for regulating neuroinflammation and oxidative stress in the brain (19).
Moreover, increasing evidence indicated that Nrf2 may regulate mitochondrial function and metabolism. Studies have confirmed that Nrf2 deficiency can lead to mitochondrial depolarization, reduced ATP levels, and impaired respiratory function. On the contrary, the above negative phenomena can be reversed by activating Nrf2 genes (26, 27) (Figure 1). Therefore, Nrf2 can directly regulate cellular energy metabolism by regulating the availability of mitochondrial respiratory substrates.
Accordingly, Nrf2 activation may reverse the pathophysiological and behavioral abnormalities associated with ASD through the above-mentioned mechanisms. Interestingly, Nrf2 activator therapy for ASD has attracted widespread clinical interest. Several known Nrf2 natural activators (e.g., curcumin, sulforaphane, resveratrol, and naringenin) played an important role in regulating Nrf2 mechanisms (11, 28, 29). Thus, we systematically reviewed current evidence on the effects of Nrf2 activators administration on ASD objects from in vitro studies, animal studies, and clinical studies, aiming to elucidate molecular mechanisms related to the etiology of ASD and to open up a new approach to prevent and/or treat ASD.
This systematic review was conducted and reported by the Preferred Reporting Items for Systematic Reviews and Meta-Analyses (PRISMA) guidelines (30).
We retrieved relevant articles in the Cochrane Library, PubMed, Web of Science, Scope, Embase, and CNKI up to September 23, 2020. We employed keywords combined with medical subject headings (MeSH) search, using the following terms: (Autism OR Autism Spectrum Disorder OR autistic OR autism disorder OR ASD OR Asperger Syndrome OR Pervasive Developmental Disorder) AND (Nuclear factor erythroid 2 related factors 2 activators OR NFE2 Related Factor 2 activator OR Nrf2 activator OR sulforaphane OR SFN OR broccoli OR Brussel sprouts OR watercress OR resveratrol OR curcumin OR naringenin OR agmatine). Additionally, we screened two registered clinical trial websites (i.e., clinicaltrials.gov and isrctn.com). Moreover, we manually searched the reference lists of the relevant articles to search for other articles.
For the records, the following criteria were applied: (1) original research published in English and Chinese, (2) identifying data regarding Nrf2 activators therapy in animal or cell culture models of autism, or patients with ASD. Reviews, editorials, and commentaries were excluded. We also excluded meeting abstracts unless it reported available outcomes.
After removing duplicates, we filtered the titles and abstracts, and then the full texts. The following data was synthesized from each article: (a) literature information (author, publication year, country), (b) therapy type, (c) study design, (d) intervention details, (e) main findings, and (f) adverse effect. Given the exploratory status in this field, maybe it is justified not to report the methodological quality of these studies.
We initially yielded 453 articles, 30 of which were ultimately eligible for the systematic review (Figure 2). Of all 30 articles, 7 represented human clinical trials, 22 represented animal studies, and one represented cell culture study. Of these, five Nrf2 activators related to ASD treatment were studied (i.e., sulforaphane, resveratrol, naringenin, curcumin, and agmatine), varying different intervention dosage and duration. Overall, these studies provided evidence that various types of Nrf2 activators may have potential beneficial and protective effects on autism-like behaviors and abnormal molecular alterations, although with divergence. Amongst 22 animal studies, fifteen and five studies were performed in rat and mice models, respectively. Animal models of ASD include pharmacologic model and genetically modified animal models, such as BTBR T~(+)tf/J (BTBR) model (n = 6), prenatal exposure to valproic acid (VPA) (n = 12), intracerebroventricular administration of propanoic acid (PPA) (n = 3), and prenatal exposure to progestins (n = 1). Of the 7 clinical studies, four were randomized controlled trials (RCTs), two were open-label trials, and one was a follow-up case series. Participants were mostly male aged 3–30 years old. The key characteristics of the included studies were shown in Tables 1, 2.
Table 1. Behavioral and molecular effects of Nrf2 activators in animal and cell culture models of ASD.
Several Nrf2 activators showed improvements in autism-like behaviors and abnormal molecular alterations in clinical and preclinical studies.
Resveratrol (3,5,4′-Trihydroxystilbene, RSV) is a natural polyphenolic stilbenoid present in berries, nuts, red wine, and grapes. It is produced when attacked by bacteria, fungi, ultraviolet radiation, and chemical substances (60). As an agonist of Nrf2, RSV could increase the level of Nrf2 and induce its translocation into the nucleus, thereby activating genes with AREs (23), which ultimately increased the expression of various antioxidant enzymes and inflammatory cytokines. Recently, studies have shown that RSV has a neuroprotective role in various diseases, including neurodegeneration diseases, autoimmune disorders, heart diseases, and cancer (55, 61).
Thirteen studies investigated the effects of RSV treatment on animal models of ASD (Table 1). Prenatal RSV treatment [at doses of 3.6 mg/kg for 13 days, intraperitoneal (i.p.)] had different effects on VPA-induced autistic behaviors in six studies. On the one hand, four studies demonstrated that prenatal RSV treatment could reverse VPA-induced social deficits (31, 36, 38, 43). Moreover, RSV also prevented sensory deficits triggered by VPA (34). However, prenatal RSV can prevent some but not all abnormalities induced by VPA (34), which RSV counteracted the deficit in reduced total reciprocal social interaction but not in the repetitive behavior in the study by Hirsch et al. (36). On the contrary, Fontes-Dutra et al. (33) demonstrated that prenatal RSV treatment did not affect the deficits of empathy-like behavior in VPA animals. Other than prenatal RSV treatment, Hidema et al. demonstrated that postnatal RSV treatment (30 mg/kg, a single administration, i.p.) could improve social impairments induced by VPA (32). Besides, prenatal RSV treatment (20 or 40 mg/kg for 7 days, i.p.) significantly reduced repetitive behavior in BTBR mice, and the high-dose group was more significant (39). In the PPA model, RSV supplementation (5, 10, 15 mg/kg for 28 days, peroral) significantly and dose-dependently improved core symptoms of ASD (i.e., repetitive behavior and social interaction) as well as counteracting other abnormities (i.e., hyperactivity, anxiety, spatial learning, memory, and depression-like behaviors) (40). As for models of prenatal/postnatal exposure to progestins, oral administration of either postnatal or prenatal RSV treatment (at the dose of 20 mg/kg for 28 days) ameliorated autism-like behaviors (41). Accordingly, the timing, method, and dosage of RSV administration may have different effects on the autism-like behaviors induced by the same or different ASD models.
RSV prevented or ameliorated abnormalities in animal models of ASD through various molecular mechanisms, mainly anti-oxidative stress, and anti-inflammatory functions. Four studies investigated the effect of RSV treatment in the BTBR model, which was mediated by an anti-inflammatory mechanism (35, 37, 39, 42). Bakheet et al. (39, 42) elucidated that RSV treatment (20 and 40 mg/kg 7 days, i.p.) corrected dysfunction of inflammation-related transcription factor signaling. On the one hand, RSV treatment downregulated the mRNA expression of chemokine receptors (CCR) (i.e., CCR3, CCR5, CCR7, CCR9, CXCR3, and CXCR5) in the brain and spleen tissues, and decreased the levels of chemokine receptor in CD4+ T cells and spleen (42). On the other hand, RSV treatment ameliorated dysregulation of T helper (Th)1, Th2, Th17, and T regulatory cell-associated transcription factors in BTBR mice (39). By using flow cytometric, Real-Time PCR, and western blot analysis, we found that RSV decreased both mRNA and protein expression of T-bet, GATA-3, RORγt, and IL-17A, and upregulated Foxp3 expression in the spleen and brain tissues (39). Similarly, Ahmad et al. suggested that RSV treatment improved neuroimmune dysregulation by inhibiting pro-inflammatory mediators, TLRs/NF-κB transcription factor signaling, and JAK-1/STAT3 signaling in BTBR mice (35, 37). Specifically, RSV treatment not only significantly reduced the levels of CD4+TLR2+, CD4+TLR3+, CD4+TLR4+, CD4+NF-κB+, and CD4+iNOS+ in splenocytes (35), but also reduced the levels of TLR2, TLR3, TLR4, NF-κB, iNOS, and COX-2 mRNA and protein expression in brain tissues (35). Moreover, RSV significantly decreased IL-6+, TNF-α+, IFN-γ+, and Signal Transducer and Activator of Transcription (STAT)3+ in CD4+ spleen cells and decreased IL-6, TNF-α, IFN-γ, JAK1, and STAT3 mRNA expression levels as well as their protein expression level. In the PPA model, RSV supplementation (5, 10, 15 mg/kg) dose-dependently attenuated inflammation and oxidative stress (40). On the one hand, RSV reduced oxidative stress markers (lipid hydroperoxide and nitrite) and increased the endogenous antioxidants (GSH, superoxide dismutase, and catalase) in the brain. On the other hand, RSV normalized matrix metalloproteinases-9 levels associated with neuroinflammation and improved the activity of brain mitochondrial complex enzymes (40). MicroRNA (miRNA) is a small non-coding RNA regulating gene expression and associated with biological processes affected by neurodevelopmental disorders. Interestingly, Hirsch et al. demonstrated that RSV downregulated miR134-5p expression in VPA rats (36). Similarly, Fontes-Dutra et al. demonstrated that VPA altered the localization of PV+-neurons in the primary sensory cortex and amygdala, and reduced the level of gephyrin in the primary somatosensory area (34). However, prenatal RSV treatment prevented all the alterations induced by VPA (34). Prenatal exposure to progestins reduced estrogen receptor (ERβ) expression in the amygdala in the offspring, however, it can be reversed by postnatal or prenatal RSV treatment (41). Moreover, further research indicated that RSV activated ERβ and its target genes by demethylating the DNA and histones on the ERβ promoter, and then minimized oxidative stress, mitochondria dysfunction, and abnormal lipid metabolism in the brain (41). In conclusion, RSV has a potential impact on the prevention and/or treatment of ASDs.
Clinical research on RSV was still in its infancy. We only retrieved one RCT that assessed the potential therapeutic effects of RSV as an adjunct to risperidone on the irritability of ASD patients (55). Except for the symptom of hyperactivity/non-compliance, adjunctive treatment with RSV had no significant effect on most symptoms of ASD (i.e., irritability, lethargy/social withdrawal, stereotypic behavior, and inappropriate speech). Notably, ASD patients often comorbid ADHD with a prevalence of 26–70% (62). So, RSV plus risperidone treatment may play a beneficial role in ASD patients who comorbid ADHD. Thus, further RCTs could be devoted to studying the effect of RSV as monotherapy on certain subgroups of ASD. Lastly, this clinical study demonstrated no significant difference in the number and severity of adverse events between the two groups (55). Taken together, our findings revealed an important preventive role of RSV, ranging from autism-like behaviors to molecular alterations related to ASD. Further exploration of the therapeutic mechanisms of RSV could provide clues to reveal the biomarkers and etiology of ASD.
Sulforaphane (1-isothiocyanate-4-methylsulfinyl butane, SFN) is an active compound extracted from cruciferous vegetables like broccoli, brussels sprouts, and cabbages (62, 63). Pharmacokinetic studies revealed that SFN concentration in human plasma increased rapidly after broccoli supplementation, reaching a peak several hours later (64). After being absorbed, SFN can easily cross the blood-brain barrier and quickly reach the central neuron system, exerting its neuroprotective effects (65, 66). Interestingly, SFN has a unique ability in Nrf2 activation, which enhances the expression of heme oxygenase-1 (HO-1) and nicotinamide adenine dinucleotide phosphate quinone oxidoreductase 1 (NQO1) (59, 67), thereby protecting neurons from 6-hydroxydopamine (6-OHDA), methyl-4-phenyl-1,2,3,6-tetrahydropyridine (MPTP) (68), amyloid β (Aβ) (69), and pro-inflammatory cytokines (70, 71). Moreover, in Nrf2-deficient mice, SFN has no significant effect on the pro-inflammatory response, indicating SFN might be an Nrf2-dependent anti-inflammatory agent (72, 73). Therefore, we speculate that Nrf2 activation mediates the anti-oxidant stress and anti-inflammatory effects of SFN, thereby exerting SFN's neuroprotective effect (73).
Six studies investigated the efficacy of SFN on autism-like behaviors, one of which was an animal study and others were human studies (Tables 1, 2). In the animal study, BTBR mice treated with SFN (at a dose of 50 mg/kg for 7 days, i.p.) had reduced repetitive behaviors, and increased social interaction in three-chambered sociability test compared to untreated. Other than in the animal study, SFN with various dosages has also shown potential effects in human studies (58). In the study by Singh et al., patients received SFN at a dose of 50–150 μmol/day for 18 weeks, followed by a 4-week washout period. It was observed that SFN treatment substantially ameliorated the core symptoms of ASD (58). Furthermore, researchers followed up and re-evaluated the cases from the study by Singh et al. Many parents and caregivers (57). Specifically, among follow-up cases, one case showed maintained behavioral improvements even after discontinuation of SFN, and nine cases were still taking SFN with persistent improvement (57). In an open-label study, SFN improved the ABC score and significantly improved the symptom of social responsiveness (assessed by SRS) (74). Furthermore, in a recent clinical trial (NCT02561481), a preliminary analysis of the Ohio Autism Clinical Impressions Scale (OACIS) showed that SFN appeared to be safe and effective in children with ASD. Besides, SFN not only had potential effects on partial autism-like behaviors as a monotherapy but also improved irritability and hyperactivity symptoms as an adjunct to risperidone (56).
Notably, five studies explored the impacts of SFN on molecular alterations caused by ASD. In the animal study, SFN-treated BTBR mice reduced Th17 immune responses (STAT3, RORC, IL-17 A, and IL-23R expression in CD4+ T cells) as well as oxidative stress parameters in neutrophils/cerebellum (NF-κB, iNOS, and lipid peroxides) (44). Furthermore, SFN upregulated enzymatic antioxidant defenses (44), which was consistent with the results of in vitro study (45). Nrf2 protein expression and binding activity were impaired in human PBMCs which cultured with LPS. However, SFN can activate Nrf2 with ex vivo treatment by reducing the NF-κB signaling, thereby reversing LPS-induced inflammation and nitrative stress in PBMCs (45). Liu et al. also obtained a similar result that SFN with ex vivo treatment decreased pro-inflammatory gene expression induced by LPS in human PBMCs (46). Consistent with the in vitro study, oral administration of SFN decreased mRNA levels of pro-inflammatory markers in PBMCs from ASD patients (46), conversely, increased the mRNA levels of cytoprotective enzymes (NQO1, HO-1, AKR1C1) and heat shock proteins (HSP27 and HSP70) (46). Besides, Bent et al. identified 77 urinary metabolites correlated with alterations in autism-like symptoms induced by SFN therapy, primarily in oxidative stress, amino acid/gut microbiome, neurotransmitters, hormones, and sphingomyelin metabolism (74). On this account, we can adopt urinary metabolomics analysis to identify how certain biological interventions affect specific symptoms of ASD, providing clues to reveal the pathophysiology of ASD. Furthermore, we can tailor treatment to each ASD individual's unique metabolomics profile in the future.
To sum up, we summarized the extensive evidence for the beneficial effects of SFN, extending from in vitro studies, to animal models, to various clinical studies. Based on the results from human studies, SFN was safe and well-tolerated without serious adverse effects.
Curcumin, (1,7-bis(4-hydroxy-3-methoxyphenyl)-1,6-heptadiene-3,5-dione) along with its mono and dimethoxy derivatives, collectively called curcuminoids (75), which is a hydrophobic polyphenol derived from the rhizome of turmeric (Curcuma longa) (76). Curcumin can cross the blood-brain barrier and has shown to be neuroprotective through upregulating the Nrf2 gene.
Six studies explored the impacts of curcumin on autism-like behaviors and molecular alterations caused by different animal models of ASD. Firstly, curcumin treatment (50, 100, and 200 mg/kg for 4 weeks, orally) restored neurological, behavioral, biochemical, and molecular alterations induced by PPA in a dose-dependent manner (51). Specifically, curcumin improved autism-like behaviors (i.e., reciprocal social interactions, repetitive behaviors, stereotypes, locomotor activity, anxiety, depression, spatial learning, and memory), and suppressed oxidative nitrosative stress (i.e., restored lipid peroxidation, nitrite, GSH, superoxide dismutase, and catalase levels), mitochondrial dysfunction, TNF-α, and matrix metalloproteinases (MMP-9) levels (51). Similarly, neonatal curcumin treatment ameliorated autism-like symptoms of BTBR mice, including social deficits, repetitive behaviors, and cognitive impairments (47). Moreover, curcumin greatly alleviated the suppression of hippocampal neurogenesis in BTBR mice, resulting in increased neurogenesis and proliferation of neural progenitor cells (47). In the VPA model, curcumin also plays a significant role in attenuating abnormalities. On the one hand, curcumin significantly restored abnormal development and behavioral disorders (i.e., social communication and interaction, learning, and memory ability) in VPA rats (48). Besides, curcumin up-regulated the expression of neurons in hippocampal DG and CA3 regions and down-regulated the expression of astrocytes (48). On the other hand, Al-Askar et al. demonstrated that postnatal curcumin treatment reversed various impaired parameters (i.e., IFN-γ, serotonin, glutamine, GSH, glutathione S-transferase, lipid peroxidase, CYP450, IL-6, glutamate, and GSSG) in the VPA model (49). Especially, curcumin improved delayed maturation and abnormal weight (49). Lastly, Chen et al. demonstrated that curcumin ameliorated autistic behavior in a dose-dependent manner (52). Besides, postnatal curcumin treatment increased brain-derived neurotrophic factor (BNDF) level in the temporal cortex at a dose of 50 mg/kg, whereas no effect at a dose of 10 or 30 mg/kg (50, 52). Taken together, curcumin may be an effective option for ASD therapy. However, more clinical studies are needed to prove whether curcumin can be applied to humans.
Agmatine is an endogenous biogenic polyamine synthesized from the decarboxylation of L-arginine in the brain (54). Like other neurotransmitters, agmatine is synthesized, stored, and released in the brain (77). Besides, agmatine could cross the blood-brain barrier (78). Most neurons containing agmatine were located in the hypothalamus, forebrain, and cerebral cortex (77), which played important roles in visceral and neuroendocrine control, pain perception, emotion, and cognition (77, 79). It was reported that agmatine could resist inflammation, apoptotic, and oxidant; could promote angiogenesis and neurogenic; could attenuate gliosis and edema (77, 80). Notably, studies evidenced the important role of agmatine in the pathogenesis of epilepsy, neurodegenerative disorders, and several psychiatric diseases (77, 81, 82). Interestingly, we found a significantly lower level of agmatine in patients with ASD compared with the control group (77), which proved that agmatine can be used as a target for ASD treatment.
Only one animal study investigated agmatine effects on ameliorating ASD symptoms using the VPA animal model. In the study by Kim et al., agmatine sulfate (25, 50, and 100 mg/kg) or saline was supplemented in the VPA model via intraperitoneal injection 30 min before each behavior test (54). As a result, the dose level over 50 mg/kg of agmatine, and not its metabolites, improved the social defect and repetitive and hyperactive behaviors, as well as decreasing seizure threshold in VPA rats. Besides, agmatine normalized the overactive ERK signaling in the prefrontal cortex and hippocampus of VPA-exposed rats (54). Taken together, it suggested a possible therapeutic role of agmatine in ameliorating autism-like symptoms in the VPA animal model.
Naringenin (5,7-Dihydroxy-2-(4-hydroxy phenyl) chroman-4-one) is a flavanone abundantly found in grapefruit, orange, and tomato peels (53, 74). Naringenin exerts its antioxidant effect by inhibiting the NF-κB pathway to reduce oxidative damage to DNA by mice exposed to radiation (83). However, due to the low solubility and high metabolism of naringenin, its bioavailability before entering the systemic circulation is poor, which hinders its clinical efficacy (84).
One study investigated the pharmacotherapeutic potential of naringenin and its brain targeted nanoformulations in ASD. In this study, naringenin (25, 50, and 100 mg/kg), uncoated and coated (GSH & Tween-80) naringenin loaded poly (lactic-co-glycolic acid) (PLGA) nanoparticles (25 mg/kg), and minocycline (50 mg/kg) were given to PPA-induced rats orally for 29 days (53). As a result, in the unencapsulated form, naringenin was only effective at a higher dose of 100 mg/kg because it cannot cross the blood-brain barriers with low bioavailability and P-glycoprotein efflux. Conversely, GSH and Tween-80 coated naringenin nanocarriers acted as multifactorial neurotherapeutic agents, restoring abnormal neuropathological manifestations in the PPA model (53). Taken together, Bhandari et al. demonstrated that PLGA nanoparticles can not only improve naringenin's bioavailability but also enhance their brain absorption capacity through coating with ligands such as GSH and Tween-80, which is an effective delivery system (53). Therefore, naringenin and its coated nanocarriers showed strong clinical potential in attenuating neuro-psychopathological abnormalities associated with ASD.
To the best of our knowledge, this is the first systematic review to qualitatively synthesize existing evidence on the effects of Nrf2 activators administration on ASD in vitro studies, animal studies, and clinical studies. Ultimately, we identified five Nrf2 activators associated with ASD therapy, including RSV, SFN, curcumin, agmatine, and naringenin. We found that the administration of Nrf2 activators could improve autism-like symptoms and molecular alterations in ASD by against inflammation/immune dysfunction, oxidative stress, and mitochondrial dysfunction. Although these five Nrf2 activators have the potential to improve ASD symptoms, they were based on different mechanisms related to Nrf2, as each has unique metabolic patterns and biological activities.
In our systematic review, although a large number of preclinical studies and a small number of clinical studies have proved the efficacy and safety of Nrf2 activators in the treatment of ASD, caution should be warranted in attempting to extrapolate their effects in human studies. If Nrf2 activators were used in high concentrations in tissues lacking Nrf2, there was even a potential risk that Nrf2 may be harmful to subjects. Strikingly, phytochemicals present in fruits and vegetables can promote activation and protection of Nrf2 at low concentration, while would cause toxicity at high doses (85). In addition to the dosage of the Nrf2 activator, the duration of its use should also be considered. To illustrate, Nrf2 activation can reduce tumorigenesis in the early stage, while it promotes the development of treatment-resistant cancer cells in the later stage (20). RSV was well-tolerated without severe side effects in our review. However, in our systematic review, the observation period of the RSV clinical research was only 10 weeks (55), which may not represent the long-term efficacy and side effects of RSV treatment. Instead, RSV supplementation in pregnant non-human primates may cause abnormalities in the fetus (86), consistent with the view that RSV is a teratogen (34). Besides, in one clinical trial of patients with multiple myeloma, RSV treatment has caused various serious adverse events, especially renal failure (87). Therefore, RSV is generally only used as a potential treatment to elucidate the etiology and pathophysiology of ASD. Similarly, while curcumin has been recognized to be safe by the US Food and Drug Administration, curcumin shows conflicting effects on genotoxicity and anti-genotoxicity. Curcumin as low as 0.1 mM may cause disproportionate DNA isolation, nuclear disruption, and micronucleation in proliferating endothelial cells (88). It was also reported that taking curcumin 0.2 mg/kg body weight daily increased the incidence of labial adenoma, hepatocellular adenoma, small intestine cancer, and hepatocellular adenoma in rodents (89). As for SFN, although it was generally safe and well-tolerated in animal models and humans, several side effects were found (i.e., insomnia, vomiting, flatulence, diarrhea, etc.). Interestingly, researchers showed that the dose-response effect of Nrf2 activators may be reversed in pathological tissues, that is, normal beneficial concentrations of phytochemicals will produce toxic effects. For instance, Fragoulis et al. demonstrated the opposite effects of SFN on naïve and TNF-α-stimulated synoviocytes (90). Namely, SFN induced apoptosis of inflammatory synovial cells by activating Nrf2 in naïve synovial cells. Taken together, despite the beneficial effects and few adverse effects of Nrf2 activators, it is necessary to balance their concentration and duration to elucidate their applicability in clinical trials.
Several animal models have been used in this systematic review, including four pharmacological models and one genetic model. VPA, a short-chain fatty acid, is widely used as an antiepileptic drug and mood stabilizer (28). This model is derived from an epidemiological study in which mothers exposed to VPA during pregnancy have a higher risk of ASD among their offspring (91). PPA is an endogenous short-chain fatty acid that can cross the blood-brain barrier (24). Moreover, PPA causes alterations in serotonin, dopamine, and glutamate levels by stimulating calcium release, which simulates what occurs in autism (92, 93). Indeed, numerous studies evidenced higher levels of PPA and other intestinal short-chain fatty acids in ASD subjects (28). The BTBR is the only available genetic model in ASD preclinical studies to date (94), initially used to study insulin resistance, diabetes-induced nephropathy, and phenylketonuria (95). BTBR mice are inbred strains, exhibiting social deficits and repetitive behaviors (96) as well as several immune abnormalities (97), similar to the core symptoms and molecular defects of ASD (35). Thus, the BTBR model is currently a good choice for studying the pathogenesis of ASD. Besides, exposure of pregnant women to any form of progestins (i.e., oral contraceptives, food, beverages, etc.) has been shown to increase the risk of ASD in their offspring (24). Generally, the first trimester of human pregnancy was considered the most sensitive period for prenatal exposure to risk factors. Accordingly, exposure to progestin in the first 7 days of pregnancy in the animal study may be more reasonable. However, prenatal progestin exposure occurred throughout the gestation of Sprague-Dawley rats in the study by Xie et al. (41), which rarely occurs in humans.
Except for agmatine, the Nrf2 activators identified in this systematic review were natural dietary phytochemicals. Mostly, their bioavailability depends on their chemical structure and mode of administration. In particular, due to their first-pass metabolism, oral bioavailability is low (98, 99). After oral administration, partial Nrf2 activators exhibited poor absorption and undergone fast biotransformation, with fewer compounds entering the systemic circulation (89). Based on this, higher doses were required to achieve therapeutic effects. However, for safety reasons, it is necessary to adjust drugs' concentrations to understand their applicability in clinical trials. To date, researchers have developed various strategies to improve the bioavailability of these drugs, thereby achieving therapeutic and safety effects in several neurodegenerative diseases (24). For example, the low oral bioavailability of curcumin hinders its development as a therapeutic drug and/or functional food. However, the animal pharmacokinetic study has shown that the bioavailability of amorphous solid dispersion of curcumin was up to ~13-fold higher than that of unformulated curcumin. Moreover, amorphous curcumin solid dispersions showed enhanced anti-inflammatory activity even at doses 10-fold lower than unconfigured curcumin (75). Besides, nanotechnology has been applied to prepare curcumin nano preparations with higher solubility and slower biotransformation rate, thereby enhancing its bioavailability. There have been several nanoformulations of curcumin including phospholipid complexes, emulsion-based delivery systems, liposomes, polymeric micelles, and curcumin nanoparticle (89). In this systematic review, Bhandari et al. demonstrated that PLGA nanoparticles can not only improve naringenin's bioavailability but also enhance their brain absorption capacity through coating with ligands such as GSH and Tween-80, which was an effective delivery system (53). In conclusion, naringenin and its coated nanocarriers have strong clinical potential in attenuating neuro-psychopathology associated with ASD. However, except for naringenin, there is currently a lack of research on targeted drug delivery systems for curcumin, RSV, SFN, and agmatine. Hence, while studying Nrf2 activators in the treatment of ASD, we should also develop a suitable drug delivery system to enhance their therapeutic potential. In this way, it will not only overcome the low bioavailability issues but also show sustained effects at a lower dose, thereby improving patient compliance.
This systematic review has several limitations. Firstly, this review has some limitations in research design. Different studies differ in research objects (species, age, and gender), intervention methods (drug type, drug dosage, administration method, administration frequency, and duration), outcome evaluation methods, etc., which may lead to inter-study heterogeneity. In terms of experimental subjects, this review covered animals (rats and mice), cells, and humans. Species differences might account for some of the variability of results concerning the efficacy of the various agents. Furthermore, there may be bioavailability differences and metabolism differences as well (inactive, active, toxic metabolites). Therefore, it is difficult to predict whether the results found in animals of different species could be applied to humans. Second, there are few studies to support the Nrf2 activators as dietary phytochemicals in ASD, even though several preclinical studies. Perhaps, these mixed results may be attributed to imperfect statistical analyses, small sample size, and inadequate study design. Therefore, caution should be warranted in attempting to extrapolate their effects in human studies. Third, most preclinical studies involved mainly male animals, and even in clinical studies mainly male patients. It was well-known that ASD affected males more than females by a ratio of 4:1 (28). Fourth, we excluded studies that were not published in English and Chinese, which may lead to eliminating potentially relevant studies.
ASD was considered as a heterogeneous disorder. Patients with ASD will show diverse efficacy in response to the same treatment and intervention (28). Therefore, further RCTs should focus on assessing the efficacy of interventions targeting specific subgroups of ASD. a different subgroup of ASD patients besides evaluating the monotherapy. Moreover, the researchers used animal models of ASD in which animals were prenatal exposure to large doses of progestin, VPA, and PPA to simulate long-term low dose exposure to them in humans, which may limit application in humans. Moreover, despite several Nrf2 activators showed fine efficacy in vitro studies, their low bioavailability limits their activities in the target tissues in vivo studies (60). Consequently, researchers must carefully interpret in vitro studies when attempting to infer the roles of in vivo studies. Most importantly, most Nrf2 activators discussed in our review have multiple functions and molecular targets, therefore, pharmacological research still cannot completely replace the genetic approach. Moreover, due to the difference in animal models and research species, it is not reliable to extrapolate results from one environment to the other. Taken together, further research should improve the standardization of study populations/model, dosage, and outcomes measured, thereby contributed to effective cross-comparisons and meta-analyses of study outcomes. Because of the complex alterations of ASD, studying the pathogenesis of ASD and determining an appropriate therapeutic target remains a formidable challenge in overcoming ASD.
Our systematic review provided suggestive evidence that Nrf2 activators have a potentially beneficial role in improving autism-like behaviors and abnormal molecular alterations through oxidant stress, inflammation, and mitochondrial dysfunction. To sum up, the Nrf2 activators (i.e., RSV, SFN, curcumin, agmatine, and naringenin) identified in this systematic review opened up a new approach to ASD treatment. While these Nrf2 activators are regarded as natural diets to treat ASD and are considered to be relatively safer and healthier methods for humans, they are still limited to becoming a standard treatment because of lacking sufficient proof of their effectiveness and safety. Accordingly, caution should be warranted in attempting to extrapolate their effects in human studies, and better design and more rigorous research are required before they can be determined as a therapeutic option. In a word, Nrf2-based therapy is still an open field issue, and when applied, we should consider a more preventative approach in the future.
The original contributions presented in the study are included in the article/supplementary material, further inquiries can be directed to the corresponding author/s.
JY and YL conceived the manuscript idea. JY and XF researched the manuscript and identified eligible studies. JY, XF, and XL extracted data and performed the quality appraisal of studies. YL was responsible for funding acquisition. All authors critically reviewed the manuscript and read and agree to the published version of the manuscript.
This research was funded by the National Natural Science Foundation of China (NO: 81873806) and the National Natural Science Foundation Hunan Province (NO: 2019JJ40437).
The authors declare that the research was conducted in the absence of any commercial or financial relationships that could be construed as a potential conflict of interest.
1. Association AP. Diagnostic and Statistical Manual of Mental Disorders (DSM-5th). Washington, DC: U.S. American Psychiatric Association (2013).
2. Yang J, Fu X, Liao X, Li Y. Effects of gut microbial-based treatments on gut microbiota, behavioral symptoms, and gastrointestinal symptoms in children with autism spectrum disorder: a systematic review. Psychiatry Res. (2020) 293:113471. doi: 10.1016/j.psychres.2020.113471
3. World Health Organization (WHO). (2019). Available online at: https://www.who.int/news-room/fact-sheets/detail/autism-spectrum-disorders (accessed 19 April, 2020)
4. Maenner MJ, Shaw KA, Baio J, Washington A, Patrick M, DiRienzo M, et al. Prevalence of autism spectrum disorder among children aged 8 years — autism and developmental disabilities monitoring network, 11 sites, United States, 2016. MMWR Surveill Summ. (2020) 69:1–12. doi: 10.15585/mmwr.ss6904a1
5. Liao X, Yang J, Wang H, Li Y. Microglia mediated neuroinflammation in autism spectrum disorder. J Psychiatr Res. (2020) 130:167–76. doi: 10.1016/j.jpsychires.2020.07.013
6. Xu G, Strathearn L, Liu B, O'Brien M, Kopelman TG, Zhu J, et al. Prevalence and treatment patterns of autism spectrum disorder in the United States, 2016. JAMA Pediatr. (2019) 173:153–59. doi: 10.1001/jamapediatrics.2018.4208
7. Serra D, Almeida LM, Dinis TCP. Polyphenols as food bioactive compounds in the context of autism spectrum disorders: a critical mini-review. Neurosci Biobehav Rev. (2019) 102:290–98. doi: 10.1016/j.neubiorev.2019.05.010
8. Rossignol DA, Frye RE. A review of research trends in physiological abnormalities in autism spectrum disorders: immune dysregulation, inflammation, oxidative stress, mitochondrial dysfunction and environmental toxicant exposures. Mol Psychiatry. (2012) 17:389–401. doi: 10.1038/mp.2011.165
9. Rossignol DA, Frye RE. Evidence linking oxidative stress, mitochondrial dysfunction, and inflammation in the brain of individuals with autism. Front Physiol. (2014) 5:150. doi: 10.3389/fphys.2014.00150
10. Rose S, Melnyk S, Pavliv O, Bai S, Nick TG, Frye RE, et al. Evidence of oxidative damage and inflammation associated with low glutathione redox status in the autism brain. Transl Psychiatry. (2012) 2:e134. doi: 10.1038/tp.2012.61
11. Hua Liu, Paul Talalay, Fahey JW. Biomarker-guided strategy for treatment of autism spectrum disorder (ASD). CNS Neurol Disord Drug Targets. (2016) 15:602–13. doi: 10.2174/1871527315666160413120414
12. Frye RE, Delatorre R, Taylor H, Slattery J, Melnyk S, Chowdhury N, et al. Redox metabolism abnormalities in autistic children associated with mitochondrial disease. Transl Psychiatry. (2013) 3:e273. doi: 10.1038/tp.2013.51
13. Rossignol DA, Frye RE. Mitochondrial dysfunction in autism spectrum disorders: a systematic review and meta-analysis. Mol Psychiatry. (2012) 17:290–314. doi: 10.1038/mp.2010.136
14. Rose S, Frye RE, Slattery J, Wynne R, Tippett M, Melnyk S, et al. Oxidative stress induces mitochondrial dysfunction in a subset of autistic lymphoblastoid cell lines. Transl Psychiatry. (2014) 4:e377. doi: 10.1038/tp.2014.15
15. Napoli E, Wong S, Hertz-Picciotto I, Giulivi C. Deficits in bioenergetics and impaired immune response in granulocytes from children with autism. Pediatrics. (2014) 133:e1405–10. doi: 10.1542/peds.2013-1545
16. Anitha A, Nakamura K, Thanseem I, Matsuzaki H, Miyachi T, Tsujii M, et al. Downregulation of the expression of mitochondrial electron transport complex genes in autism brains. Brain Pathol. (2013) 23:294–302. doi: 10.1111/bpa.12002
17. Tang G, Gutierrez Rios P, Kuo SH, Akman HO, Rosoklija G, Tanji K, et al. Mitochondrial abnormalities in temporal lobe of autistic brain. Neurobiol Dis. (2013) 54:349–61. doi: 10.1016/j.nbd.2013.01.006
18. Masi A, Quintana DS, Glozier N, Lloyd AR, Hickie IB, Guastella AJ. Cytokine aberrations in autism spectrum disorder: a systematic review and meta-analysis. Mol Psychiatry. (2015) 20:440–6. doi: 10.1038/mp.2014.59
19. Sandberg M, Patil J, D'Angelo B, Weber SG, Mallard C. NRF2-regulation in brain health and disease: implication of cerebral inflammation. Neuropharmacology. (2014) 79:298–306. doi: 10.1016/j.neuropharm.2013.11.004
20. Sporn MB, Liby KT. NRF2 and cancer: the good, the bad and the importance of context. Nat Rev Cancer. (2012) 12:564–71. doi: 10.1038/nrc3278
21. Dinkova-Kostova AT, Kostov RV, Canning P. Keap1, the cysteine-based mammalian intracellular sensor for electrophiles and oxidants. Arch Biochem Biophys. (2017) 617:84–93. doi: 10.1016/j.abb.2016.08.005
22. Shih RH, Wang CY, Yang CM. NF-kappaB signaling pathways in neurological inflammation: a mini review. Front Mol Neurosci. (2015) 8:77. doi: 10.3389/fnmol.2015.00077
23. Li YJ, Zhang X, Li YM. Antineuroinflammatory therapy: potential treatment for autism spectrum disorder by inhibiting glial activation and restoring synaptic function. CNS Spectr. (2019) 25:493–501. doi: 10.1017/S1092852919001603
24. Bhandari R, Paliwal KJ, Kuhad A. Dietary phytochemicals as neurotherapeutics for autism spectrum disorder: plausible mechanism and evidence. In: Essa MM, Qoronfleh MW, editors. Personalized Food Intervention and Therapy for Autism Spectrum Disorder Management. Switzerland: Advances in Neurobiology. Vol. 24. Cham: Springer. (2020) p. 615–46. doi: 10.1007/978-3-030-30402-7_23
25. Yu M, Li H, Liu Q, Liu F, Tang L, Li C, et al. Nuclear factor p65 interacts with Keap1 to repress the Nrf2-ARE pathway. Cell Signal. (2011) 23:883–92. doi: 10.1016/j.cellsig.2011.01.014
26. Holmstrom KM, Baird L, Zhang Y, Hargreaves I, Chalasani A, Land JM, et al. Nrf2 impacts cellular bioenergetics by controlling substrate availability for mitochondrial respiration. Biol Open. (2013) 2:761–70. doi: 10.1242/bio.20134853
27. Kim TH, Hur EG, Kang SJ, Kim JA, Thapa D, Lee YM, et al. NRF2 blockade suppresses colon tumor angiogenesis by inhibiting hypoxia-induced activation of HIF-1alpha. Cancer Res. (2011) 71:2260–75. doi: 10.1158/0008-5472.CAN-10-3007
28. Malaguarnera M, Khan H, Cauli O. Resveratrol in autism spectrum disorders: behavioral and molecular effects. Antioxidants. (2020) 9:E188. doi: 10.3390/antiox9030188
29. Shin JW, Chun KS, Kim DH, Kim SJ, Kim SH, Cho NC, et al. Curcumin induces stabilization of Nrf2 protein through keap1 cysteine modification. Biochem Pharmacol. (2020) 173:113820. doi: 10.1016/j.bcp.2020.113820
30. Moher D, Liberati A, Tetzlaff J, Altman DG. Preferred reporting items for systematic reviews and meta-analyses: the prisma statement the PRISMA statement. Ann Intern Med. (2009) 151:264–69. doi: 10.7326/0003-4819-151-4-200908180-00135
31. Juybari KB, Sepehri G, Meymandi MS, Vakili Shahrbabaki SS, Moslemizadeh A, Saeedi N, et al. Sex dependent alterations of resveratrol on social behaviors and nociceptive reactivity in VPA-induced autistic-like model in rats. Neurotoxicol Teratol. (2020) 81:106905. doi: 10.1016/j.ntt.2020.106905
32. Hidema S, Kikuchi S, Takata R, Yanai T, Shimomura K, Horie K, et al. Single administration of resveratrol improves social behavior in adult mouse models of autism spectrum disorder. Biosci Biotechnol Biochem. (2020) 84:2207–14. doi: 10.1080/09168451.2020.1794783
33. Fontes-Dutra M, Nunes GD-F, Santos-Terra J, Souza-Nunes W, Bauer-Negrini G, Hirsch MM, et al. Abnormal empathy-like pro-social behaviour in the valproic acid model of autism spectrum disorder. Behav Brain Res. (2019) 364:11–18. doi: 10.1016/j.bbr.2019.01.034
34. Fontes-Dutra M, Santos-Terra J, Deckmann I, Brum Schwingel G, Della-Flora Nunes G, Hirsch MM, et al. Resveratrol prevents cellular and behavioral sensory alterations in the animal model of autism induced by valproic acid. Front Synaptic Neurosci. (2018) 10:9. doi: 10.3389/fnsyn.2018.00009
35. Ahmad SF, Ansari MA, Nadeem A, Alzahrani MZ, Bakheet SA, Attia SM. Resveratrol improves neuroimmune dysregulation through the inhibition of neuronal toll-like receptors and COX-2 signaling in BTBR T(+) Itpr3(tf)/J mice. Neuromolecular Med. (2018) 20:133–46. doi: 10.1007/s12017-018-8483-0
36. Hirsch MM, Deckmann I, Fontes-Dutra M, Bauer-Negrini G, Della-Flora Nunes G, Nunes W, et al. Behavioral alterations in autism model induced by valproic acid and translational analysis of circulating microRNA. Food Chem Toxicol. (2018) 115:336–43. doi: 10.1016/j.fct.2018.02.061
37. Ahmad SF, Ansari MA, Nadeem A, Bakheet SA, Alzahrani MZ, Alshammari MA, et al. Resveratrol attenuates pro-inflammatory cytokines and activation of JAK1-STAT3 in BTBR T(+) Itpr3(tf)/J autistic mice. Eur J Pharmacol. (2018) 829:70–8. doi: 10.1016/j.ejphar.2018.04.008
38. Dai XF, Qin LY, Lian JQ. Resveratrol improves autistic behaviors in rat model. J Third Military Med Univ. (2017) 39:1360–5 doi: 10.16016/j.1000-5404.201703153
39. Bakheet SA, Alzahrani MZ, Ansari MA, Nadeem A, Zoheir KMA, Attia SM, et al. Resveratrol ameliorates dysregulation of Th1, Th2, Th17, and T regulatory cell-related transcription factor signaling in a BTBR T + tf/J mouse model of autism. Mol Neurobiol. (2017) 54:5201–12. doi: 10.1007/s12035-016-0066-1
40. Bhandari R, Kuhad A. Resveratrol suppresses neuroinflammation in the experimental paradigm of autism spectrum disorders. Neurochem Int. (2017) 103:8–23. doi: 10.1016/j.neuint.2016.12.012
41. Xie W, Ge X, Li L, Yao A, Wang X, Li M, et al. Resveratrol ameliorates prenatal progestin exposure-induced autism-like behavior through ERbeta activation. Mol Autism. (2018) 9:43. doi: 10.1186/s13229-018-0225-5
42. Bakheet SA, Alzahrani MZ, Nadeem A, Ansari MA, Zoheir KMA, Attia SM, et al. Resveratrol treatment attenuates chemokine receptor expression in the BTBR T+tf/J mouse model of autism. Mol Cell Neurosci. (2016) 77:1–10. doi: 10.1016/j.mcn.2016.09.004
43. Bambini-Junior V, Zanatta G, Della Flora Nunes G, Mueller de Melo G, Michels M, Fontes-Dutra M, et al. Resveratrol prevents social deficits in animal model of autism induced by valproic acid. Neurosci Lett. (2014) 583:176–81. doi: 10.1016/j.neulet.2014.09.039
44. Nadeem A, Ahmad SF, Al-Harbi NO, Attia SM, Bakheet SA, Ibrahim KE, et al. Nrf2 activator, sulforaphane ameliorates autism-like symptoms through suppression of Th17 related signaling and rectification of oxidant-antioxidant imbalance in periphery and brain of BTBR T+tf/J mice. Behav Brain Res. (2019) 364:213–24. doi: 10.1016/j.bbr.2019.02.031
45. Nadeem A, Ahmad SF, L.Y AL-A, Attia SM, Al-Harbi NO, Alzahrani KS, et al. Differential regulation of Nrf2 is linked to elevated inflammation and nitrative stress in monocytes of children with autism. Psychoneuroendocrinology. (2020) 113:104554. doi: 10.1016/j.psyneuen.2019.104554
46. Liu H, Zimmerman AW, Singh K, Connors SL, Diggins E, Stephenson KK, et al. Biomarker exploration in human peripheral blood mononuclear cells for monitoring sulforaphane treatment responses in autism spectrum disorder. Sci Rep. (2020) 10:5822. doi: 10.1038/s41598-020-62714-4
47. Zhong H, Xiao R, Ruan R, Liu H, Li X, Cai Y, et al. Neonatal curcumin treatment restores hippocampal neurogenesis and improves autism-related behaviors in a mouse model of autism. Psychopharmacology. (2020). doi: 10.1007/s00213-020-05634-5
48. Huang D. Effect of curcumin on hippocampal neurons and astrocytes in autistic rat model. (Master). Jiamusi University, Jiamusi, China (2019)
49. Al-Askar M, Bhat RS, Selim M, Al-Ayadhi L, El-Ansary A. Postnatal treatment using curcumin supplements to amend the damage in VPA-induced rodent models of autism. BMC Complement Altern Med. (2017) 17:259. doi: 10.1186/s12906-017-1763-7
50. Chen S, Jiang Z, Zhang S, Sun Q, Guo L, Pang W. Effect of curcumin on behavior of autism rats and the expression of brain derived neurotrophic factor. J Appl Clin Pediatr. (2012) 27:1187–9 doi: 10.3969/j.issn.1003-515X.2012.15.019
51. Bhandari R, Kuhad A. Neuropsychopharmacotherapeutic efficacy of curcumin in experimental paradigm of autism spectrum disorders. Life Sci. (2015) 141:156–69. doi: 10.1016/j.lfs.2015.09.012
52. Chen SJ. Effect of curcumin on behavior and bdnf expression in autistic animal model rats. (Master). Jiamusi University, Jiamusi (2012).
53. Bhandari R, Paliwal JK, Kuhad A. Naringenin and its nanocarriers as potential phytotherapy for autism spectrum disorders. J Funct Foods. (2018) 47:361–75. doi: 10.1016/j.jff.2018.05.065
54. Kim JW, Seung H, Kim KC, Gonzales ELT, Oh HA, Yang SM, et al. Agmatine rescues autistic behaviors in the valproic acid-induced animal model of autism. Neuropharmacology. (2017) 113:71–81. doi: 10.1016/j.neuropharm.2016.09.014
55. Hendouei F, Sanjari Moghaddam H, Mohammadi MR, Taslimi N, Rezaei F, Akhondzadeh S. Resveratrol as adjunctive therapy in treatment of irritability in children with autism: a double-blind and placebo-controlled randomized trial. J Clin Pharm Ther. (2019) 45:324–34. doi: 10.1111/jcpt.13076
56. Momtazmanesh S, Amirimoghaddam-Yazdi Z, Moghaddam HS, Mohammadi MR, Akhondzadeh S. Sulforaphane as an adjunctive treatment for irritability in children with autism spectrum disorder: a randomized, doubleblind, placebo-controlled clinical trial. Psychiatry Clin Neurosci. (2020) 74:398–405. doi: 10.1111/pcn.13016
57. Lynch R, Diggins EL, Connors SL, Zimmerman AW, Singh K, Liu H, et al. Sulforaphane from broccoli reduces symptoms of autism: a follow-up case series from a randomized double-blind study. Glob Adv Health Med. (2017) 6:2164957X17735826. doi: 10.1177/2164957X17735826
58. Singh K, Connors SL, Macklin EA, Smith KD, Fahey JW, Talalay P, et al. Sulforaphane treatment of autism spectrum disorder (ASD). Proc Natl Acad Sci USA. (2014) 111:15550–5. doi: 10.1073/pnas.1416940111
59. Townsend BE, Johnson RW. Sulforaphane induces Nrf2 target genes and attenuates inflammatory gene expression in microglia from brain of young adult and aged mice. Exp Gerontol. (2016) 73:42–8. doi: 10.1016/j.exger.2015.11.004
60. Gambini J, Ingles M, Olaso G, Lopez-Grueso R, Bonet-Costa V, Gimeno-Mallench L, et al. Properties of resveratrol: in vitro and in vivo studies about metabolism, bioavailability, and biological effects in animal models and humans. Oxid Med Cell Longev. (2015) 2015:837042. doi: 10.1155/2015/837042
61. Albani D, Polito L, Signorini A, Forloni G. Neuroprotective properties of resveratrol in different neurodegenerative disorders. BioFactors. (2010) 36:370–6. doi: 10.1002/biof.118
62. Tarozzi A, Angeloni C, Malaguti M, Morroni F, Hrelia S, Hrelia P. Sulforaphane as a potential protective phytochemical against neurodegenerative diseases. Oxid Med Cell Longev. (2013) 2013:415078. doi: 10.1155/2013/415078
63. Houghton CA, Fassett RG, Coombes JS. Sulforaphane: translational research from laboratory bench to clinic. Nutr Rev. (2013) 71:709–26. doi: 10.1111/nure.12060
64. Egner PA, Chen JG, Wang JB, Wu Y, Sun Y, Lu JH, et al. Bioavailability of Sulforaphane from two broccoli sprout beverages: results of a short-term, cross-over clinical trial in Qidong, China. Cancer Prev Res. (2011) 4:384–95. doi: 10.1158/1940-6207.CAPR-10-0296
65. Benedict AL, Mountney A, Hurtado A, Bryan KE, Schnaar RL, Dinkova-Kostova AT, et al. Neuroprotective effects of sulforaphane after contusive spinal cord injury. J Neurotrauma. (2012) 29:2576–86. doi: 10.1089/neu.2012.2474
66. Carrasco-Pozo C, Tan KN, Borges K. Sulforaphane is anticonvulsant and improves mitochondrial function. J Neurochem. (2015) 135:932–42. doi: 10.1111/jnc.13361
67. Alfieri A, Srivastava S, Siow RCM, Cash D, Modo M, Duchen MR, et al. Sulforaphane preconditioning of the Nrf2/HO-1 defense pathway protects the cerebral vasculature against blood-brain barrier disruption and neurological deficits in stroke. Free Radic Biol Med. (2013) 65:1012–22. doi: 10.1016/j.freeradbiomed.2013.08.190
68. Jazwa A, Rojo AI, Innamorato NG, Hesse M, Fernandez-Ruiz J, Cuadrado A. Pharmacological targeting of the transcription factor Nrf2 at the basal ganglia provides disease modifying therapy for experimental parkinsonism. Antioxid Redox Signal. (2011) 14:2347–60. doi: 10.1089/ars.2010.3731
69. Hou TT, Yang HY, Wang W, Wu QQ, Tian YR, Jia JP. Sulforaphane inhibits the generation of amyloid-beta oligomer and promotes spatial learning and memory in alzheimer's disease (PS1V97L) transgenic mice. J Alzheimers Dis. (2018) 62:1803–13. doi: 10.3233/JAD-171110
70. Schachtele SJ, Hu S, Lokensgard JR. Modulation of experimental herpes encephalitis-associated neurotoxicity through sulforaphane treatment. PLoS ONE. (2012) 7:e36216. doi: 10.1371/journal.pone.0036216
71. Qin S, Yang C, Huang W, Du S, Mai H, Xiao J, et al. Sulforaphane attenuates microglia-mediated neuronal necroptosis through down-regulation of MAPK/NF-kappaB signaling pathways in LPS-activated BV-2 microglia. Pharmacol Res. (2018) 133:218–35. doi: 10.1016/j.phrs.2018.01.014
72. Holloway PM, Gillespie S, Becker F, Vital SA, Nguyen V, Alexander JS, et al. Sulforaphane induces neurovascular protection against a systemic inflammatory challenge via both Nrf2-dependent and independent pathways. Vascul Pharmacol. (2016) 85:29–38. doi: 10.1016/j.vph.2016.07.004
73. Huang C, Wu J, Chen D, Jin J, Wu Y, Chen Z. Effects of sulforaphane in the central nervous system. Eur J Pharmacol. (2019) 853:153–68. doi: 10.1016/j.ejphar.2019.03.010
74. Bent S, Lawton B, Warren T, Widjaja F, Dang K, Fahey JW, et al. Identification of urinary metabolites that correlate with clinical improvements in children with autism treated with sulforaphane from broccoli. Mol Autism. (2018) 9:35. doi: 10.1186/s13229-018-0218-4
75. Chuah AM, Jacob B, Jie Z, Ramesh S, Mandal S, Puthan JK, et al. Enhanced bioavailability and bioefficacy of an amorphous solid dispersion of curcumin. Food Chem. (2014) 156:227–33. doi: 10.1016/j.foodchem.2014.01.108
76. He Y, Liu H, Bian W, Liu Y, Liu X, Ma S, et al. Molecular interactions for the curcumin-polymer complex with enhanced anti-inflammatory effects. Pharmaceutics. (2019) 11:442. doi: 10.3390/pharmaceutics11090442
77. Esnafoglu E, Irende I. Decreased plasma agmatine levels in autistic subjects. J Neural Transm. (2018) 125:735–40. doi: 10.1007/s00702-017-1836-2
78. Piletz JE, May PJ, Wang G, Zhu H. Agmatine crosses the blood-brain barrier. Ann N Y Acad Sci. (2003) 1009:64–74. doi: 10.1196/annals.1304.007
79. Reis DJ, Regunathan S. Is agmatine a novel neurotransmitter in brain? Trends Pharmacol Sci. (2000) 21:187–93. doi: 10.1016/S0165-6147(00)01460-7
80. Freitas AE, Neis VB, Rodrigues ALS. Agmatine, a potential novel therapeutic strategy for depression. Eur Neuropsychopharmacol. (2016) 26:1885–99. doi: 10.1016/j.euroneuro.2016.10.013
81. Piletz JE, Aricioglu F, Cheng J-T, Fairbanks CA, Gilad VH, Haenisch B, et al. Agmatine: clinical applications after 100 years in translation. Drug Discov Today. (2013) 18:880–93. doi: 10.1016/j.drudis.2013.05.017
82. Moretti M, Matheus FC, de Oliveira PA, Neis VB, Ben J, Walz R, et al. Role of agmatine in neurodegenerative diseases and epilepsy. Front Biosci. (2014) 6:341–59.
83. Kumar S, Tiku AB. Biochemical and molecular mechanisms of radioprotective effects of naringenin, a phytochemical from citrus fruits. J Agric Food Chem. (2016) 64:1676–85. doi: 10.1021/acs.jafc.5b05067
84. Bhandari R, Kuhad A, Paliwal JK, Kuhad A. Development of a new, sensitive, and robust analytical and bio-analytical RP-HPLC method for in-vitro and in-vivo quantification of naringenin in polymeric nanocarriers. J Anal Sci Technol. (2019) 10:11. doi: 10.1186/s40543-019-0169-1
85. Son TG, Camandola S, Mattson MP. Hormetic dietary phytochemicals. Neuromolecular Med. (2008) 10:236–46. doi: 10.1007/s12017-008-8037-y
86. Roberts VH, Pound LD, Thorn SR, Gillingham MB, Thornburg KL, Friedman JE, et al. Beneficial and cautionary outcomes of resveratrol supplementation in pregnant nonhuman primates. FASEB J. (2014) 28:2466–77. doi: 10.1096/fj.13-245472
87. Berman AY, Motechin RA, Wiesenfeld MY, Holz MK. The therapeutic potential of resveratrol: a review of clinical trials. NPJ Precis Oncol. (2017) 1:35. doi: 10.1038/s41698-017-0038-6
88. Urdaneta KE, Castillo MA, Montiel N, Semprun-Hernandez N, Antonucci N, Siniscalco D. Autism spectrum disorders: potential neuro-psychopharmacotherapeutic plant-based drugs. Assay Drug Dev Technol. (2018) 16:433–44. doi: 10.1089/adt.2018.848
89. Bhat A, Mahalakshmi AM, Ray B, Tuladhar S, Hediyal TA, Manthiannem E, et al. Benefits of curcumin in brain disorders. BioFactors. (2019) 45:666–89. doi: 10.1002/biof.1533
90. Fragoulis A, Laufs J, Müller S, Soppa U, Siegl S, Reiss LK, et al. Sulforaphane has opposing effects on TNF-alpha stimulated and unstimulated synoviocytes. Arthritis Res Ther. (2012) 14:R220. doi: 10.1186/ar4059
91. Bromley RL, Mawer G, Clayton-Smith J, Baker GA: Liverpool and Manchester Neurodevelopment Group. Autism spectrum disorders following in utero exposure to antiepileptic drugs. Neurology. (2008) 71:1923–24. doi: 10.1212/01.wnl.0000339399.64213.1a
92. DeCastro M, Nankova BB, Shah P, Patel P, Mally PV, Mishra R, et al. Short chain fatty acids regulate tyrosine hydroxylase gene expression through a cAMP-dependent signaling pathway. Brain Res Mol Brain Res. (2005) 142:28–38. doi: 10.1016/j.molbrainres.2005.09.002
93. Afaf K, El-Ansary, Bacha AB, Kotb M. Etiology of autistic features: the persisting neurotoxic effects of propionic acid. J Neuroinflammation. (2012) 9:74. doi: 10.1186/1742-2094-9-74
94. Meyza KZ, Blanchard DC. The BTBR mouse model of idiopathic autism - current view on mechanisms. Neurosci Biobehav Rev. (2017) 76:99–110. doi: 10.1016/j.neubiorev.2016.12.037
95. Bolivar VJ, Walters SR, Phoenix JL. Assessing autism-like behavior in mice: variations in social interactions among inbred strains. Behav Brain Res. (2007) 176:21–6. doi: 10.1016/j.bbr.2006.09.007
96. Silverman JL, Tolu SS, Barkan CL, Crawley JN. Repetitive self-grooming behavior in the BTBR mouse model of autism is blocked by the mGluR5 antagonist MPEP. Neuropsychopharmacology. (2010) 35:976–89. doi: 10.1038/npp.2009.201
97. Li X, Chauhan A, Sheikh AM, Patil S, Chauhan V, Li XM, et al. Elevated immune response in the brain of autistic patients. J Neuroimmunol. (2009) 207:111–16. doi: 10.1016/j.jneuroim.2008.12.002
98. D'Archivio M, Filesi C, Vari R, Scazzocchio B, Masella R. Bioavailability of the polyphenols: status and controversies. Int J Mol Sci. (2010) 11:1321–42. doi: 10.3390/ijms11041321
Keywords: Nrf2 activator, Autism spectrum disorder, oxidative stress, inflammation, mitochondrial dysfunction, dietary supplements
Citation: Yang J, Fu X, Liao X and Li Y (2020) Nrf2 Activators as Dietary Phytochemicals Against Oxidative Stress, Inflammation, and Mitochondrial Dysfunction in Autism Spectrum Disorders: A Systematic Review. Front. Psychiatry 11:561998. doi: 10.3389/fpsyt.2020.561998
Received: 14 May 2020; Accepted: 27 October 2020;
Published: 20 November 2020.
Edited by:
Trevor Ronald Norman, The University of Melbourne, AustraliaReviewed by:
Ahmed Nadeem, King Saud University, Saudi ArabiaCopyright © 2020 Yang, Fu, Liao and Li. This is an open-access article distributed under the terms of the Creative Commons Attribution License (CC BY). The use, distribution or reproduction in other forums is permitted, provided the original author(s) and the copyright owner(s) are credited and that the original publication in this journal is cited, in accordance with accepted academic practice. No use, distribution or reproduction is permitted which does not comply with these terms.
*Correspondence: Yamin Li, YW1pbm55QGNzdS5lZHUuY24=
Disclaimer: All claims expressed in this article are solely those of the authors and do not necessarily represent those of their affiliated organizations, or those of the publisher, the editors and the reviewers. Any product that may be evaluated in this article or claim that may be made by its manufacturer is not guaranteed or endorsed by the publisher.
Research integrity at Frontiers
Learn more about the work of our research integrity team to safeguard the quality of each article we publish.