- Science and Technology on Metrology and Calibration Laboratory, Beijing Institute of Radio Metrology and Measurement, Beijing, China
Quantum frequency standards are crucial for time measurement, satellite navigation, telecommunication, and other essential applications. Beijing Institute of Radio Metrology and Measurement (BIRMM) has been working on quantum frequency standards and their applications for tens of years. This paper introduces the latest progress on quantum frequency standards at BIRMM, including a calcium optical clock, an active hydrogen maser, and a mercury ion microwave clock. Based on the 1S0-3P1 transition of calcium atoms, a transportable optical clock prototype is built with a stability of 8 × 10–15 at 1 s. A compact active hydrogen maser has been developed for the Chinese space station. It will be used for scientific research such as examining Einstein’s theory of general relativity and has just been lunched. The preliminary frequency stability of the maser is 1.27 × 10–15 at 10000 s. Additionally, a prototype mercury ion microwave clock is developed using the hyperfine transition between 62S1/2, F = 0 and 62S1/2, F = 1. The trapped Hg+ ions are pumped by mercury discharge lamps and cooled by Helium gas. The measured clock transition linewidth is about 1 Hz, and frequency stability of 4 × 10–13 at 1 s and 4 × 10–14 at 1000 s is achieved.
Introduction
In the international system of units (SI), time is the most accurately measured fundamental physical quantity. Before the mid-20th century, the definition and measurement of time mainly depended on observing the sun, stars, or related celestial bodies. A more precise method of measuring and defining time is made possible by the development of atomic spectroscopy and quantum mechanics; specifically, the time unit “second” is defined and measured by stimulating and amplifying the radiation emission of cesiums’ atomic transition to generate standard frequency. The invention of the quantum frequency standard has promoted the revolution in the time and frequency metrology field. In the past 70 years, the development of quantum frequency standard technology has improved the accuracy of time measurement from 10–11 to 10–18 or even better, almost one order of magnitude improvement every 10 years [1].
Quantum frequency standard plays a vital role in precision measurement, satellite navigation, communication network, and scientific research. The progress of quantum frequency standard technology accelerates the development of time measurement and dramatically improves the measurement accuracy of other physical quantities. The resolution of the SI units adopted by the 26th General Conference on Weights and Measures [2] in 2018 demonstrates that other fundamental physical quantities’ measurement accuracy will be improved or has already been improved by switching to time or frequency measurement. The quantum frequency standard is also the base for satellite navigation. Due to the high speed of the electromagnetic wave, the accuracy of time measurement is required to be in the order of nanoseconds or more accurate. Quantum frequency standard is also used in communication, electric power grid, transportation, and other fields to provide accurate synchronous time and frequency signals.
Beijing Institute of Radio Metrology and measurement (BIRMM) is an institution that has been researching on time measurement and quantum frequency standards for tens of years. It has established the atomic time scale UTC (BIRM) and participated in the International time and frequency coordination. The institute tries to build time-keeping clocks to give precise frequency standards and time standards. This paper introduces the latest researches on quantum frequency standards at BIRMM, mainly including a calcium atom beam optical clock [3, 4], an active hydrogen maser for the Chinese space station [5], and a mercury ion microwave clock [6–8]. All these clocks can be used as time-keeping clocks and be applied to fields such as time scale, satellite navigation and space exploration.
Ca atomic beam optical clock
Utilizing an optical clock to keep the time directly seems more realizable since the laser and quantum systems have become robust. Many techniques have been developed to build compact, transportable and robust optical clocks. Groups in PTB developed a transportable Sr clock in 2017 [9], which verified the relativistic gravitational redshift. A compact commercial Yb+ ion clock is developed to operate in lab conditions [10]. The research group at Skolkovo Institute of Science and Technology developed a compact transportable 171Yb+ single-ion clock [11].
An optical clock based on an atomic beam has the potential to become a compact clock with good stability due to its simple structure and high signal-to-noise ratio (SNR). The calcium atomic beam clock [12] is one promising scheme for its minimal number of lasers and the availability of the lasers. This atomic beam clock operates with many atoms, and the fluorescent transition can be detected continuously, thus providing a high signal-to-noise ratio. A good frequency stability can be achieved. Many efforts have been made based on different interrogating and detecting schemes [13–15], achieving excellent results. The National Institute of Standards and Technology (NIST) group realized an stability of 6 × 10–15 at 1 s with the Ramsey method on the Ca beam in 2012 [16], and then an stability smaller than 6 × 10–16 at 1 s and 2 × 10–16 at 1000 s was achieved in 2019 [4]. The group at Peking University realized an stability of about 5 × 10–14 at 1 s in 2017 [3, 15].The clock transition of calcium is 1S0-3P1, as shown in Figure 1, with the frequency 455 986 240 494 140 (8) Hz and wavelength 657 459 439.291 683 (12) femtometer [17]. The clock transition can be read out by a 423 nm or a 431 nm laser. The former one resonates with the 1S0-1P1 transition, the linewidth of which is about 35 MHz. Its operation is simple, and only a 423 nm external cavity diode laser (ECDL) locking to the 1S0-1P1 transition is needed. It also has some defects. The linewidth of 423 nm transition is quite larger than that of 657 nm transition, which means for all the atoms that read out by the 423 nm laser, only the well collimated beam can interact with the 657 nm clock laser. Thus the contrast of 657 nm fluorescence signal is limited. The (4s4p)3P1-(4p2)3P0 cycle transition may solve this problem since the 431 nm laser can only detect the atoms at the (4s4p)3P1 state. The frequency stabilization of the 431 nm laser poses a problem since it cannot be locked to the atomic transition directly. A hollow cathode lamp or a wavemeter may solve this locking problem. The NIST’s group utilizes an 862 nm ECDL locking to a cavity to solve the problem, and then the frequency is doubled to 431 nm to detect the atoms.
BIRMM is also working on the Ca atomic beam scheme, trying to build a compact, transportable clock with a long continuous operating time. The proposed system uses a two-laser scheme [18], including a 657 nm interrogation laser and a 423 nm readout laser. Figure 2 shows the clock setup. The system contains three locking loops. The 423 nm laser is first locked to the 1S0-1P1 transition. The 657 nm ECDL is locked to a ULE cavity through the Pound-Drever-Hall (PDH) method. Then the cavity stabilized 657 nm laser is locked to the 1S0-3P1 transition by feedback to the AOM2. For now, the saturated absorption spectrum is used to stabilize the clock laser, to keep the system simple and robust. Some methods such as Ramsey spectrum, cooled atomic beams, transverse cooling can further improve the clock stability.
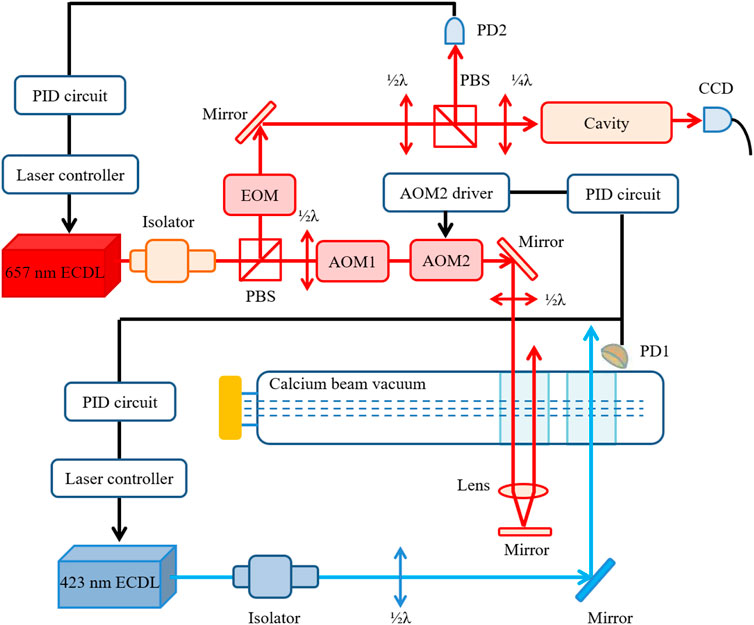
FIGURE 2. Scheme of the calcium beam frequency standard. The 423 nm ECDL is locked to 1S0-1P1 transition and used as a readout laser. The 657 nm laser is first locked to a cavity. Then the laser frequency is shifted and locked to 1S0-3P1 transition through two AOMs.
The Ca atomic beam frequency standard consists of four parts, a 657 nm PDH laser frequency stabilization unit, a Ca atomic beam tube, a frequency shift and detection optics units, and control electronics. A customized base is designed on which all the optics and the Ca beam vacuum are installed. Transporting or shaking the base does not affect the optics significantly, but the vibration can affect the locking. Thus, the vibration has to be isolated. The 657 nm PDH, the frequency shift, and detection optics are mounted on an active vibration isolation table. An enclosure is used to suppress the influence of airflow and temperature fluctuation. The total size of the optics and physics parts is about 0.9 m3. The 657 nm clock laser stability is about (5–7) × 10–15 at 1–10 s.
A Ca atomic beam vacuum tube is designed and manufactured as Figure 3 shows. The oven contains about 3 g calcium and support about 1 year operation (for experiment, not 7 × 24 hours at 600°C). The life span mainly determined by the size of the nozzle and temperature. By using a combination of small tubes as the nozzle, we achieved a very high atomic flux with small divergence. The resonant atomic flux is about 4 × 1012 per second at 600°C and 1 × 1013 per second at 650°C. The divergence is smaller than 30 mrad [19]. The nozzle aperture is 3 mm × 6.8 mm (horizontal × vertical). The saturated absorption spectrum is detected as shown in Figure 3. The right top curve is the saturated absorption spectrum. The linewidth of the spectrum is about 450 kHz. The right bottom curve is the error signal, which is utilized to lock the clock laser.
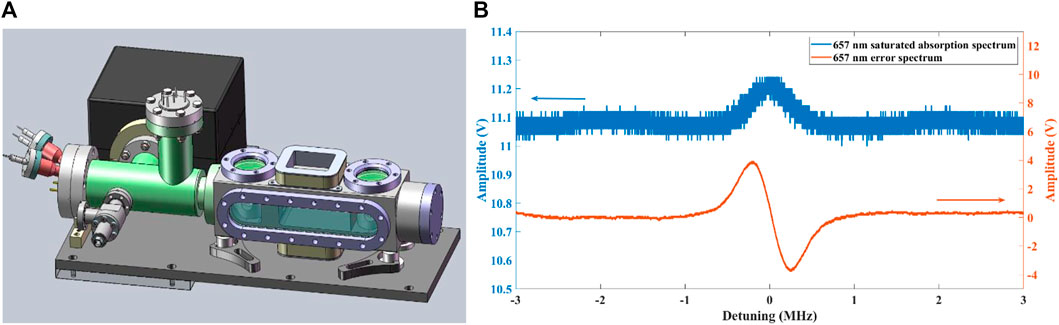
FIGURE 3. Design of the atomic beam vacuum tube and the clock transition spectrum. (A) Design of the atomic beam vacuum tube. Two fluorescence detection windows are set to test the beam collimation. The 657 nm clock laser incident at the middle of the side window. (B) The clock transition spectrum. The blue curve is the saturated absorption spectrum. The linewidth of the spectrum is about 450 kHz. The red curve is the error signal.
For the control electronics, a mode-hop monitoring and controlling algorithm is developed. A wave meter is used to monitor the wavelength of the single longitudinal mode. When the laser mode hops, the software program automatically sweeps the laser PZT and current to find another appropriate setting. Then the software program tunes the laser wavelength to the target value and relocks to the atoms or cavity. The 423 nm readout laser is locked to 1S0-1P1 transition peak through 5 kHz frequency modulation. The 657 nm clock laser is locked through 2 kHz frequency modulation.
A fully automatic running algorithm is developed to keep the three loops locked. The 423 nm fluorescence amplitude signal is used to check if the 423 nm laser loses lock. The transmitted signal from the 657 nm cavity checks if the PDH loses lock. The cavity stabilized laser’s ability to maintain lock to the atoms is tested using the error signal voltage and AOM feedback frequency. Any time one of these loops loses lock, the relock process is triggered. The above program will automatically tune the laser frequency if a laser mode hops. Currently, the calcium frequency standard system can be locked independently in the lab for approximately 1 day. The deterioration of the ECDLs’ modes is the main cause of those lock losses. The automatically running algorithm allows a losing lock to relock within 10 min.
A calcium beam frequency standard prototype is built. The output stability of the frequency standard is not yet measured. The clock laser is locked to the atoms through an AOM. The frequency feedback to the AOM is recorded. Since we only have one setup, we use this feedback frequency to evaluate the short-term stability roughly. It shows a stability of 8 × 10–15 at 1 s and 2 × 10–14 at 10 s. We deduce that the atoms, detection optics and locking electronics worsen the stability. Since we use the 423 nm fluorescence amplitude signal to lock the 423 nm laser and the clock laser, the stability of the 423 nm fluorescence affects the clock stability directly. A significant fluctuation of the 423 nm voltage has been observed. It may result from the power fluctuation of the 423 nm laser, temperature fluctuation of the atomic oven, and the spontaneous emission noise of the 423 nm single mode laser. In the subsequent studies, the authors intend to increase the fluorescence stability and develop a second calcium frequency standard to measure the stability.
Active hydrogen maser for space station
The active hydrogen maser is the quantum frequency standard which holds nearly the best short-term and medium-term frequency stability at microwave frequency up to 105 s. A full-size active hydrogen maser having a cavity with an intrinsic quality factor bigger than the oscillation threshold value can be operated actively. Rarely is the use of a full-size active hydrogen maser in space reported due to the volume and weight. In 2011, the Vremya-CH firm in Russia created the first full-scale active hydrogen maser operating in space design with frequency stability of 3 × 10–13 at 1 s and 3 × 10–15 at 1000 s, 60 kg, and size 460 mm × 729 mm.
A space active hydrogen maser (SAHM) is being constructed with funding from China’s scientific space program. It is used to verify some physical effects in outer space under a microgravity environment. For example, the study of the Sun’s gravitational field distribution, the verification of Einstein’s “Theory of relativity”, the detection of gravitational waves, measurement of the fine structure constants, lunar exploration, etc [20, 21]. A SAHM is constructed to be disciplined to a cold microwave clock and an optical clock, which can create more accurate and stable frequency signals [5].
SAHM Design
Although a full-size active hydrogen maser has an excellent performance in medium-term frequency stability, the volume and weight limit its application in space. For Chinese space station, the active hydrogen maser should be lighter than 40 kg and smaller than 370 mm × 460 mm × 600 mm. Since the microwave cavity takes three-quarters of the total active hydrogen maser volume, it is conducive to reduce the size of the microwave cavity for the miniaturization of the maser. The microwave cavity is filled with dielectrics having large permittivity and low loss, such as sapphire. However, the sapphire that filled the cavity significantly deteriorates the stability of the cavity resonant frequency due to the high frequency-temperature coefficient. Currently, the frequency-temperature coefficient of the sapphire microwave cavity of the active hydrogen masers [22] is −65 kHz/°C, which is too sensitive to optimize the long-term frequency stability. The authors have tried to reduce the microwave cavity temperature coefficient and volume to overcome the above technical bottleneck with a new NMT (magnesium tantalate microwave ceramic) dielectric as the loading material. The dielectric loss (Qf) of NMT is 2.4 × 105, almost similar to sapphire’s 3 × 105, which can ensure the cavity’s quality factor bigger than the oscillation threshold value. Moreover, the frequency-temperature coefficient of NMT is 0∼± 2 ppm/K, much better than sapphire’s −50 ppm/K. Performances of these two dielectric materials are shown in Table 1. This newly designed dielectric-loaded microwave cavity has a Q value of 40,000 and a temperature coefficient of −10 kHz/°C, with a storage bulb of about 0.9 L. The diameter is remarkably reduced from 200 to 140 mm. Figure 4 compares NMT-loaded and sapphire-loaded cavities.
Based on the NMT-cavity, the physics package is designed with four magnetic shielding layers. The design is shown in Figure 5. There are two getter pumps in the physics package. The getter pump, combined with the ion pump maintains the vacuum in the hydrogen atomic beam formation system. The other one on the top of the vacuum tank creates a vacuum in the RF cavity. The overall size of the physics package is Φ220 mm × 500 mm.
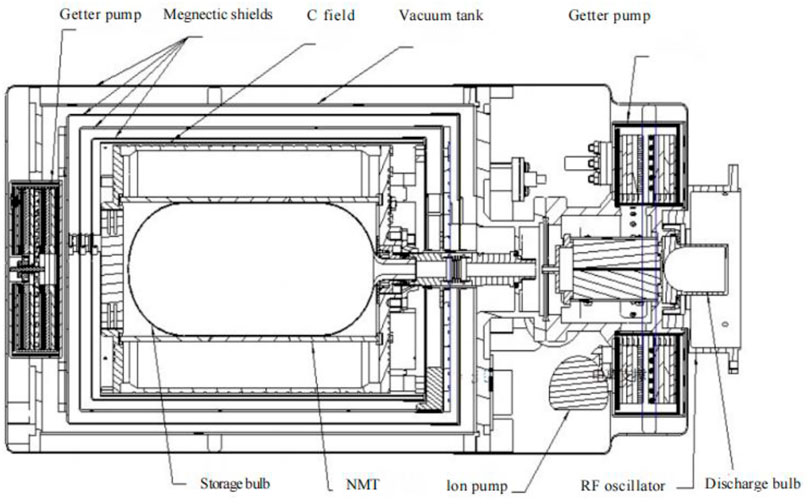
FIGURE 5. Physics design based on NMT-loaded cavity. Physics package has four magnetic shielding layers and two getter pumps combined with the ion pump maintains the vacuum in the hydrogen atomic beam formation system and vacuum in the RF cavity.
The electronics package combines the techniques of heterodyne receiving and frequency synthesis. The maser signal at −100 dBm is sent through a ferrite isolator to isolate the electronics and physics packages. The 1420.405751 MHz preamplifier has very low noise (noise factor 1.5 dB), which is necessary to minimize the white phase noise added by the receiver. The local signal (1400 MHz, 20 MHz) and 1420.405751 MHz output from the maser are mixed to give a beat output of 0.405751 MHz, amplified by the intermediate-frequency amplifiers having a narrow bandwidth and phase detected with a synthesized signal of 405.751 kHz. The output of the physics package is accurately related to the quartz oscillator using a phase-locked loop. The electronics are shown in Figure 6. The DDS1 outputs two frequencies alternately to detect the transition probability at both sides of the atomic resonant peak.
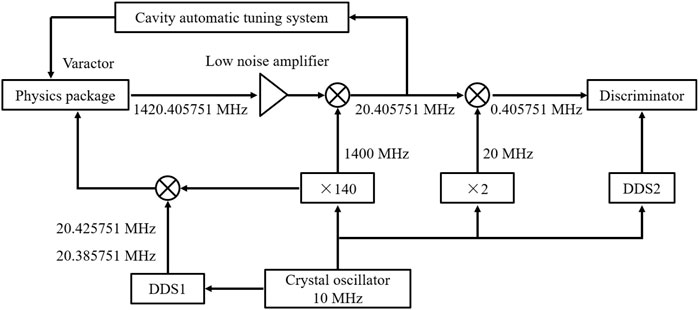
FIGURE 6. Electronics package of the active hydrogen maser. The local signal (1400 MHz) and 1420.405751 MHz output from the maser are mixed to give a beat output of 20.405751 MHz, phase detected with a synthesized signal of 405.751 kHz. The output of the physics package is accurately related to the quartz oscillator using phase-locked loop. The DDS1 outputs two frequencies alternately to detect the transition.
Performance verification
The SAHM was subjected to shock, sinusoidal, and random vibration tests to ensure it comply China’s space station reliability requirement. Figure 7 shows the complete SAHM unit, which is covered by thermal insulation. After the mechanical test, the frequency stability and phase noise of SHAM are measured, as shown in Figures 8, 9. In Figure 8, the reference is a full-size active hydrogen maser designed and developed by BIRMM. There are no significant changes in the SAHM before and after. Phase noise, temperature sensitivity, and other parameters of the SAHM are shown in Table 2.
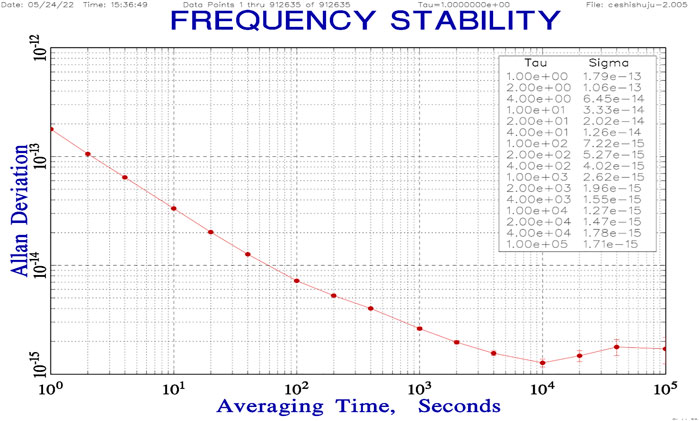
FIGURE 8. Frequency stability measurement results after the mechanical test (the frequency reference is a full-size active hydrogen maser).
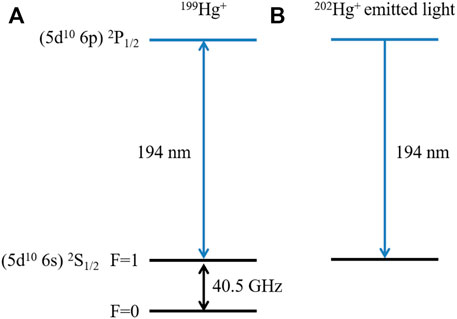
FIGURE 9. Simplified energy levels of 199Hg+. (A) The hyperfine structure of 199Hg+ ions. The 62S1/2 (F = 0) and 62S1/2 (F = 1) hyperfine ground states are the upper and lower clock states. (B) The 194 nm light emitted by the 202Hg+ ions.
In conclusion, a new compact active hydrogen maser is introduced. Because of the reduced volume of the microwave cavity, overall size and weight have significantly reduced. Therefore, it is more suitable for portable or space-born applications. The latest stability of this SAHM maser developed by BIRMM is 1.79 × 10–13 at 1 s and 1.71 × 10–15 at 1 day.
Mercury ion microwave clock
In order to meet the needs of the next generation satellite navigation system, the mercury ion microwave clock has been studied at BIRMM. The mercury trapped ion clocks have many advantages for miniaturization and performance [23, 24]. The 40.5 GHz hyperfine transition (62S1/2, F = 0–62S1/2, F = 1) frequency is the highest among the existing microwave clocks, which provides a high-quality factor for short-term performance. The trapped 199Hg+ can be pumped by mercury discharge lamps and cooled by Helium gas. Thus, this ion clock is relatively easy to be miniaturized. The ions are trapped by four short rods forming a linear trap. The first-order Doppler Effect can be ignored according to the Lamb-Dicke effect. A getter pump is enough to maintain the vacuum pressure without an ion pump. These advantages make the mercury-trapped ion clocks a potential candidate for satellite navigation and deep space exploration [25, 26]. The mercury-ion Deep Space Atomic Clock at NASA was launched into low earth orbit in 2019 [27]. This first-generation mercury ion clock for deep space demonstration has a volume, mass, and power of 17 L, 16 kg, and 47 W, respectively. Clock performances with fractional frequency stability of 3 × 10–15 at 1 day and 3 × 10–15 at 23 days [27] have been measured on orbit (no drift removed). Several institutions in China are engaged in the research of mercury ion clocks. The representative institutes mainly include BIRMM and The Innovation Academy for Precision Measurement Science and Technology (APM) of the Chinese Academy of Sciences (CAS). The two groups have rapidly progressed and preliminarily completed closed loop locking [28, 29].
A202Hg+ discharge lamp optically pumps the trapped 199Hg+ ions. The 202Hg+ 194 nm transition is fortuitously resonant with the transition of 199Hg+ 62S1/2 (F = 1) − 62P1/2 as shown in Figure 9. The ions decay from the 62P1/2 state to the two hyperfine states (62S1/2, F = 0 and 62S1/2, F = 1). The 194 nm continues illumination populates the 199Hg+ ions to the lower hyperfine state (62S1/2, F = 0). Applying resonance radiation at the hyperfine frequency of 40.5 GHz restores the population of the upper state (62S1/2, F = 1).
A mercury-trapped ion clock consists of four parts: a mercury lamp, an ion trap vacuum, a 40.5 GHz microwave source, and related control electronics. The basic scheme is shown in Figure 10. Mercury atoms are generated from a mercury oven when heated to 280°C, and mercury ions are achieved via electron bombardment produced by an electron gun. An 1800-V peak-to-peak voltage at 1 MHz is generally applied to two diagonal trap rods, and the remaining two rods are grounded. A 20 V DC voltage is loaded on the end cap electrodes. A getter pump maintains the vacuum of the ion trap. A 194 nm mercury discharge lamp completes mercury ions’ pumping and state preparation. A 40.5 GHz microwave generated by the microwave frequency synthesizer is used to stimulate the clock transition. The trapped 199Hg+ ions emit a 194 nm fluorescence signal. The signal is detected by a photomultiplier, and then the data is obtained by a photon counting module. The local quartz oscillator is locked to the clock transition of the trapped 199Hg+ ions by the servo system to output a stable 10 MHz standard frequency signal.
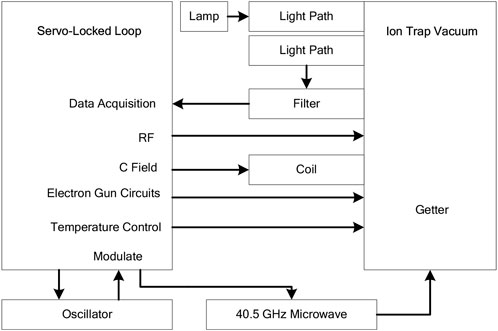
FIGURE 10. The basic scheme of the mercury ion microwave clock. The mercury ions are trapped by the linear ion trap inside a vacuum tube. A 40.5 GHz microwave source is used to excite the ions from the lower clock state to the upper clock state. The servo-locked loop locks the oscillator to the 199Hg+ clock transition.
The mercury lamp used for optical pumping and state detection may limit the ultimate stability of the clock. Many experiments have been done to optimize the lamp. A mercury lamp includes a bulb, an RF circuit, and a temperature control circuit. The bulb’s design includes the material, shape, argon pressure, and mass of mercury. The model of bulb material is JGS1. The shape of the designed bulb is shown in Figure 11 [30].
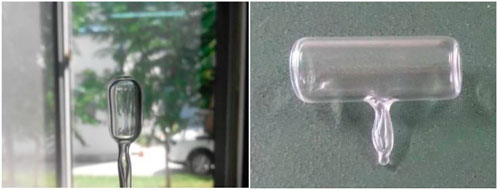
FIGURE 11. Photos of the bulb. The difference between them is the position of the cold end. Different bulbs are selected according to different structural designs.
Many efforts have been made based on varied argon pressures to achieve a perfect mercury bulb, and the experiment results shown in Figure 12 show a 194 nm radiation peak at specific argon pressures. The intensity of the 194 nm spectrum is maximum when the argon pressure is 1 Torr. Then an RF excitation circuit and a temperature control circuit are completed. Finally, a compact mercury lamp is achieved, as shown in Figure 13.
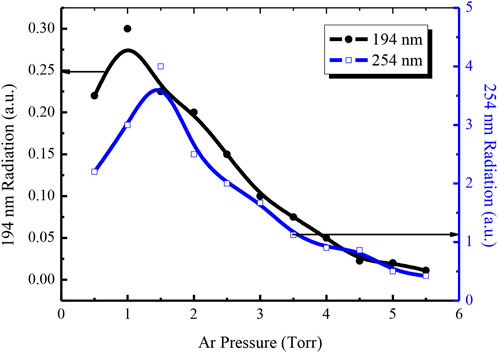
FIGURE 12. The light power of 194 nm (black) and 254 nm (blue) changing with varying argon pressure in a 14 mm diameter bulb. The y-axis’s unit is arbitrary. A calibrated gauge estimates the argon pressure, and a grating-spectrometer analyzes the light intensity.
The resonance curve is measured directly by frequency scanning, as shown in Figure 14. The frequency is stepped in 0.1 Hz increments. The linewidth of the clock transition is about 1 Hz, corresponding to a Q factor of 4 × 1010. The maximum photon counts reach 45000 when the PMT sampling time is 1s. Stepping the oscillator 0.5 Hz to either side of the difference in fluorescence rates allows the 40.5 GHz oscillator to be locked to the 1 Hz broad resonance line. Finally, the clock with frequency stability of 4 × 10–13 at 1 s and 4 × 10–14 at 1000 s is achieved. A hydrogen maser built in BIRMM provides the reference frequency. Since no magnetic field is applied to the trap, the Allan variance data has not been measured beyond 1000 s in this preliminary test.
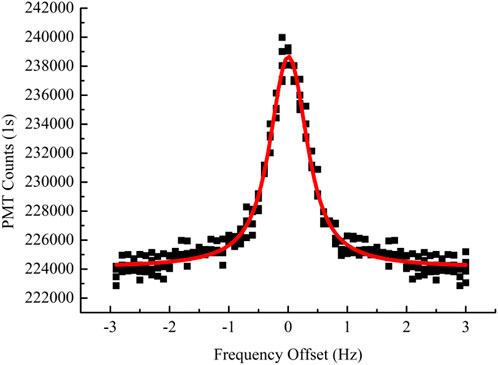
FIGURE 14. The clock microwave resonance signal is measured as ion fluorescence at 194 nm vs. microwave frequency applied to ions in the 4-pole trap. The center frequency is about 40.507348 GHz.
Many issues still need to be addressed to improve the mercury microwave clock. The lamp has a finite lifespan and needs to be enhanced. In order to attain improved clock stability, the SNR must be increased. Additionally, the servo control system requires optimization.
Summary
The quantum frequency standard is the key to time measurement and satellite navigation. This paper established the experimental system of thermal atomic beam optical frequency standard. The miniaturization and practicality of optical frequency standards are being realized by reducing the number of lasers and the system’s complexity. For the construction of China’s space station and related space science research, the world’s first NMT-loaded active hydrogen maser has been developed, with a stability of 1.27 × 10–15 at 10000 s and 1.7 × 10–15 at 100000 s. A mercury ion microwave frequency standard is studied and constructed to meet the time synchronization requirements of deep space exploration and navigation in the future. The stability is 4 × 10–13 at 1 s and 4 × 10–14 at 1000 s. The above technical researches and breakthroughs will further drive the development of quantum frequency standards and quantum precision measurement technology.
Data availability statement
The original contributions presented in the study are included in the article/supplementary material, further inquiries can be directed to the corresponding authors.
Author contributions
SZ and JG contribute to Section 1, 5 and is responsible for the whole paper. XX contributes to Section 2, TZ contributes to Section 3, and NW contributes to Section 4. They are all responsible for the data, figures, and conclusions.
Acknowledgments
Prof. Yiqiu Wang and the authors of this work have been close friends for more than 10 years. The authors have learned a lot from Prof. Wang throughout their 10 years of collaboration and communication. For their research on atomic clocks and time measurement, authors most frequently refer to Prof. Wang’sbook Principle of Quantum Frequency Standard. Prof. Wang, who is in his 90’s, not only provided the authors with lectures on scientific knowledge but also with an attitude of diligence in thinking and writing. We sincerely wish Prof. Wang to be healthy and happy.
Conflict of interest
The authors declare that the research was conducted in the absence of any commercial or financial relationships that could be construed as a potential conflict of interest.
Publisher’s note
All claims expressed in this article are solely those of the authors and do not necessarily represent those of their affiliated organizations, or those of the publisher, the editors and the reviewers. Any product that may be evaluated in this article, or claim that may be made by its manufacturer, is not guaranteed or endorsed by the publisher.
References
1. McCarthy DD, Seidelmann PK. Time: From earth rotation to atomic physics. 2nd ed. Cambridge: Cambridge University Press (2018).
2. Göbel EO, Siegner U. The new international system of units (SI): Quantum metrology and quantum standards. Weinheim: Viley-VCH Verlag GmbH & Co (2019).
3. Xue X, Zhang Z, Zhang X, Zhao H, Wang N, Chen X, et al. A compact design of a transportable calcium optical frequency standard. In: Proceedings of 2018 IEEE international frequency control symposium (IFCS) (2018). p. 288–90. doi:10.1109/fcs.2018.8597454
4. Olson J, Fox RW, Fortier TM, Sheerin TF, Brown RC, Leopardi H, et al. Ramsey-bordé matter-wave interferometry for laser frequency stabilization at 10^{-16} frequency instability and below. Phys Rev Lett (2019) 123:073202. doi:10.1103/PhysRevLett.123.073202
5. Zhou T, Wang M, Ren S, Wu Q, Wang X, Zhang C, et al. New research progress in active hydrogen maser in BIRMM. In: J Sun, C Yang, and J Xie, editors. China satellite navigation conference (CSNC) 2020 proceedings: Volume II. CSNC 2020. Lecture notes in electrical engineering, 651. Singapore: Springer (2020). p. 463–71. doi:10.1007/978-981-15-3711-0_41
6. Kaur Gulati G, Chung S, Le T, Prestage J, Yi L, Tjoelker R, et al. Miniatured and low power mercury microwave ion clock. IFCS 2018-IEEE Int Frequency Control Symp (2018). doi:10.1109/FCS.2018.8597538
7. Chung TMS, Thanh Le K, Prestage JD, Yi L, Tjoelker RI, Yu N. Performance of micro mercury trapped ion clock. In: 2019 joint conference of the IEEE international frequency control symposium and European frequency and time forum (2019). p. 1–2. doi:10.1109/FCS.2019.8856065
8. Chung TMSK, Le T, Prestage JD, Yi L, Tjoelker RL, Yu N. Stability demonstration of a 15-cc mercury-ion vacuum trap tube. Proceedings (2020). doi:10.1109/IFCS-ISAF41089.2020.9234925
9. Koller SB, Grotti J, Vogt S, Al-Masoudi A, Dörscher S, Häfner S, et al. Transportable optical lattice clock with 7×10^{-17} uncertainty. Phys Rev Lett (2017) 118:073601. doi:10.1103/PhysRevLett.118.073601
10. Stuhler J, Abdel Hafiz M, Arar B, Bawamia A, Bergner K, Biethahn M, et al. Opticlock: Transportable and easy-to-operate optical single-ion clock. Meas Sensors (2021) 18:100264. doi:10.1016/j.measen.2021.100264
11. Abbasov T, Makarenko K, Sherstov I, Axenov M. Compact transportable 171Yb+ single-ion optical fully automated clock with 4.9×10-16 relative instability (2020). arXiv: Instrumentation and Detectors.
12. Riehle F, Morinagax A, Ishikawaz J, Helmcke J. A calcium frequency standard: Frequency stabilization by means of nonlinear Ramsey resonances. Appl Phys B (1989) 48:165–71.
13. Olson J, Fox R, Brown R, Fortier T, Sheerin T, Oates CW, Ludlow AD. High-stability laser using ramsey-bordé interferometry. In: proceedings of 2017 joint conference of the European frequency and time forum (EFTF) and IEEE international frequency control symposium. Besancon, France: IFCS (2017). p. 32–3.
14. Hemingway B, Taylor J, Swanson TB, Peil S. Preliminary investigation of a calcium thermal beam in order to build an operational optical clock. In: Proceedings of 2018 IEEE international frequency control symposium (IFCS) (2018). p. 277–9. doi:10.1109/fcs.2018.8597569
15. Shang H, Zhang X, Zhang S, Pan D, Chen H, Chen J. Miniaturized calcium beam optical frequency standard using fully-sealed vacuum tube with 10^−15 instability. Opt Express (2017) 25:30459–67. doi:10.1364/oe.25.030459
16. Fox RW, Sherman JA, Douglas W, Olson JB. A high stability optical frequency reference based on thermal calcium atoms. In: 2012 IEEE international frequency control symposium. Baltimore: IFCS (2012)May 21-24. doi:10.1109/fcs.2012.6243750
17. Zhang X, Zhang S, Jiang Z, Li M, Shang H, Meng F, et al. A transportable calcium atomic beam optical clock. In: 2016 IEEE international frequency control symposium (IFCS). New Orleans (2016). doi:10.1109/fcs.2016.7563535
18.BIPM. BIPM recommended values of standard frequencies. calcium (2005). Available at: https://www.bipm.org/documents/20126/41549606/M-e-P_Ca_657.pdf/dd81019b-1286-ceae-e202-afa84556ff05
19. Zhang L, Xiao Q, Chen X, Ge J, Xue X. Performance evaluation of calcium atom beam vacuum system. In: 24th national laser conference & fifteenth national conference on laser technology and optoelectronics (2020). p. 117170V. Proc. SPIE 11717. doi:10.1117/12.2585357
20. Salomon C, Cacciapuoti C, Dimarcq N. Atomic clock ensemble in space: An update. Int J Mod Phys D (2007) 16:2511–23. doi:10.1142/s0218271807011681
21. Cacciapuoti L, Salomon C. Atomic clock ensemble in space. J Phys Conf Ser, 327 (2011). p. 012049. doi:10.1088/1742-6596/327/1/012049
22. Zhou T, Wu Q, Huang J, Gao L. Progress on sapphire hydrogen maser for Beidou navigation system. In: J Sun, W Jiao, H Wu, and C Shi, editors. China satellite navigation conference (CSNC) 2013 proceedings. Lecture notes in electrical engineering, 245. Berlin, Heidelberg: Springer (2013). p. 357–69. doi:10.1007/978-3-642-37407-4_33
23. Hoang TM. Performance of micro mercury trapped ion clock. In: 2019 joint conference of the IEEE international frequency control symposium and European frequency and time forum. Orlando, FL: EFTF/IFCS (2019). p. 1–2. doi:10.1109/fcs.2019.8856065
24. Bandi T, Prestage J, Chung S, Le T. Demonstration of long vacuum integrity lifetime of a trapped ion clock package. Interplanet Netw Prog Rep (2016) 204:1–9.
25. Tjoelker RL, Prestage JD, Burt EA, Chen P, Chong YJ, Chung SK, et al. Mercury ion clock for a NASA technology demonstration mission. IEEE Trans Ultrason Ferroelect., Freq Contr (2016) 63:1034–43. doi:10.1109/tuffc.2016.2543738
26. Ely TA, Burt EA, Prestage JD, Seubert JM, Tjoelker RL. Using the deep space atomic clock for navigation and science. IEEE Trans Ultrason Ferroelect., Freq Contr (2018) 65:950–61. doi:10.1109/tuffc.2018.2808269
27. Ely TA, Seubert J, Prestage J. Deep space atomic clock mission overview. In: AAS/AIAA astrodynamics specialist conference, AAS19-796 (2019). p. 1–20.
28. Liu H, Yang Y, He Y, Li H-X, Chen Y-H, She L, et al. Microwave-optical double-resonance spectroscopy experiment of 199 Hg + ground state hyperfine splitting in a linear ion trap. Chin Phys. Lett. (2014) 31(6):063201. doi:10.1088/0256-307x/31/6/063201
29. Wang N, Chen X, Zhao H. Design of mercury lamp for trapped ion frequency standards. J Astronautic Metrology Meas (2020) 40(3):27–30., No.
Keywords: time and frequency metrology, positioning navigation and timing, quantum frequency standard, optical clock, active hydrogen maser, ion microwave clock
Citation: Xue X, Zhou T, Wang N, Zhang S and Ge J (2022) Recent progress on quantum frequency standards at BIRMM. Front. Phys. 10:971036. doi: 10.3389/fphy.2022.971036
Received: 16 June 2022; Accepted: 09 September 2022;
Published: 30 September 2022.
Edited by:
Xuzong Chen, Peking University, ChinaReviewed by:
Yige Lin, National Institute of Metrology, ChinaRong Wei, Shanghai Institute of Optics and Fine Mechanics (CAS), China
Copyright © 2022 Xue, Zhou, Wang, Zhang and Ge. This is an open-access article distributed under the terms of the Creative Commons Attribution License (CC BY). The use, distribution or reproduction in other forums is permitted, provided the original author(s) and the copyright owner(s) are credited and that the original publication in this journal is cited, in accordance with accepted academic practice. No use, distribution or reproduction is permitted which does not comply with these terms.
*Correspondence: Shengkang Zhang, zhangsk@126.com; Jun Ge, 13466728668@163.com