- 1Department of Breast Surgery and Oncology, The Second Affiliated Hospital, Zhejiang University School of Medicine, Hangzhou, Zhejiang, China
- 2Cancer Institute (Key Laboratory of Cancer Prevention and Intervention, China National Ministry of Education), The Second Affiliated Hospital, Zhejiang University School of Medicine, Hangzhou, China
- 3Department of Surgical Oncology, Linhai Branch, The Second Affiliated Hospital, Zhejiang University School of Medicine, Taizhou, Zhejiang, China
Globally, breast cancer represents the most common cancer and the primary cause of death by cancer in women. Lipids are crucial in human physiology, serving as vital energy reserves, structural elements of biological membranes, and essential signaling molecules. The metabolic reprogramming of lipid pathways has emerged as a critical factor in breast cancer progression, drug resistance, and patient prognosis. In this study, we delve into the clinical implications of lipid pathway-targeted therapy in breast cancer. We highlight key enzymes and potential therapeutic targets involved in lipid metabolism reprogramming, and their associations with cancer progression and treatment outcomes. Furthermore, we detail the clinical trials exploring the anticancer and cancer chemopreventive activity of therapies targeting these molecules. However, the clinical efficacy of these therapies remains controversial, highlighting the urgent need for predictive biomarkers to identify patient subpopulations likely to benefit from such treatment. We propose the Selective Lipid Metabolism Therapy Benefit Hypothesis, emphasizing the importance of personalized medicine in optimizing lipid pathway-targeted therapy for breast cancer patients.
1 Introduction
Globally, breast cancer represents the most common cancer and the primary cause of death by cancer in women (Bray et al., 2024). China has 357,200 new cases of breast cancer each year, posing a serious threat to the lives and health of a large number of women (Zheng et al., 2024). In spite of the progress made in diagnostic techniques and therapeutic interventions, a considerable proportion of patients encounter relapse subsequent to their initial course of treatment, resulting in diminished overall survival (OS) rates and a compromised quality of life (DeSantis et al., 2019; Zhou et al., 2023; Garcia-Martinez et al., 2021). This notable public health challenge underscores the urgency to explore further into the intricacies of breast cancer development and actively seek out promising therapeutic avenues.
Lipids empower diverse cellular life activities, constituting one of the three key energy sources. Furthermore, lipids are crucial in the constitution of cell membranes and function as pivotal signaling molecules (Xiao et al., 2024). Recent studies have revealed alterations in lipid metabolism within tumor cells and microenvironment, which furnish a robust substrate for tumor cell proliferation during therapy and drive the development of therapy resistance (Ligorio et al., 2021; Cheng et al., 2018; Bian et al., 2021; Liu et al., 2024). Correspondingly, the significance of lipid metabolism reprogramming in breast cancer is gradually coming to light (Zipinotti Dos Santos et al., 2023; Nelson et al., 2014; Guo et al., 2020; Bacci et al., 2021; Wang et al., 2024). Increasing evidence suggests that lipid remodeling plays a vital role in conferring drug resistance to breast cancer cells via mechanisms involving ferroptosis, immune escape, endoplasmic reticulum stress, and stemness (Zhang et al., 2019; Havas et al., 2017; Wang et al., 2018; Luis et al., 2021). Consequently, targeting lipid metabolism emerges as a promising therapeutic avenue to tackle resistance to conventional treatments. Notably, lipids occupy pivotal roles in maintaining normal physiological functions (Petrenko et al., 2023). Therefore, prior to contemplating systemic therapies aimed at lipid metabolism as therapeutic modalities for breast cancer, it is imperative to achieve a thorough comprehension of lipid functions within both cancer cells and normal cells, as well as their interplay.
In this study, we present an overview of the physiological processes underlying lipid metabolism and its remodeling in breast cancer. We have also summarized potential therapeutic targets within the lipid metabolism remodeling. Furthermore, we offer a detailed assessment of the evidence derived from both completed and ongoing clinical trials, encompassing those targeting lipid metabolism directly for anticancer effects, those utilizing lipid metabolism modulation as adjunctive therapy to mitigate the toxicity of traditional treatments, and those employing it as a prophylactic measure to prevent breast cancer occurrence. Despite the variability observed in the findings, specific breast cancer patients may indeed benefit from therapies that regulate the lipid pathway (Serageldin et al., 2024; El-Khayat et al., 2021; Kamal et al., 2024; Yulian et al., 2021; Dewidar et al., 2022; Yulian et al., 2023; Serageldin et al., 2023). Consequently, we propose the Selective Lipid Metabolism Therapy Benefit Hypothesis, suggesting that lipid pathway-targeted therapy may confer benefits to a selected group of patients. This hypothesis can aid in better understanding the role of lipid metabolism reprogramming in breast cancer, facilitating the discovery of more stable biomarkers that can predict treatment response, and thereby enabling effective clinical translation.
2 Physiological processes and key molecules of lipid metabolism
Lipids constitute one of the three key energy sources for cells, vital components of cell membranes, and essential signaling molecules, holding a paramount position as fundamental constituents within the physiological machinery of the body (Xiao et al., 2024). Over a thousand distinct lipid types have been identified within living cells, such as fatty acids (FAs), cholesterol, phospholipids, and others (Corradi et al., 2019). Understanding the physiological processes and key molecules of lipid metabolism in normal cells is crucial to grasping how it impacts the behavior of malignant cells and their therapeutic potential as targets (Figure 1).
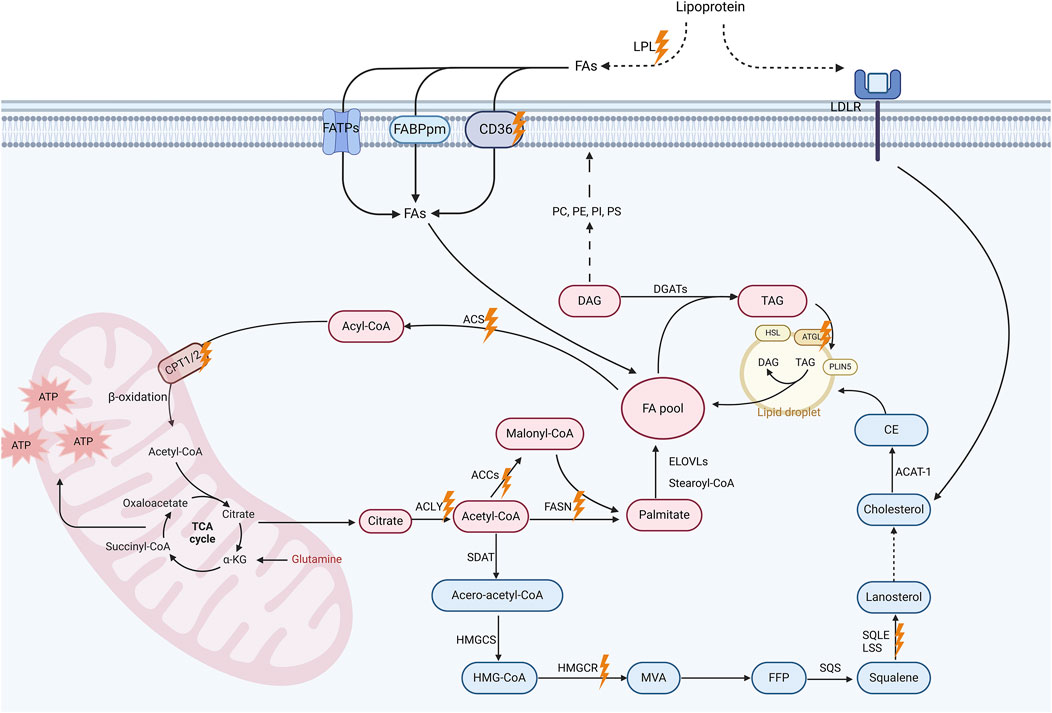
Figure 1. Lipid metabolism pathways and promising targets for breast cancer treatment. This schematic diagram illustrates the key processes and molecular involved in lipid metabolism, including fatty acid (FA) uptake, De novo lipogenesis (DNL), FA oxidation (FAO) and cholesterol biosynthesis. Extracellular triglycerides are hydrolyzed by lipoprotein lipase (LPL), releasing free FAs for cellular uptake via membrane-associated proteins such as CD36, fatty acid transport protein (FATP), and plasma membrane fatty acid-binding protein (FABP). Intracellularly, acetyl-CoA generated from FAs or glucose enters the tricarboxylic acid (TCA) cycle to produce energy in mitochondria. Acetyl-CoA also serve as a substrate for DNL. This metabolic pathway leads to the synthesis of malonyl-CoA and, ultimately, palmitate, which can subsequently undergo elongation and desaturation processes to yield a diverse array of FAs. These FAs can be modified to form diverse lipids including diacylglycerols (DAGs) and triacylglycerols (TAGs). TAGs are stored in lipid droplets, which regulate lipid storage and release under metabolic demands. Additionally, the cholesterol biosynthesis pathway, which is initiated by acetyl-CoA, proceeds through the formation of intermediate compounds such as farnesyl pyrophosphate (FPP) and squalene, eventually leading to the production of cholesterol. Cholesterol contributes to membrane fluidity and steroid hormone synthesis, with excess cholesterol stored as cholesterol esters in lipid droplets. Importantly, these metabolic pathways altered in breast cancer cells, which is likely associated with cancer progression. Promising therapeutic targets are marked with orange lightning symbols. Created in https://BioRender.com.
The essential details of the biochemical processes underlying lipid metabolism have been comprehensively discussed elsewhere (Yu et al., 2021). Briefly, FA uptake is facilitated by lipoprotein lipase (LPL)-mediated extracellular hydrolysis of triglycerides into free FAs and glycerol, with CD36, among other membrane-associated proteins, playing a pivotal role in cellular FA transport (Ligorio et al., 2021; Abumrad et al., 2021; Samovski et al., 2023). De novo lipogenesis (DNL, i.e., de novo FA synthesis) occurs in the cytoplasm, utilizing acetyl-coenzyme A (acetyl-CoA) as the base stock. Key enzymes in this process include adenosine triphosphate (ATP)-citrate lyase (ACLY), acetyl-CoA carboxylase (ACC), and FA synthase (FASN), which catalyze the formation of cytosolic acetyl-CoA, malonyl-CoA, and long-chain saturated FAs (primarily palmitate), respectively (Vasseur and Guillaumond, 2022; Currie et al., 2013; Martin-Perez et al., 2022). FAs are able to undergo conversion into diverse types of lipids (Zipinotti Dos Santos et al., 2023; Dyall et al., 2022). For instance, FAs can be further metabolized to produce various glycerophospholipids, e.g., phosphatidylserine (PS), which are essential for membrane structure and function (Vance, 2015; Baenke et al., 2013; Baxter et al., 2015; Kopecka et al., 2020). FA oxidation (FAO) is a critical energy-producing process that occurs in four stages: FA activation, mitochondrial transfer, β-oxidation yielding acetyl-CoA, and acetyl-CoA entering the tricarboxylic acid (TCA) cycle. Enzymes such as acyl-CoA synthetase (ACS) and carnitine palmitoyltransferase (CPT) 1 take an active part in this process (Kemp et al., 2024; Li et al., 2017; Knottnerus et al., 2018; Carracedo et al., 2013).
Cholesterol stands out as another vital lipid, crucial for cellular function, impacting membrane fluidity, signal transduction, and steroid hormone synthesis (Luo et al., 2020). Cholesterol can be produced via the endogenous mevalonate (MVA) pathway or obtained extracellularly through transmembrane receptor proteins, primarily low density lipoprotein receptor (LDLR). Cholesterol biosynthesis involves a series of essential enzymes, including 3-hydroxy-3-methylglutaryl-coenzyme A (HMG-CoA) reductase (HMGCR), which serves as the rate-limiting enzyme in the MVA pathway, as well as squalene synthase (SQS), squalene epoxidase (SQLE), and lanosterol synthase (LSS) (Vasseur and Guillaumond, 2022; Luo et al., 2020). Excessive cholesterol is metabolized either into its primary metabolite, 27-hydroxycholesterol (27HC), or into cholesterol ester, which is then stored in lipid droplets (Zipinotti Dos Santos et al., 2023). Lipid droplets, composed of FAs, sterol esters, and triacylglycerols, encapsulated by a cholesterol and phospholipid monolayer, function as crucial subcellular organelles managing cellular lipid metabolism. Enzymes such as adipose triglyceride lipase (ATGL) regulate lipid catabolism on their surface (Yu et al., 2021; Farese and Walther, 2009; Olzmann and Carvalho, 2019; Tauchi-Sato et al., 2002).
3 Potential and obstacles in targeting lipid remodeling in breast cancer
The rapid proliferation of cancer cells heightens the necessity for local oxygen and nutrients, yet inadequately structured blood vessels fail to meet this demand. Consequently, the tumor microenvironment becomes acidic, hypoxic, and glucose-deprived. This triggers the activation and utilization of lipids as a primary energy source and key regulators within tumor cells, disrupting various signaling pathways and immune activities, while fostering tumor cell growth, proliferation, and migration (Zipinotti Dos Santos et al., 2023; Yu et al., 2021). Given that the landscape of lipid metabolism in breast cancer has been extensively covered in recent reviews (Xiao et al., 2024; Wang et al., 2024), our focus herein is primarily on the potential therapeutic targets within the lipid remodeling (Figure 2).
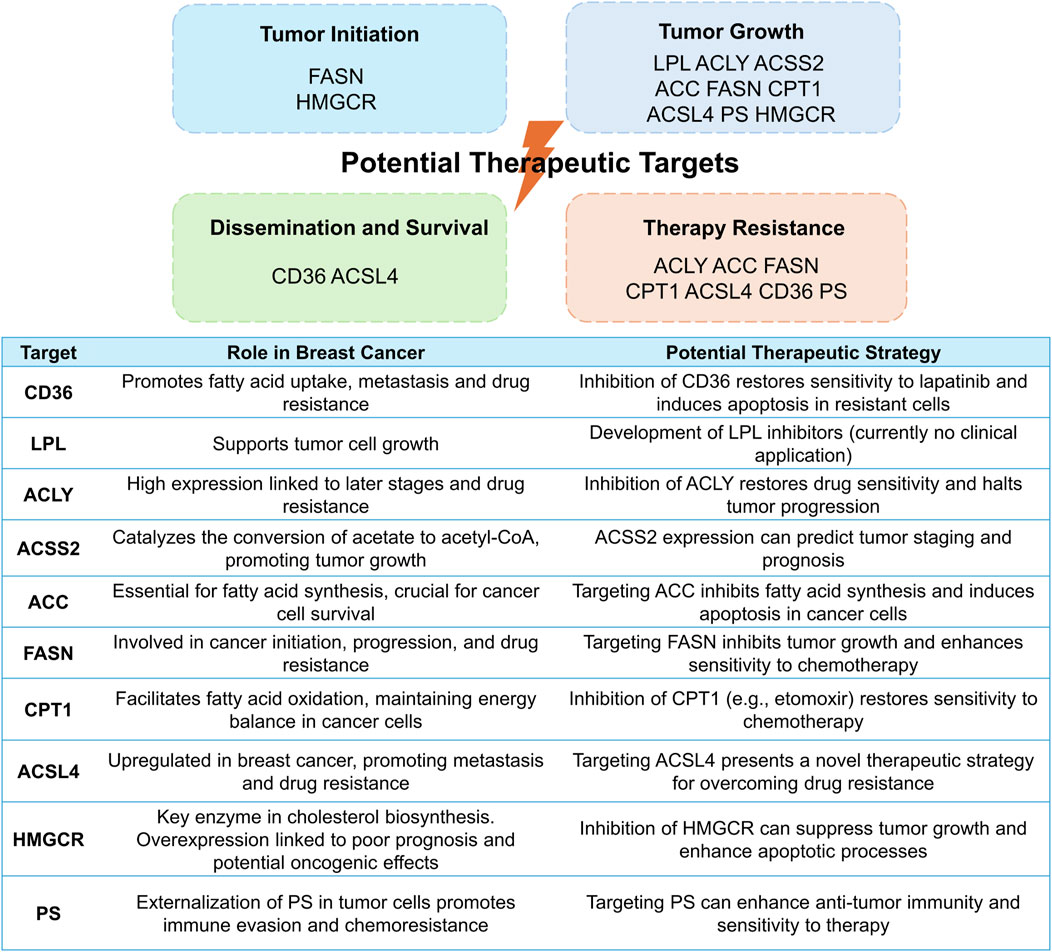
Figure 2. Potential therapeutic targets within lipid remodeling pathways in breast cancer: mechanisms, roles, and strategies. This figure elucidates diverse therapeutic targets in lipid remodeling, underlying tumorigenesis and progression (top), and underscores their significance in breast cancer, presenting potential strategies to leverage these pathways for therapeutic advantage (bottom).
3.1 Fatty acid uptake
The overexpression of CD36 in cancer cells has reportedly been to enhance the transcription of genes associated with metastasis formation, facilitating the spread of cancer to lymph nodes in mice (Pascual et al., 2017). Feng and colleagues found that CD36 acted as a key player in resistance to lapatinib in human epidermal growth factor receptor 2 (HER2)-positive breast cancer cells, and inhibiting CD36 restored sensitivity to lapatinib and triggered apoptosis in those lapatinib-resistant cells (Feng et al., 2019). Moreover, elevated CD36 levels were found to be correlated with unfavorable prognoses (Pascual et al., 2017; Koundouros and Poulogiannis, 2020). Correspondingly, CD36 may provide a promising therapeutic target (Zhao J. et al., 2017). Besides, literature showed that LPL had the potential to accelerate the growth of tumor cells (Kuemmerle et al., 2011) However, no inhibitors targeting enzymes implicated in FA uptake have been introduced into clinical practice yet, restricting the translational potential of these findings.
3.2 Fatty acid synthesis
High expression of ACLY in breast cancer tissues is conversely proportional to disease stage and prognosis (Xiao et al., 2024; Chen et al., 2020). Furthermore, ACLY is implicated in the resistance to tamoxifen, palbociclib, and paclitaxel in breast cancer, whereas ACLY inhibition can re-establish sensitivity to these drugs and halts tumor progression (Chen et al., 2020; Ismail et al., 2022; Ismail et al., 2020; Velez et al., 2023). Besides cleavage of citrate by ACLY, the supply of cytosolic acetyl-CoA pool can be achieved via the conjugation of acetate with CoA under hypoxia and lipid-depleted conditions, catalyzed by acetyl-CoA synthetase 2 (ACSS2) (Schug et al., 2015). Hence, ACSS2 is essential for tumor growth under metabolic stress, and its expression can predict tumor staging and patient prognosis (Schug et al., 2015; Ling et al., 2022). As the rate-limiting enzyme of lipid biosynthesis, ACC definitely holds a pivotal position in breast cancer cell development (Brunet et al., 2008; Chajès et al., 2006), while silencing ACC hinders cell proliferation and triggers apoptosis in breast cancer cell lines (Chajès et al., 2006). Accordingly, ACC emerges as a potential target for suppressing FA biosynthesis in human cancers with pharmacological inhibitors exhibiting encouraging antitumor effects (Tong, 2005; Lally et al., 2019). As another crucial enzyme involved in DNL, FASN also contributes to breast cancer initiation, progression, and treatment resistance (Menendez and Lupu, 2017). Mechanistically, besides supplying energy to cancer cells, FASN exerts its pro-tumor effects through its positive feedback loop with HER2 (Menendez et al., 2021; Kumar-Sinha et al., 2003; Vazquez-Martin et al., 2008) as well as its interaction with nuclear factor-κB (NF-κB) (Liu et al., 2013; Wu et al., 2016), which affect downstream signaling pathways and cellular processes critical for cancer cell survival and resistance to treatment. Targeting FASN may therefore hold promise to inhibit tumor cell growth and enhance the vulnerability of breast cancer cells to traditional anticancer treatments (Menendez and Lupu, 2017).
3.3 Fatty acid oxidation
ACSs are categorized into five groups based on the length of the FA chain they act upon. Among them, long-chain ACSs (ACSLs) are accountable for the catalyzation of intracellular free long-chain FAs, which are then transported by transporter proteins such as CD36 (Rossi Sebastiano and Konstantinidou, 2019). Among the ACSL family, ACSL4 has been widely researched and linked to tumor progression. ACSL4 exhibits an abnormal upregulation in triple-negative breast cancer (TNBC) cells and is correlated with unfavorable prognosis and metastasis in TNBC patients (Qiu et al., 2024). Preclinical studies have revealed that ACSL4 can be activated in drug-resistant breast cancer cells (Li et al., 2022). In turn, this activation can impede the apoptotic pathway and enhance the mTOR pathway, thereby promoting cancer growth and conferring resistance to chemotherapy, endocrinotherapy, and radiotherapy (Li et al., 2022; Wu et al., 2013; Orlando et al., 2019). Consequently, targeting ACSL4 presents a novel therapeutic strategy for breast cancer, while transferring the basic scientific discoveries into clinical practice poses a significant hurdle. As the rate-limiting enzyme in FAO, CPT1 serves as a key factor in the initiation, progression, and migration of cancer cells (Wang et al., 2021). This enzyme not only fulfills the energy demands of cancer cells by facilitating FAO but also exerts its influence through various signaling pathways, cytokines, or microRNAs (Wang et al., 2021). Elevated levels of CPT1 expression have been detected in recurrent breast cancer cases, which are associated with unfavorable patient outcomes (Han et al., 2019). Moreover, serum CPT1 levels reliably signify the disease progression in breast cancer patients (Tan et al., 2021), underscoring its potential utility as a biomarker in breast cancer management. Accumulating evidence suggests that a key mechanism underlying CPT1’s role in fostering treatment resistance is its ability to sustain stemness in stem cells (Wang et al., 2018; Han et al., 2019; He et al., 2019; Shim et al., 2022; Chen et al., 2023). Preclinical studies have demonstrated that etomoxir (a CPT1 inhibitor) can re-sensitize multiple cancers, including breast cancer, to conventional chemotherapy and radiotherapy (Han et al., 2019; Shim et al., 2022; Chen et al., 2023; Tan et al., 2018; Pucer et al., 2013). The mechanism by which CPT2 mediates radiotherapy resistance in breast cancer cells is analogous to that of CPT1 (Han et al., 2019). Unfortunately, despite their potential, none of the FAO-related therapeutic targets have been successfully translated into clinical practice for breast cancer treatment.
3.4 Cholesterol synthesis
In cancer patients, there is an increase in endogenous cholesterol synthesis, coupled with elevated circulating cholesterol levels (Kopecka et al., 2020). The heightened cholesterol levels can augment downstream oncogenic pathway signaling, including Hedgehog- and mammalian target of rapamycin complex 1 (mTORC1)-dependent pathways (Ding et al., 2019). Furthermore, by exerting its influence on membrane fluidity, lipid raft function, and transcriptional processes, cholesterol can also regulate the activity of drug efflux transporters, thereby contributing to drug resistance (Kopecka et al., 2020; Ding et al., 2019; Celestino et al., 2015). The overexpression of MVA pathway-related molecules was observed in breast cancer patients with a worse prognosis (Kuzu et al., 2016; Brown et al., 2016; Clendening et al., 2010; Kim et al., 2019; Bjarnadottir et al., 2020). Additionally, HMGCR may possess oncogenic properties, and perturbations within the MVA pathway could facilitate cellular transformation (Clendening et al., 2010). Research has indicated that HMGCR and SQLE represent compelling targets for cancer treatment, albeit clinical studies on the latter are still lacking (Feltrin et al., 2020).
3.5 Phospholipids
Glycerophospholipids hold a critical position in the initiation and progression of cancer, and they mediate the resistance of cancer cells to chemotherapy via various mechanisms, encompassing modulating the composition of cellular membranes, influencing FA metabolism, acting as second messengers to activate DNA repair mechanisms, and upregulating drug efflux transporters (Kopecka et al., 2020). PS is a phospholipid confined to the inner leaflet of the cell membrane. In solid tumors, hypoxia and other pathophysiological stressors induce the externalization of PS, where it migrates to the outer leaflet (Gerber et al., 2018). The interaction between PS and its receptors critically regulates immuno-inflammatory responses, fostering an immune-evasive tumor microenvironment. Additionally, PS-receptor interaction mediates chemoresistance in cancer cells by the upregulation of drug efflux transporters and the activation of signaling cascades, e.g., phosphatidylinositol 3-kinase (PI3K)/Akt pathways (Zhou et al., 2020). These findings indicate the promising potential of PS-targeted therapy in combating tumor invasion and chemoresistance.
3.6 Obstacles in targeting lipid remodeling
As mentioned above, despite the existence of numerous promising targets, their successful clinical translation remains elusive. Presently, the therapeutic strategies targeting lipid metabolism in breast cancer face several obstacles that require attention. Firstly, the inhibition of lipid metabolic pathways may raise safety concerns due to their potential to induce detrimental effects on normal cells, thereby limiting the development of safe and specific inhibitors (Ligorio et al., 2021). Secondly, the translation of preclinical findings into a clinical setting poses significant challenges, as experiment conditions utilized in preclinical studies may employ supra-physiological concentrations of lipid-modulating drugs (Goodwin et al., 2022). Thirdly, the metabolic adaptability of cancer cells may undermine the effectiveness of inhibiting a single enzyme or pathway alone in sustaining long-term tumor suppression (Ligorio et al., 2021; Fendt et al., 2020). Notwithstanding the challenges, some therapeutic strategies targeting lipid metabolism have still progressed into clinical trials, which will be exhaustively presented in the following section.
4 Clinical trials concerning regulation of lipid metabolism in patients with breast cancer
4.1 Anticancer activity
Despite strong evidence supporting FASN as a promising target for breast cancer therapy, only a few compounds inhibiting FASN have entered clinical studies to date. TVB-2640 is a small molecule that stands as the first highly selective human FASN inhibitor to progress into clinical trials (Falchook et al., 2021). A phase I clinical trial have confirmed the biological activities and safety of the TVB-2640 in solid tumors, including breast cancer (Falchook et al., 2021). Accordingly, an ongoing Phase II clinical study (NCT03179904) will further investigate the efficacy and safety profile of TVB-2640 administered in conjunction with trastuzumab plus paclitaxel or endocrine therapy in the management of advanced HER2-positive breast cancer (Table 1). Besides the FASN inhibitor TVB-2640, several other clinical medications or plant-derived polyphenols have been demonstrated to block FASN, among other targets, and enhance the effectiveness of numerous traditional therapies. Examples include omeprazole (Sardesai et al., 2021), conjugated linoleic acid (CLA) (McGowan et al., 2013), and epigallocatechin-3-gallate (EGCG) (Brusselmans et al., 2003), which have undergone corresponding clinical trials. Notably, research results for the former two have been published (Table 2). Consequently, the combination of omeprazole and neoadjuvant chemotherapy has yielded a promising pathological complete response (pCR) rate (Sardesai et al., 2021), and preoperative administration of CLA for ten to 28 days resulted in significant reductions in Spot 14 and Ki67 levels (McGowan et al., 2013).
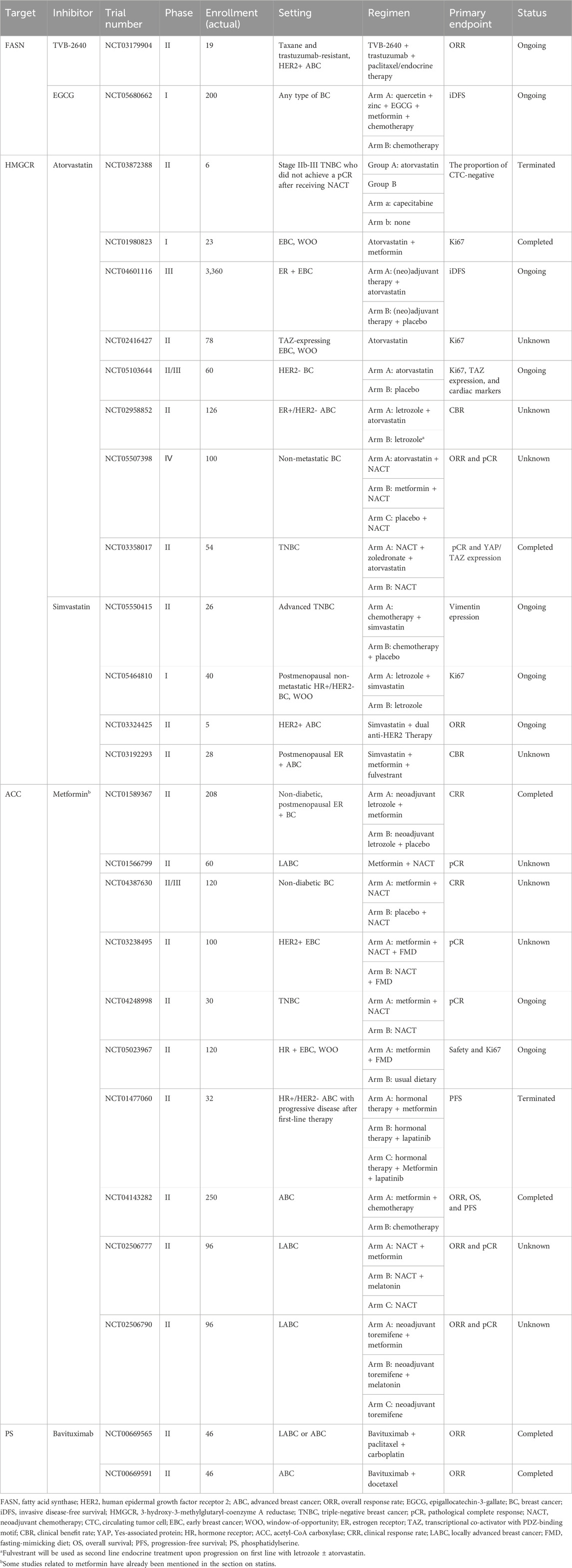
Table 1. Completed (unpublished) and ongoing trials of lipid pathway-targeted therapy in breast cancer (BC).
Statins, functioning via the inhibition HMGCR, are commonly prescribed for patients with cardiovascular disease and high cholesterol levels to lower their cholesterol (Clendening and Penn, 2012). In recent years, growing trials have focused on their promising anticancer benefits (Kamal et al., 2024; Yulian et al., 2021; Dewidar et al., 2022; Yulian et al., 2023; Garwood et al., 2010; Feldt et al., 2015; Bjarnadottir et al., 2015; Nielsen et al., 2012). Currently, clinical trials for drugs such as fluvastatin, atorvastatin, simvastatin, and pitavastatin have been initiated worldwide, primarily for use before surgery in early breast cancer. The potential of these drugs, either as monotherapy or in addition to traditional standard therapy, is being evaluated through clinical or pathological responses (Tables 1, 2). Among them, results from three window-of-opportunity studies have been published, generally finding that short-term monotherapy with statins could suppress tumor growth and enhance apoptotic processes (Kamal et al., 2024; Garwood et al., 2010; Feldt et al., 2015; Bjarnadottir et al., 2015). Additionally, two published studies in the neoadjuvant setting suggested that statins combined with standard neoadjuvant chemotherapy could, to some extent, enhance clinical and pathological responses compared to placebo, although the statistical differences were not always significant (Yulian et al., 2021; Dewidar et al., 2022; Yulian et al., 2023). Ongoing trials will delve deeper into the therapeutic potential of statins as monotherapy, alongside chemotherapy or with endocrine therapy for early- and advanced-stage breast cancer. For instance, the ongoing Phase III MASTER trial (NCT04601116) aims to determine whether long-term statin therapy can enhance the prognosis of women with early breast cancer.
Metformin, a frequently prescribed biguanide, stands as a cornerstone medication in managing hyperglycemia and type 2 diabetes (Pernicova and Korbonits, 2014). Increasing evidence suggests a potential effectiveness of this compound as an anticancer agent (Pernicova and Korbonits, 2014; Thompson, 2014). A fundamental way in which metformin exerts its influence encompasses the stimulation of AMP-activated protein kinase (AMPK), a vital metabolic modulator essential for maintaining energy balance and regulating cellular growth (Ben Sahra et al., 2010). Of note, AMPK has an impact on inhibiting ACC activity (Fullerton et al., 2013; Svensson et al., 2016). Accordingly, the antitumor activity of metformin could be at least partially facilitated by hindering FA biosynthesis (Ligorio et al., 2021). Herein, we also provide an overview of the clinical studies regarding the application of metformin in breast cancer (Table 1 and 2). Unfortunately, the research findings remain controversial. Some studies have found that preoperative monotherapy with metformin for 2-4 weeks could significantly reduce Ki67 levels and oncogenic signaling (Hadad et al., 2011; Dowling et al., 2015; Niraula et al., 2012; DeCensi et al., 2014). Furthermore, a study conducted by Serageldin et al. demonstrated that metformin could provide an additional benefit in terms of clinical and pathological responses compared to neoadjuvant chemotherapy alone (Serageldin et al., 2024). However, most research, including a phase III trial (MA.32) involving 3,649 patients (Goodwin et al., 2022), has indicated that metformin offered no advantage in antitumor effect, whether in early-stage (El-Khayat et al., 2021; Goodwin et al., 2022; Goodwin et al., 2023; Barakat et al., 2022; Kalinsky et al., 2014; Huang et al., 2023; Martin-Castillo et al., 2018; Cuyàs et al., 2019a; Cuyàs et al., 2019b; Lopez-Bonet et al., 2019; Bonanni et al., 2012; Cazzaniga et al., 2013; DeCensi et al., 2015; Rocca et al., 2021) or late-stage (Zhao Y. et al., 2017; Essa et al., 2022; Nanni et al., 2019; Pimentel et al., 2019; Yam et al., 2019) breast cancer, and regardless of whether the treatment was administered as monotherapy or in addition to conventional regimens. Notably, preclinical studies on ACC inhibition have exhibited contradictory outcomes regarding its anticancer effectiveness (Ligorio et al., 2021). Furthermore, Liu et al. discovered that the conjunction of metformin and simvastatin exhibits synergistic inhibition of various cancer cells, suggesting its potential in antitumor therapy when used in conjunction (Liu et al., 2023).
Bavituximab represents an unconjugated chimeric immunoglobulin G1 (IgG1) monoclonal antibody specifically binds to PS (Gerber et al., 2018). The unique external exposure of PS on tumor cells makes bavituximab an effective agent for targeting tumor specifically (Jennewein et al., 2008). Preclinical studies have shown that chemotherapy and radiation therapy could increase the exposure of PS, further enhancing bavituximab binding and immune activation (Huang et al., 2005; He et al., 2007). Therefore, bavituximab may serve as an ideal partner for chemotherapy and radiation therapy. A phase I clinical trial has thus far confirmed the safety of bavituximab administration in breast cancer (Chalasani et al., 2015). Additionally, two ongoing phase Ⅱ trials (NCT00669565 and NCT00669591) have completed their investigation into the potential value of combining bavituximab with chemotherapy in advanced breast cancer, although the research data have not yet been disclosed (Table 1). However, a randomized phase III trial comparing docetaxel plus bavituximab to docetaxel alone in patients with previously treated advanced non-squamous non-small-cell lung cancer failed to demonstrate survival benefit for the combination therapy (Gerber et al., 2018).
4.2 Complication reduction and cancer prevention potential
Despite contradictory research findings regarding its direct anticancer effects in breast cancer, lipid pathway-targeted therapy has been widely accepted for its crucial role in managing complications associated with treatments such as chemotherapy. For instance, metformin has been shown to alleviate chemotherapy-induced peripheral neuropathy neutropenia, cardiac toxicity, oral mucositis, and fatigue in non-diabetic breast cancer patients (Serageldin et al., 2023; Nanni et al., 2019; Bakry et al., 2023). Based on the preclinical evidence, statins appear to have the potential to mitigate chemotherapy-induced cardiotoxicity (Padegimas et al., 2020; Pecoraro et al., 2023; Bjørnstad et al., 2022). However, results from published clinical studies indicate that statins did not affect declines in left ventricular ejection fraction among certain breast cancer patients (Hundley et al., 2022; Makhlin et al., 2024; Thavendiranathan et al., 2023). An ongoing study is currently assessing the use of atorvastatin for prophylactic cardioprotection in patients undergoing anti-HER2 targeted therapy (NCT05559164). Lipid pathway-targeted therapy is also capable of protecting patients from the side effects of radiation therapy. In a phase Ⅱ double-blind, placebo-controlled randomized trial, the prophylactic application of EGCG solution significantly decreased both the occurrence and magnitude of radiation-induced dermatitis among breast cancer patients receiving adjuvant radiotherapy (Zhao et al., 2022). Statins are also believed to alleviate radiation-related complications (Ricco and Kron, 2023), however, clinical studies related to statin protection during radiotherapy in breast cancer patients have been terminated (NCT04385433, NCT00902668).
Furthermore, there are currently numerous completed as well as ongoing clinical trials exploring the potential of lipid pathway-targeted therapy in breast cancer prevention. The omega-3 preparation Lovaza was reported to reduce breast cancer risk in obese women (Manni et al., 2017). Although many observational analyses have found that metformin therapy did not practically influence cancer incidence (Dankner et al., 2019; Dickerman et al., 2023), there are also studies that supported a potential beneficial impact of metformin on reducing cancer risk (Evans et al., 2005; Muszyńska-Ogłaza et al., 2017; Tapia et al., 2021; Tseng, 2014). A clinical trial that is still ongoing will specifically explore the potential of metformin in preventing the development of invasive breast cancer among patients with a history of breast carcinoma in situ or atypical hyperplasia (NCT01905046). Similarly, there is no definitive conclusion pertaining to the association between statin administration and breast cancer risk (Higgins et al., 2012; Vinayak et al., 2013). Of note, several studies have indicated that lipophilic statins (i.e., simvastatin, fluvastatin, and lovastatin) may offer superior breast cancer prevention benefits compared to hydrophilic statins (i.e., atorvastatin and pravastatin) (Ahern et al., 2011; Cauley et al., 2006).
4.3 Selective lipid metabolism therapy benefit hypothesis
Based on the above, the clinical evidence projecting lipid pathway-targeted therapy as an anticancer or cancer chemopreventive strategy is variable. Nonetheless, it is recognized that certain breast cancer patients can indeed benefit from lipid pathway-targeted therapy (Serageldin et al., 2024; El-Khayat et al., 2021; Kamal et al., 2024; Yulian et al., 2021; Dewidar et al., 2022; Yulian et al., 2023; Serageldin et al., 2023). Consequently, we advance the Selective Lipid Metabolism Therapy Benefit Hypothesis, suggesting that therapeutic strategies targeting lipid metabolism may selectively benefit a subset of patients. This hypothesis neither unconditionally advocates for the absolute benefits of targeting lipid metabolism nor conclusively denies its significance based solely on negative trial results. Instead, it offers a more objective and dialectical understanding of the clinical value of lipid pathway-targeted therapies. This hypothesis highlights the need for a more precise identification of the patient subpopulation that may potentially benefit from such treatment, which is of paramount importance. Currently, some clinical trials have identified potential biomarkers capable of predicting the treatment response to lipid metabolism-targeting therapies, which will be comprehensively summarized in the next section.
4.4 Selective biomarkers
Currently, a number of clinical trials have identified a panel of promising biomarkers (Feldt et al., 2015; Cuyàs et al., 2019a; Hadad et al., 2015; Pimentel et al., 2021; Goodwin et al., 2015; Goodwin et al., 2021a; Feldt et al., 2020; Ahern et al., 2020; Goodwin et al., 2021b). Based on an exploratory analysis of a window-of-opportunity breast cancer trial, Feldt and colleagues suggested that the expression levels of cyclin D1, p27, and low-density lipoprotein receptor in tumor tissues might influence the anti-proliferative efficacy of atorvastatin (Feldt et al., 2015; Feldt et al., 2020). Furthermore, evidence exists indicating that HER2 overexpression and genetic variations in ABCB1, CYP3A4, SLCO1B1, and CYP3A5 serve as biomarkers for predicting the response of breast tumors to simvastatin (Yulian et al., 2021; Ahern et al., 2020). Regarding Metformin, studies have found it to be more effective in patients with a BMI ≥25 (El-Khayat et al., 2021), although this finding remains controversial (Kalinsky et al., 2014). The Phase Ⅱ METTEN trial suggested that the C allele of ataxia telangiectasia mutated (ATM) rs11212617 might function as a predictive biomarker to guide the personalized use of metformin in patients with breast cancer (Cuyàs et al., 2019a); however, subsequent findings from the Phase Ⅲ MA.32 trial refuted this notion (Goodwin et al., 2021b). This controversy may stem from differences in the patient populations included in the two studies, as some research indicates that the anticancer effects of metformin in breast cancer exhibit subtype specificity (Yulian et al., 2021; Bonanni et al., 2012). Specifically, the METTEN trial exclusively enrolled HER2-positive breast cancer patients, whereas the MA.32 trial included patients regardless of their subtype. Additionally, the METTEN trial proposed that serum homocysteine levels might serve as an indicator connecting the antifolate-mimicking effects of metformin with tumor response (Cuyàs et al., 2019b). An observable trend towards differing metformin efficacy, based on insulin resistance status, is also noted (Bonanni et al., 2012; Cazzaniga et al., 2013). Further exploration and validation of stable biomarkers for lipid pathway-targeted therapy in large-scale clinical trials are warranted.
5 Conclusion and perspectives
The reprogramming of lipid metabolism has a vital role in breast cancer progression, drug resistance, and clinical outcome. We herein elaborate on the potential of regulating lipid metabolism in the clinical application for breast cancer, focusing on key enzymes and clinical trials. However, contrary to expectations, many trials regarding lipid pathway-targeted therapy have yielded negative results, possibly due to patient heterogeneity and the intricate crosstalk between lipid metabolism and cancer biology. Therefore, we propose the Selective Lipid Metabolism Therapy Benefit Hypothesis, advocating for the development of predictive biomarkers to guide personalized treatment decisions. Future research should focus on identifying and validating these biomarkers, as well as exploring novel therapeutic strategies to target lipid metabolism in breast cancer patients. By optimizing lipid pathway-targeted therapy, we can potentially improve patient outcomes, reduce complications, and prevent cancer recurrence.
Data availability statement
The original contributions presented in the study are included in the article/supplementary material, further inquiries can be directed to the corresponding authors.
Author contributions
DL: Conceptualization, Investigation, Writing–original draft. PJ: Conceptualization, Writing–original draft. YC: Software, Writing–original draft. SW: Writing–original draft. XG: Writing–original draft. ZZ: Writing–review and editing. KL: Writing–review and editing. PL: Writing–review and editing. YH: Project administration, Supervision, Writing–review and editing. YZ: Conceptualization, Project administration, Writing–review and editing.
Funding
The author(s) declare that financial support was received for the research, authorship, and/or publication of this article. This work was funded by the National Natural Science Foundation of China (Grant No. 82373437, 82072900), the Key Research and Development Program of Zhejiang Province (Grant No. 2024C03183), A Project Supported by Scientific Research Fund of Zhejiang Provincial Education Department (Grant No. Y202353498).
Conflict of interest
The authors declare that the research was conducted in the absence of any commercial or financial relationships that could be construed as a potential conflict of interest.
Generative AI statement
The author(s) declare that no Generative AI was used in the creation of this manuscript.
Publisher’s note
All claims expressed in this article are solely those of the authors and do not necessarily represent those of their affiliated organizations, or those of the publisher, the editors and the reviewers. Any product that may be evaluated in this article, or claim that may be made by its manufacturer, is not guaranteed or endorsed by the publisher.
References
Abumrad, N. A., Cabodevilla, A. G., Samovski, D., Pietka, T., Basu, D., and Goldberg, I. J. (2021). Endothelial cell receptors in tissue lipid uptake and metabolism. Circ. Res. 128 (3), 433–450. doi:10.1161/CIRCRESAHA.120.318003
Ahern, T. P., Damkier, P., Feddersen, S., Kjærsgaard, A., Lash, T. L., Hamilton-Dutoit, S., et al. (2020). Predictive pharmacogenetic biomarkers for breast cancer recurrence prevention by simvastatin. Acta Oncol. 59 (9), 1009–1015. doi:10.1080/0284186X.2020.1759820
Ahern, T. P., Pedersen, L., Tarp, M., Cronin-Fenton, D. P., Garne, J. P., Silliman, R. A., et al. (2011). Statin prescriptions and breast cancer recurrence risk: a Danish nationwide prospective cohort study. J. Natl. Cancer Inst. 103 (19), 1461–1468. doi:10.1093/jnci/djr291
Bacci, M., Lorito, N., Smiriglia, A., and Morandi, A. (2021). Fat and furious: lipid metabolism in antitumoral therapy response and resistance. Trends Cancer 7 (3), 198–213. doi:10.1016/j.trecan.2020.10.004
Baenke, F., Peck, B., Miess, H., and Schulze, A. (2013). Hooked on fat: the role of lipid synthesis in cancer metabolism and tumour development. Dis. Model Mech. 6 (6), 1353–1363. doi:10.1242/dmm.011338
Bakry, H. M., Mansour, N. O., ElKhodary, T. R., and Soliman, M. M. (2023). Efficacy of metformin in prevention of paclitaxel-induced peripheral neuropathy in breast cancer patients: a randomized controlled trial. Front. Pharmacol. 14, 1181312. doi:10.3389/fphar.2023.1181312
Barakat, H. E., Hussein, R. R. S., Elberry, A. A., Zaki, M. A., and Ramadan, M. E. (2022). The impact of metformin use on the outcomes of locally advanced breast cancer patients receiving neoadjuvant chemotherapy: an open-labelled randomized controlled trial. Sci. Rep. 12 (1), 7656. doi:10.1038/s41598-022-11138-3
Baxter, A. A., Hulett, M. D., and Poon, I. K. (2015). The phospholipid code: a key component of dying cell recognition, tumor progression and host-microbe interactions. Cell Death Differ. 22 (12), 1893–1905. doi:10.1038/cdd.2015.122
Ben Sahra, I., Le Marchand-Brustel, Y., Tanti, J. F., and Bost, F. (2010). Metformin in cancer therapy: a new perspective for an old antidiabetic drug? Mol. Cancer Ther. 9 (5), 1092–1099. doi:10.1158/1535-7163.MCT-09-1186
Bian, X., Liu, R., Meng, Y., Xing, D., Xu, D., and Lu, Z. (2021). Lipid metabolism and cancer. J. Exp. Med. 218 (1), e20201606. doi:10.1084/jem.20201606
Bjarnadottir, O., Feldt, M., Inasu, M., Bendahl, P. O., Elebro, K., Kimbung, S., et al. (2020). Statin use, HMGCR expression, and breast cancer survival - the Malmö Diet and Cancer Study. Sci. Rep. 10 (1), 558. doi:10.1038/s41598-019-57323-9
Bjarnadottir, O., Kimbung, S., Johansson, I., Veerla, S., Jönsson, M., Bendahl, P. O., et al. (2015). Global transcriptional changes following statin treatment in breast cancer. Clin. Cancer Res. 21 (15), 3402–3411. doi:10.1158/1078-0432.CCR-14-1403
Bjørnstad, R., Reiten, I. N., Knudsen, K. S., Schjøtt, J., and Herfindal, L. (2022). A liposomal formulation of simvastatin and doxorubicin for improved cardioprotective and anti-cancer effect. Int. J. Pharm. 629, 122379. doi:10.1016/j.ijpharm.2022.122379
Bonanni, B., Puntoni, M., Cazzaniga, M., Pruneri, G., Serrano, D., Guerrieri-Gonzaga, A., et al. (2012). Dual effect of metformin on breast cancer proliferation in a randomized presurgical trial. J. Clin. Oncol. 30 (21), 2593–2600. doi:10.1200/JCO.2011.39.3769
Bray, F., Laversanne, M., Sung, H., Ferlay, J., Siegel, R. L., Soerjomataram, I., et al. (2024). Global cancer statistics 2022: GLOBOCAN estimates of incidence and mortality worldwide for 36 cancers in 185 countries. CA Cancer J. Clin. 74 (3), 229–263. doi:10.3322/caac.21834
Brown, D. N., Caffa, I., Cirmena, G., Piras, D., Garuti, A., Gallo, M., et al. (2016). Squalene epoxidase is a bona fide oncogene by amplification with clinical relevance in breast cancer. Sci. Rep. 6, 19435. doi:10.1038/srep19435
Brunet, J., Vazquez-Martin, A., Colomer, R., Graña-Suarez, B., Martin-Castillo, B., and Menendez, J. A. (2008). BRCA1 and acetyl-CoA carboxylase: the metabolic syndrome of breast cancer. Mol. Carcinog. 47 (2), 157–163. doi:10.1002/mc.20364
Brusselmans, K., De Schrijver, E., Heyns, W., Verhoeven, G., and Swinnen, J. V. (2003). Epigallocatechin-3-gallate is a potent natural inhibitor of fatty acid synthase in intact cells and selectively induces apoptosis in prostate cancer cells. Int. J. Cancer 106 (6), 856–862. doi:10.1002/ijc.11317
Carracedo, A., Cantley, L. C., and Pandolfi, P. P. (2013). Cancer metabolism: fatty acid oxidation in the limelight. Nat. Rev. Cancer 13 (4), 227–232. doi:10.1038/nrc3483
Cauley, J. A., McTiernan, A., Rodabough, R. J., LaCroix, A., Bauer, D. C., Margolis, K. L., et al. (2006). Statin use and breast cancer: prospective results from the Women's Health Initiative. J. Natl. Cancer Inst. 98 (10), 700–707. doi:10.1093/jnci/djj188
Cazzaniga, M., DeCensi, A., Pruneri, G., Puntoni, M., Bottiglieri, L., Varricchio, C., et al. (2013). The effect of metformin on apoptosis in a breast cancer presurgical trial. Br. J. Cancer 109 (11), 2792–2797. doi:10.1038/bjc.2013.657
Celestino, A. T., Levy, D., Maria Ruiz, J. L., and Bydlowski, S. P. (2015). ABCB1, ABCC1, and LRP gene expressions are altered by LDL, HDL, and serum deprivation in a human doxorubicin-resistant uterine sarcoma cell line. Biochem. Biophys. Res. Commun. 457 (4), 664–668. doi:10.1016/j.bbrc.2015.01.045
Chajès, V., Cambot, M., Moreau, K., Lenoir, G. M., and Joulin, V. (2006). Acetyl-CoA carboxylase alpha is essential to breast cancer cell survival. Cancer Res. 66 (10), 5287–5294. doi:10.1158/0008-5472.CAN-05-1489
Chalasani, P., Marron, M., Roe, D., Clarke, K., Iannone, M., Livingston, R. B., et al. (2015). A phase I clinical trial of bavituximab and paclitaxel in patients with HER2 negative metastatic breast cancer. Cancer Med. 4 (7), 1051–1059. doi:10.1002/cam4.447
Chen, Y., Li, K., Gong, D., Zhang, J., Li, Q., Zhao, G., et al. (2020). ACLY: a biomarker of recurrence in breast cancer. Pathol. Res. Pract. 216 (9), 153076. doi:10.1016/j.prp.2020.153076
Chen, Z., Xu, P., Wang, X., Li, Y., Yang, J., Xia, Y., et al. (2023). MSC-NPRA loop drives fatty acid oxidation to promote stemness and chemoresistance of gastric cancer. Cancer Lett. 565, 216235. doi:10.1016/j.canlet.2023.216235
Cheng, C., Geng, F., Cheng, X., and Guo, D. (2018). Lipid metabolism reprogramming and its potential targets in cancer. Cancer Commun. (Lond) 38 (1), 27. doi:10.1186/s40880-018-0301-4
Clendening, J. W., Pandyra, A., Boutros, P. C., El Ghamrasni, S., Khosravi, F., Trentin, G. A., et al. (2010). Dysregulation of the mevalonate pathway promotes transformation. Proc. Natl. Acad. Sci. U. S. A. 107 (34), 15051–15056. doi:10.1073/pnas.0910258107
Clendening, J. W., and Penn, L. Z. (2012). Targeting tumor cell metabolism with statins. Oncogene 31 (48), 4967–4978. doi:10.1038/onc.2012.6
Corradi, V., Sejdiu, B. I., Mesa-Galloso, H., Abdizadeh, H., Noskov, S. Y., Marrink, S. J., et al. (2019). Emerging diversity in lipid-protein interactions. Chem. Rev. 119 (9), 5775–5848. doi:10.1021/acs.chemrev.8b00451
Currie, E., Schulze, A., Zechner, R., Walther, T. C., and Farese, R. V. (2013). Cellular fatty acid metabolism and cancer. Cell Metab. 18 (2), 153–161. doi:10.1016/j.cmet.2013.05.017
Cuyàs, E., Buxó, M., Ferri Iglesias, M. J., Verdura, S., Pernas, S., Dorca, J., et al. (2019a). The C allele of ATM rs11212617 associates with higher pathological complete remission rate in breast cancer patients treated with neoadjuvant metformin. Front. Oncol. 9, 193. doi:10.3389/fonc.2019.00193
Cuyàs, E., Fernández-Arroyo, S., Buxó, M., Pernas, S., Dorca, J., Álvarez, I., et al. (2019b). Metformin induces a fasting- and antifolate-mimicking modification of systemic host metabolism in breast cancer patients. Aging (Albany NY) 11 (9), 2874–2888. doi:10.18632/aging.101960
Dankner, R., Agay, N., Olmer, L., Murad, H., Keinan Boker, L., Balicer, R. D., et al. (2019). Metformin treatment and cancer risk: cox regression analysis, with time-dependent covariates, of 320,000 persons with incident diabetes mellitus. Am. J. Epidemiol. 188 (10), 1794–1800. doi:10.1093/aje/kwz157
DeCensi, A., Puntoni, M., Gandini, S., Guerrieri-Gonzaga, A., Johansson, H. A., Cazzaniga, M., et al. (2014). Differential effects of metformin on breast cancer proliferation according to markers of insulin resistance and tumor subtype in a randomized presurgical trial. Breast Cancer Res. Treat. 148 (1), 81–90. doi:10.1007/s10549-014-3141-1
DeCensi, A., Puntoni, M., Guerrieri-Gonzaga, A., Cazzaniga, M., Serrano, D., Lazzeroni, M., et al. (2015). Effect of metformin on breast ductal carcinoma in situ proliferation in a randomized presurgical trial. Cancer Prev. Res. (Phila) 8 (10), 888–894. doi:10.1158/1940-6207.CAPR-15-0048
DeSantis, C. E., Ma, J., Gaudet, M. M., Newman, L. A., Miller, K. D., Goding Sauer, A., et al. (2019). Breast cancer statistics, 2019. CA Cancer J. Clin. 69 (6), 438–451. doi:10.3322/caac.21583
Dewidar, S. A., Hamdy, O., Eltantawy, A., El-Mesery, M., El Gayar, A. M., and Soliman, M. M. (2022). Effect of concomitant use of pitavastatin with neoadjuvant chemotherapy protocols in breast cancer patients: a randomized controlled clinical trial. Saudi Pharm. J. 30 (10), 1486–1496. doi:10.1016/j.jsps.2022.07.011
Dickerman, B. A., García-Albéniz, X., Logan, R. W., Denaxas, S., and Hernán, M. A. (2023). Evaluating metformin strategies for cancer prevention: a target trial emulation using electronic health records. Epidemiology 34 (5), 690–699. doi:10.1097/EDE.0000000000001626
Ding, X., Zhang, W., and Yang, H. (2019). The role of cholesterol metabolism in cancer. Am. J. Cancer Res. 9 (2), 219–227.
Dowling, R. J., Niraula, S., Chang, M. C., Done, S. J., Ennis, M., McCready, D. R., et al. (2015). Changes in insulin receptor signaling underlie neoadjuvant metformin administration in breast cancer: a prospective window of opportunity neoadjuvant study. Breast Cancer Res. 17 (1), 32. doi:10.1186/s13058-015-0540-0
Dyall, S. C., Balas, L., Bazan, N. G., Brenna, J. T., Chiang, N., da Costa Souza, F., et al. (2022). Polyunsaturated fatty acids and fatty acid-derived lipid mediators: recent advances in the understanding of their biosynthesis, structures, and functions. Prog. Lipid Res. 86, 101165. doi:10.1016/j.plipres.2022.101165
El-Khayat, S. M., Abouegylah, M., Abdallah, D., Geweil, A. G., Elenbaby, A. M., and Zahra, O. S. (2021). The effect of metformin when combined with neoadjuvant chemotherapy in breast cancer patients. Med. Oncol. 39 (1), 1. doi:10.1007/s12032-021-01599-3
Essa, N. M., Salem, H. F., Elgendy, M. O., Gabr, A., Omran, M. M., Hassan, N. A., et al. (2022). Efficacy of metformin as adjuvant therapy in metastatic breast cancer treatment. J. Clin. Med. 11 (19), 5505. doi:10.3390/jcm11195505
Evans, J. M., Donnelly, L. A., Emslie-Smith, A. M., Alessi, D. R., and Morris, A. D. (2005). Metformin and reduced risk of cancer in diabetic patients. Bmj 330 (7503), 1304–1305. doi:10.1136/bmj.38415.708634.F7
Falchook, G., Infante, J., Arkenau, H. T., Patel, M. R., Dean, E., Borazanci, E., et al. (2021). First-in-human study of the safety, pharmacokinetics, and pharmacodynamics of first-in-class fatty acid synthase inhibitor TVB-2640 alone and with a taxane in advanced tumors. EClinicalMedicine 34, 100797. doi:10.1016/j.eclinm.2021.100797
Farese, R. V., and Walther, T. C. (2009). Lipid droplets finally get a little R-E-S-P-E-C-T. Cell 139 (5), 855–860. doi:10.1016/j.cell.2009.11.005
Feldt, M., Bjarnadottir, O., Kimbung, S., Jirström, K., Bendahl, P. O., Veerla, S., et al. (2015). Statin-induced anti-proliferative effects via cyclin D1 and p27 in a window-of-opportunity breast cancer trial. J. Transl. Med. 13, 133. doi:10.1186/s12967-015-0486-0
Feldt, M., Menard, J., Rosendahl, A. H., Lettiero, B., Bendahl, P. O., Belting, M., et al. (2020). The effect of statin treatment on intratumoral cholesterol levels and LDL receptor expression: a window-of-opportunity breast cancer trial. Cancer Metab. 8 (1), 25. doi:10.1186/s40170-020-00231-8
Feltrin, S., Ravera, F., Traversone, N., Ferrando, L., Bedognetti, D., Ballestrero, A., et al. (2020). Sterol synthesis pathway inhibition as a target for cancer treatment. Cancer Lett. 493, 19–30. doi:10.1016/j.canlet.2020.07.010
Fendt, S. M., Frezza, C., and Erez, A. (2020). Targeting metabolic plasticity and flexibility dynamics for cancer therapy. Cancer Discov. 10 (12), 1797–1807. doi:10.1158/2159-8290.CD-20-0844
Feng, W. W., Wilkins, O., Bang, S., Ung, M., Li, J., An, J., et al. (2019). CD36-Mediated metabolic rewiring of breast cancer cells promotes resistance to HER2-targeted therapies. Cell Rep. 29 (11), 3405–3420. doi:10.1016/j.celrep.2019.11.008
Fullerton, M. D., Galic, S., Marcinko, K., Sikkema, S., Pulinilkunnil, T., Chen, Z. P., et al. (2013). Single phosphorylation sites in Acc1 and Acc2 regulate lipid homeostasis and the insulin-sensitizing effects of metformin. Nat. Med. 19 (12), 1649–1654. doi:10.1038/nm.3372
Garcia-Martinez, L., Zhang, Y., Nakata, Y., Chan, H. L., and Morey, L. (2021). Epigenetic mechanisms in breast cancer therapy and resistance. Nat. Commun. 12 (1), 1786. doi:10.1038/s41467-021-22024-3
Garwood, E. R., Kumar, A. S., Baehner, F. L., Moore, D. H., Au, A., Hylton, N., et al. (2010). Fluvastatin reduces proliferation and increases apoptosis in women with high grade breast cancer. Breast Cancer Res. Treat. 119 (1), 137–144. doi:10.1007/s10549-009-0507-x
Gerber, D. E., Horn, L., Boyer, M., Sanborn, R., Natale, R., Palmero, R., et al. (2018). Randomized phase III study of docetaxel plus bavituximab in previously treated advanced non-squamous non-small-cell lung cancer. Ann. Oncol. 29 (7), 1548–1553. doi:10.1093/annonc/mdy177
Goodwin, P. J., Chen, B. E., Gelmon, K. A., Whelan, T. J., Ennis, M., Lemieux, J., et al. (2022). Effect of metformin vs placebo on invasive disease-free survival in patients with breast cancer: the MA.32 randomized clinical trial. Jama 327 (20), 1963–1973. doi:10.1001/jama.2022.6147
Goodwin, P. J., Chen, B. E., Gelmon, K. A., Whelan, T. J., Ennis, M., Lemieux, J., et al. (2023). Effect of metformin versus placebo on new primary cancers in Canadian cancer trials group MA.32: a secondary analysis of a phase III randomized double-blind trial in early breast cancer. J. Clin. Oncol. 41 (35), 5356–5362. doi:10.1200/JCO.23.00296
Goodwin, P. J., Dowling, R. J. O., Ennis, M., Chen, B. E., Parulekar, W. R., Shepherd, L. E., et al. (2021a). Cancer antigen 15-3/mucin 1 levels in cctg MA.32: a breast cancer randomized trial of metformin vs placebo. JNCI Cancer Spectr. 5 (5), pkab066. doi:10.1093/jncics/pkab066
Goodwin, P. J., Dowling, R. J. O., Ennis, M., Chen, B. E., Parulekar, W. R., Shepherd, L. E., et al. (2021b). Effect of metformin versus placebo on metabolic factors in the MA.32 randomized breast cancer trial. NPJ Breast Cancer 7 (1), 74. doi:10.1038/s41523-021-00275-z
Goodwin, P. J., Parulekar, W. R., Gelmon, K. A., Shepherd, L. E., Ligibel, J. A., Hershman, D. L., et al. (2015). Effect of metformin vs placebo on and metabolic factors in NCIC CTG MA.32. J. Natl. Cancer Inst. 107 (3), djv006. doi:10.1093/jnci/djv006
Guo, R., Chen, Y., Borgard, H., Jijiwa, M., Nasu, M., He, M., et al. (2020). The function and mechanism of lipid molecules and their roles in the diagnosis and prognosis of breast cancer. Molecules 25 (20), 4864. doi:10.3390/molecules25204864
Hadad, S., Iwamoto, T., Jordan, L., Purdie, C., Bray, S., Baker, L., et al. (2011). Evidence for biological effects of metformin in operable breast cancer: a pre-operative, window-of-opportunity, randomized trial. Breast Cancer Res. Treat. 128 (3), 783–794. doi:10.1007/s10549-011-1612-1
Hadad, S. M., Coates, P., Jordan, L. B., Dowling, R. J. O., Chang, M. C., Done, S. J., et al. (2015). Evidence for biological effects of metformin in operable breast cancer: biomarker analysis in a pre-operative window of opportunity randomized trial. Breast Cancer Res. Treat. 150 (1), 149–155. doi:10.1007/s10549-015-3307-5
Han, S., Wei, R., Zhang, X., Jiang, N., Fan, M., Huang, J. H., et al. (2019). CPT1A/2-Mediated FAO enhancement-A metabolic target in radioresistant breast cancer. Front. Oncol. 9, 1201. doi:10.3389/fonc.2019.01201
Havas, K. M., Milchevskaya, V., Radic, K., Alladin, A., Kafkia, E., Garcia, M., et al. (2017). Metabolic shifts in residual breast cancer drive tumor recurrence. J. Clin. Invest 127 (6), 2091–2105. doi:10.1172/JCI89914
He, J., Luster, T. A., and Thorpe, P. E. (2007). Radiation-enhanced vascular targeting of human lung cancers in mice with a monoclonal antibody that binds anionic phospholipids. Clin. Cancer Res. 13 (17), 5211–5218. doi:10.1158/1078-0432.CCR-07-0793
He, W., Liang, B., Wang, C., Li, S., Zhao, Y., Huang, Q., et al. (2019). MSC-regulated lncRNA MACC1-AS1 promotes stemness and chemoresistance through fatty acid oxidation in gastric cancer. Oncogene 38 (23), 4637–4654. doi:10.1038/s41388-019-0747-0
Higgins, M. J., Prowell, T. M., Blackford, A. L., Byrne, C., Khouri, N. F., Slater, S. A., et al. (2012). A short-term biomarker modulation study of simvastatin in women at increased risk of a new breast cancer. Breast Cancer Res. Treat. 131 (3), 915–924. doi:10.1007/s10549-011-1858-7
Huang, J., Tong, Y., Hong, J., Huang, O., Wu, J., He, J., et al. (2023). Neoadjuvant docetaxel, epirubicin, and cyclophosphamide with or without metformin in breast cancer patients with metabolic abnormality: results from the randomized Phase II NeoMET trial. Breast Cancer Res. Treat. 197 (3), 525–533. doi:10.1007/s10549-022-06821-y
Huang, X., Bennett, M., and Thorpe, P. E. (2005). A monoclonal antibody that binds anionic phospholipids on tumor blood vessels enhances the antitumor effect of docetaxel on human breast tumors in mice. Cancer Res. 65 (10), 4408–4416. doi:10.1158/0008-5472.CAN-05-0031
Hundley, W. G., D'Agostino, R., Crotts, T., Craver, K., Hackney, M. H., Jordan, J. H., et al. (2022). Statins and left ventricular ejection fraction following doxorubicin treatment. NEJM Evid. 1 (9). doi:10.1056/evidoa2200097
Ismail, A., Doghish, A. S., E M Elsadek, B., Salama, S. A., and Mariee, A. D. (2020). Hydroxycitric acid potentiates the cytotoxic effect of tamoxifen in MCF-7 breast cancer cells through inhibition of ATP citrate lyase. Steroids 160, 108656. doi:10.1016/j.steroids.2020.108656
Ismail, A., Mokhlis, H. A., Sharaky, M., Sobhy, M. H., Hassanein, S. S., Doghish, A. S., et al. (2022). Hydroxycitric acid reverses tamoxifen resistance through inhibition of ATP citrate lyase. Pathol. Res. Pract. 240, 154211. doi:10.1016/j.prp.2022.154211
Jennewein, M., Lewis, M. A., Zhao, D., Tsyganov, E., Slavine, N., He, J., et al. (2008). Vascular imaging of solid tumors in rats with a radioactive arsenic-labeled antibody that binds exposed phosphatidylserine. Clin. Cancer Res. 14 (5), 1377–1385. doi:10.1158/1078-0432.CCR-07-1516
Kalinsky, K., Crew, K. D., Refice, S., Xiao, T., Wang, A., Feldman, S. M., et al. (2014). Presurgical trial of metformin in overweight and obese patients with newly diagnosed breast cancer. Cancer Invest 32 (4), 150–157. doi:10.3109/07357907.2014.889706
Kamal, A., Boerner, J., Assad, H., Chen, W., and Simon, M. S. (2024). The effect of statins on markers of breast cancer proliferation and apoptosis in women with in situ or early-stage invasive breast cancer. Int. J. Mol. Sci. 25 (17), 9587. doi:10.3390/ijms25179587
Kemp, F., Braverman, E. L., and Byersdorfer, C. A. (2024). Fatty acid oxidation in immune function. Front. Immunol. 15, 1420336. doi:10.3389/fimmu.2024.1420336
Kim, H., Seol, Y. M., Choi, Y. J., Shin, H. J., Chung, J. S., Shin, N., et al. (2019). HMG CoA reductase expression as a prognostic factor in Korean patients with breast cancer: a retrospective study. Med. Baltim. 98 (13), e14968. doi:10.1097/MD.0000000000014968
Knottnerus, S. J. G., Bleeker, J. C., Wüst, R. C. I., Ferdinandusse, S., Ijlst, L., Wijburg, F. A., et al. (2018). Disorders of mitochondrial long-chain fatty acid oxidation and the carnitine shuttle. Rev. Endocr. Metab. Disord. 19 (1), 93–106. doi:10.1007/s11154-018-9448-1
Kopecka, J., Trouillas, P., Gašparović, A. Č., Gazzano, E., Assaraf, Y. G., and Riganti, C. (2020). Phospholipids and cholesterol: inducers of cancer multidrug resistance and therapeutic targets. Drug Resist Updat 49, 100670. doi:10.1016/j.drup.2019.100670
Koundouros, N., and Poulogiannis, G. (2020). Reprogramming of fatty acid metabolism in cancer. Br. J. Cancer 122 (1), 4–22. doi:10.1038/s41416-019-0650-z
Kuemmerle, N. B., Rysman, E., Lombardo, P. S., Flanagan, A. J., Lipe, B. C., Wells, W. A., et al. (2011). Lipoprotein lipase links dietary fat to solid tumor cell proliferation. Mol. Cancer Ther. 10 (3), 427–436. doi:10.1158/1535-7163.MCT-10-0802
Kumar-Sinha, C., Ignatoski, K. W., Lippman, M. E., Ethier, S. P., and Chinnaiyan, A. M. (2003). Transcriptome analysis of HER2 reveals a molecular connection to fatty acid synthesis. Cancer Res. 63 (1), 132–139.
Kuzu, O. F., Noory, M. A., and Robertson, G. P. (2016). The role of cholesterol in cancer. Cancer Res. 76 (8), 2063–2070. doi:10.1158/0008-5472.CAN-15-2613
Lally, J. S. V., Ghoshal, S., DePeralta, D. K., Moaven, O., Wei, L., Masia, R., et al. (2019). Inhibition of acetyl-CoA carboxylase by phosphorylation or the inhibitor ND-654 suppresses lipogenesis and hepatocellular carcinoma. Cell Metab. 29 (1), 174–182. doi:10.1016/j.cmet.2018.08.020
Li, X., Qian, X., and Lu, Z. (2017). Local histone acetylation by ACSS2 promotes gene transcription for lysosomal biogenesis and autophagy. Autophagy 13 (10), 1790–1791. doi:10.1080/15548627.2017.1349581
Li, Y. J., Fahrmann, J. F., Aftabizadeh, M., Zhao, Q., Tripathi, S. C., Zhang, C., et al. (2022). Fatty acid oxidation protects cancer cells from apoptosis by increasing mitochondrial membrane lipids. Cell Rep. 39 (9), 111044. doi:10.1016/j.celrep.2022.111044
Ligorio, F., Pellegrini, I., Castagnoli, L., Vingiani, A., Lobefaro, R., Zattarin, E., et al. (2021). Targeting lipid metabolism is an emerging strategy to enhance the efficacy of anti-HER2 therapies in HER2-positive breast cancer. Cancer Lett. 511, 77–87. doi:10.1016/j.canlet.2021.04.023
Ling, R., Chen, G., Tang, X., Liu, N., Zhou, Y., and Chen, D. (2022). Acetyl-CoA synthetase 2(ACSS2): a review with a focus on metabolism and tumor development. Discov. Oncol. 13 (1), 58. doi:10.1007/s12672-022-00521-1
Liu, H., Wu, X., Dong, Z., Luo, Z., Zhao, Z., Xu, Y., et al. (2013). Fatty acid synthase causes drug resistance by inhibiting TNF-α and ceramide production. J. Lipid Res. 54 (3), 776–785. doi:10.1194/jlr.M033811
Liu, J., Wang, H., Zhang, M., Li, Y., Wang, R., Chen, H., et al. (2023). Metformin and simvastatin synergistically suppress endothelin 1-induced hypoxia and angiogenesis in multiple cancer types. Cancer Sci. 114 (2), 640–653. doi:10.1111/cas.15602
Liu, S., Zhang, X., Wang, W., Li, X., Sun, X., Zhao, Y., et al. (2024). Metabolic reprogramming and therapeutic resistance in primary and metastatic breast cancer. Mol. Cancer 23 (1), 261. doi:10.1186/s12943-024-02165-x
Lopez-Bonet, E., Buxó, M., Cuyàs, E., Pernas, S., Dorca, J., Álvarez, I., et al. (2019). Neoadjuvant metformin added to systemic therapy decreases the proliferative capacity of residual breast cancer. J. Clin. Med. 8 (12), 2180. doi:10.3390/jcm8122180
Luis, G., Godfroid, A., Nishiumi, S., Cimino, J., Blacher, S., Maquoi, E., et al. (2021). Tumor resistance to ferroptosis driven by Stearoyl-CoA Desaturase-1 (SCD1) in cancer cells and Fatty Acid Biding Protein-4 (FABP4) in tumor microenvironment promote tumor recurrence. Redox Biol. 43, 102006. doi:10.1016/j.redox.2021.102006
Luo, J., Yang, H., and Song, B. L. (2020). Mechanisms and regulation of cholesterol homeostasis. Nat. Rev. Mol. Cell Biol. 21 (4), 225–245. doi:10.1038/s41580-019-0190-7
Makhlin, I., Demissei, B. G., D'Agostino, R., Hundley, W. G., Baleanu-Gogonea, C., Wilcox, N. S., et al. (2024). Statins do not significantly affect oxidative nitrosative stress biomarkers in the PREVENT randomized clinical trial. Clin. Cancer Res. 30 (11), 2370–2376. doi:10.1158/1078-0432.CCR-23-3952
Manni, A., Richie, J. P., Schetter, S. E., Calcagnotto, A., Trushin, N., Aliaga, C., et al. (2017). Stearoyl-CoA desaturase-1, a novel target of omega-3 fatty acids for reducing breast cancer risk in obese postmenopausal women. Eur. J. Clin. Nutr. 71 (6), 762–765. doi:10.1038/ejcn.2016.273
Martin-Castillo, B., Pernas, S., Dorca, J., Álvarez, I., Martínez, S., Pérez-Garcia, J. M., et al. (2018). A phase 2 trial of neoadjuvant metformin in combination with trastuzumab and chemotherapy in women with early HER2-positive breast cancer: the METTEN study. Oncotarget 9 (86), 35687–35704. doi:10.18632/oncotarget.26286
Martin-Perez, M., Urdiroz-Urricelqui, U., Bigas, C., and Benitah, S. A. (2022). The role of lipids in cancer progression and metastasis. Cell Metab. 34 (11), 1675–1699. doi:10.1016/j.cmet.2022.09.023
McGowan, M. M., Eisenberg, B. L., Lewis, L. D., Froehlich, H. M., Wells, W. A., Eastman, A., et al. (2013). A proof of principle clinical trial to determine whether conjugated linoleic acid modulates the lipogenic pathway in human breast cancer tissue. Breast Cancer Res. Treat. 138 (1), 175–183. doi:10.1007/s10549-013-2446-9
Menendez, J. A., and Lupu, R. (2017). Fatty acid synthase (FASN) as a therapeutic target in breast cancer. Expert Opin. Ther. Targets 21 (11), 1001–1016. doi:10.1080/14728222.2017.1381087
Menendez, J. A., Papadimitropoulou, A., Vander Steen, T., Cuyàs, E., Oza-Gajera, B. P., Verdura, S., et al. (2021). Fatty acid synthase confers tamoxifen resistance to ER+/HER2+ breast cancer. Cancers (Basel) 13 (5), 1132. doi:10.3390/cancers13051132
Muszyńska-Ogłaza, A., Zarzycka-Lindner, G., Olejniczak, H., Polaszewska-Muszyńska, M., and Junik, R. (2017). Use of metformin is associated with lower incidence of cancer in patients with type 2 diabetes. Endokrynol. Pol. 68 (6), 652–658. doi:10.5603/EP.a2017.0054
Nanni, O., Amadori, D., De Censi, A., Rocca, A., Freschi, A., Bologna, A., et al. (2019). Metformin plus chemotherapy versus chemotherapy alone in the first-line treatment of HER2-negative metastatic breast cancer. The MYME randomized, phase 2 clinical trial. Breast Cancer Res. Treat. 174 (2), 433–442. doi:10.1007/s10549-018-05070-2
Nelson, E. R., Chang, C. Y., and McDonnell, D. P. (2014). Cholesterol and breast cancer pathophysiology. Trends Endocrinol. Metab. 25 (12), 649–655. doi:10.1016/j.tem.2014.10.001
Nielsen, S. F., Nordestgaard, B. G., and Bojesen, S. E. (2012). Statin use and reduced cancer-related mortality. N. Engl. J. Med. 367 (19), 1792–1802. doi:10.1056/NEJMoa1201735
Niraula, S., Dowling, R. J. O., Ennis, M., Chang, M. C., Done, S. J., Hood, N., et al. (2012). Metformin in early breast cancer: a prospective window of opportunity neoadjuvant study. Breast Cancer Res. Treat. 135 (3), 821–830. doi:10.1007/s10549-012-2223-1
Olzmann, J. A., and Carvalho, P. (2019). Dynamics and functions of lipid droplets. Nat. Rev. Mol. Cell Biol. 20 (3), 137–155. doi:10.1038/s41580-018-0085-z
Orlando, U. D., Castillo, A. F., Medrano, M. A. R., Solano, A. R., Maloberti, P. M., and Podesta, E. J. (2019). Acyl-CoA synthetase-4 is implicated in drug resistance in breast cancer cell lines involving the regulation of energy-dependent transporter expression. Biochem. Pharmacol. 159, 52–63. doi:10.1016/j.bcp.2018.11.005
Padegimas, A., Clasen, S., and Ky, B. (2020). Cardioprotective strategies to prevent breast cancer therapy-induced cardiotoxicity. Trends Cardiovasc Med. 30 (1), 22–28. doi:10.1016/j.tcm.2019.01.006
Pascual, G., Avgustinova, A., Mejetta, S., Martín, M., Castellanos, A., Attolini, C. S. O., et al. (2017). Targeting metastasis-initiating cells through the fatty acid receptor CD36. Nature 541 (7635), 41–45. doi:10.1038/nature20791
Pecoraro, M., Marzocco, S., Belvedere, R., Petrella, A., Franceschelli, S., and Popolo, A. (2023). Simvastatin reduces doxorubicin-induced cardiotoxicity: effects beyond its antioxidant activity. Int. J. Mol. Sci. 24 (8), 7573. doi:10.3390/ijms24087573
Pernicova, I., and Korbonits, M. (2014). Metformin--mode of action and clinical implications for diabetes and cancer. Nat. Rev. Endocrinol. 10 (3), 143–156. doi:10.1038/nrendo.2013.256
Petrenko, V., Sinturel, F., Riezman, H., and Dibner, C. (2023). Lipid metabolism around the body clocks. Prog. Lipid Res. 91, 101235. doi:10.1016/j.plipres.2023.101235
Pimentel, I., Chen, B. E., Lohmann, A. E., Ennis, M., Ligibel, J., Shepherd, L., et al. (2021). The effect of metformin vs placebo on sex hormones in Canadian cancer trials group MA.32. J. Natl. Cancer Inst. 113 (2), 192–198. doi:10.1093/jnci/djaa082
Pimentel, I., Lohmann, A. E., Ennis, M., Dowling, R. J. O., Cescon, D., Elser, C., et al. (2019). A phase II randomized clinical trial of the effect of metformin versus placebo on progression-free survival in women with metastatic breast cancer receiving standard chemotherapy. Breast 48, 17–23. doi:10.1016/j.breast.2019.08.003
Pucer, A., Brglez, V., Payré, C., Pungerčar, J., Lambeau, G., and Petan, T. (2013). Group X secreted phospholipase A(2) induces lipid droplet formation and prolongs breast cancer cell survival. Mol. Cancer 12 (1), 111. doi:10.1186/1476-4598-12-111
Qiu, Y., Wang, X., Sun, Y., Jin, T., Tang, R., Zhou, X., et al. (2024). ACSL4-Mediated membrane phospholipid remodeling induces integrin β1 activation to facilitate triple-negative breast cancer metastasis. Cancer Res. 84 (11), 1856–1871. doi:10.1158/0008-5472.CAN-23-2491
Ricco, N., and Kron, S. J. (2023). Statins in cancer prevention and therapy. Cancers (Basel) 15 (15), 3948. doi:10.3390/cancers15153948
Rocca, A., Cortesi, P., Cortesi, L., Gianni, L., Matteucci, F., Fantini, L., et al. (2021). Phase II study of liposomal doxorubicin, docetaxel and trastuzumab in combination with metformin as neoadjuvant therapy for HER2-positive breast cancer. Ther. Adv. Med. Oncol. 13, 1758835920985632. doi:10.1177/1758835920985632
Rossi Sebastiano, M., and Konstantinidou, G. (2019). Targeting long chain acyl-CoA synthetases for cancer therapy. Int. J. Mol. Sci. 20 (15), 3624. doi:10.3390/ijms20153624
Samovski, D., Jacome-Sosa, M., and Abumrad, N. A. (2023). Fatty acid transport and signaling: mechanisms and physiological implications. Annu. Rev. Physiol. 85, 317–337. doi:10.1146/annurev-physiol-032122-030352
Sardesai, S. D., Thomas, A., Gallagher, C., Lynce, F., Ottaviano, Y. L., Ballinger, T. J., et al. (2021). Inhibiting fatty acid synthase with omeprazole to improve efficacy of neoadjuvant chemotherapy in patients with operable TNBC. Clin. Cancer Res. 27 (21), 5810–5817. doi:10.1158/1078-0432.CCR-21-0493
Schug, Z. T., Peck, B., Jones, D. T., Zhang, Q., Grosskurth, S., Alam, I. S., et al. (2015). Acetyl-CoA synthetase 2 promotes acetate utilization and maintains cancer cell growth under metabolic stress. Cancer Cell 27 (1), 57–71. doi:10.1016/j.ccell.2014.12.002
Serageldin, M. A., El-Bassiouny, N. A., El-Kerm, Y., Aly, R. G., Helmy, M. W., El-Mas, M. M., et al. (2024). A randomized controlled study of neoadjuvant metformin with chemotherapy in nondiabetic breast cancer women: the METNEO study. Br. J. Clin. Pharmacol. 90, 3160–3175. doi:10.1111/bcp.16193
Serageldin, M. A., Kassem, A. B., El-Kerm, Y., Helmy, M. W., El-Mas, M. M., and El-Bassiouny, N. A. (2023). The effect of metformin on chemotherapy-induced toxicities in non-diabetic breast cancer patients: a randomised controlled study. Drug Saf. 46 (6), 587–599. doi:10.1007/s40264-023-01305-4
Shim, J. K., Choi, S., Yoon, S. J., Choi, R. J., Park, J., Lee, E. H., et al. (2022). Etomoxir, a carnitine palmitoyltransferase 1 inhibitor, combined with temozolomide reduces stemness and invasiveness in patient-derived glioblastoma tumorspheres. Cancer Cell Int. 22 (1), 309. doi:10.1186/s12935-022-02731-7
Svensson, R. U., Parker, S. J., Eichner, L. J., Kolar, M. J., Wallace, M., Brun, S. N., et al. (2016). Inhibition of acetyl-CoA carboxylase suppresses fatty acid synthesis and tumor growth of non-small-cell lung cancer in preclinical models. Nat. Med. 22 (10), 1108–1119. doi:10.1038/nm.4181
Tan, Z., Xiao, L., Tang, M., Bai, F., Li, J., Li, L., et al. (2018). Targeting CPT1A-mediated fatty acid oxidation sensitizes nasopharyngeal carcinoma to radiation therapy. Theranostics 8 (9), 2329–2347. doi:10.7150/thno.21451
Tan, Z., Zou, Y., Zhu, M., Luo, Z., Wu, T., Zheng, C., et al. (2021). Carnitine palmitoyl transferase 1A is a novel diagnostic and predictive biomarker for breast cancer. BMC Cancer 21 (1), 409. doi:10.1186/s12885-021-08134-7
Tapia, E., Villa-Guillen, D. E., Chalasani, P., Centuori, S., Roe, D. J., Guillen-Rodriguez, J., et al. (2021). A randomized controlled trial of metformin in women with components of metabolic syndrome: intervention feasibility and effects on adiposity and breast density. Breast Cancer Res. Treat. 190 (1), 69–78. doi:10.1007/s10549-021-06355-9
Tauchi-Sato, K., Ozeki, S., Houjou, T., Taguchi, R., and Fujimoto, T. (2002). The surface of lipid droplets is a phospholipid monolayer with a unique Fatty Acid composition. J. Biol. Chem. 277 (46), 44507–44512. doi:10.1074/jbc.M207712200
Thavendiranathan, P., Houbois, C., Marwick, T. H., Kei, T., Saha, S., Runeckles, K., et al. (2023). Statins to prevent early cardiac dysfunction in cancer patients at increased cardiotoxicity risk receiving anthracyclines. Eur. Heart J. Cardiovasc Pharmacother. 9 (6), 515–525. doi:10.1093/ehjcvp/pvad031
Thompson, A. M. (2014). Molecular pathways: preclinical models and clinical trials with metformin in breast cancer. Clin. Cancer Res. 20 (10), 2508–2515. doi:10.1158/1078-0432.CCR-13-0354
Tong, L. (2005). Acetyl-coenzyme A carboxylase: crucial metabolic enzyme and attractive target for drug discovery. Cell Mol. Life Sci. 62 (16), 1784–1803. doi:10.1007/s00018-005-5121-4
Tseng, C. H. (2014). Metformin may reduce breast cancer risk in Taiwanese women with type 2 diabetes. Breast Cancer Res. Treat. 145 (3), 785–790. doi:10.1007/s10549-014-2985-8
Vance, J. E. (2015). Phospholipid synthesis and transport in mammalian cells. Traffic 16 (1), 1–18. doi:10.1111/tra.12230
Vasseur, S., and Guillaumond, F. (2022). Lipids in cancer: a global view of the contribution of lipid pathways to metastatic formation and treatment resistance. Oncogenesis 11 (1), 46. doi:10.1038/s41389-022-00420-8
Vazquez-Martin, A., Colomer, R., Brunet, J., Lupu, R., and Menendez, J. A. (2008). Overexpression of fatty acid synthase gene activates HER1/HER2 tyrosine kinase receptors in human breast epithelial cells. Cell Prolif. 41 (1), 59–85. doi:10.1111/j.1365-2184.2007.00498.x
Velez, B. C., Petrella, C. P., DiSalvo, K. H., Cheng, K., Kravtsov, R., Krasniqi, D., et al. (2023). Combined inhibition of ACLY and CDK4/6 reduces cancer cell growth and invasion. Oncol. Rep. 49 (2), 32. doi:10.3892/or.2022.8469
Vinayak, S., Schwartz, E. J., Jensen, K., Lipson, J., Alli, E., McPherson, L., et al. (2013). A clinical trial of lovastatin for modification of biomarkers associated with breast cancer risk. Breast Cancer Res. Treat. 142 (2), 389–398. doi:10.1007/s10549-013-2739-z
Wang, J., Xiang, H., Lu, Y., Wu, T., and Ji, G. (2021). The role and therapeutic implication of CPTs in fatty acid oxidation and cancers progression. Am. J. Cancer Res. 11 (6), 2477–2494.
Wang, J., Zhang, W., Liu, C., Wang, L., Wu, J., Sun, C., et al. (2024). Reprogramming of lipid metabolism mediates crosstalk, remodeling, and intervention of microenvironment components in breast cancer. Int. J. Biol. Sci. 20 (5), 1884–1904. doi:10.7150/ijbs.92125
Wang, T., Fahrmann, J. F., Lee, H., Li, Y. J., Tripathi, S. C., Yue, C., et al. (2018). JAK/STAT3-Regulated fatty acid β-oxidation is critical for breast cancer stem cell self-renewal and chemoresistance. Cell Metab. 27 (1), 136–150.e5. doi:10.1016/j.cmet.2018.04.018
Wu, X., Dong, Z., Wang, C. J., Barlow, L. J., Fako, V., Serrano, M. A., et al. (2016). FASN regulates cellular response to genotoxic treatments by increasing PARP-1 expression and DNA repair activity via NF-κB and SP1. Proc. Natl. Acad. Sci. U. S. A. 113 (45), E6965-E6973–e6973. doi:10.1073/pnas.1609934113
Wu, X., Li, Y., Wang, J., Wen, X., Marcus, M. T., Daniels, G., et al. (2013). Long chain fatty Acyl-CoA synthetase 4 is a biomarker for and mediator of hormone resistance in human breast cancer. PLoS One 8 (10), e77060. doi:10.1371/journal.pone.0077060
Xiao, Q., Xia, M., Tang, W., Zhao, H., Chen, Y., and Zhong, J. (2024). The lipid metabolism remodeling: a hurdle in breast cancer therapy. Cancer Lett. 582, 216512. doi:10.1016/j.canlet.2023.216512
Yam, C., Esteva, F. J., Patel, M. M., Raghavendra, A. S., Ueno, N. T., Moulder, S. L., et al. (2019). Efficacy and safety of the combination of metformin, everolimus and exemestane in overweight and obese postmenopausal patients with metastatic, hormone receptor-positive, HER2-negative breast cancer: a phase II study. Invest New Drugs 37 (2), 345–351. doi:10.1007/s10637-018-0700-z
Yu, W., Lei, Q., Yang, L., Qin, G., Liu, S., Wang, D., et al. (2021). Contradictory roles of lipid metabolism in immune response within the tumor microenvironment. J. Hematol. Oncol. 14 (1), 187. doi:10.1186/s13045-021-01200-4
Yulian, E. D., Siregar, N. C., and Bajuadji, (2021). Combination of simvastatin and FAC improves response to neoadjuvant chemotherapy in locally advanced breast cancer. Cancer Res. Treat. 53 (4), 1072–1083. doi:10.4143/crt.2020.1024
Yulian, E. D., Siregar, N. C., Sudijono, B., and Hwei, L. R. Y. (2023). The role of HMGCR expression in combination therapy of simvastatin and FAC treated locally advanced breast cancer patients. Breast Dis. 42 (1), 73–83. doi:10.3233/BD-220021
Zhang, N., Zhang, H., Liu, Y., Su, P., Zhang, J., Wang, X., et al. (2019). SREBP1, targeted by miR-18a-5p, modulates epithelial-mesenchymal transition in breast cancer via forming a co-repressor complex with Snail and HDAC1/2. Cell Death Differ. 26 (5), 843–859. doi:10.1038/s41418-018-0158-8
Zhao, H., Zhu, W., Zhao, X., Li, X., Zhou, Z., Zheng, M., et al. (2022). Efficacy of epigallocatechin-3-gallate in preventing dermatitis in patients with breast cancer receiving postoperative radiotherapy: a double-blind, placebo-controlled, phase 2 randomized clinical trial. JAMA Dermatol 158 (7), 779–786. doi:10.1001/jamadermatol.2022.1736
Zhao, J., Zhi, Z., Wang, C., Xing, H., Song, G., Yu, X., et al. (2017a). Exogenous lipids promote the growth of breast cancer cells via CD36. Oncol. Rep. 38 (4), 2105–2115. doi:10.3892/or.2017.5864
Zhao, Y., Gong, C., Wang, Z., Zhang, J., Wang, L., Zhang, S., et al. (2017b). A randomized phase II study of aromatase inhibitors plus metformin in pre-treated postmenopausal patients with hormone receptor positive metastatic breast cancer. Oncotarget 8 (48), 84224–84236. doi:10.18632/oncotarget.20478
Zheng, R. S., Chen, R., Han, B. F., Wang, S. M., Li, L., Sun, K. X., et al. (2024). Cancer incidence and mortality in China, 2022. Zhonghua Zhong Liu Za Zhi 46, 221–231. doi:10.3760/cma.j.cn112152-20240119-00035
Zhou, Y., Guo, X., Shen, L., Liu, K., Sun, Q., Wang, Y., et al. (2023). Predictive significance of systemic immune-inflammation index in patients with breast cancer: a retrospective cohort study. Onco Targets Ther. 16, 939–960. doi:10.2147/OTT.S434193
Zhou, Y., Yao, Y., Deng, Y., and Shao, A. (2020). Regulation of efferocytosis as a novel cancer therapy. Cell Commun. Signal 18 (1), 71. doi:10.1186/s12964-020-00542-9
Zipinotti Dos Santos, D., de Souza, J. C., Pimenta, T. M., da Silva Martins, B., Junior, R. S. R., Butzene, S. M. S., et al. (2023). The impact of lipid metabolism on breast cancer: a review about its role in tumorigenesis and immune escape. Cell Commun. Signal 21 (1), 161. doi:10.1186/s12964-023-01178-1
Keywords: breast cancer, lipid metabolism reprogramming, clinical trial, targeted therapy, predictive biomarkers, hypothesis
Citation: Li D, Jin P, Cai Y, Wu S, Guo X, Zhang Z, Liu K, Li P, Hu Y and Zhou Y (2025) Clinical significance of lipid pathway-targeted therapy in breast cancer. Front. Pharmacol. 15:1514811. doi: 10.3389/fphar.2024.1514811
Received: 21 October 2024; Accepted: 17 December 2024;
Published: 06 January 2025.
Edited by:
Zhendong Shi, Tianjin Medical University Cancer Institute and Hospital, ChinaReviewed by:
Wei Yang, Stony Brook University, United StatesPeiguo Shi, Columbia University, United States
Copyright © 2025 Li, Jin, Cai, Wu, Guo, Zhang, Liu, Li, Hu and Zhou. This is an open-access article distributed under the terms of the Creative Commons Attribution License (CC BY). The use, distribution or reproduction in other forums is permitted, provided the original author(s) and the copyright owner(s) are credited and that the original publication in this journal is cited, in accordance with accepted academic practice. No use, distribution or reproduction is permitted which does not comply with these terms.
*Correspondence: Yunxiang Zhou, eXh6aG91QHpqdS5lZHUuY24=; Yue Hu, aHV5dWV6anVAemp1LmVkdS5jbg==
†These authors have contributed equally to this work