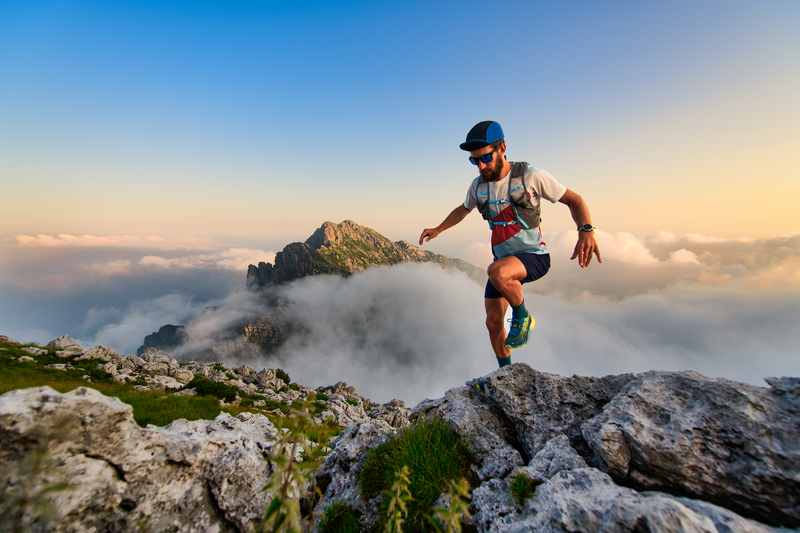
95% of researchers rate our articles as excellent or good
Learn more about the work of our research integrity team to safeguard the quality of each article we publish.
Find out more
ORIGINAL RESEARCH article
Front. Phys. , 17 December 2024
Sec. Medical Physics and Imaging
Volume 12 - 2024 | https://doi.org/10.3389/fphy.2024.1511830
This article is part of the Research Topic Challenges in VHEE Radiotherapy View all 9 articles
Very High-Energy Electrons (VHEE) present a promising innovation in radiation therapy (RT), particularly for the treatment of deep-seated tumors using Ultra High Dose Rate (UHDR) within the framework of FLASH-RT. VHEE offers significant advantages, such as improved tumor targeting, reduced treatment times, and potential utilization of the FLASH effect, which may minimize normal tissue toxicity. However, the lack of an international technical standard for VHEE systems, especially for UHDR applications, remains a critical challenge. Current standards for radiation therapy equipment, such as IEC 60601-2-1 and IEC 60601-2-64, do not encompass VHEE technology. This regulatory gap underscores the need for developing a structured international standard to ensure the basic safety and essential performance of VHEE medical devices. Addressing this challenge requires overcoming complex dose delivery issues, such as the interaction of multiple fields and beam conformality and incorporating novel techniques like broad beam or pencil beam scanning. Establishing comprehensive regulatory standards is essential to ensure patient safety, consistent treatment practices, and the successful clinical integration of VHEE systems. These standards must encompass design guidelines, radiation protection protocols, and integration with existing oncology practices. Collaborative research and development efforts are crucial to formulating evidence-based guidelines, fostering the safe and effective use of VHEE in clinical settings. By addressing these challenges, VHEE technology has the potential to revolutionize cancer therapy, particularly for deep-seated tumors, while enhancing therapeutic outcomes for patients.
Very High-Energy Electrons (VHEE) represent a promising advancement in radiation therapy (RT), particularly for treating deep-seated tumors using Ultra High Dose Rate (UHDR) in the context of FLASH-RT. However, the lack of an international technical standard governing VHEE systems, especially for UHDR applications, poses a significant challenge.
To design future VHEE medical devices, it is essential to consider the following current evidence [1–3]:
- UHDR VHEE is a promising and likely alternative to other UHDR External Beam RT (EBRT) modalities for treating deep-seated tumors, as electrons, even at very high energies, offer advantages in terms of easier high-current beam acceleration and potential cost reductions in clinical linear accelerator (linac) implementation.
- Optimizing VHEE dose delivery requires addressing challenges beyond conventional radiation oncology, factoring in beam temporal structure and the interaction of overlapping beams.
- Unlike single-field, lower-energy potential FLASH treatments (e.g., in the framework of Intra Operative RT (IORT) or dermatological treatments), VHEE conformality requires multiple fields, utilizing either broad beam and/or pencil beam scanning techniques.
Current standards, such as IEC 60601-2-1 [4], which covers electron RT equipment up to 50 MeV, and IEC 60601-2-64 [5], which addresses light ions, do not encompass VHEE technology. This regulatory gap underlines the necessity for a structured approach to establish an international technical standard that ensures the basic safety and essential performance of VHEE-capable medical equipment.
The development of such a standard would provide the foundation for safe clinical application of VHEE systems. Given that basic safety and essential performance are already determined for other forms of RT, including electron and proton systems, it becomes clear that the objective is not merely to address technological advancements, but to establish a clear regulatory framework. This would guide the design, manufacturing, and clinical use of VHEE systems in a way that prioritizes patient safety while maximizing therapeutic efficacy.
From a clinical perspective [6], VHEE holds great promise for improving outcomes in several challenging cancer types. Preclinical studies on FLASH-RT delivered with ultra-high high dose rate intermediate energy electrons (4–20 MeV) have shown encouraging results in multiple organs and tumor types. If confirmed with VHEE, this technology could be a game changer for multiple indications in radiation oncology.
For instance, in the treatment of multiple brain metastases or primary brain tumors such as high-grade gliomas, current methods are often limited by the radiosensitivity of healthy brain tissue [7, 8]. These tumors typically require large-volume irradiation [9], yet delivering curative doses is often impossible without damaging surrounding brain structures [10]. FLASH-RT with intermediate energy electrons showed a significant advantage over conventional dose rate RT in the brain tissues, particularly in preserving cognitive function in animal models, suggesting that neurocognitive sparing might be possible with FLASH-RT in adult [11–14] and juvenile animals [15]. If these effects are confirmed in human trials, it could open the door for dose-escalation studies aimed at enhancing local control of intracranial disease and potentially improving overall survival rates.
Locally advanced non-small cell lung cancer (NSCLC) is another area where VHEE could provide a breakthrough. In cases where surgery is not an option, RT plays a critical role, but long-term survival remains low, with only 15%–20% of patients surviving 5 years post-diagnosis [16, 17]. The challenge lies in delivering curative radiation doses while sparing healthy lung tissue, as the limiting toxicities—acute pneumonitis and late fibrosis—are directly related to the volume of lung exposed to radiation [18–20]. Preclinical data suggest that FLASH-RT delivered with intermediate energy electrons induces significantly less lung fibrosis compared to similar doses of conventional dose rate RT [21, 22]. This positions VHEE as a potentially game-changing modality for lung cancer treatment, enabling higher tumoricidal doses with reduced risks of debilitating side effects.
Recent studies in various preclinical models suggest that, compared to conventional dose-rate RT (CONV), FLASH-RT has distinct effects on circulating immune cells, the tumor immune microenvironment, cytokine production, and inflammatory responses [23–25]. Based on these findings, FLASH-RT could be an ideal complement to immunomodulating drugs, potentially enhancing the therapeutic window of current radioimmunotherapy strategies.
Vertebral metastases, which often affect patients requiring palliative RT, represent another potential application for VHEE. The main challenge is protecting the radiosensitive spinal cord while delivering optimal doses to the vertebra [26]. Exceeding the maximum tolerated dose to the spinal cord can lead to irreversible damage, making it difficult to treat metastatic tumors effectively. Although preclinical studies on the FLASH effect in spinal tissues are still needed, VHEE could allow higher therapeutic doses to be delivered while minimizing spinal cord risk. This makes vertebral metastases an ideal candidate for early clinical trials of VHEE, given the straightforward treatment geometry and high therapeutic potential. For pancreatic cancer, where prognosis remains poor due to local recurrence and early metastasis [27], VHEE could have a profound impact. The proximity of critical organs like the duodenum and small intestine limits RT, making high tumoricidal doses difficult to deliver without severe gastrointestinal complications [28]. While stereotactic ablative RT (SABR) has been explored, complication rates remain high. Emerging data on the protective effects of FLASH-RT on intestinal tissues provide a compelling rationale for investigating VHEE in this context. If validated, this approach could allow higher doses and/or volumes to be delivered safely, without increasing the possible side-effects, and thereby potentially improving local control and survival outcomes in pancreatic cancer patients and other challenging intra-abdominal tumor locations. As we move toward clinical translation, several technological challenges must be addressed. Firstly, there is no clinically certified VHEE linear accelerator available. Moreover, the existing methods of beam delivery for high-energy electrons differ from those for low-energy electrons. Importantly, no pre-clinical study pertaining to the FLASH effect has employed VHEE. Similar to other multiple-beam VHEE applications, understanding the “volume effect” and whether time delays between field transitions could affect the FLASH effect (FE) will be critical for the safe and effective use of VHEE in the clinic.
While FLASH-RT has generated substantial interest in the radiation oncology community, the key to its widespread adoption, together with the technological development and radiobiological knowledge advancement, will be the establishment of a comprehensive international technical standard for VHEE systems. Such a standard would determine the basic safety and essential performance requirements, ensuring that VHEE technology can be implemented safely and effectively in clinical settings.
The complexities associated with VHEE dose delivery, particularly in the context of UHDR modalities, necessitate a thorough understanding of basic safety and essential performance metrics as outlined in IEC standards, specifically IEC 60601-2-1, which governs medical electrical equipment, including electron linacs up to 50 MeV.
VHEE therapy, operating in the range of 50–300 MeV, presents unique challenges that differ significantly from conventional radiation oncology techniques. Traditional methods primarily focus on optimizing conformality—the precision with which the radiation dose conforms to the shape of the tumor. In contrast, VHEE treatments require an integrated approach that considers both the temporal beam structure and the spatial overlap of multiple beams. This dual consideration is particularly critical given that UHDR VHEE modalities can deliver high doses rapidly, which may enhance tumor targeting while minimizing damage to surrounding healthy tissues [3].
The temporal dynamics of UHDR VHEE beams, characterized by their ultra-short pulse delivery, can influence the biological effectiveness of the treatment. Research indicates that the FLASH effect—whereby high doses delivered in very short time frames reduce damage to normal tissues while maintaining tumor control—can be particularly useful in VHEE therapies. This necessitates a comprehensive evaluation of how these time dynamics interact with spatial dose distributions, especially in multi-beam configurations.
Basic Safety and Essential Performance consist in properly setting (via Human Machine Interface–HMI), monitoring (using a monitoring systems in conjunction with HMI) and reporting on the HMI the physical observables involved in the dose delivery and essential for guaranteeing both safety and quality of and during the treatment. For conventional therapy machines, the observables are the type of radiation (X-rays, electrons, protons, etc.), beam energy E, beam fluence φ and dose (total or for each fraction). With UHDR, also the temporal beam structure should be included in the physical observables, and for VHEE, depending on the delivery technology, also beamlet position and/or divergence. In Table 1 the physical observables are summarized.
Table 1. Physical observables for Basic Safety and Essential Performance, conventional RT and VHEE UHDR.
According to IEC 60601-2-1, basic safety also encompasses the protection of patients and operators from electrical, mechanical, and radiation hazards. Also, for VHEE systems, this should include the following requirements:
- Radiation Leakage: The system must minimize radiation exposure to non-target areas, ensuring compliance with strict limits on leakage radiation.
- Electrical Safety: All electrical components must be designed to prevent electric shock and ensure safe operation under fault conditions.
- Mechanical Integrity: The equipment must maintain structural integrity to prevent accidents or malfunctions during operation.
Essential performance refers to the equipment’s ability to operate as intended without compromising safety. For conventional medical accelerators, this is defined by IEC 60976 [29]. Although much of this standard may be applicable, modifications are necessary to account for the temporal beam structure for VHEE systems. Thus, an analogous standard for VHEE systems should take into consideration the following:
- Dose Delivery Accuracy: The system must accurately deliver the prescribed dose to the target while minimizing exposure to surrounding organs at risk (OARs). This requires accurate calibration and real-time monitoring of dose delivery.
- Conformality and Homogeneity: VHEE treatments should achieve a high degree of dose conformality and homogeneity, particularly when treating complex tumor geometries. Studies have shown that VHEE beams can provide superior dose distribution compared to conventional photon beams, leading to better sparing of OARs and enhanced tumor coverage [3].
- Temporal Control: The ability to modulate the dose rate and timing of beam delivery is crucial for optimizing treatment effectiveness and minimizing side effects. This includes the implementation of advanced treatment planning systems capable of accounting for the unique temporal characteristics of VHEE beams.
Additionally, periodic tests to assure the integrity of essential performance are mandatory. The IEC 60977 standard [30] describes the type tests required for conventional linacs, but adaptations are necessary for the higher energy and unique temporal beam structure.
The integration of basic safety and essential performance metrics, as defined by IEC standards, is vital for the successful implementation of VHEE therapy. As the field of RT advances with modalities like VHEE, establishing comprehensive standards to address these unique challenges posed by these systems will be key to ensuring both patient safety and treatment efficacy. The interplay of temporal and spatial factors in VHEE dose delivery underscores the need for continued research and development to refine treatment protocols and enhance clinical outcomes.
In this article, we will only discuss the essential elements of the certification process in the European context (CE marking), although with the entry in force of the EU Medical Device Regulation (MDR) 2017/745 [31], the evidence supporting this process is quite similar to that in other major economic areas (United States, Canada, United Kingdom, Japan, China, etc.).
The certification process for a Medical Device (MD) is based on two conditions that are both necessary: a “technical” verification, whose focus is essentially product safety, and clinical evaluation, which integrates clinical safety and expected clinical performance for the product.
The “technical” verification is essentially based on compliance with technical regulations applicable to the medical device, based on its intended use.
These standards are issued by standardization bodies such as IEC [32].
Dealing with medical accelerators, the reference standard is IEC 60601-1 [33], a general standard that applies to all electro-medical devices, flanked by other collateral standards such as 60601-1-2 [34] for electromagnetic compatibility, 60601-1-6 [35] for usability etc., and particular standards dependent on the type of accelerator (60601-2-8 [36] X ray in the range 10 kV up to 1 MV, 60601-2-1 [4] for electrons between 1 and 50 MeV, 60601-2-64 [5] light ions).
From this it follows that although there will be, in the near future, for VHEE accelerators a particular technical standard, to the analogous IEC 60601-2-1, which is used today for electron accelerators in the class between 1 and 50 MeV, this standard will only be the first necessary step to provide the basis for getting to clinical use of a VHEE system, but it will not be a sufficient condition.
Indeed, it will be necessary for the manufacturer of a MD for VHEE to also demonstrate clinical efficacy for target tumors and areas through a clinical evaluation in compliance with MDR. This pathway will be equally challenging.
Indeed, the clinical evaluation of a medical device, and particularly an innovative medical device such as a device for VHEE, will have to demonstrate the safety and clinical performance of the device, now inferred from various pre-clinical studies in in vitro and/or animal models, on humans.
Without a clinical evaluation according to the requirements of MDR2017/745, demonstrating that what has already been achieved in pre-clinical testing is replicable in humans in a safe and effective manner, there can be no DM on the market that can be authorized for use in humans for VHEE.
In fact, the MD regulatory landscape in Europe, prescribes that the clinical evaluation to be based on clinical data obtained by a scientifically valid method from a critical review of the currently available scientific literature on the issues of safety, performance, design characteristics and intended use of the device, and/or a critical analysis of the results of all available clinical investigations and a review of any alternative treatment options currently available for the same purpose (ref. to Art.61 of the MDR).
All this clinical data must demonstrate that the proposed device, such as the device for VHEE, is capable of providing the intended benefit (e.g., treatment of target tumors) with a benefit-to-risk ratio that is equal to or less than currently commercially available treatment options.
For clinical evaluations to be performed in humans (clinical investigations) the MDR 2017/745, in order to protect the health of the patients involved, prescribes specific authorization pathways by the National Competent Authorities (Italian Healt Ministry in Italy, BfArm in Germany etc.), and due to the very nature of the target diseases to be treated, clinical investigations can only be lengthy, since post-treatment follow-ups will necessary require important, time-consuming and clinically complex observations.
From these considerations, therefore, it is clear that it cannot be overlooked that while there is extremely interesting pre-clinical data on Flash technology today, the need to obtain clinical confirmations on humans will be a major challenge from all parties (manufacturers, scientific community) who would like to confirm the validity of this technology on humans. A challenge as necessary as of defining a particular standard to be used for verification of radiogenic safety, similar to 60601-2-1, and no less complicated, indeed.
Future standards for Very High Energy Electron (VHEE) therapy must focus on ensuring optimal Dose Volume Histograms (DVH) and Dose Rate Volume Histograms (DRVH) on a voxel-by-voxel basis [37], specifically tailored to the unique characteristics of each dose delivery mechanism. This approach is essential for accurately assessing treatment plans and improving patient outcomes.
To effectively implement these standards, a double threshold must be established:
- Energy Threshold: The lower threshold should be set at 50 MeV, as this is the point beyond which IEC 60601-2-1 standards do not apply. This regulation ensures that equipment used for VHEE therapy meets stringent safety and performance criteria, which are crucial for high-energy applications.
- Average/Instantaneous Dose Rate and Dose per Pulse Threshold: The second threshold should focus on average/instantaneous dose rates and/or dose per pulse metrics. This is particularly important for UHDR modalities, where the delivery of high doses in short time frames can significantly impact treatment efficacy and normal tissue sparing.
Defining precise mathematical parameters for UHDR in the context of VHEE is essential, as current definitions designed for proton therapies may not directly apply. This requires the development of new models and algorithms that consider the unique interactions and physical characteristics of VHEE beams, including their energy deposition patterns and biological effects. The relationship between the quantities measured by the beam monitoring system, those verified through quality assurance, and those calculated by the treatment planning system is particularly complex.
In conventional RT, the physical observable is the delivered dose, monitored by the monitoring system (monitor chamber, electrometer, HMI). However, in the case of UHDR, it is critical to not only define the delivered dose but also account for the temporal characteristics of each beam delivery within the different radiation fields. This ensures:
- Accurate evaluation of radiobiological effects: The treatment planning system must be able to correctly assess the radiobiological impact of the dose delivery.
- Real-time monitoring of quality and conformity: During treatment, the beam monitoring system must verify that the delivery aligns with the calculated plan in terms of quality and dose conformity both spatially and temporally.
In addition, for pencil beam scanning, a new formalism must be developed to manage beamlet penumbra and scanning time, similar to the approach used for protons [38].
There are two conditions which seem to trigger the FLASH effect: average dose rate greater than 40 Gy/s and a total irradiation time less than 0.2 s [39, 40]. Nevertheless, the temporal beam structure is quite complex, and these two parameters are not enough to fully describe it [41–43]. Given the relationship between VHEE and UHDR, it is crucial to establish standard definitions for the beam’s temporal structure, along with the mathematical relationships between these parameters:
- Temporal beam structure: The temporal sequence of the beam delivery. It is identified by the entire set of Dose per pulse, Pulse duration, Time between pulses, Pulse Repetition Frequency, Number of Pulses, Irradiation Time, and Delay Time.
- Dose per pulse (Dp): Dose of a single pulse at the Equipment reference point (ERP).
- Pulse duration (tp): Temporal width of a single pulse.
- Time between pulses (tr): Time between two pulses in a sequence of pulses of irradiation.
- Pulse repetition frequency (PRF): Frequency of repetition of the pulses in a sequence of pulses of irradiation.
- Number of pulses (np): Number of pulses in a sequence of pulses of the irradiation.
- Irradiation time (tFL): Temporal duration of a sequence of pulses of the irradiation.
- Delay time (tD): Time separation between two subsequent sequences of pulses of the irradiation.
- Delivered dose (DD): Total delivered dose at ERP during tFL.
- Total irradiation time (tIRR): The sum of all irradiation and delay times.
- Total delivered dose (TD): Total delivered dose at ERP during tIRR.
- Average Dose rate (DR): Dose rate during a sequence of pulses of the irradiation at ERP. The ratio of Delivered Dose (DD) and Irradiation Time (tFL).
- Intra-pulse Dose rate (DRp): Dose rate within the pulse at ERP. The ratio of Dose per Pulse (Dp) and Pulse Duration (tp).
- Instantaneous Dose rate (IDR): Dose rate value at a specific moment of time within the pulse at ERP.
In general, medical linacs inherently generate pulsed beams; each irradiation event encompasses a sequence of pulses, each pulse of the duration tp of a few microseconds. The pulses are spaced apart by a time interval denoted as tr, which typically ranges in the order of tenths of milliseconds. The value of tr is inversely related to the Pulse Repetition Frequency (PRF), determining the rate at which pulses are delivered. A representation of this general scheme is depicted in Figure 1. An irradiation can also consist in a series of multiple sub-irradiations separated by a delay time tD. A representation of this general scheme is depicted in Figure.
Where:
− Dp(n),k dose of nth pulse in the kth irradiation at the ERP [Gy]
− tpk time width of a single pulse in the kth irradiation [s]
− trk time between two pulses in the kth irradiation [s]
− PRFk Pulse Repetition Frequency in the kth irradiation [s–1]
− np,k Number of pulses of the kth irradiation
− tFLk irradiation time of the kth irradiation [s]
− tDk delay time, time separation between the kth and (k+1)th irradiations [s]
− DDk delivered dose at ERP during tFLk [Gy]
− tIRR Total irradiation time [s]
− TD total delivered dose at ERP during tIRR [Gy]
− DRk Average Dose Rate during the kth irradiation at ERP [Gy s–1]
− DRpi,k Dose rate within the ith pulse during the kth irradiation at ERP [Gy s–1]
− IDRi,k Instantaneous Dose Rate within the ith pulse during the kth irradiation at ERP [Gy s–1]
In case of a single irradiation (Figure 1), the following relations hold:
If the irradiation consists of multiple sub-irradiations (Figure 2), the previous equations can be easily generalized and the additional relations hold:
To reach the fluences required in for UHDR mode, electron beam current accelerated is increased respect to the current electron accelerators. In these conditions, the power absorbed by e-beam becomes comparable with the power absorbed by the accelerating waveguide; therefore, any e-beam current variation implies a variation in the kinetic energy [44]. Let be W the power generated by the radiofrequency, it is
The power absorbed by the accelerated waveguide, and thus the electric field, can be calculated as
where E0 is the on axis electric field inside the accelerating waveguide, S its overall length, T the transit time factor and ZLINAC its shunt impedance.
The power absorbed by the electron beam can be calculated as
In the following example, consider the case of a high current VHEE linac [45]:
- Nominal energy
- Beam Current
- Linac length
Assuming a shunt impedance per unit length of 100 MΩ/m, resulting in
And
Therefore, the two terms are comparable; any variation in the beam current implies a fluctuation in beam energy as well. Hence both beam current and beam energy should be measured independently, and an additional interlock on beam energy could be considered.
Effective beam monitoring is vital for ensuring treatment accuracy and safety. The temporal structure of VHEE beams, along with the potential spatial overlap of individual beamlets in pencil beam scanning delivery, must be meticulously monitored. Therefore, alternatives to conventional ionization chambers and detectors, which are susceptible to saturation at ultra-high dose rates [44] must be considered [46, 47].
Real-time beam monitoring presents significant challenges, particularly with respect to ultra-high dose rate delivery and the beam’s temporal structure of VHEE system. Conventional transmission detectors, such as gas-filled transmission ionization chambers used as golden standard in radiotherapy, encounter issues like ion recombination effect under UHDR exposures [44]. Additionally, these detectors must provide rapid feedback signals to halt the beam once the intended radiation dose has been delivered. However, ionization chambers, which are commonly used as beam monitors in clinical radiotherapy, tend to have slow timing responses with ion collections times on the order of 300 µs in 5 mm air gap separation. As a result, new beam monitoring systems may be required for safe delivery of clinical VHEE RT machines.
Currently, no ideal detector exists for monitoring UHDR VHEE beams. Nonetheless, various approaches are being explored to adapt ionization chambers for this purpose. One promising design is the multi-gap ionization chamber proposed by Giordanengo et al. [48]. This instrument features three parallel-plate ionization chambers, each with a different gap width. The charge collected by each chamber exhibits varying levels of ion recombination depending on the electrode spacing, which can be phenomenologically modeled to facilitate corrections for charge collection efficiency.
Ultra-thin silicon detectors have also been investigated as potential beam monitors due to their high sensitivity, exceptional spatial resolution, and strong radiation hardness [49]. Previous studies have demonstrated excellent linearity with dose rates for both photon [50] and proton beams [51]; however, further evaluation is needed to assess their applicability for pulsed electron beams. As such, the design and development of an optimal geometry for silicon detectors are still in progress.
Silicon carbide (SiC) detectors are gaining traction as a promising alternative for dosimetry and monitoring in UHDR beam applications [52]. These detectors offer significant advantages, including strong radiation resistance, rapid response times, high sensitivity, and stability across varying dose rates [53, 54]. However, extensive research is still required to fully harness the capabilities of this technology.
Beam current transformers (BCTs) are non-intercepting, inductive current monitors that have shown potential for real-time monitoring of UHDR electron beams [55–57]. Commonly used in research electron accelerators, they facilitate non-destructive charge measurements of individual beam pulses with high accuracy and reproducibility [58]. However, a key limitation of BCTs is their inability to provide information on beam cross-section and spatial distribution, rendering them ineffective for determining beam dimensions or flatness [29].
Recently, calorimetric methods have emerged as viable options for monitoring UHDR exposures [59]. A significant advantage of calorimetry is its dose-rate independence due to the physical nature of the phenomena. Furthermore, a transmission calorimeter can be designed with multiple sections to effectively detect beam symmetry and flatness. While further development is needed to fully exploit the capabilities of calorimetric methods, they continue to show promise as a technique for monitoring UHDR beams.
Additionally, it is important to distinguish between the treatment approaches for broad and pencil beams, as each modality presents unique advantages and challenges. Broad beams offer the advantage of quicker implementation since they are more closely aligned with conventional irradiation techniques and single-field UHDR irradiation. The beam monitoring requirements are less stringent, with the primary distinction from IEC 60601-2-1 being the UHDR component. Monitoring for broad beams must focus on the dose delivered, current and energy variations, temporal beam structure, and gantry rotation, which affects the delay between irradiation trains. However, broad beams face significant challenges, particularly because clinical implementation heavily relies on the tissue-sparing effect of FLASH. This is compounded by the fact that broad beams offer less conformality compared to pencil beams and result in a higher surface dose than protons or light ions. Radiation safety can also be a concern, as beam broadening (whether passive or active) and field collimation introduce additional stray radiation and neutron production due to interactions between high-energy electrons and beamline components.
On the other hand, pencil beams offer greater conformality [60], which reduces the reliance on a stronger FLASH effect and allows for more complex treatments. This approach also minimizes stray radiation through magnetic scanning techniques. However, pencil beam scanning presents a more complex formalism, similar to UHDR protons [38], and necessitates monitoring of the angular divergence of beamlets, as seen in proton therapy (IEC 60601-2–64). Furthermore, pencil beams require a more advanced beam monitoring system to track the position of individual beamlets, necessitating sophisticated technology beyond the integration systems like ACCTs (Alternating-Current Current Transformers) typically used for UHDR delivery [61]. In summary, the development of future standards for VHEE therapy should prioritize the development of comprehensive DVH and DRVH metrics that are energy-specific and dose-rate-focused. By establishing clear thresholds and enhancing beam monitoring capabilities, the treatment planning process can be significantly improved. This approach will ultimately lead to better patient outcomes through optimized dose delivery and reduced risks to healthy surrounding tissues, paving the way for the clinical implementation of VHEE therapies in radiation oncology.
The design of the human-machine interface (HMI) and control console for VHEE systems is crucial for ensuring both intuitive operation and robust safety protocols. As VHEE technology advances, it is essential to evaluate how these systems differ from existing standards, particularly concerning radiation protection aspects such as neutron yield and stray radiation.
The HMI for VHEE systems must prioritize user-friendliness to facilitate seamless operation by medical personnel. Key features include:
- Intuitive Controls: The interface should provide clear visual indicators and controls that allow operators to easily adjust settings and monitor system performance in real-time.
- Safety Features: Built-in safety mechanisms, such as emergency shut-off controls and interlocks, are paramount to protect both patients and staff.
Current regulations, including IEC 60601-2-1, may not adequately encompass the specific safety requirements presented by VHEE systems. Key areas that require attention include:
- Neutron Yield: VHEE systems can produce photoneutrons due to high-energy electron interactions, which poses unique challenges in radiation protection. Studies indicate that the neutron yield from VHEE systems is comparable to that of conventional proton therapy [62]. However, it is crucial to carefully evaluate the implications for both patient and staff exposure.
- Stray Radiation: The risk of stray radiation from VHEE systems necessitates additional monitoring and shielding strategies to ensure compliance with safety standards. This is particularly important in clinical settings where multiple treatment areas may be in proximity, in addition to UHDR radiation safety requirements [63].
A simple amalgamation of existing standards, such as IEC 60601-2-1 and IEC 60601-2-64, is insufficient to guarantee the basic safety and essential performance of VHEE systems. Instead, a dedicated set of standards tailored to the unique characteristics of VHEE technology is required. This includes:
- Advanced Beam Monitoring Requirements: Establishing precise standards for real-time beam monitoring systems is critical, especially for UHDR VHEE beams. This includes defining the performance specifications for new detectors, tailored to the ultra-high dose rates and unique temporal structures of VHEE beams. These standards must address rapid feedback mechanisms, accuracy in dose delivery, and spatial beam monitoring to ensure treatment precision and patient safety.
- Integration with Imaging and Treatment Planning: Updates to the broader family of radiation oncology systems must encompass imaging, planning, and positioning technologies to accommodate the new VHEE requirements. This integration will enable comprehensive treatment planning that considers both therapeutic and safety aspects.
- Specific Radiation Protection Guidelines: Developing guidelines that address the unique neutron and radiation profiles of VHEE systems will enhance safety protocols and operational standards.
The design of the human-machine interface and control console for VHEE systems must balance intuitive operation with stringent safety measures. As VHEE technology progresses, it is crucial to develop dedicated standards that address the unique radiation protection challenges associated with high-energy electron therapy. By focusing on specific guidelines for neutron yield, stray radiation, and comprehensive treatment planning, the medical community can ensure the safe and effective implementation of VHEE systems in clinical practice.
The integration of VHEE technology into clinical practice represents a transformative advancement in the treatment of deep-seated tumors, particularly through the application of Ultra-High Dose Rate (UHDR) modalities. While the potential benefits are substantial, the absence regulatory standards poses significant challenges that must be addressed through collaborative research and development efforts.
VHEE technology offers several advantages for radiation oncology, particularly in the treatment of tumors located deep within the body. Key benefits include:
- Enhanced Tumor Targeting: VHEE beams can deliver high doses of radiation with precision, allowing for effective treatment of complex tumor geometries while minimizing damage to surrounding healthy tissues.
- Potential for FLASH Effect: The ultra-high dose rates associated with VHEE therapy may leverage the FLASH effect, which has been shown to reduce normal tissue toxicity while maintaining tumor control, thus improving patient outcomes.
- Reduced Treatment Times: The UHDR capabilities of VHEE systems enable rapid dose delivery, which can improve patient throughput and enhance overall treatment efficiency.
Despite the promising advances in VHEE technology, the absence of established regulatory standards poses several challenges:
- Safety and Efficacy Concerns: Without comprehensive standards, there is a risk that VHEE systems may not meet the necessary safety and performance benchmarks, potentially compromising patient safety and treatment efficacy.
- Variability in Practice: The lack of standardized protocols can lead to inconsistencies in treatment practices across different institutions, making it challenging to ensure consistent patient care and outcomes.
- Need for Collaborative Research: Addressing these challenges necessitates a collaborative effort among stakeholders - including regulatory bodies, manufacturers, and clinical practitioners -to develop evidence-based guidelines that reflect the unique characteristics of VHEE technology.
To facilitate the successful integration of VHEE systems into clinical practice, it is essential to establish a comprehensive set of standards that encompass:
- Design and Implementation Guidelines: Clear guidelines for the design and operational protocols of VHEE systems will aid manufacturers in creating equipment that meets safety and performance criteria.
- Integration with Existing Oncology Practices: Standards should also consider the integration of VHEE technology with existing imaging, planning, and treatment delivery systems to create a cohesive treatment environment.
- Radiation Protection Protocols: Specific protocols addressing the unique radiation profiles of VHEE systems, including neutron yield and stray radiation, are crucial for ensuring the safety of both patients and healthcare providers.
In conclusion, the future of radiation oncology may significantly depend on the successful integration of VHEE technology, contingent upon the development and adoption of essential regulatory standards. By addressing the current challenges through collaborative research and establishing comprehensive guidelines, the medical community can harness the full potential of VHEE systems to enhance the treatment of deep-seated tumors. This proactive approach will not only ensure patient safety and treatment efficacy but also foster innovative advancements in cancer therapy, ultimately improving patients’ outcomes worldwide.
The original contributions presented in the study are included in the article/supplementary material, further inquiries can be directed to the corresponding authors.
JP: Conceptualization, Investigation, Writing–original draft, Writing–review and editing. FD: Conceptualization, Writing–original draft, Writing–review and editing. AC: Writing–review and editing. MC: Writing–review and editing. AD: Writing–review and editing. MD: Writing–review and editing. GF: Writing–review and editing. LG: Writing–review and editing. LM: Writing–review and editing. GM: Writing–review and editing. PM-G: Writing–review and editing. FP: Writing–review and editing. MP: Writing–original draft, Writing–review and editing. VP: Writing–review and editing. SP: Writing–review and editing. PP: Writing–review and editing. FR: Writing–review and editing. ASa: Writing–review and editing. ASu: Writing–original draft, Writing–review and editing. AV: Writing–review and editing. GF: Conceptualization, Investigation, Writing–original draft, Writing–review and editing.
The author(s) declare that financial support was received for the research, authorship, and/or publication of this article. We thank Fondazione Pisa for funding CPFR with the grant “prog. n. 134/2021.” The research is also partially supported by INFN CSN5 funded project “FRIDA.” The research has received funding from the European Union—NextGenerationEU through the Italian Ministry of University and Research under PNRR—M4C2-I1.3 Project PE_00000019 “HEAL ITALIA.” This work was supported by United Kingdom Research and Innovation (grant MR/Y018761/1). This work was funded by Piano Nazionale di Ripresa e Resilienza (PNRR), Missione 4, Componente 2, Ecosistemi dell’Innovazione–Tuscany Health Ecosystem (THE), Spoke 1 “Advanced Radiotherapies and Diagnostics in Oncology”—CUP I53C22000780001.
Authors MD, LG, and GF were employed by Sordina IORT Technologies S.p.A. Author MP was employed by Maytal International Ltd. – Global Med Dev Expertise.
The remaining authors declare that the research was conducted in the absence of any commercial or financial relationships that could be construed as a potential conflict of interest.
The author(s) declare that no Generative AI was used in the creation of this manuscript.
All claims expressed in this article are solely those of the authors and do not necessarily represent those of their affiliated organizations, or those of the publisher, the editors and the reviewers. Any product that may be evaluated in this article, or claim that may be made by its manufacturer, is not guaranteed or endorsed by the publisher.
1. Schüler E, Eriksson K, Hynning E, Hancock SL, Hiniker SM, Bazalova-Carter M, et al. Very high-energy electron (VHEE) beams in radiation therapy; Treatment plan comparison between VHEE, VMAT, and PPBS. Med Phys (2017) 44(6):2544–55. doi:10.1002/mp.12233
2. Bazalova-Carter M, Qu B, Palma B, Hårdemark B, Hynning E, Jensen C, et al. Treatment planning for radiotherapy with very high-energy electron beams and comparison of VHEE and VMAT plans. Med Phys (2015) 42(5):2615–25. doi:10.1118/1.4918923
3. Muscato A, Arsini L, Battistoni G, Campana L, Carlotti D, De Felice F, et al. Treatment planning of intracranial lesions with VHEE: comparing conventional and FLASH irradiation potential with state-of-the-art photon and proton radiotherapy. Front Phys (2023) 11:1185598. doi:10.3389/fphy.2023.1185598
4. Webstore. IEC 60601-2-1:2020, Medical electrical equipment - Part 2-1: particular requirements for the basic safety and essential performance of electron accelerators in the range 1 MeV to 50 MeV (2020) Available from: https://webstore.iec.ch/publication/31388. Accessed on 15th of October 2024.
5. Webstore. IEC 60601-2-64:2014. Particular requirements for the basic safety and essential performance of light ion beam medical electrical equipment (2014) Available from: https://webstore.iec.ch/en/publication/2680. Accessed on 15th of October 2024.
6. Ursino S, Gadducci G, Giannini N, Gonnelli A, Fuentes T, Di Martino F, et al. New insights on clinical perspectives of FLASH radiotherapy: from low-to very high electron energy. Front Oncol (2023) 13:1254601. doi:10.3389/fonc.2023.1254601
7. Achrol AS, Rennert RC, Anders C, Soffietti R, Ahluwalia MS, Nayak L, et al. Brain metastases. Nat Rev Dis Primers (2019) 5(1):5. doi:10.1038/s41572-018-0055-y
8. Rodríguez-Camacho A, Flores Vazquez JG, Moscardini Martelli J, Torres-Rio JA, Olmos-Guzman A, Ortiz-Arce CS, et al. Glioblastoma treatment: state-of-the-art and future perspectives. Int J Mol Sci (2022) 23:7207. doi:10.3390/ijms23137207
9. Kruser TJ, Bosch WR, Badiyan SN, Bovi JA, Ghia AJ, Kim MM, et al. NRG brain tumor specialists consensus guidelines for glioblastoma contouring. J Neurooncol (2019) 143(1):157–66. doi:10.1007/s11060-019-03152-9
10. Zhong X, Huang B, Feng J, Yang W, Liu H. Delayed leukoencephalopathy of non-small cell lung cancer patients with brain metastases underwent whole brain radiation therapy. J Neurooncol (2015) 125(1):177–81. doi:10.1007/s11060-015-1888-9
11. Montay-Gruel P, Petersson K, Jaccard M, Boivin G, Germond JF, Petit B, et al. Irradiation in a flash: unique sparing of memory in mice after whole brain irradiation with dose rates above 100 Gy/s. Radiother Oncol (2017) 124(3):365–9. doi:10.1016/j.radonc.2017.05.003
12. Montay-Gruel P, (2019) Long-term neurocognitive benefits of FLASH radiotherapy driven by reduced reactive oxygen species. Proc Natl Acad Sci U S A 116, 10943–51. doi:10.1073/pnas.1901777116
13. Montay-Gruel P, Acharya MM, Gonçalves Jorge P, Petit B, Petridis IG, Fuchs P, et al. Hypofractionated FLASH-RT as an effective treatment against glioblastoma that reduces neurocognitive side effects in mice. Clin Cancer Res (2021) 27(3):775–84. doi:10.1158/1078-0432.ccr-20-0894
14. Allen BD, Alaghband Y, Kramár EA, Ru N, Petit B, Grilj V, et al. Elucidating the neurological mechanism of the FLASH effect in juvenile mice exposed to hypofractionated radiotherapy. Neuro-oncology (2023) 25(5):927–39. doi:10.1093/neuonc/noac248
15. Alaghband Y, Allen BD, Kramár EA, Zhang R, Drayson OG, Ru N, et al. Uncovering the protective neurologic mechanisms of hypofractionated FLASH radiotherapy. Cancer Res Commun (2023) 3(4):725–37. doi:10.1158/2767-9764.crc-23-0117
16. Casal-Mouriño A, Ruano-Ravina A, González ML, Rodríguez-Martínez A, Osorio AG, Lema LV, et al. Epidemiology of stage III lung cancer: frequency, diagnostic characteristics, and survival. Transl Lung Cancer Res (2021) 10(1):506–18. doi:10.21037/tlcr.2020.03.40
17. Nichols L, Saunders R, Knollmann FD. Causes of death of patients with lung cancer. Arch Pathol Lab Med (2012) 136(12):1552–7. doi:10.5858/arpa.2011-0521-OA
18. Käsmann L, Dietrich A, Staab-Weijnitz CA, Manapov F, Behr J, Rimner A, et al. Radiation-induced lung toxicity - cellular and molecular mechanisms of pathogenesis, management, and literature review. Radiat Oncol (2020) 15(1):214. doi:10.1186/s13014-020-01654-9
19. Meng J, Li Y, Wan C, Sun Y, Dai X, Huang J, et al. Targeting senescence-like fibroblasts radiosensitizes non-small cell lung cancer and reduces radiation-induced pulmonary fibrosis. JCI Insight (2021) 6(23):e146334. doi:10.1172/jci.insight.146334
20. Arroyo-Hernández M, Maldonado F, Ruiz FL, Muñoz-Montaño WM, Nuñez-Baez M, Arrieta O. Radiation-induced lung injury: current evidence. BMC Pulm Med (2021) 21:9. doi:10.1186/s12890-020-01376-4
21. Favaudon V, Caplier L, Monceau V, Pouzoulet F, Sayarath M, Fouillade C, et al. Ultrahigh dose-rate FLASH irradiation increases the differential response between normal and tumor tissue in mice. Sci translational Med (2014) 6.245. doi:10.1126/scitranslmed.3008973
22. Fouillade C, Curras-Alonso S, Giuranno L, Quelennec E, Heinrich S, Bonnet-Boissinot S, et al. FLASH irradiation spares lung progenitor cells and limits the incidence of radio-induced senescence. Clin Cancer Res (2020) 26:1497–506. doi:10.1158/1078-0432.ccr-19-1440
23. Montay-Gruel P, Acharya MM, Petersson K, Alikhani L, Yakkala C, Allen BD, et al. Long-term neurocognitive benefits of FLASH radiotherapy driven by reduced reactive oxygen species. Proc Natl Acad Sci U S A (2019) 116(22):10943–51. doi:10.1073/pnas.1901777116
24. Buonanno M, Grilj V, Brenner DJ. Biological effects in normal cells exposed to FLASH dose rate protons. Radiother Oncol (2019) 139:51–5. doi:10.1016/j.radonc.2019.02.009
25. Montay-Gruel P, Markarian M, Allen BD, Baddour JD, Giedzinski E, Jorge PG, et al. Ultra-high-dose-rate FLASH irradiation limits reactive gliosis in the brain. Radiat Res (2020) 194(6):636–45. doi:10.1667/rade-20-00067.1
26. Peyraga G, Ducassou A, Arnaud F-X, Lizée T, Pouédras J, Moyal E. Radiothérapie et toxicité médullaire: actualités et perspectives [Radiotherapy and spinal toxicity: News and perspectives]. Cancer Radiother (2021) 25(1):55–61. doi:10.1016/j.canrad.2020.05.017
27. Park W, Chawla A, Eileen M. O’Reilly pancreatic cancer: a review. JAMA (2021) 326(9):851–62. doi:10.1001/jama.2021.13027
28. Goldsmith C, Plowman PN, Melanie M, Green MM, Dale RG, Price PM, et al. Stereotactic ablative radiotherapy (SABR) as primary, adjuvant, consolidation and re-treatment option in pancreatic cancer: scope for dose escalation and lessons for toxicity. Radiat Oncol (2018) 19 13(1):204. doi:10.1186/s13014-018-1138-3
29. Webstore. Medical electrical equipment - medical electron accelerators - functional performance characteristics (2007) Available from: https://webstore.iec.ch/en/publication/4088. Accessed on 15th of October 2024.
30. Webstore. IEC TR 60977:2008. Medical electrical equipment - medical electron accelerators - guidelines for functional performance characteristics (2008) Available from: https://webstore.iec.ch/en/publication/4089. Accessed on 15th of October 2024.
31. EU MDR: REGULATION (EU) 2017/745 of the EUROPEAN parliament and of the council of 5 april 2017 on medical devices, amending directive 2001/83/EC, regulation (ec) No 178/2002 and regulation (EC) No 1223/2009 and repealing council directives 90/385/EEC and 93/42/EEC
32. Available from: https://www.iec.ch/homepage. Accessed on 15th of October 2024.
33. Webstore. IEC 60601-1 Consolidated version, Medical electrical equipment - Part 1: general requirements for basic safety and essential performance. Available from: https://webstore.iec.ch/en/publication/67497. Accessed on 15th of October 2024.
34. Webstore. IEC 60601-1-2 Consolidated version, Medical electrical equipment - Part 1-2: general requirements for basic safety and essential performance - collateral Standard: electromagnetic disturbances - requirements and tests. Available from: https://webstore.iec.ch/en/publication/67554. Accessed on 15th of October 2024.
35. Webstore. IEC 60601-1-6 Consolidated version, Medical electrical equipment - Part 1-6: general requirements for basic safety and essential performance - collateral standard. Usability. Available from: https://webstore.iec.ch/en/publication/67381. Accessed on 15th of October 2024.
36. Webstore. IEC 60601-2-8 Consolidated version, Medical electrical equipment - Part 2-8: particular requirements forthe basic safety and essential performance of therapeutic X-ray equipment operating in the range 10 kV to 1 MV. Available from: https://webstore.iec.ch/en/publication/23416. Accessed on 15th of October 2024.
37. Folkerts MM, Abel E, Busold S, Perez JR, Krishnamurthi V, Ling CC. A framework for defining FLASH dose rate for pencil beam scanning. Med Phys (2020) 47(12):6396–404. doi:10.1002/mp.14456
38. Schwarz M, Traneus E, Safai S, Kolano A, van de Water S. Treatment planning for Flash radiotherapy: general aspects and applications to proton beams. Med Phys (2022) 49(4):2861–74. doi:10.1002/mp.15579
39. Limoli CL, Kramár EA, Almeida A, Petit B, Grilj V, Baulch JE, et al. The sparing effect of FLASH-RT on synaptic plasticity is maintained in mice with standard fractionation. Radiother Oncol (2023) 2023:109767. doi:10.1016/j.radonc.2023.109767
40. Liljedahl E, Konradsson E, Gustafsson E, Jonsson KF, Olofsson JK, Ceberg C, et al. Long-term anti-tumor effects following both conventional radiotherapy and FLASH in fully immunocompetent animals with glioblastoma. Scientific Rep (2022) 12(1):12285. doi:10.1038/s41598-022-16612-6
41. Garibaldi C, Beddar S, Bizzocchi N, Böhlen TT, Iliaskou C, Moeckli R, et al. Minimum and optimal requirements for a safe clinical implementation of ultra-high dose rate radiotherapy: a focus on patient’s safety and radiation protection. Radiother Oncol (2024) 196:110291. doi:10.1016/j.radonc.2024.110291
42. McGarrigle JM, Long KR, Prezado Y. The FLASH effect—an evaluation of preclinical studies of ultra-high dose rate radiotherapy. Front Oncol (2024) 14:1340190. doi:10.3389/fonc.2024.1340190
43. Vozenin M-C, Montay-Gruel P, Limoli C, Germond JF. All irradiations that are ultra-high dose rate may not be FLASH: the critical importance of beam parameter characterization and in vivo validation of the FLASH effect. Radiat Res (2020) 194(6):571–2. doi:10.1667/rade-20-00141.1
44. Di Martino F, Barca P, Barone S, Bortoli E, Borgheresi R, De Stefano S, et al. FLASH radiotherapy with electrons: issues related to the production, monitoring, and dosimetric characterization of the beam. Front Phys (2020) 8:570697. doi:10.3389/fphy.2020.570697
45. Faillace L, Alesini D, Bisogni G, Bosco F, Carillo M, Cirrone P, et al. Perspectives in linear accelerator for FLASH VHEE: study of a compact C-band system. Physica Med (2022) 104:149–59. doi:10.1016/j.ejmp.2022.10.018
46. Felici G, Barca P, Barone S, Bortoli E, Borgheresi R, De Stefano S, et al. Transforming an IORT linac into a FLASH research machine: procedure and dosimetric characterization. Front Phys (2020) 8:374. doi:10.3389/fphy.2020.00374
47. Romano F, Bailat C, Jorge PG, Lerch MLF, Darafsheh A. Ultra-high dose rate dosimetry: challenges and opportunities for FLASH radiation therapy. Med Phys (2022) 49(7):4912–32. doi:10.1002/mp.15649
48. Giordanengo S, Guarachi LF, Braccini S, Cirrone GAP, Donetti M, Fausti F, et al. Fluence beam monitor for high-intensity particle beams based on a multi-gap ionization chamber and a method for ion recombination correction. Appl Sci (2022) 12(23):12160. doi:10.3390/app122312160
49. Medina E, Ferro A, Abujami M, Camperi A, Centis Vignali M, Data E, et al. First experimental validation of silicon-based sensors for monitoring ultra-high dose rate electron beams. Front Phys (2024) 12:1258832. doi:10.3389/fphy.2024.1258832
50. Vignati A, Giordanengo S, Fausti F, Martì Villarreal OA, Mas Milian F, Mazza G, et al. Beam monitors for tomorrow: the challenges of electron and photon FLASH RT. Front Phys (2020) 8:375. doi:10.3389/fphy.2020.00375
51. Wyrsch N, Antognini L, Ballif C, Braccini S, Casolaro P, Dunand S, et al. Amorphous silicon detectors for proton beam monitoring in FLASH radiotherapy. Radiat Meas (2024) 177:107230. arXiv preprint arXiv:2401.14300. doi:10.1016/j.radmeas.2024.107230
52. Okpuwe C, Amato A, D'Amico I, De Liso V, De Napoli M, Di Martino F, et al. Silicon carbide detectors for dosimetry and monitoring of ultra-high dose rate beams. J Instrumentation (2024) 19(03):C03064. doi:10.1088/1748-0221/19/03/c03064
53. De Napoli M. SiC detectors: a review on the use of silicon carbide as radiation detection material. Front Phys (2022) 10:898833. doi:10.3389/fphy.2022.898833
54. Romano F, Milluzzo G, Di Martino F, D’Oca MC, Felici G, Galante F, et al. First characterization of novel silicon carbide detectors with ultra-high dose rate electron beams for FLASH radiotherapy. Appl Sci (2023) 13:2986. doi:10.3390/app13052986
55. Gonçalves Jorge P, Grilj V, Bourhis J, Vozenin C-M, Germond JF, Bochud F, et al. Technical note: validation of an ultrahigh dose rate pulsed electron beam monitoring system using a current transformer for FLASH preclinical studies. Med Phys (2022) 49:1831–8. doi:10.1002/mp.15474
56. Oesterle R, Gonçalves Jorge P, Grilj V, Bourhis J, Vozenin M-C, Germond J-F, et al. Implementation and validation of a beam current transformer on a medical pulsed electron beam LINAC for FLASH-RT beam monitoring. J Appl Clin Med Phys (2021) 22:165–71. doi:10.1002/acm2.13433
57. Vojnovic B, Tullis IDC, Newman RG, Petersson K. Monitoring beam charge during FLASH irradiations. Front Phys (2023) 11:1185237. doi:10.3389/fphy.2023.1185237
58. Schüller A, Illemann J, Renner F, Makowski C, Kapsch RP. Traceable charge measurement of the pulses of a 27 MeV electron beam from a linear accelerator. J Instrumentation (2017) 12(03):P03003. doi:10.1088/1748-0221/12/03/p03003
59. Flynn SF, Lee ND, Thomas RAS, Palmans H, Duane S. Transmission calorimeter for measuring dose of radiation (2022) Available from: https://worldwide.espacenet.com/patent/search/family/082324167/publication/WO2023227909A1?q=WO2023227909. Accessed on 15th of October 2024.
60. Fischer J, Whitmore L, Desrosiers C, Sheehy S, Bazalova-Carter M. Very high-energy electrons as radiotherapy opportunity. The Eur Phys J Plus (2024) 139(8):728. doi:10.1140/epjp/s13360-024-05455-x
61. Di Martino F, Del Sarto D, Bass G, Capaccioli S, Celentano M, Coves D, et al. Architecture, flexibility and performance of a special electron linac dedicated to Flash radiotherapy research: electronFlash with a triode gun of the centro pisano flash radiotherapy (CPFR). Front Phys (2023) 11:1268310. doi:10.3389/fphy.2023.1268310
62. Masilela TAM, Delorme R, Prezado Y. Dosimetry and radioprotection evaluations of very high energy electron beams. Scientific Rep (2021) 11(1):20184. doi:10.1038/s41598-021-99645-7
Keywords: VHEE radiotherapy, FLASH radiotherapy, UHDR, regulatory standards, IEC (international electrotechnical commission)
Citation: Pensavalle JH, Di Martino F, Cavalieri A, Celentano M, De Gregorio A, Di Francesco M, Franciosini G, Galluzzo L, Masturzo L, Milluzzo G, Montay-Gruel P, Paiar F, Pantaleoni M, Patera V, Pioli S, Poortmans P, Romano F, Sarti A, Subiel A, Vannozzi A and Felici G (2024) Standard requirements for clinical very high energy electron and ultra high dose rate medical devices. Front. Phys. 12:1511830. doi: 10.3389/fphy.2024.1511830
Received: 15 October 2024; Accepted: 02 December 2024;
Published: 17 December 2024.
Edited by:
Jiaru Shi, Tsinghua University, ChinaReviewed by:
Kiki Theodorou, University of Thessaly, GreeceCopyright © 2024 Pensavalle, Di Martino, Cavalieri, Celentano, De Gregorio, Di Francesco, Franciosini, Galluzzo, Masturzo, Milluzzo, Montay-Gruel, Paiar, Pantaleoni, Patera, Pioli, Poortmans, Romano, Sarti, Subiel, Vannozzi and Felici. This is an open-access article distributed under the terms of the Creative Commons Attribution License (CC BY). The use, distribution or reproduction in other forums is permitted, provided the original author(s) and the copyright owner(s) are credited and that the original publication in this journal is cited, in accordance with accepted academic practice. No use, distribution or reproduction is permitted which does not comply with these terms.
*Correspondence: J. H. Pensavalle, amFrZWhhcm9sZC5wZW5zYXZhbGxlQGdtYWlsLmNvbQ==; G. Felici, Z2l1c2VwcGVmZWxpY2kxOTcxQGdtYWlsLmNvbQ==
Disclaimer: All claims expressed in this article are solely those of the authors and do not necessarily represent those of their affiliated organizations, or those of the publisher, the editors and the reviewers. Any product that may be evaluated in this article or claim that may be made by its manufacturer is not guaranteed or endorsed by the publisher.
Research integrity at Frontiers
Learn more about the work of our research integrity team to safeguard the quality of each article we publish.