- 1Department of Chemistry, CICECO—Aveiro Institute of Materials, University of Aveiro, Aveiro, Portugal
- 2Champalimaud Centre for the Unknown, Champalimaud Foundation, Lisboa, Portugal
- 3STFC Rutherford Appleton Laboratory, ISIS Neutron and Muon Source, Chilton, United Kingdom
The effect of water on the physicochemical properties of deep eutectic solvents (DES) is a trending research topic. In this work, inelastic neutron scattering (INS) spectroscopy, was used to probe intermolecular interactions in the water-deep eutectic solvent mixtures for the cases of choline chloride (the hydrogen bond acceptor) and three different hydrogen bond donors, with different degrees of acidity: urea, glycerol and lactic acid. It was found that quenching samples in liquid nitrogen is a procedure that may retain the liquid phase morphology of DES at the low temperatures required by INS spectroscopy. The three studied systems share the preference of water molecules to bind to chloride anion, as predicted by numerous molecular dynamics simulations. Despite this similarity, the three systems present several distinct INS features upon water addition that are related to their unique properties and structure at the molecular level. In the choline chloride:urea system, water molecules promote a strengthening of hydrogen bonds with the NH and OH donors, while for the choline chloride:lactic acid system INS probed the existence of solvated DES clusters instead of specifically interfering water molecules. This study takes advantage from the unique capabilities of INS and paves the way for future studies in these systems.
Introduction
Deep eutectic solvents (DES) are a trending research topic [1–3], largely due to the need for sustainable alternatives to conventional solvents and for their versatility. This opens a wide range of applications, from biomass delignification [4], to electrochemical energy storage [5] and to pharmaceutical formulation [6]. At present, their range of applications keeps growing, arguably much faster than our current understanding of their behaviour. The most studied DES are formed by the association of a hydrogen bond-capable salt with a neutral hydrogen bond donor species. At the eutectic composition, the mixture has a melting point much lower than that of the corresponding ideal mixture. The physicochemical properties of DES are a macroscopic manifestation of the microscopic interactions of its components and this work aims to contribute to the understanding of these fundamental interactions.
A matter that emerges from a large number of experimental reports is the effect of water on the properties of DES. It has become evident that the effect of water on the physicochemical properties and on the structural features of DES has important practical implications [7–24]. Water addition promotes structural changes on DES at the microscopic level and these changes are not yet fully understood. Recent results clearly demonstrated that there are three main DES-water regimes: summarised as “low water”, “medium water”, and “high water” contents [7–9, 14, 15]. Even small amounts of water (0–15% w/w) change the heterogeneous 3D structures of the pure components [7]. Up to about 50% (w/w) of water added, the strong interactions between the two components of the DES weaken gradually, but the supramolecular structures are to some extent preserved [8, 15]. Subsequent water additions led to the progressive disappearance of such interactions, until the mixture becomes a “simple” aqueous solution. Some studies propose that the passage from the water-in-DES system to a DES-in-water system occurs at a certain hydration level [2]. These questions are of utmost importance when considering the interactions of DES with active pharmaceutical ingredients (APIs), particularly the effects of DES on API solubility and bioavailability, a broader interest which this work falls in [6]. In fact, understanding the grounds of DES-water interactions is a key step to assess the principles that govern the increased API solubility in API-DES-water systems.
One technique to characterize the structure and dynamics of bulk materials at a molecular level that is gaining momentum is inelastic neutron scattering (INS) spectroscopy. INS spectroscopy provides a unique assessment of the structural dynamics of hydrogenous materials that is not amenable from its optical counterparts, infrared and Raman spectroscopies, as it is not constrained by symmetry-related selection rules [25]. In INS, the intensity of each vibrational transition is expressed, for a given atom, by the dynamic structure factor:
where Q (Å−1) is the momentum transferred to the sample, n is the quanta involved (n = 1 for fundamental modes), νk is the energy of a vibrational mode, Ui (Å) is the displacement vector of atom i in mode k, σ is the neutron scattering cross section of atom i and αi (Å) is related to a mass-weighted sum of the displacements of the atom in all vibrational modes, a term whose magnitude is in part determined by the thermal motion of the molecule [26]. This means that the intensity of a band associated with a given vibrational mode is proportional to the amplitude of nuclei displacement and to neutron scattering cross section of the moving nuclei, both of which are particularly large for hydrogen atoms. Thus, while there are no selection rules, there is a strong “propensity rule” that modes involving hydrogen motion will dominate the spectrum. Low frequency vibrations, such as the torsion of a methyl group or an intermolecular mode in a solid, typically involve large amplitude motions of the hydrogen atoms and generate intense INS bands. This makes INS particularly suited to assess low energy vibrational motions with high sensitivity.
This work aims to foster new insights on the role of water molecules in DES systems, taking advantage of the unique capabilities of INS spectroscopy described above. The DES systems selected are based on choline chloride (ChCl, the hydrogen bond acceptor) and three different hydrogen bond donors, with different degrees of acidity: urea (basic), glycerol (neutral) and lactic acid (acidic) (Figure 1 and Table 1).
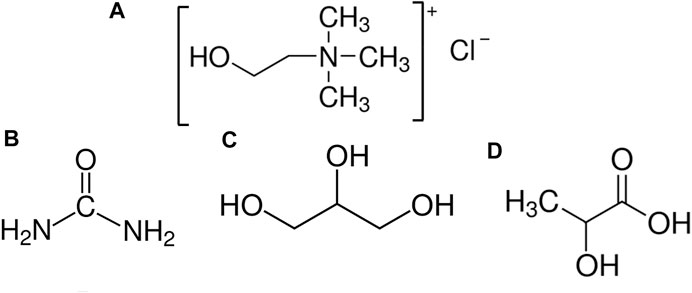
FIGURE 1. Schematic representation of DES components: (A) Choline chloride (ChCl); (B) Urea; (C) Glycerol; (D) Lactic acid.
ChCl and urea, mixed in a 1:2 M proportion, form the iconic deep eutectic solvent known as “Reline”, probably the most studied DES system and whose structure was already assessed through INS spectroscopy [28]. The similar 1:2 mixture of ChCl and glycerol (often known as “Glyceline”) is also of interest, mainly due to its low viscosity properties [27, 29]. The ChCl: lactic acid 1:1 mixture was originally included in the study under the broader interest on the interactions of APIs with DES systems: the solvation of the API lidocaine on this DES has been studied using DFT and molecular dynamics calculations [30], thus providing additional information for the interpretation of spectroscopic data. The DES:water systems were prepared with molar ratios up to 1:5, which correspond to a maximum of ca. 30% water content (water mass/total mass), falling within the “low–medium water” regions. Higher water contents are not advisable due to the overwhelming intensity of ice in the INS spectrum.
This work also addresses the question on the nature of samples probed by INS spectroscopy. Due to the blurring consequences of the Debye-Waller factor at high temperatures (the exponential term in Eq. 1), INS spectra are recorded at very low temperatures, typically below 20 K. Hence, the use of INS spectroscopy to study DES assumes that it is possible, to a large extent, to preserve the room-temperature morphology of the liquid phase at these low temperatures. To this end, samples are usually quenched in liquid nitrogen (“shock frozen”), at ca. 77 K, prior to placement inside the cryostat in which the spectrum is collected. The sudden temperature drop, combined with the high viscosity of DES, is expected to prevent crystallization or phase separation in the sample at low temperature and preserve a liquid-like organization. Although the formation of glassy (non-crystalline) structures from quenching the sample in liquid nitrogen has been reported for molecular liquids (e.g., water [31] and ionic liquids [32]), to the best of our knowledge the procedure has not yet been tested/proved on DES.
Experimental Details
Sample preparation: Cholinium chloride, urea, glycerol and lactic acid were obtained commercially: ChCl (≥99% purity, Sigma-Aldrich), urea (≥99% purity, Sigma-Aldrich), glycerol (≥99% purity, Sigma-Aldrich) and DL-lactic acid (≥85% purity, Acros Organics). The DES ChCl:urea (1:2), ChCl:glycerol (1:2) and ChCl:lactic acid (1:1) were all prepared, by the heat method. Prior to preparation of dry mixtures, the individual components were dried during 5 days under vacuum at 60°C and up to a constant weight by using phosphorus pentoxide. After dried the components were weighted inside a glovebox into sealed glass vials and placed under constant stirring and temperature (up to 80°C). After obtaining a homogeneous transparent liquid, the mixtures were then kept for 1 h at this maximum temperature and allowed to return to room temperature. DES-water mixtures were prepared from these by addition of water in the appropriate molar amount, with DES:water molar proportions of 1:1, 1:2, 1:3, and 1:5. For the sake of simplicity, these samples are referred to in the text below by 1, 2, 3 and 5w, respectively. Due to the highly hygroscopic character of ChCl, the dry samples, nominally 1:0 DES:water molar ratio (or 0w), were determined (from Karl-Fisher titration) to have 0.8% water in total mass in the case of ChCl:Urea and 0.6% water in the case of ChCl:glycerol. In the case of ChCl:lactic acid system, the nearly dry mixture did not produce a clear solution, and only 1, 3, and 5 samples are reported.
Spectra acquisition: The INS spectra were collected using the TOSCA [26, 33–35] and MAPS [26, 36] time-of-flight spectrometers at the ISIS Neutron and Muon Source of the STFC Rutherford Appleton Laboratory (Chilton, United Kingdom). Each sample, weighing ca. 0.7–1.0 g, was placed inside a flat thin-walled aluminium can, which was then mounted perpendicular to the incident beam using a regular TOSCA center stick. Eutectic mixtures, in the liquid state, were “shock-frozen” by quenching samples in liquid nitrogen before placement in the beam path, in order to avoid phase separation and preserve the room-temperature morphology of possible amorphous and crystalline regions. For the slow cooling sample, a simple procedure was adopted: the sample filled aluminium can was wrapped in an isolating film to slow down heat transfer and placed at 277 K (4°C) for 12 h and then at 255 K (−18°C) for 24 h before, “shock freezing” and placing in the TOSCA cryostat. Spectra were collected below 15 K, measured for the 0 to 8,000 cm−1 (TOSCA) and the 0 to 6,000 cm−1 (MAPS) energy-transfer ranges. In MAPS, three incident energies were used (807, 2016 and 5,243 cm−1) in order to accurately observe the low and high frequency ranges. Data was converted to the conventional scattering law, S (Q,ν) vs energy transfer (in cm−1), using the MANTID program (version 4.0.0) [37].
Most of the INS spectra of DES samples and the INS spectrum of pure lactic acid were recorded during project’s RB1920244 mission [38], while the INS spectra of remaining pure compounds were obtained in previous projects: ChCl (RB1610481 [39]) and glycerol (TOSCA XPRESS XB1890136 [40]). The TOSCA spectrum of urea was previously recorded by Johnson and colleagues [41] and is available at the INS database [42]. The INS spectra of dry “Reline” (slow cooling vs. shock freezing) were obtained within project RB1810054 [43]. The MAPS spectra of ChCl:urea:water were recorded within project RB1620171 [44].
In order to emphasize the spectral differences resulting from the addition of water to DES samples, difference spectra were obtained by subtracting the spectrum of the driest sample from those of wet samples. Since the signal strength of INS is mostly a quantitative measurement to the hydrogen content of the samples (hydrogen has an incoherent scattering length almost two orders of magnitude larger than that for nitrogen, carbon, oxygen, and chlorine), the subtraction procedure just requires an adequate weighting of both spectra to match the band intensities of DES components (i.e., the integrated “difference spectra intensity” for the DES components over a water-free range should be zero).
Results
Quenching vs. Slow Cooling
Figure 2 compares the INS spectra of ChCl:urea 1:2 eutectic mixture subjected to “shock freezing” and to a “slow freezing” procedure. As it can be seen, the “shock frozen” and “slow freezing” samples produced drastically different spectra. While the shock frozen spectrum presents the broad features consistent with an amorphous sample, the slow freezing spectrum shows much sharper bands related to those from crystalline ChCl and also some new ones, indicated by arrows (Figure 2, slow cooling). The new, sharp features must result from a new structural organization and can be ascribed to the presence of the co-crystal previously found by X-ray powder diffraction [45]. This a very important result, as it validates the quenching of samples in liquid nitrogen as a reliable procedure to prevent crystallization or phase separation in the DES sample at low temperature and to preserve a liquid-like organization.
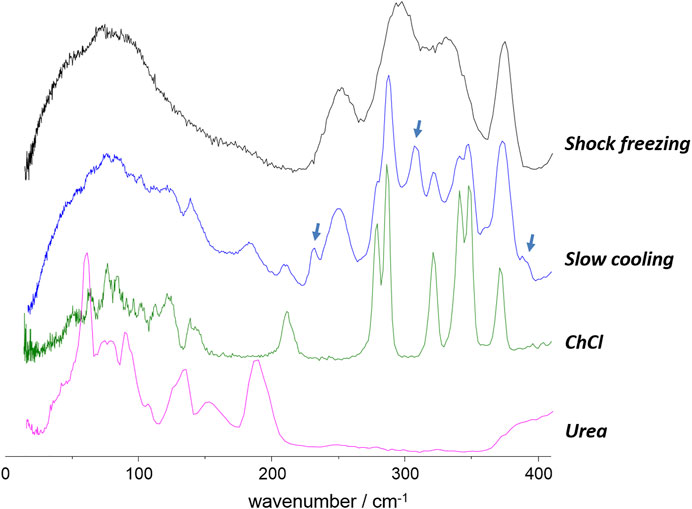
FIGURE 2. Comparison between the INS spectra of ChCl:urea DES in the 0–400 cm−1 region. From top to bottom: “shock freezing” vs. “slow cooling” and pure components, ChCl and urea. The arrows identify sharp bands not directly related to pure components.
Effect of Water in ChCl:Urea DES
The effect of water increase in the INS spectra of the eutectic mixture of ChCl:urea is shown in Figure 3, for the 0–1,000 cm−1 range (as mentioned in the experimental section, the sample that is nominally 1:0 DES:water molar ratio (labelled 0w), was determined to have 0.8% water in total mass).
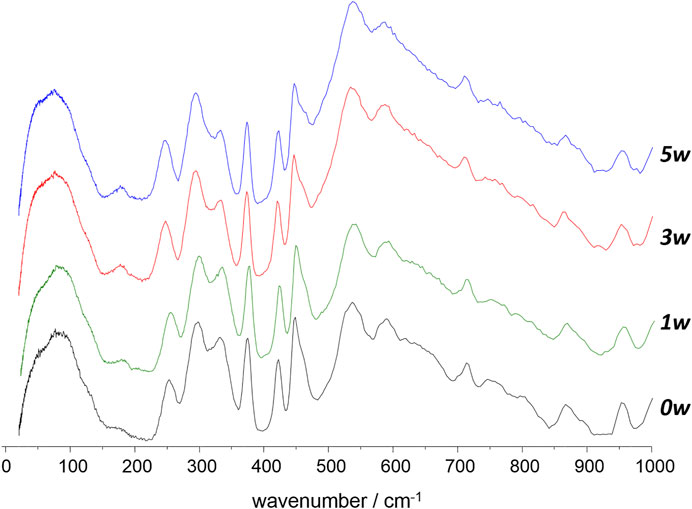
FIGURE 3. INS spectra of ChCl:urea eutectic mixture with increasing water content, in the 0–1,000 cm−1 range. From bottom to top: DES:water molar ratio of 1:0 (ca. 0.8%water wt, 0w), 1:1 (ca. 6.5% wt, 1w), 1:3 (ca. 17.0% wt, 3w), and 1:5 (ca. 26.0% wt, 5w).
As can be seen, the presence of water induced several changes, apart from the expected increase in the region above ca. 500 cm−1, which arises from the librational modes of water molecules and were observed in several water-containing samples [46]. The band at ca. 175 cm−1 consistently increases with water content and is assigned to the intermolecular stretching motion (also called anti-translational mode [47]) of the water molecule hydrogen-bonded to DES components. The region around 300 cm−1 is dominated by the methyl torsional modes (τ1, τ2, τ3) of ChCl [28], at ca. 252, 296 and 333 cm−1, respectively. They are virtually unchanged by the addition of one molecule of water (0w and 1w spectra in Figures 3, are nearly identical in this region). However, addition of three molecules gives rise to a shift of the lower wavenumber band (from 252 cm−1 to 246 cm−1) and to a change of the intensity ratio between the 333/296 cm−1 bands. Further addition of water, up to the 1:5 M ratio (5w), does not change the position of the lower wavenumber band, but deepens the decrease in intensity ratio for the bands at higher wavenumber.
The effects on the region of methyl torsional modes are highlighted in Figure 4, which shows the difference spectra obtained by subtracting the spectrum of 0w sample from the spectra of water-containing samples, in the 200–400 cm−1 range. The negligible effect of the addition of one water molecule in this region is evident from the difference 1w-0w spectrum (Figure 4, bottom line). The shift of the τ1 band for higher water contents (3w and 5w) is confirmed by the “band derivative” profile centered at ca. 250 cm−1 observed in the corresponding difference spectra. On the other side, the intensity changes of τ2 and τ3 are in fact associated with the loss of a broad intensity complex profile centered at ca. 340 cm−1 and spanning from 280 up to 400 cm−1.
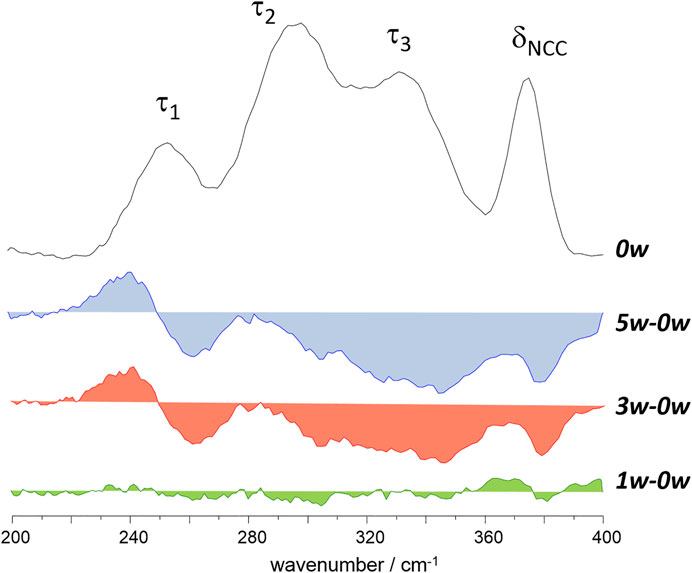
FIGURE 4. A close-up of the region of methyl torsions in the ChCl:urea mixture: the difference spectra (wet minus dry) for the DES:water molar ratios of 1:1 (1w-0w), 1:3 (3w-0w), and 1:5 (5w-0w), compared with the spectrum of the dry sample (0w).
For the region of 1,000–2,000 cm−1, a similar procedure of subtracting spectra unveils a few changes, as shown in Figure 5. The main changes observed in this region are proportional to the water content, namely, the intensity loss at ca. 1,450 cm−1, and the intensity gain centred at ca. 1,320 and 1700 cm−1. In a previous assignment of this system [28], based on discrete and periodic DFT calculations using a model cluster with two ChCl ion pairs and four urea molecules, the band at ca. 1,450 cm−1 was found to arise from CH2 and CH3 deformations, with significant contributions from OH bending modes of hydrogen bonded OH groups (OH···Cl and OH···O=C). The bending modes of free OH groups are expected to occur at lower wavenumber and stronger bonding would give rise to intensity at higher wavenumber. The intensity changes revealed by the difference spectra in Figure 5 are consistent with that situation and may be ascribed to the strengthening of the hydrogen bonding with choline’s hydroxyl group.
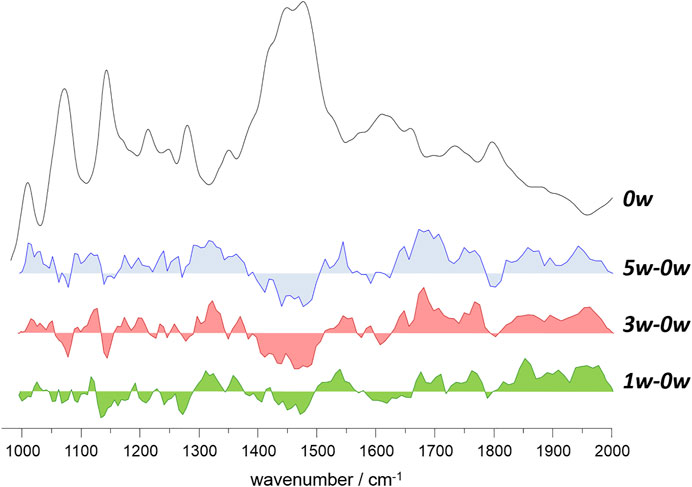
FIGURE 5. INS spectra of ChCl:urea mixture: the difference spectra (wet minus dry) for the DES:water molar ratio of 1:1 (1w-0w), 1:3 (3w-0w), and 1:5 (5w-0w), compared with the spectrum of the dry sample (0w).
The TOSCA spectrometer is optimal in the low wavenumber region, degrading in resolution with increasing energy transfer. The MAPS instrument allows a better description of the high wavenumber region. In particular, MAPS allows assessing the regions of the NH2 bending modes and NH/OH stretching modes. The spectra recorded with MAPS for the 1,200–1800 and 2,800–3,800 cm−1 ranges are shown in Figure 6. The first region (1,200–1,800 cm−1, Figure 6A) has been used to identify the NH2 bending modes at ca. 1,620 cm−1 [28]. Upon hydration, this intensity becomes less defined, with a possible shift to higher energy. This behavior was predicted from MD simulations and ascribed to a more heterogeneous environment of the NH2 groups and stronger hydrogen bonds involving the water molecules [11]. Pietro et al. [21], also relate the red shift of the NH2 rocking band observed in Raman spectra with new hydrogen bond interactions of urea with water. In the second region (2,700–3,800 cm−1, Figure 6B), there are two main broad features, assigned to the CH and NH + OH stretching modes, centered at ca. 3,000 and 3,400 cm−1, respectively. The addition of water results in an increase of the OH stretching intensity relative to that of the CH stretching oscillators. A more interesting effect is revealed by the analysis of band contributions, as shown in Figure 6B, bottom. The addition of the first water molecule leads to a general increase of the band intensity, nearly keeping the relative importance of each component. The second water molecule results in a significant increase of the lower energy component at ca. 3,300 cm−1 relative to the component at ca. 3,400 cm−1 (arrows in Figure 6B). Keeping in mind the limitations of a band fit analysis, an intensity increase in the lower wavenumber side of the NH/OH stretching band indicates an increase of the hydrogen-bond strength in the system.
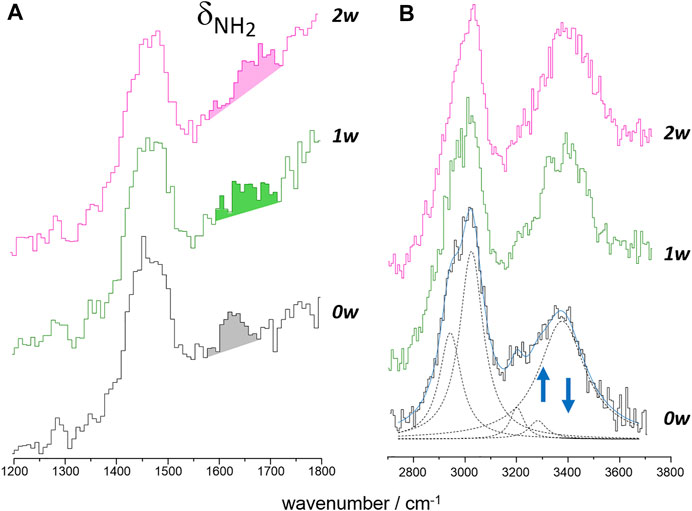
FIGURE 6. INS spectra of ChCl:urea:water mixtures recorded using MAPS, with an incident energy of 2016 cm−1 [0 ≤ q ≤ 9 Å−1, (A)] and 5,243 cm−1 [0 ≤ q ≤ 12 Å−1, (B)]. In panel B, the dashed lines represent the contributions to the best fit adjustment of the 0w spectrum and the arrows indicate the observed intensity changes upon hydration (best fit lines are omitted for 1w and 2w spectra for simplicity).
Effect of Water in ChCl:Glycerol DES
Figure 7 compares the INS spectra of the ChCl:glycerol mixture with increasing water content, from 0w to 5w. The INS spectrum of pure glycerol is also shown (Figure 7, bottom), with intensity scaled to fit the visible contributions in the spectrum of the dry sample (Figure 7, 0w). Figure 8 presents the difference spectra (obtained as in Figures 4, 5) for the 200–400 and 1,000–2000 cm−1 intervals.
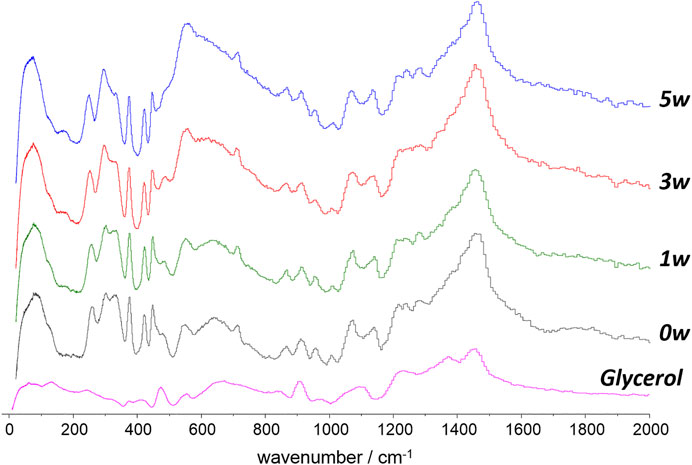
FIGURE 7. INS spectra of glycerol and the ChCl:glycerol eutectic mixture with increasing water content, in the 0–2000 cm−1 range. From bottom to top: glycerol, DES:water molar ratio of 1:0 (ca. 0.6% wt, 0w), 1:1 (ca. 5.3% wt, 1w), 1:3 (ca. 14.3% wt, 3w), and 1:5 (ca. 21.7% wt, 5w).
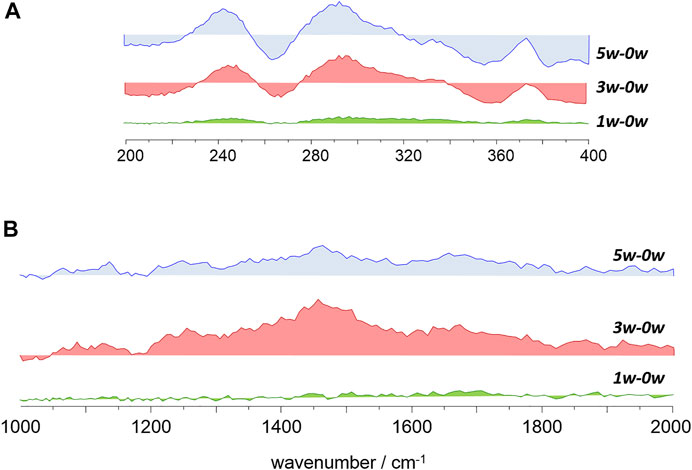
FIGURE 8. INS difference spectra (wet minus dry, nw-0w, n = 1, 3, 5) of the ChCl:glycerol mixture, for the 200–400 cm−1 (A) and 1,000–2000 cm−1 (B) regions.
For this system, the presence of water is clearly evidenced through the librational modes above ca. 500 cm−1. The band assigned to the water … DES hydrogen-bond stretching mode is observed at ca. 169 cm−1, slightly below its position in the ChCl:urea system. On the other hand, the position of the τ1 band displays a nearly linear dependence on the number of water molecules: it shifts from ca. 259 cm−1 (0w) to ca. 256 cm−1 with the first water molecule added, and then to 252 cm−1 (3w) and 248 cm−1 (5w). As in the case of the previous system, water addition leads to the decrease of the τ3/τ2 intensity ratio. Yet, the change in the intensity ratio is now explained by an intensity increase of the τ2 mode, without significant changes for the τ3 mode.
An interesting effect is evidenced in Figure 7 for the higher wavenumber region, particularly considering the band maxima observed in the 1,200–1,300 cm−1 range (and assigned to choline’s CH3 rocking modes [48]). These band maxima are manifest for 0w and 1w spectra and are blurred upon further hydration, in the 3w spectrum. However, they reappear in the 5w spectra, with a general profile nearly identical to that observed for low hydration levels. The difference spectra shown in Figure 8B also emphasises this effect. The region of 1,000–2000 cm−1 (Figure 8B) is nearly insensitive to the addition of the first water molecule, but evidences a strong intensity increase in the region centred at ca. 1,500 cm−1 and spanning ca. 1,200–1800 cm−1, for the 3w sample. However, the intensity in this region reduces upon further addition of water, as it can be seen from the 5w-0w difference spectra. This spectral region has contributions from CH2 and COH bending modes of both glycerol, and ChCl [28]. These modes are highly mixed, but the general wavenumber trend is COH(free) ≈ CH2twist < CH2wag < CH2scissor. Hydrogen-bonding can occur between OH groups or between OH groups and water or the Cl− anion and shifts the COH mode to the higher wavenumbers.
Effect of Water in ChCl:Lactic Acid DES
In the case of the eutectic mixture ChCl: lactic acid it was not possible to obtain the INS spectrum of the dry (or nearly dry) sample. Figure 9 presents the INS spectra of the wet samples (1w, 3w and 5w) and the INS spectra of lactic acid (scaled to provide a visual fit with the corresponding bands in the DES spectra).
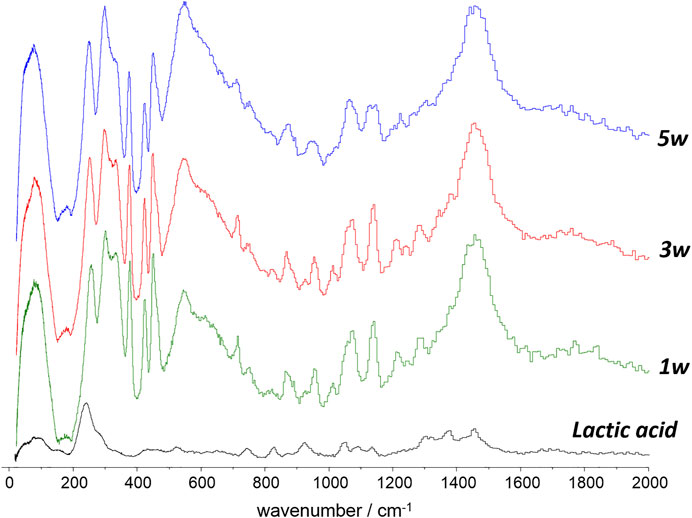
FIGURE 9. INS spectra of lactic acid and the ChCl:lactic acid eutectic mixture with increasing water content, in the 0–2000 cm−1 range. From bottom to top: lactic acid, DES:water molar ratio of 1:1 (ca. 7.3% wt, 1w), 1:3 (ca. 19.0% wt, 3w), and 1:5 (ca. 28.2% wt, 5w).
As in the previous systems, the presence of water is observed through the intense H2O librational modes (above ca. 500 cm−1) and the less intense band at ca. 176 cm−1, assigned to the water … DES hydrogen-bond stretching mode. This value of 176 cm−1 is close to the one observed for ChCl:urea system. In fact, in the low wavenumber region, the effects of water addition to the ChCl:lactic acid system mimics that seen for the ChCl:urea system: the difference spectrum 5w-1w of ChCl:lactic acid (not shown) is identical to the corresponding 5w-0w for ChCl:urea (Figure 4).
A different situation arises for the higher wavenumber regions. In the 600–1800 cm−1 range the effect of water addition is scant, even considering that comparison cannot be done with the dry form. The INS spectra of 1w and 3w samples in this region are nearly identical and their difference spectrum falls within the experimental error. However, further addition of two water molecules result in a clear broadening of the well-defined bands in this region, namely those observed at 713, 870 and 955 cm−1 (νNC modes [28]), and 1,011, 1,068, 1,140, 1,215, and 1,284 cm−1 (mainly choline’s ρCH3 modes [28]). No changes are observed for the band profile centred at ca. 1,450 cm−1 (mainly choline’s CH2 and CH3 deformation, with contributions from COH deformation modes [28]).
Discussion
Looking at all three systems together, and to the spectral changes observed upon water addition (e.g., Figures 3, 6, 7), some similarities can be highlighted. For instance, all the samples show an intensity increase centred at ca. 500–550 cm−1, that is proportional to the water content. It has been reported that for samples with low water content (up to 20–30% of the dry mass) the presence of water molecules becomes evident in INS through a broad asymmetric band, spanning roughly from 400 to 800 cm−1 [46]. This broad band arises from the librational modes of the water molecules interacting with the DES components and its intensity and profile is markedly different from the one observed for ice crystals. This supports the absence of water clusters at these hydration levels, since the presence of water clusters would result in ice microcrystals upon freezing and the ice crystals have an unambiguous spectral signature [28, 49].
The presence of water is also evident in the three systems from the INS band at ca. 169–175 cm−1. Due to its intensity dependence on water content and its low wavenumber position, this band is tentatively assigned to the stretching motion (also called anti-translational mode) of the hydrogen bond formed between water molecules and DES components, in the specific geometry of the formed complex. The differences in the position of the band maxima for the three systems suggests that these specific water interactions are somewhat weaker for the ChCl:glycerol system than for the ChCl:urea and ChCl:lactic acid mixtures.
Other similarities can be found for bands arising from torsional motions of the choline’s methyl groups, labelled τ1, τ2 and τ3 in Figure 4. Methyl torsions give rise to strong INS intensities, and so these modes dominate the 200–300 cm−1 spectral range for the three systems herein considered. Upon hydration, τ1 mode is red-shifted while the ratio τ3/τ2 decreases. The red-shift of τ1 mode has been observed for situations in which there are competitive hydrogen bond donors for the chloride anion [28, 50]. In pure ChCl, all the methyl groups of choline are hydrogen-bonded to chloride anions (CCDC database, RefCode:CHOCHL01), and the methyl torsional motions are restricted. In the presence of alternative hydrogen bond donors, such as urea [28] or matrix donors [50], chloride anions are displaced and some methyl groups became freer. Consequently, methyl torsions move to lower wavenumbers. Results from molecular dynamics (MD) simulations on the hydration of DES systems [9, 11–13, 19] point out that water molecules tend to preferentially bond to the chloride anion. This water-chloride bonding promotes the release of more methyl groups from the methyl-chloride interaction, thus contributing to the observed red-shift of the τ1 mode.
Although the general trend of the methyl torsion bands upon hydration is the same for the three systems, the behaviour is not identical. In particular, the red-shift of the τ1 band in the urea-based DES is only observed for the 3w and 5w samples, while for the glycerol-based DES the effect is evident from 1w and the red-shift up to the 5w sample is larger. In the same region, the difference spectra shown in Figures 4, 8A also evidence the distinct origin of the change in τ3/τ2 ratio for these systems. The molecular origin of this effect cannot be obtained from these results alone and requires further investigation. For the lactic acid-based DES, the difference spectra in this region are identical to those observed for ChCl:urea, an observation that singles out glycerol among the three H-bond donors. The different behaviour of ChCl:urea and ChCl:glycerol was previously reported by Pandey and Pandey [51], who propose that while water molecules in the ChCl:urea system tend to prefer an interstitial accommodation, water molecules in ChCl:glycerol will preferentially bind to glycerol and/or ChCl. This different behaviour is corroborated by other authors, but on different grounds. For ChCl:glycerol DES, Ahmadi et al. [52], using Raman and infrared spectroscopies followed by multivariate analysis, concluded that the stoichiometry of the DES–water association is 1:9, a value which exhibits the domination of DES–water interactions over DES–DES interactions. From MD simulations, Weng and Toner [53] stated that initial addition of water strengthens the direct hydrogen bonding between ChCl and glycerol, but the general trend is the gradual decrease in the overall number of interactions between choline and glycerol with invreasing water content. For ChCl:urea DES, Hammond et al. [14] used neutron diffraction to analyse the effect of water in and conclude that it contributes slightly to the hydrogen-bond network, strengthening choline-urea bonding, but only for low hydration levels (≤1w). By the same token, Abranches et al. [10] concluded from thermodynamic modelling that the interactions in ChCl:urea DES are weaker, not stronger, in the presence of water.
Looking at higher wavenumbers, more differences among these systems emerge. For the ChCl:urea system, the intensity changes in the 1,400 cm−1 region may be ascribed to the strengthening of hydrogen bonds with choline’s hydroxyl group. Analysis of the INS spectra obtained with the MAPS instrument (which allows a better description of the high wavenumber region than TOSCA) reveals the changes in the NH2 bending modes upon hydration. These changes, previously related to a more heterogeneous environment of the amine groups upon water addition (11), are consistent with the strengthening of hydrogen bonds with the NH donors. The same conclusion is drawn from the INS-MAPS spectra in the region of OH/NH stretching modes, with an intensity increase of the lower wavenumber side of the broad νOH/NH band.
In the case of the ChCl:glycerol system, the most relevant issue revealed in Figures 7, 8B is the unusual behaviour of the 3w sample, which seems to be the sweet spot of the hydration effect before disruption. To the best of our knowledge, there are no experimental reports on this DES pointing to this behaviour. Nevertheless, recent work from Ferreira et al. [12], using NMR spectroscopy to study the ChCl:glycerol system with added water, at water contents ranging from 1% wt to 70% wt, identified three distinct water behaviour domains. up to 11% wt, between 11% and 35%wt, and above 35% wt. The plot of the spin lattice relaxation rates of choline and glycerol hydroxyl groups as function of the water content shows a maximum at 11% wt (which compares well with the 14.3% wt of 3w sample). In addition, the above mentioned MD simulations of Weng and Toner [53] concluded that the effect of water increase on hydrogen bonding between ChCl and glycerol reverses at ca. 10 wt% of water content. Hence, our observation of an inflection point at 3w is directly comparable and agree quite well with those results (12,53).
The third hydrogen bond donor herein considered, lactic acid, also promotes a distinct DES behaviour with increasing water content. While in the low wavenumber region, discussed above, there are striking similarities between ChCl:lactic acid and ChCl:urea in the INS spectra upon water addition, in the 1,000–2000 cm−1 region ChCl:lactic acid is unique. Contrary to both ChCl:urea and ChCl:glycerol, ChCl:lactic acid does not present large intensity changes, observed as broad features in the difference spectra. In fact, within the experimental error, difference spectra 3w-1w and 5w-1w are close to straight lines. On the other hand, the broadening of several sharp bands in the 700–1,300 cm−1 range is evident. This is not observed, to a similar extent, for either of the remaining systems and points to a unique property of the acidic hydrogen-bond donor component. All the affected bands are assigned to choline (including mainly NC stretching and CH3 rocking modes), which points to a strong increase of heterogeneity in the choline cation’s interactions. Alcalde el al. [13], from MD simulations, state that lactic acid molecules are mainly occupied in ChCl-lactic acid hydrogen bonding, which is not weakened upon addition of water molecules. ChCl-lactic acid cluster prevail from small to large water concentrations, up to the limit of dispersed “ChCl-lactic acid monomers” in the hydrogen-bonded water fluid [13]. Such a solvation process of the ChCl-lactic acid clusters may explain the observed broadening effect on the choline anion bands.
Conclusion
This work has addressed several questions in the study of water effects in DES systems as probed by INS. The first was to understand whether known pitfalls on how freezing DES samples (due to the very low temperature required by INS, < 20 K) would affect sample integrity, i.e. do the frozen samples mirror the liquid phase?
The comparison between shock freezing and slow freezing of DES samples evidenced that shock freezing avoids crystallization or phase separation of DES components. Thus, quenching samples in liquid nitrogen is a procedure that may retain the liquid phase morphology of DES at the low temperatures required by INS spectroscopy. This allows the application of INS spectroscopy to unravel the DES interactions at a molecular level. In particular, INS spectroscopy gives access to large amplitude/low wavenumber modes not easily amenable from other spectroscopic techniques.
All the systems studied present similar spectroscopic changes upon water addition. The presence of water is evident from the increase of a broad profile centered at ca. 550 cm−1, ascribed to non-freezing water molecules, i.e., water molecules bound to the DES components without forming water clusters, and thus unable to form ice crystals when subject to a sudden temperature drop. In addition, a band a ca. 175 cm−1 displays an intensity dependence on the water content and is assigned to the intermolecular stretching mode of water molecules hydrogen-bonded to DES components.
The methyl torsional modes also share identical behavior upon water addition. In particular, the low wavenumber component, τ1, moves to lower wavenumber with increasing water content. This behavior is consistent with the preference of water molecules to bind to chloride anion, as predicted by numerous MD simulations dealing with different choline chloride-based DES: the torsional motions of methyl groups in choline chloride are hampered by hydrogen bonding with chloride anion and the preferential interactions of chloride anion with water releases the methyl groups.
Despite all the above similarities, the three systems present several distinct INS features upon water addition that can be related to their unique properties and structure at the molecular level, as revealed by their most relevant spectral features. As such, in the ChCl:urea system a strengthening of hydrogen bonds with the NH and OH donors was observed. In the ChCl:glycerol counterpart, the red-shift of the τ1 band alongside the ideal water content (3w) were the most relevant phenomena observed. Finally, in the ChCl:lactic acid system INS could probe the existence of solvated DES clusters instead of specifically interfering water molecules. All these signature features arising from each system were found to confirm simulation data from the literature.
Data Availability Statement
The datasets presented in this study can be found in online repositories. The names of the repository/repositories and accession number(s) can be found below: Data from the neutron scattering experiments can be accessed freely at https://data.isis.stfc.ac.uk/#/browse/facility/ISIS/instrument/17/facilityCycle/100682278/investigation/108643327/dataset. https://data.isis.stfc.ac.uk/#/browse/facility/ISIS/instrument/7/facilityCycle/81894398/investigation/83550900/dataset. https://data.isis.stfc.ac.uk/#/browse/facility/ISIS/instrument/17/facilityCycle/90581116/investigation/92921697/dataset.
Author Contributions
All authors contributed toward the planning, preparation and implementation of the fieldwork, data analysis and interpretation, and writing the manuscript. In addition, MMN was the Principal Investigator and lead author; MN and PR-C were responsible for projects for INS data collection at ISIS; SP was responsible for laboratory work and sample preparation, as a part of her Ph.D. project; MN, PR-C, PV, CV and SP carried out INS data collection during in-situ missions; SR and SP, the instrument scientists at ISIS, provided the expertise on INS data collection and analysis; AS provided the financial support and student supervision, in co-supervision with CF and MF.
Conflict of Interest
The authors declare that the research was conducted in the absence of any commercial or financial relationships that could be construed as a potential conflict of interest.
Publisher’s Note
All claims expressed in this article are solely those of the authors and do not necessarily represent those of their affiliated organizations, or those of the publisher, the editors and the reviewers. Any product that may be evaluated in this article, or claim that may be made by its manufacturer, is not guaranteed or endorsed by the publisher.
Acknowledgments
This work was developed within the scope of the project CICECO-Aveiro Institute of Materials, (UIDB/50011/2020 and UIDP/50011/2020), financed by national funds through the FCT/MEC. FCT is also acknowledged for the doctoral grant to SP (SFRH/BD/132584/2017) and the research contracts to MN under the program IF 2015 (IF/01468/2015), and to CV under Scientific Employment Stimulus (CEECIND/00263/2018). The STFC Rutherford Appleton Laboratory is thanked for access to neutron beam facilities (TOSCA/RB1920244, TOSCA/RB1610481, TOSCA/RB1810054 and MAPS/RB1620171, and XB1890136; DOIs 10.5286/ISIS.E.RB1920244, 10.5286/ISIS.E.RB1610481, 10.5286/ISIS.E.RB1810054, and 10.5286/ISIS.E.RB1620171).
References
1. Hansen BB, Spittle S, Chen B, Poe D, Zhang Y, Klein JM, et al. Deep Eutectic Solvents: A Review of Fundamentals and Applications. Chem Rev (2021) 121(3):1232–85. doi:10.1021/acs.chemrev.0c00385
2. El Achkar T, Greige-Gerges H, Fourmentin S. Basics and Properties of Deep Eutectic Solvents: a Review. Environ Chem Lett (2021) 19(4):3397–408. doi:10.1007/s10311-021-01225-8
3. Yu D, Xue Z, Mu T. Eutectics: Formation, Properties, and Applications. Chem Soc Rev (2021) 50(15):8596–638. doi:10.1039/d1cs00404b
4. Morais ES, Da Costa Lopes AM, Freire MG, Freire CSR, Silvestre AJD. Unveiling Modifications of Biomass Polysaccharides during Thermal Treatment in Cholinium Chloride : Lactic Acid Deep Eutectic Solvent. ChemSusChem (2021) 14(2):686–98. doi:10.1002/cssc.202002301
5. Wu J, Liang Q, Yu X, Lu Q-F, Ma L, Qin X, et al. Deep Eutectic Solvents for Boosting Electrochemical Energy Storage and Conversion: A Review and Perspective. Adv Funct Mater (2021) 31(22). doi:10.1002/adfm.202011102
6. Pedro SN, Freire MG, Freire CSR, Silvestre AJD. Deep Eutectic Solvents Comprising Active Pharmaceutical Ingredients in the Development of Drug Delivery Systems. Expert Opin Drug Deliv (2019) 16(5):497–506. doi:10.1080/17425247.2019.1604680
7. Ma C, Laaksonen A, Liu C, Lu X, Ji X. The peculiar Effect of Water on Ionic Liquids and Deep Eutectic Solvents. Chem Soc Rev (2018) 47(23):8685–720. doi:10.1039/c8cs00325d
8. Gabriele F, Chiarini M, Germani R, Tiecco M, Spreti N. Effect of Water Addition on Choline Chloride/glycol Deep Eutectic Solvents: Characterization of Their Structural and Physicochemical Properties. J Mol Liq (2019) 291. doi:10.1016/j.molliq.2019.111301
9. Shah D, Mjalli FS. Effect of Water on the Thermo-Physical Properties of Reline: An Experimental and Molecular Simulation Based Approach. Phys Chem Chem Phys (2014) 16(43):23900–7. doi:10.1039/c4cp02600d
10. Abranches DO, Silva LP, Martins MAR, Coutinho JAP. Differences on the Impact of Water on the Deep Eutectic Solvents Betaine/urea and Choline/urea. J Chem Phys (2021) 155(3):034501. doi:10.1063/5.0052303
11. Fetisov EO, Harwood DB, Kuo I-FW, Warrag SEE, Kroon MC, Peters CJ, et al. First-Principles Molecular Dynamics Study of a Deep Eutectic Solvent: Choline Chloride/Urea and its Mixture with Water. J Phys Chem B (2018) 122(3):1245–54. doi:10.1021/acs.jpcb.7b10422
12. Ferreira ASD, Craveiro R, Duarte AR, Barreiros S, Cabrita EJ, Paiva A. Effect of Water on the Structure and Dynamics of Choline Chloride/glycerol Eutectic Systems. J Mol Liq (2021) 342. doi:10.1016/j.molliq.2021.117463
13. Alcalde R, Gutierrez A, Atilhan M, Aparicio S. An Experimental and Theoretical Investigation of the Physicochemical Properties on Choline Chloride - Lactic Acid Based Natural Deep Eutectic Solvent (NADES). J Mol Liq (2019) 290. doi:10.1016/j.molliq.2019.110916
14. Hammond OS, Bowron DT, Edler KJ. The Effect of Water upon Deep Eutectic Solvent Nanostructure: An Unusual Transition from Ionic Mixture to Aqueous Solution. Angew Chem Int Ed (2017) 56(33):9782–5. doi:10.1002/anie.201702486
15. Dai Y, Witkamp G-J, Verpoorte R, Choi YH. Tailoring Properties of Natural Deep Eutectic Solvents with Water to Facilitate Their Applications. Food Chem (2015) 187:14–9. doi:10.1016/j.foodchem.2015.03.123
16. Lapeña D, Lomba L, Artal M, Lafuente C, Giner B. The NADES Glyceline as a Potential Green Solvent: A Comprehensive Study of its Thermophysical Properties and Effect of Water Inclusion. The J Chem Thermodynamics (2019) 128:164–72. doi:10.1016/j.jct.2018.07.031
17. Chen Y, Yu D, Chen W, Fu L, Mu T. Water Absorption by Deep Eutectic Solvents. Phys Chem Chem Phys (2019) 21(5):2601–10. doi:10.1039/c8cp07383j
18. Gutierrez A, Atilhan M, Aparicio S. Molecular Dynamics Study on Water Confinement in Deep Eutectic Solvents. J Mol Liq (2021) 339. doi:10.1016/j.molliq.2021.116758
19. Kumari P, Shobhna KS, Kaur S, Kashyap HK. Influence of Hydration on the Structure of Reline Deep Eutectic Solvent: A Molecular Dynamics Study. ACS OMEGA (2018) 3(11):15246–55. doi:10.1021/acsomega.8b02447
20. Percevault L, Jani A, Sohier T, Noirez L, Paquin L, Gauffre F, et al. Do Deep Eutectic Solvents Form Uniform Mixtures beyond Molecular Microheterogeneities? J Phys Chem B (2020) 124(41):9126–35. doi:10.1021/acs.jpcb.0c06317
21. Di Pietro ME, Tortora M, Bottari C, Colombo Dugoni G, Pivato RV, Rossi B, et al. In Competition for Water: Hydrated Choline Chloride:Urea vs Choline Acetate:Urea Deep Eutectic Solvents. ACS Sust Chem. Eng. (2021) 9(36):12262–73. doi:10.1021/acssuschemeng.1c03811
22. Sapir L, Harries D. Restructuring a Deep Eutectic Solvent by Water: The Nanostructure of Hydrated Choline Chloride/Urea. J Chem Theor Comput. (2020) 16(5):3335–42. doi:10.1021/acs.jctc.0c00120
23. Meng X, Ballerat-Busserolles K, Husson P, Andanson J-M. Impact of Water on the Melting Temperature of Urea + Choline Chloride Deep Eutectic Solvent. New J Chem (2016) 40(5):4492–9. doi:10.1039/c5nj02677f
24. Sakpal SS, Deshmukh SH, Chatterjee S, Ghosh D, Bagchi S. Transition of a Deep Eutectic Solution to Aqueous Solution: A Dynamical Perspective of the Dissolved Solute. J Phys Chem Lett (2021) 12(36):8784–9. doi:10.1021/acs.jpclett.1c02118
25. Mitchell PCH, Parker SF, Ramirez-Cuesta AJ, Tomkinson J. Vibrational Spectroscopy with Neutrons. In With Applications in Chemistry, Biology, Materials Science and Catalysis. Finney JL, Worcester DL (Singapore: World Scientific Publishing Co) (2005).
26. Parker SF, Lennon D, Albers PW. Vibrational Spectroscopy with Neutrons: A Review of New Directions. Appl Spectrosc (2011) 65(12):1325–41. doi:10.1366/11-06456
27. Faraone A, Wagle DV, Baker GA, Novak EC, Ohl M, Reuter D, et al. Glycerol Hydrogen-Bonding Network Dominates Structure and Collective Dynamics in a Deep Eutectic Solvent. J Phys Chem B (2018) 122(3):1261–7. doi:10.1021/acs.jpcb.7b11224
28. Li J. Inelastic Neutron Scattering Studies of Hydrogen Bonding in Ices. J Chem Phys (1996) 105(16):6733–55. doi:10.1063/1.472525
29. Abbott AP, Harris RC, Ryder KS, D'Agostino C, Gladden LF, Mantle MD. Glycerol Eutectics as Sustainable Solvent Systems. Green Chem (2011) 13(1):82–90. doi:10.1039/c0gc00395f
30. Gutiérrez A, Atilhan M, Aparicio S. A Theoretical Study on Lidocaine Solubility in Deep Eutectic Solvents. Phys Chem Chem Phys (2018) 20(43):27464–73. doi:10.1039/c8cp05641b
31. Rosu-Finsen A, Amon A, Armstrong J, Fernandez-Alonso F, Salzmann CG. Deep-Glassy Ice VI Revealed with a Combination of Neutron Spectroscopy and Diffraction. J Phys Chem Lett (2020) 11(3):1106–11. doi:10.1021/acs.jpclett.0c00125
32. Mamontov E, Osti NC, Ryder MR. Order-disorder in Room-Temperature Ionic Liquids Probed via Methyl Quantum Tunneling. Struct Dyn (2021) 8(2):024303. doi:10.1063/4.0000094
33.Science and Technology Facilities Council. ISIS Facility INS/TOSCA. [Internet] (2021). Available from: https://www.isis.stfc.ac.uk/Pages/tosca.aspx (Accessed: January 14, 2022).
34. Parker SF, Fernandez-Alonso F, Ramirez-Cuesta AJ, Tomkinson J, Rudic S, Pinna RS, et al. Recent and Future Developments on TOSCA at ISIS. In: Jimenez Ruiz M, Parker S, editors. J Phys: Conf Ser. Journal of Physics Conference Series, 554 (2014). p. 012003. doi:10.1088/1742-6596/554/1/012003J Phys Conf Ser
35. Pinna RS, Rudić S, Parker SF, Armstrong J, Zanetti M, Škoro G, et al. The Neutron Guide Upgrade of the TOSCA Spectrometer. Nucl Instr Methods Phys Res Section A: Acc Spectrometers, Detectors Associated Equipment (2018) 896:68–74. doi:10.1016/j.nima.2018.04.009
36.Science and Technology Facilities Council. ISIS Facility INS/MAPS. [Internet] (2021). Available from: https://www.isis.stfc.ac.uk/Pages/maps.aspx (Accessed: January 14, 2022).
37. Arnold O, Bilheux JC, Borreguero JM, Buts A, Campbell SI, Chapon L, et al. Mantid-Data Analysis and Visualization Package for Neutron Scattering and μ SR Experiments. Nucl Instr Methods Phys Res Section A: Acc Spectrometers, Detectors Associated Equipment (2014) 764:156–66. doi:10.1016/j.nima.2014.07.029
38. Ribeiro-Claro PJA, Rudic S, Nolasco MM, Araujo CF, Freire MC, Pedro S, et al. API-DES: Improving Drug Solubility with Deep Eutectic Solvents. [Internet]. STFC ISIS Neutron Muon Source (2019). doi:10.5286/ISIS.E.RB1920244
39. Nolasco MM, Vaz PD, Rudic S, Ribeiro-Claro PJA. INS Study for the Structural Characterization of Cellulosic Nanostructured Materials. [Internet]. STFC ISIS Neutron Muon Source (2016). doi:10.5286/ISIS.E.RB1610481
41. Johnson MR, Parlinski K, Natkaniec I, Hudson BS. Ab Initio calculations and INS Measurements of Phonons and Molecular Vibrations in a Model Peptide Compound - Urea. Chem Phys (2003) 291(1):53–60. doi:10.1016/s0301-0104(03)00178-2
43. Nolasco MM, Ribeiro A, Ribeiro-Claro PJA, Rudic S, Araujo CF, Vaz PD. Exploring Asymmetry-Induced Entropy in Deep Eutectic Solvents. [Internet]. STFC ISIS Neutron Muon Source (2018). doi:10.5286/ISIS.E.RB1810054
44. Nolasco MM, Araujo CF, Parker SF, Vaz PD, Ribeiro-Claro PJA. INS Study of Deep Eutectic Solvents. [Internet]. STFC ISIS Neutron Muon Source (2016). Available from. doi:10.5286/ISIS.E.RB1620171
45. Morrison HG, Sun CC, Neervannan S. Characterization of thermal Behavior of Deep Eutectic Solvents and Their Potential as Drug Solubilization Vehicles. Int J Pharm (2009) 378(1–2):136–9. doi:10.1016/j.ijpharm.2009.05.039
46. Araujo C, Freire CSR, Nolasco MM, Ribeiro-Claro PJA, Rudić S, Silvestre AJD, et al. Hydrogen Bond Dynamics of Cellulose through Inelastic Neutron Scattering Spectroscopy. Biomacromolecules (2018) 19(4):1305–13. doi:10.1021/acs.biomac.8b00110
47. Vaz PD, Nolasco MM, Gil FP, Ribeiro-Claro PJ, Tomkinson J. Hydrogen-bond Dynamics of C-H.O Interactions: the chloroform.Acetone Case. Chemistry (2010) 16(30):9010–7. doi:10.1002/chem.201000479
48. Araujo CF, Coutinho JAP, Nolasco MM, Parker SF, Ribeiro-Claro PJA, Rudić S, et al. Inelastic Neutron Scattering Study of Reline: Shedding Light on the Hydrogen Bonding Network of Deep Eutectic Solvents. Phys Chem Chem Phys (2017) 19(27):17998–8009. doi:10.1039/c7cp01286a
49. del Rosso L, Celli M, Colognesi D, Rudić S, English NJ, Ulivi L. Density of Phonon States in Cubic Ice Ic. J Phys Chem C (2021) 125(42):23533–8. doi:10.1021/acs.jpcc.1c07647
50. Vilela C, Freire CSR, Araújo C, Rudić S, Silvestre AJD, Vaz PD, et al. Understanding the Structure and Dynamics of Nanocellulose-Based Composites with Neutral and Ionic Poly(methacrylate) Derivatives Using Inelastic Neutron Scattering and DFT Calculations. Molecules (2020) 25(7). doi:10.3390/molecules25071689
51. Pandey A, Pandey S. Solvatochromic Probe Behavior within Choline Chloride-Based Deep Eutectic Solvents: Effect of Temperature and Water. J Phys Chem B (2014) 118(50):14652–61. doi:10.1021/jp510420h
52. Ahmadi R, Hemmateenejad B, Safavi A, Shojaeifard Z, Shahsavar A, Mohajeri A, et al. Deep Eutectic-Water Binary Solvent Associations Investigated by Vibrational Spectroscopy and Chemometrics. Phys Chem Chem Phys (2018) 20(27):18463–73. doi:10.1039/c8cp00409a
Keywords: reline, glyceline, cholinium chloride, hydration, shock freezing, quenching, DES (deep eutectic solvents), INS (inelastic neutron scattering)
Citation: Nolasco MM, Pedro SN, Vilela C, Vaz PD, Ribeiro-Claro P, Rudić S, Parker SF, Freire CS, Freire MG and Silvestre AJD (2022) Water in Deep Eutectic Solvents: New Insights From Inelastic Neutron Scattering Spectroscopy. Front. Phys. 10:834571. doi: 10.3389/fphy.2022.834571
Received: 13 December 2021; Accepted: 14 January 2022;
Published: 03 February 2022.
Edited by:
Silvina Ponce Dawson, University of Buenos Aires, ArgentinaReviewed by:
Mohammad Tariq, Universidade NOVA de Lisboa, PortugalMauro C. C. Ribeiro, Universidade de São Paulo, Brazil
Copyright © 2022 Nolasco, Pedro, Vilela, Vaz, Ribeiro-Claro, Rudić, Parker, Freire, Freire and Silvestre. This is an open-access article distributed under the terms of the Creative Commons Attribution License (CC BY). The use, distribution or reproduction in other forums is permitted, provided the original author(s) and the copyright owner(s) are credited and that the original publication in this journal is cited, in accordance with accepted academic practice. No use, distribution or reproduction is permitted which does not comply with these terms.
*Correspondence: Mariela M. Nolasco, mnolasco@ua.pt