- 1Department of Biliary-Pancreatic Surgery, Renji Hospital Affiliated to Shanghai Jiao Tong University School of Medicine, Shanghai, China
- 2Shanghai Key Laboratory of Biliary Tract Disease Research, Shanghai, China
- 3State Key Laboratory of Oncogenes and Related Genes, Shanghai, China
- 4Shanghai Research Center of Biliary Tract Disease, Shanghai, China
Bile acids (BAs) constitute essential components of cholesterol metabolites that are synthesized in the liver, stored in the gallbladder, and excreted into the intestine through the biliary system. They play a crucial role in nutrient absorption, lipid and glucose regulation, and the maintenance of metabolic homeostasis. In additional, BAs have demonstrated the ability to attenuate disease progression such as diabetes, metabolic disorders, heart disease, and respiratory ailments. Intriguingly, recent research has offered exciting evidence to unveil their potential antitumor properties against various cancer cell types including tamoxifen-resistant breast cancer, oral squamous cell carcinoma, cholangiocarcinoma, gastric cancer, colon cancer, hepatocellular carcinoma, prostate cancer, gallbladder cancer, neuroblastoma, and others. Up to date, multiple laboratories have synthesized novel BA derivatives to develop potential drug candidates. These derivatives have exhibited the capacity to induce cell death in individual cancer cell types and display promising anti-tumor activities. This review extensively elucidates the anticancer activity of natural BAs and synthetic derivatives in cancer cells, their associated signaling pathways, and therapeutic strategies. Understanding of BAs and their derivatives activities and action mechanisms will evidently assist anticancer drug discovery and devise novel treatment.
1 Introduction
Bile acids (BAs) are physiological metabolites that are synthesized in the liver, stored in the gallbladder, and excreted into the intestine through the biliary system (Chiang and Ferrell, 2019). BAs participate in the nutrient absorption and secretion, and regulate lipids and glucose metabolism, thus maintaining metabolic homeostasis (Collins et al., 2023). Although BAs regulate intestinal flora growth, the intestinal flora can in turn metabolize BAs and control their composition and storage in the enterohepatic circulation through an enterohepatic circulation. A number of factors including fasting and ingesting specific nutrients can regulate BA synthesis, intestinal flora composition, and blood circulation hormones to keep systemic metabolic homeostasis and prevent from BA-associated metabolic diseases. Activation of BA receptor signaling offers protection to the gastrointestinal tract against inflammation and damage. Furthermore, various factors, including gene mutations for the BA synthesis and transport, high-fat diets, medications, and circadian rhythm disturbances, are found to mediate the pathologies of multiple diseases that involve cholestatic liver disease, inflammatory bowel disease, diabetes, obesity, tumors, and related metabolic disorders (Li and Chiang, 2014; Fiorucci et al., 2021; Fu et al., 2021; Perino et al., 2021; Yang et al., 2021; Shi et al., 2023). In recent years, several researches have demonstrated that BAs have antitumor properties in various cancer cell types, such as tamoxifen-resistant breast cancer (Luu et al., 2018; Kovács et al., 2019), oral squamous cell carcinoma (Talebian et al., 2020), cholangiocarcinoma (Lee et al., 2022), gastric cancer (Zhang et al., 2022), colon cancer (Kim E. K. et al., 2017), hepatocellular carcinoma (Fan et al., 2023), prostate cancer (Lee et al., 2017), gallbladder cancer (Lin et al., 2020; Li et al., 2022), neuroblastoma (Trah et al., 2020) etc., by inhibiting cancer cell proliferation and migration. In addition, new BA derivatives have been synthesized in several laboratories to investigate their anticancer properties. These derivatives were demonstrated to trigger cell death in cancer cells and exhibit anti-tumor properties (Katona et al., 2009; Sreekanth et al., 2013; Tang et al., 2018; Markov et al., 2019; Agarwal et al., 2021; Melloni et al., 2022). This review discusses the anticancer activity of natural BAs and synthetic derivatives in cancer cells and their signaling pathways and therapeutic approaches potentially targeted to human cancers.
2 Bile acid biosynthesis
BAs are the final products of cholesterol catabolism in the liver and consist of a variety of lipid-soluble acids, including cholic acid (CA), deoxycholic acid (DCA), chenodeoxycholic acid (CDCA), glycochenodeoxycholic acid (GCDCA), ursodeoxycholic acid (UDCA), glycoursodeoxycholic acid (GUDCA), glycodeoxycholic acid (GDCA), glycocholic acid (GCA), taurocholic acid (TCA), taurochenodeoxycholic acid (TCDCA), tauroursodeoxycholic acid (TUDCA), taurodeoxycholic acid (TDCA), lithocholic acid (LCA), glycolithocholic acid (GLCA), and taurolithocholic acid (TLCA) (Li et al., 2022). BAs have two main ways of biosynthesis: classical and alternative synthetic pathways (Figure 1) (Chiang, 2009). The microsomal rate-limiting enzyme cholesterol 7α-hydroxylase (CYP7A1) initiates the classical BA synthesis pathway by which CYP7A1 oxidizes cholesterol into 7α-hydroxycholesterol. Subsequently, 3β-hydroxy∆5-C27-steroid dehydrogenase (HSD3B7) catalyzes the conversion of 7α-hydroxycholesterol to 7α-hydroxy-4-cholesten-3-one (C4), a precursor of the primary BAs, CA and CDCA. C4 also serves as a common serum biomarker used to evaluate levels of BA synthesis. Microsome sterol 12α-hydroxylase (CYP8B1) can convert C4 to 7α, 12α-dihydroxy-4-cholestene 3-1thatisfurther metabolized to be a precursor of the CA 3-alpha, 7-alpha, 12-alpha trihydroxycholestanoicacid (THCA) by aldo-keto reductases (AKR) AKR1D1/1C4 and mitochondrial sterol cholesterol 27-hydroxylase (CYP27A1). In the absence of 12α-hydroxylation, C4 undergoes conversion into 3α, 7α dihydroxycholestanoic acid (DHCA), which serves as the precursor for CDCA. THCA and DHCA are transported to peroxisomes for steroid side chain cleavage, which occurs similarly to fatty acid β-oxidation.
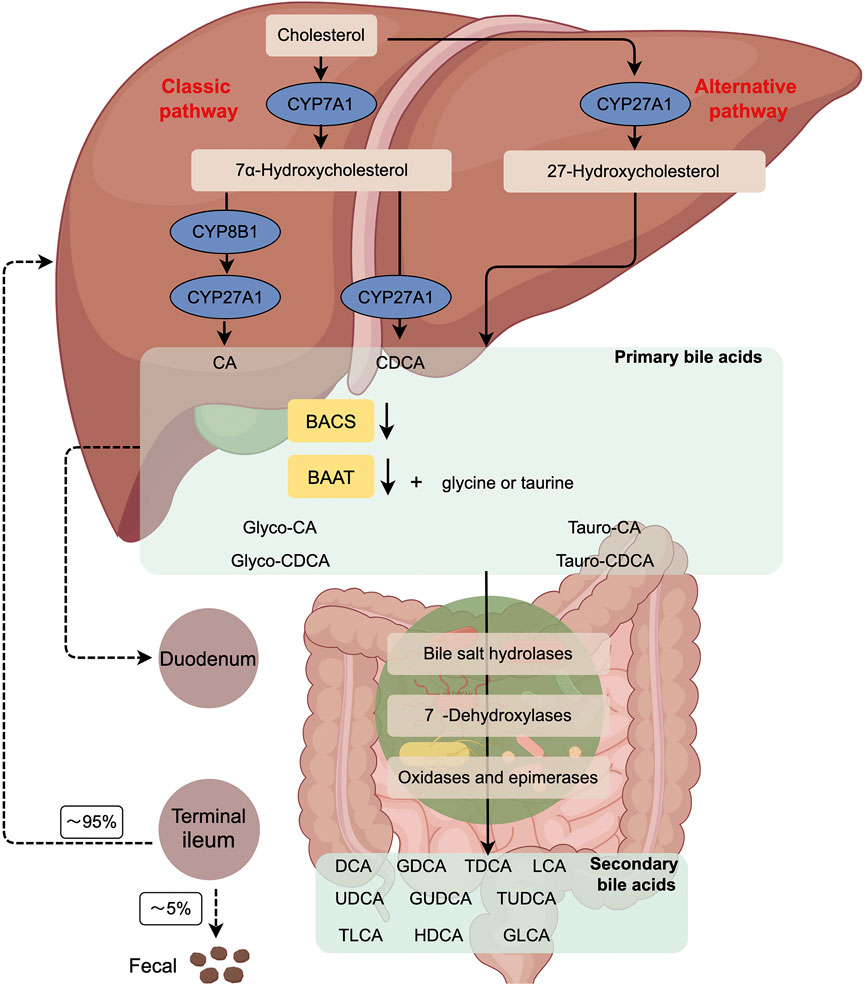
FIGURE 1. The Diagram of the classical and alternative bile acid synthesis in human. Primary BAs are generated from cholesterol by the classic (CYP7A1-mediated) or alternative (CYP27A1-mediated) pathway. Subsequently, BACS and BAAT catalyze the conjugation of BAs with glycine or taurine in the liver, resulting in the formation of bile salts. Once released into the gut, these bile acids undergo modification by the gut microbiome, leading to the production of secondary BAs. Approximately 95% of the BAs that reach the terminal ileum are reabsorbed, allowing for their recycling by the liver. CYP7A1, cholesterol 7α-hydroxylase; CYP27A1, sterol 27- hydroxylase; BACS, BA-CoA synthetase; BAAT, BA-CoA: amino acid N-acyltransferase; CA, cholic acid; CDCA, chenodeoxycholic acid; CYP8B1, sterol12α-hydroxylase.
Initially, BA coenzyme A (CoA) synthase (BACS; SLC27A5) catalyzes THCA and DHCA into acyl-CoA thioesters. Subsequently, these thioesters are transported to peroxisomes through the peroxisomal BA-acyl transporter ABCD3. Among them, α-methylacyl-CoA racemase (AMACR), acyl-CoA oxidase (ACOX2), and D-bifunctional enzyme (ACOX2) are the most common enzymes. HSD17B4 completes the racemization, hydration, and dehydration steps. Finally, the sterol carrier protein x (SCPx) cleans releases propanoyl-coA from the steroid side chains of THCA and DHCA to form cholyl-coA and chenodeoxycholyl-coA, respectively. BA-coA: amino acid N-acyltransferase (BAAT) couples cholyl-coA and CDCA-coA to taurine or glycine to form T/G-CA and T/G-CDCA, respectively (Perino et al., 2021).
In the alternative synthetic pathway, CYP27A1 is crucial in converting cholesterol to 27-hydroxycholesterol and 3β-hydroxy-5-cholesterol in the liver, macrophages, and adrenal glands. Oxysterol 7α-hydroxylase (CYP7B1) hydroxylates C7, resulting in the formation of 7α, 27-dihydroxycholesterol and 3β, 7α-dihydroxy-5 cholestenoic acid. In the brain, cholesterol is converted to 24-hydroxycholesterol by the enzyme sterol 24-hydroxylase (CYP46A1), which is then hydroxylated at the 7α position by a specific sterol 7α-hydroxylase (CYP39A1) in the liver. The oxysterols generated in extrahepatic tissues can serve as substrates for synthesizing CDCA and CA.
Negative feedback mechanisms tightly regulate classical and alternative BA synthesis pathways (Di Ciaula et al., 2017; Collins et al., 2023). In human, the synthesis of BAs is primarily derived from the classical pathway, whereas approximately 50% of BAs in rodents are synthesized from the alternative pathway. CA and CDCA are the two primary BAs synthesized in the human liver. CDCA, a hydrophobic BA, undergoes further conversion to α-muricholic acid (α-MCA) by a mouse-specific enzyme sterol-6β-hydroxylase (Cyp2c70). Furthermore, α-MCA can be epimerized to be 7β-epimer, known as β-MCA. Cyp2c70 also hydroxylizes the secondary BA UDCA produced by gut bacteria to β-MCA. α-MCA and β-MCA are the primary BAs produced in rodent liver and are highly water-soluble and non-toxic. In human, bacterial 7β-hydroxysteroid dehydrogenase (7β-HSDH) converts merely 2% of CDCA as a secondary BA to the 7β-epidermoid UDCA that is a highly water-soluble and non-toxic BA.
3 The anticancer effect of natural BAs
BAs are typically appreciated as major signal molecules that act as emulsifiers in dietary lipid digestion and absorption (Melloni et al., 2022). Interestingly, they are found to intervene the development of diabetes, metabolic disorders, heart disease, respiratory ailments, and tumors (Collins et al., 2023; Shi et al., 2023). In this section, our attention primarily focuses on exploring the anticancer impacts of natural BAs (Figure 2.) on cancer cells in vitro (e.g., proliferation, invasion, migration, and adhesion) (Table 1).
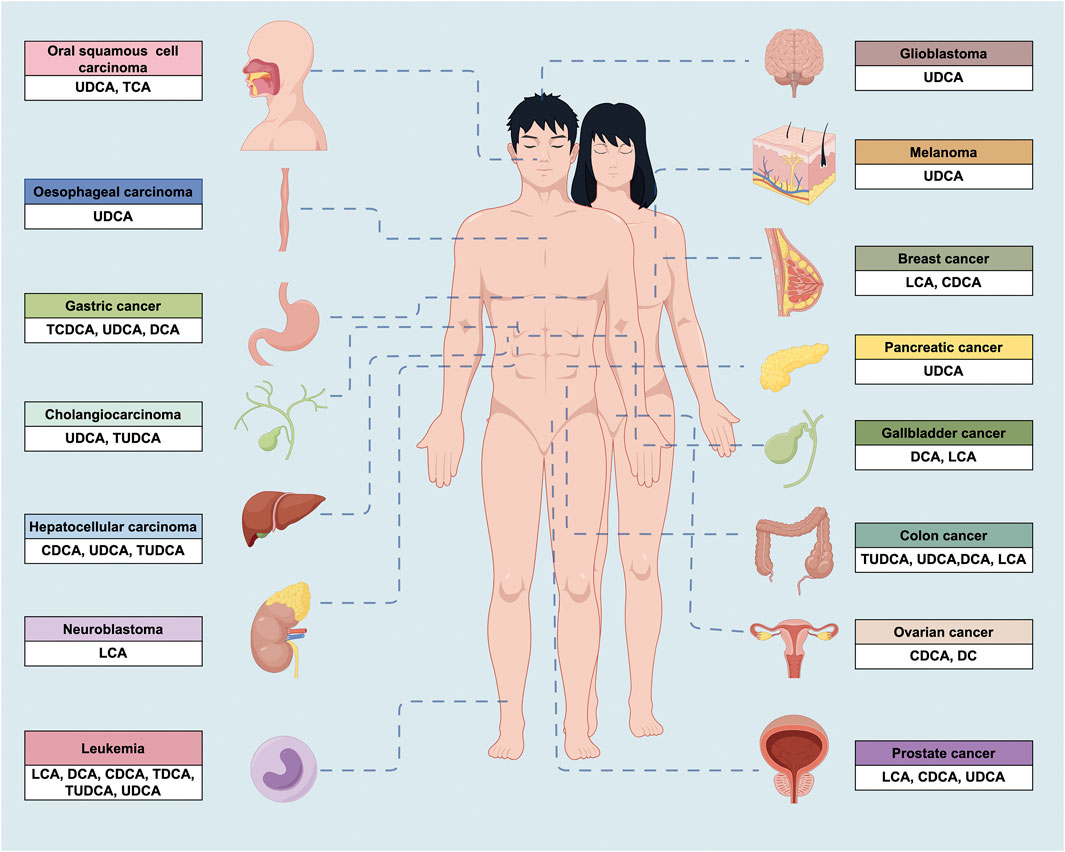
FIGURE 2. The anticancer properties of BAs in a wide variety of cancers. UDCA, ursodeoxycholic acid; TCA, taurocholic acid; TCDCA, taurochenodeoxycholic acid; DCA, deoxycholic acid; TUDCA, tauroursodeoxycholic acid; CDCA, chenodeoxycholic acid; LCA, lithocholic acid; TDCA, taurodeoxycholic acid.
3.1 Glioblastoma (GB)
Glioblastoma (GB) is the most prevalent and aggressive form of adult human brain tumor. Despite the implementation of aggressive regimens involving surgery, radiation and chemotherapy, the prognosis for GBM patients remains poor with a median survival of 15 months (Schaff and Mellinghoff, 2023). UDCA demonstrates the ability to penetrate through the blood-brain barrier; thus it implicates powerful activity to block brain tumor (Palmela et al., 2015). Yao et al. (2020) demonstrated that UDCA inhibited GB progression in multiple aspects such as inducing G1 phase arrest, reducing mitochondrial membrane potential (MMP), promoting overproduction of reactive oxygen species (ROS), and inducing endoplasmic reticulum (ER) stress. Combining UDCA with bortezomib (BTZ) also synergistically enhances the PERK/ATF4/CHOP pathway and protracts ER stress (Yao et al., 2020).
3.2 Neuroblastoma (NB)
Nephroblastoma (NB) ranks as the second most common intraabdominal cancer and the fifth most prevalent malignancy in children (Walz et al., 2023). Extensive research efforts have enhanced the survival rate from less than 30% to high 85%–90%. Nevertheless, the relapse rate persists within the range of 15%–50% (Saltzman et al., 2023). Strikingly, LCA effectively induced NB cell death in vitro through apoptosis without neuron cytotoxicity. This elimination was achieved by triggering the intrinsic (initiator caspase-9 activation) and extrinsic apoptosis pathways (the initiator caspase-8 activation) (Goldberg et al., 2011; Trah et al., 2020).
3.3 Oral squamous cell carcinoma (OSCC)
Oral cancers represent prevalent malignant tumors within the head and neck and are primarily classified as squamous cell carcinomas that involve the transformation of mucous membranes in the gums, tongue, and face into cancerous tissues (Tan et al., 2023). UDCA has demonstrated potential in preventing gum and periodontal dysfunctions, as well as reducing gum bleeding (Pang et al., 2015). As the result, it is suggested that UDCA may hold promise in the treatment of oral cancers. Pang et al. (2015) demonstrated that UDCA triggered apoptosis in oral squamous cell cancer cells (HSC-3) via caspase activation. They also found that high UDCA levels exhibited cytotoxic effects in vitro (Pang et al., 2015).
Elevated levels of BAs have been recently known to be associated with impaired immune cell function, increased patient morbidity and even mortality. Consequently, high levels of BAs are considered immune suppressors, in which TCA is the most potent one of tumor immune inhibitors (Liu et al., 2018). Talebian et al. (2020) reported that TCA exhibited anti-inflammatory activities in human OSCC cells in vitro.
3.4 Oesophageal carcinoma
Esophageal carcinoma is prevalent in the developing countries and is characterized with significantly high morbidity and mortality, whereas its incidence is declining in the developed countries (Li et al., 2023). Abdel-Latif et al. (2016) revealed that pretreatment with UDCA effectively inhibited DCA-induced nuclear factor kappa B (NF-κB) and activator protein-1 (AP-1) DNA-binding activities in oesophageal carcinoma cells, thus decreasing cell survival.
3.5 Cholangiocarcinoma
Cholangiocarcinoma represents a malignant tumor associated with 20%–30% rate of 5-year survival even after resection. For those unable to undergo resection, the prognosis is especially poorer in which most patients fail to survive longer than 2 years (Greten et al., 2023). Although non-surgical palliative chemotherapy and radiation therapy are alternatively optional, their outcomes have not yielded satisfactory results. UDCA inhibited the growth of cholangiocarcinoma, and the combined UDCA and gefitinib displayed a more robust effect. Thus, UDCA demonstrates a potential adjuvant or palliative anticancer drug, providing a therapeutic option to enhance the effects of other chemotherapeutic agents synergistically (Lee et al., 2022). UDCA suppressed cholangiocarcinoma cell proliferation and invasiveness by triggering apoptosis, activating p53, and blocking DCA-induced activated EGFR-ERK and PI3K-AKT signaling (Lee et al., 2021). TUDCA impeded the proliferation of bile duct cancer cells by activating the mitogen-activated protein kinase (MAPK) p42/44 and PKCα signaling pathways (Alpini et al., 2004).
3.6 Gallbladder cancer (GBC)
Gallbladder cancer is a highly malignant disease that is often misdiagnosed at early stages. Thus, rapid development of GBC at later stages has largely limited the possibility of surgical intervention, leading to a poor prognosis (Li et al., 2014; Song et al., 2020a; Geng et al., 2022; Wang et al., 2023a; Wang et al., 2023b). DCA treatment has been found to halt GBC cell proliferation and reduce miR-92b-3p expression in an m6A-dependent post-transcriptional modification manner by facilitating METTL3 dissociation from METTL3-METTL14-WTAP complex and thus inactivating PI3K/AKT signaling pathway (Lin et al., 2020). LCA treatment has demonstrated tumor-suppressive function in GBC by decreasing glutaminase expression, interfering with glutamine metabolism and reducing GSH/GSSG and NADPH/NADP+ ratios. These effects lead to cellular ferroptosis and suppress tumor growth of GBC cell lines (Li et al., 2022).
3.7 Hepatocellular carcinoma (HCC)
Hepatocellular carcinoma (HCC) accounts for 85%–95% of primary liver cancer. Approximately 80% of HCC patients are diagnosed at advanced stages when surgical intervention is not applicable. The overall 5-year survival rate is less than 30% in advanced HCC patients as most of those patients with 80% experience cancer recurrence (Brown et al., 2023). Consequently, there is an urgent need to elucidate the mechanisms underlying HCC progression and develop effective therapy. CDCA robustly induced the expression of N-Myc downstream-regulated gene 2 (NDRG2) to hinder the proliferation of hepatoma cells (Langhi et al., 2013). Combining UDCA with anti-PD-1 enhanced anticancer immunity and promoted the development of tumor-specific immune memory. Additionally, UDCA phosphorylated transforming growth factor-beta (TGF-β) at the T282 site by activating the TGR5-cAMP-PKA axis, which increased the binding of TGF-β to the carboxyl terminus of the Hsc70-interacting protein. Combination therapy using anti-PD-1 or anti-PD-L1 antibody together with UDCA was more effective in treating tumor patients than singleanti-PD-1 or anti-PD-L1 antibody (Shen et al., 2022). Combining sorafenib and UDCA chemotherapy showed efficacy in advanced HCC by inhibiting cell proliferation and inducing apoptosis through ROS-dependent activation of ERK and Stat3 dephosphorylation (Lee et al., 2018). TUDCA attenuated apoptosis induced by ER stress (Vandewynckel et al., 2015). UDCA suppressed HCC growth in vivo in a dose- and time-dependent apoptosis fashion by upregulating the Bax to Bcl-2 ratio, Smac, Livin and caspase-3 expressions (Zhu et al., 2014; Liu et al., 2015), serving as a therapeutic candidate for HCC treatment. UDCA also exhibited selective ability to inhibit proliferation and induce apoptosis in HCC cell lines by disrupting the cell cycle and modulating the expression of Bax/Bcl-2 genes (Liu et al., 2007). Likewise, UDCA acted as an anti-proliferative agent in HCC by inducing DLC1 protein expression and inhibiting proteasomal DLC1 degradation (Chung et al., 2011). In HepG2 cells, UDCA transformed oxaliplatin-induced necrosis into apoptosis by inhibiting ROS generation and activating the p53-caspase 8 pathway. The combination of UDCA with chemotherapy effectively inhibited HCC by diminishing inflammatory responses (Lim et al., 2010).
3.8 Pancreatic cancer
Pancreatic cancer shows a notably low survival rate primarily owe to late diagnosis and resistance to therapies (Halbrook et al., 2023). The adverse effects of these chemotherapy treatments are also detrimental. Thus, optimal treatment remains to be developed. UDCA displayed the ability to prevent epithelial-mesenchymal transition (EMT) in pancreatic cancer cell lines, indicating its potential as an agent with antineoplastic properties (Kim Y. J. et al., 2017). UDCA suppressed intracellular ROS and Prx2 levels, EMT and stem cell formation in pancreatic cancer cells. These findings suggest that UDCA’s antioxidant effects may provide favorable therapeutic benefits for patients with pancreatic cancer (Kim Y. J. et al., 2017). A high BA level could inhibit cell proliferation and migration by inducing ROS and EMT pathways, thereby promoting apoptosis of pancreatic cancer cells (Zhu et al., 2022). BAs could reduce the proliferation of pancreatic cancer cells due to direct cytotoxicity (Wu et al., 2003). Specifically, DCA and CA induced cell cycle arrest, while GCA and TDCA elevated the S phase cell number, suggesting enhanced DNA synthesis and progression through the cell cycle (Wu et al., 2003).
3.9 Gastric cancer (GC)
Gastric cancer (GC) is one of the leading causes of cancer-related mortality worldwide. Most patients are diagnosed at advanced stages due to the neglect of minimal symptoms at earlier stages and the lack of regular early screening. Systemic therapies for GC including chemotherapy, targeted therapy, and immunotherapy, have been notably practiced in recent years (Guan et al., 2023). However, the favorable efficacy remains to be evaluated. TCDCA inhibited gastric cancer proliferation and invasion and induced apoptosis. Traditional Chinese medicine in experimental studies offered encouraging evidence for the potential application in the blockade of tumor (Zhang et al., 2022). DCA triggered apoptosis in gastric carcinoma cells by activating intrinsic mitochondrial-dependent, p53-mediatedcell death pathway (Yang et al., 2015). Furthermore, the upregulation of the Bax/Bcl-2 ratio and disruption of the mitochondrial membrane potential significantly contributed to the induction of DCA-mediated apoptosis in gastric carcinoma cells (Song et al., 2013). DCA also induced MUC2 expression in GC cells, inhibiting tumor progression. Accordingly, MUC2-expressing GC cells demonstrated decreased Snail expression (Pyo et al., 2015). UDCA drove apoptosis and autophagy, overcoming drug resistance (Lim and Han, 2015). Additionally, UDCA and DCA demonstrated suppressive effects in gastric cancer cells by activating the ERK signaling molecules (Lim et al., 2012). UDCA inhibited invasion by suppressing chenodeoxycholic acid induced PGE2 production (Wu et al., 2018). Furthermore, UDCA promoted GC apoptosis by activating the death receptor 5 (DR5) in lipid rafts (Lim et al., 2011).
3.10 Colon cancer
Colon cancer represents approximately 10% of all human cancers worldwide and, is also a leading cause of cancer-related deaths (Gallois et al., 2023). Except the essential early diagnosis and prevention required for clinic practice, effective therapies emerge as the most powerful aspect to improve patient survival. BAs play a causal role in colon cancer by inducing DNA damage (Kandell and Bernstein, 1991). TUDCA inhibited the NF-κB signaling pathway and alleviated colitis-associated tumorigenesis, indicating the valuable therapeutic means for colon cancer treatment (Kim et al., 2019). DCA increased intracellular ROS, genomic DNA breakage, and expression of ERK1/2, caspase 3, and PARP. In addition, DCA inhibited colonic cell proliferation through activation in the cell cycle and apoptosis pathways (Zeng et al., 2015). DCA exerted common and distinct effects on cell cycle, apoptosis, and MAP kinase pathway in human colon cancer cells (Zeng et al., 2009). DCA inhibited the proliferation by inducing apoptosis through AP-1 and C/EBP-mediated GADD153 expression (Qiao et al., 2002). Both DCA and CDCA suppressed cell proliferation by inducing apoptosis (Powell et al., 2001). UDCA suppressed cell proliferation by regulating oxidative stress in colon cancer cells (Kim E. K. et al., 2017). Treatment of colon carcinoma cells with UDCA inhibited cell proliferation by suppressing c-Myc expression and several cell cycle regulatory molecules (Peiró-Jordán et al., 2012). UDCA suppressed cell growth by inhibiting the mitogenic activity of receptor tyrosine kinases such as EGFR through increased receptor degradation (Feldman and Martinez, 2009). UDCA exerted a partial inhibitory effect on DCA-induced apoptosis via disrupting EGFR/Raf-1/ERK signaling pathway (Im and Martinez, 2004). UDCA prevented colon tumor and polyp formation by balancing the toxic effects of DCA and enhanced the potential cytoprotective effects of muricholic acids in the water-soluble fraction in rat feces (Batta et al., 1998). UDCA induced apoptosis by blocking the PI3K, MAPK, or cAMP pathways (Saeki et al., 2012). UDCA inhibited interleukin β1 and blocking NF-κB and AP-1 activation in colon cells (Shah et al., 2006). TUDCA augmented the cytotoxicity of hydrophobic BAs in vitro, and gaining a better understanding of how BAs interact in the colon can significantly impact the alteration of tumor promotion (Shekels et al., 1996). LCA was found to activate the vitamin D receptor (VDR), blocking inflammatory signals in colon cells (Sun et al., 2008). LCA also activated p53 that binds to MDM4 and MDM2, abrogating cell proliferation (Vogel et al., 2012).
3.11 Breast cancer
Breast cancer continues to be the first ranked cancer in women, which is characterized by significant disease heterogeneity, metastasis, and therapeutic resistance (Nolan et al., 2023). Growing evidence has found that LCA blocked breast cancer cell proliferation by stimulating oxidative stress that is under mined during breast cancer progresses (Kovács et al., 2019). LCA was able to regulate lipid metabolism reprogramming to inhibit breast cancer cells (Luu et al., 2018). Moreover, natural BAs negatively impacted on human breast cancer cell growth and steroid receptor function (Baker et al., 1992). Like LCA in breast cancer treatment, CDCA prompted cell death and resensitized tamoxifen resistant breast cancer (Giordano et al., 2011; Alasmael et al., 2016). Additionally, LCA exerted inhibitory effects on breast cancer proliferation, epithelial-mesenchymal transition (EMT), vascular endothelial growth factor (VEGF) production, and immune responses through the activation of the Takeda-G-protein-receptor-5 (TGR5) receptor (Mikó et al., 2018).
3.12 Prostate cancer
In man, prostate cancer is ranked as the most widespread cancer globally and is the second leading cause of cancer-related mortality in most developed countries. It is of note that a significant population of elderly patients are unable to withstand the conventional chemotherapy (Hamdy et al., 2023). In addition, increasing resistance to hormonal therapy has emerged as the substantial challenge in clinical treatment. Hence, alternative new drug development has been largely taken into account. LCA exhibited potent and non-selective effects on prostate cancer cells while sparing highly differentiated podocytes at lower concentrations, rendering it potential for an effective anticancer drug (Trah et al., 2020). LCA induced approximately 98% of cancer cell cytotoxicity at nominal concentrations in cultured medium (Goldberg et al., 2013). LCA induced autophagy and ER stress in PC-3 cells. However, this signature was found to be associated with initial protection and subsequent consequences rather than the ultimate cytotoxicity and mitochondrial dysfunction mediated by ROS (Gafar et al., 2016). LCA suppressed the proliferation of androgen-dependent (AD) LNCaP prostate cancer cells by inducing an apoptotic pathway (partially dependent on caspase-8 activation). Notably, LCA increased Bid and Bax cleavage, Bcl-2 downregulation, mitochondrial outer membrane permeabilization, and caspase-9 activation. UDCA drove apoptosis in prostate cancer cells by activating extrinsic and intrinsic apoptotic pathways (Lee et al., 2017). CDCA and DCA were shown to destabilize HIF-1α, significantly suppressing clonogenic growth, invasion, and migration (Liu et al., 2016). CDCA inhibited prostate cancer cells via activating the Farnesoid X receptor (FXR) and upregulating phosphatase and tensin homolog (PTEN) (Liu et al., 2014).
3.13 Ovarian cancer
Ovarian cancer is an aggressive disease that is often detected at advanced stages and typically exhibits a strong initial response to platinum-based chemotherapy. Despite this, the majority of patients experience relapse after the initial surgery and chemotherapy, implicating the critical necessity for the development of new therapeutic strategies (Konstantinopoulos and Matulonis, 2023). CDCA and DCA exhibited noteworthy cytotoxic activity in ovarian cancer cells by inducing apoptosis (Horowitz et al., 2007). CDCA and DCA could upregulate BRCA1 and downregulate ESR1 expression to inhibit BRCA1 mutated ovarian cancer progression (Jin et al., 2018).
3.14 Leukemia
Leukemia represents a highly fatal hematologic malignancy characterized by the accumulation of poorly differentiated myeloid cells in the bone marrow and blood, even in other tissues and organs. This widespread feature results ultimately in systemic dysfunction (DiNardo et al., 2023). To date, numerous research endeavors have been added to enhance treatment outcomes (Kayser and Levis, 2023), yet the rate of complete remission remains low. CDCA suppressed acute myeloid leukemia (AML) progression by promoting both lipid droplets (LD) accumulation and lipid peroxidation via ROS/p38 MAPK/DGAT1 pathway. CDCA also inhibited the polarization of M2 macrophages, contributing to its anti-leukemic properties (Liu et al., 2022). DCA, UDCA, TDCA, and TUDCA induced a delay in cell cycle progression in the human T leukemia cell line. Furthermore, DCA significantly increased the apoptotic cell fraction. DCA, CDCA and LCA inhibited the proliferation by accumulating the G0/G1 transition and inhibiting the differentiation (Zimber et al., 1994). Given the hydrophobic properties of DCA accounted for its cytotoxicity, it is possible to develop its derivatives as new anti-leukemia drugs for cancer therapy (Fimognari et al., 2009).
3.15 Melanoma
Melanoma has demonstrated the most lethal form of skin cancer and its incidence within the population has steadily risen in recent years. The high mortality rate of melanoma patients has continued to stimulate new research efforts to the regimens and drug development, expectedly improving the efficacy (Carvajal et al., 2023). UDCA could effectively inhibit melanoma cell proliferation in a time- and dose-dependent manner through cell cycle arrest in the G2/M phase, and cell apoptosis via the ROS-triggered mitochondrial-associated pathway (Yu et al., 2019).
4 Synthetic BA derivatives against cancer
Over past recent years, a large volume of researchers have paid the particular attention on modifying the structure of BAs and synthesizing derivatives in order to create novel agents to block cancers. This section mainly focuses on several synthetic derivatives of BAs that have been increasingly reported to inhibit cancer progression effectively (Table 2).
4.1 UDCA derivatives
The novel derivative HS-1030 derived from UDCA impeded hepatocellular carcinoma and breast cancer cell growth by inducing apoptosis (Park et al., 1997). Similarly, HS-1183, HS-1199 and HS-1200 generated from UDCA, inhibited proliferation of acute myeloid leukemia by inducing apoptotic cell death by downregulation of caspase-3/8 (Choi et al., 2001). Accordingly, these three derivatives inhibited human prostate carcinoma proliferation due to apoptosis induction via arresting cell cycle progression (Choi et al., 2003). In human cervical carcinoma cells, HS-1183, HS-1199, and HS-1200 suppressed cell growth and induced apoptosis by activating the JNK and NF-κB signaling pathways (Im et al., 2005). Moreover, all of HS-1030, HS-1183, HS-1199, and HS-1200 displayed the ability to inhibit colon cancer cell growth by arresting cell cycle progression at the G1 phase (Park et al., 2004). Finally, HS-1183, HS-1199 and HS-1200 derivatives not only inhibited breast carcinoma cell proliferation in a dose-dependent method, but also induced apoptotic nuclear changes and sub-G1 population and DNA fragmentation through ap53-independent pathway (Im et al., 2001).
In recent studies, CDCA and UDCA were conjugated with the anticancer drug paclitaxel (PTX) via a high-yield condensation reaction. The resulting product chenodeoxycholic-PTX hybrid (CDC-PTX) displayed comparable cytotoxicity and cytoselectivity to PTX. This activity was distinct from the ursodeoxycholic-PTX hybrid (UDC-PTX) that displayed limited anticancer effects on only colon cancer cells (Melloni et al., 2022).
4.2 CDCA derivatives
CDCA derivatives HS-1199 and HS-1200 induced caspase-dependent apoptosis in gastric cancer cell lines. This activity was also found dependent on elevated orphan receptor Nur77 (TR3) (Jeong et al., 2003). HS-1200 demonstrated an anticancer effect on human hepatoma cells as it reduced expression levels of cyclin A/D1 and Cdk2 and upregulated p21 WAF1/CIP1 and p27 KIP1 in a p53-dependent manner. HS-1200 also decreased cyclooxygenase (COX)-2 levels and induced early expression of Egr-1 (Park S. E. et al., 2008). In line with these findings, HS-1200 showed potential to induce apoptosis of hepatocellular carcinoma (HCC) (Liu et al., 2008). HS-1200 sensitized human breast carcinoma cells to radiation-induced apoptosis by increasing Bax expression and translocation into the mitochondria and thus increasing cytochrome c release (Yee et al., 2007). Both HS-1199 and HS-1200 exerted an anticancer effect on malignant GB cells through various apoptotic manifestations, including caspase-3 activation, DNA fragmentation factor (DFF) degradation, poly (ADP-ribose) polymerase cleavage, nuclear condensation, and proteasome activity inhibition (Yee et al., 2005). These two derivatives could induce apoptosis in GC cells through a caspase- and mitochondria-dependent manner (Moon et al., 2004). Treatment of thyroid carcinoma cells with HS-1200 increased cell death accompanied by procaspase-3/7 degradation, ADP-ribose polymerase degradation, histone hyperacetylation and peripheral chromatin condensation (Kim et al., 2009). Compound IIIb inhibited multiple myeloma cell proliferation in a way associated with Mcl-1 and PARP-1 cleavage, NF-κB signaling inhibition and/or DNA fragmentation (El Kihel et al., 2008).
4.3 DCA derivatives
DCA-chalcone amides were synthesized and tested for their antitumor effects on human lung and cervical cancer cells. The studies demonstrated that specific synthesized DCA-chalcone conjugates exhibited promising outcomes to inhibit cancer cells as potential anticancer agents (Patel et al., 2022). Recently, a series of new DCA derivatives were synthesized by incorporating aliphatic diamine and amino alcohol or morpholine moieties at the C3 position through 3, 26-epoxide ring-opening reactions. The mechanistic studies demonstrated that compound 9 induced cell death in colon cancer cells by activating apoptosis and autophagy. Vitamin D receptor was the primary target of this compound (Markov et al., 2019).
Considerable efforts were added to investigate the anticancer effects of amino-substituted α-cyanostilbene derivatives and CA and DCA amides on the human osteosarcoma (HOS) cancer cells. These studies revealed that all CA α-cyanostilbene amides exhibited anticancer effects on HOS cells with an effective range from 2 to 13 μM through induction of apoptotic cascade (Agarwal et al., 2018).
A pH-responsive micellar hydrogel system was developed using DCA-micelle (DCA-Mic) and carboxymethyl chitosan hydrogel (CMC Hyd) to improve the effectiveness of 5-FU against skin cancer and minimize side effects. This system facilitated the delivery of 5-FU into the skin and exhibited enhanced anticancer activity against melanoma cell growth compared to 5-FU alone. The 5-FU@Mic-Hyd platform showed a promising delivery system with improved efficacy for managing skin cancer in the absence of notable systemic toxicity (Pourmanouchehri et al., 2022).
A conjugate of heparin with DCA exhibited cytostatic and antiangiogenic properties, enhanced the anticancer effects of Doxorubicin (DOX) on squamous cell carcinoma and melanoma cells. Furthermore, the combination treatment using these two drugs resulted in improving therapeutic efficacy while minimizing cytotoxic effects (Park K. et al., 2008).
4.4 LCA derivatives
LCA and its derivatives ent-LCA induced apoptosis through CD95 activation, leading to increased ROS generation and subsequent cleavage of procaspase-8 (Katona et al., 2009). A group of BA derivatives, including CA, CDCA, UDCA, and LCA against colon cancer were designed and synthesized. All the compounds exhibited an anti-proliferative signature in various human malignant tumors. Four specific compounds from 4–7 significantly inhibited colon cancer colony formation, migration, and invasion. In addition to their antitumor effects, these compounds induced apoptosis by cell cycle arresting, resulting in a blockage of the mitotic process. Furthermore, they decreased the potential of the mitochondrial membrane but increased intracellular levels of ROS. These compounds downregulated the expression of Bcl-2 and p-STAT3, contributing to their apoptotic and anti-proliferative effects. Interestingly, these compounds also exhibited anti-inflammatory activity by inhibiting the production of nitric oxide (NO) and downregulating the expression of TNF-alpha, both of which are associated with inflammation in colon cancer (Wang et al., 2022).
Using LCA as a basis, ten cationic amphiphiles with variations in their head cationic charged groups were synthesized, and the anticancer effects of these amphiphiles were determined in colon cancer. LCA-based amphiphile containing piperidine head group (LCA-PIP) was approximately 10 times more cytotoxic than its precursor. The enhanced activity of LCA-PIP was attributed to a high level of cellular apoptosis. LCA-PIP induced sub-G0 arrest and caspase cleavage, promoting programmed cell death (Singh et al., 2015).
A heparin-lithocholic conjugate (HL) was created by covalently bonding lithocholate to heparin, and subsequent conjugation with folate to synthesize folate-HL conjugate (FHL). Although HL and FHL showed low anticoagulant activity, they sustained antiangiogenic properties. HL and FHL demonstrated similar antiangiogenic activity and inhibition of proliferation, while FHL exhibited stronger apoptotic effects than HL. These findings highlighted the potential of FHL as an effective anticancer agent with antiangiogenic and apoptotic properties (Yu et al., 2007).
LCA acetate induced leukemia cell differentiation. Combined treatment with LCA acetate and cotylenin A displayed more effectiveness in inducing monocytic differentiation than LCA acetate or cotylenin A alone. LCA acetate activated MAPK signaling that mediates cell differentiation. The synergistic effects of LCA acetate and cotylenin A on cell differentiation were partially ascribed to the MAPK activation induced by both agents (Horie et al., 2008).
4.5 CA derivatives
LLC-202, a prodrug for liver cancer, was developed by conjugating oxaliplatin with CA. The conjugation was achieved using 3-NH (2) (−) cyclobutane-1,1-dicarboxylate as a linker between the oxaliplatin analog and the CA moiety. The CA component was firmly bonded to the linker via an amide bond. Compared to oxaliplatin alone, LLC-202 exhibited enhanced absorption by human liver cancer cells while showing less affinity for normal liver cells. LLC-202 possessed higher anticancer activity and efficacy than oxaliplatin through the induction of apoptosis. These findings highlighted the promising potential of LLC-202 as a liver cancer-specific prodrug (Jiang et al., 2023).
A series of BAs (CA and DCA) aryl/heteroaryl amides linked with alpha-amino acid were synthesized and evaluated for the anticancer properties. More specifically, CA derivatives 6a, 6c, and 6m bearing phenyl, benzothiazole, and 4-methyl phenyl groups showed inhibitory activity against breast cancer cells compared with cisplatin and doxorubicin. Meanwhile, 6e, 6i, and 6m exhibited robust activity against the GB cancer cells relative to cisplatin and doxorubicin (Agarwal et al., 2016).
4.6 Other bile derivatives
Different BA derivatives were synthesized with modified side chains and the steroid skeleton, in which the former included reaction with 2-amino-2-methylpropanol and 4,4-dimethyl oxazoline group, and cyclization of amides. The latter involved addition of steroid skeleton oxo groups in positions 7 (2, 2a, 2b) and 7,12 (3, 3a, 3b). By Wittig reaction, the ethylidene groups were introduced regio- and stereo-selectively on C-7 and without stereoselectivity on C-3. Compounds containing both C-7 ethylidene and C-12 carbonyl groups (6, 6a, and 6b) showed significant anticancer activity. Altering the carboxylic group to the amide or oxazoline group enhanced cytotoxicity (Bjedov et al., 2017).
A series of new seco-A ring BA diamides were synthesized and evaluated for their anti-proliferative activities. These compounds enhanced G1 arrest and increased anti-migration activity, demonstrating improved anti-proliferative activities relative to the parent bile acid. A compound 27 conjugated with piperazine showed promising results with strong cytotoxicity in cancer cells (Mao et al., 2016). Moreover, all tested compounds exhibited lower cytotoxic activity on noncancerous cells.
Fifteen new piperazinyl bile carboxamides derived from various BAs, including CA, UDCA, CDCA, DCA, and LCA, were synthesized and evaluated for their pro-apoptotic potency in colon cancer cells. Most of the synthetic bile carboxamide derivatives were found to significantly decrease cell viability, in which compound 9c and 9d exhibited the most significant dose-response effect and solubility on colon cancer cells. The presence of a benzyl group in the structure of the derivatives was associated with enhanced anti-proliferative activity. Furthermore, introducing an α-hydroxyl group at the 7-position of the steroid skeleton was particularly beneficial (Brossard et al., 2014).
Two BA tamoxifen conjugates were synthesized using LCA, DCA, and CA, whereby1, 2, or 3 tamoxifen molecules were attached to the hydroxyl groups of BAs with free acid and amine functionalities in their tail regions. In these conjugates, the cholic acid-tamoxifen conjugate with a free amine headgroup (CA-Tam3-Am) demonstrated the strongest potency as an anticancer agent to induce apoptosis, cell cycle arrest, and high ROS generation. These findings highlighted that BAs could be utilized as a new framework to achieve high effective drug potency. The antitumor properties of these conjugates were significantly influenced by the charge and hydrophobicity of the lipid-drug conjugate (Sreekanth et al., 2013).
Four cationic bile acid-based facial amphiphiles were synthesized and evaluated for their cytotoxic activities against colon cancer cells. The critical factors examined were charge, hydration, and hydrophobicity. Among the synthesized amphiphiles, the singly charged amphiphile based on lithocholic acid (LCA-TMA1) exhibited the highest cytotoxicity. In contrast, the triply charged cationic amphiphile based on cholic acid (CA-TMA3) showed negligible cytotoxicity. These cytotoxic effects were observed at late apoptosis. The LCA-TMA1 amphiphile demonstrated high hydrophobicity combined with a burdensome charge, leading to efficient dehydration and significant membrane perturbations. These characteristics facilitated its translocation and resulted in high cytotoxicity. On the other hand, the highly hydrated and multiple-charged amphiphile CA-TMA3 showed the least membrane penetration, limiting its translocation and subsequent cytotoxicity. Amphiphiles based on deoxycholic acid (DCA-TMA2) and chenodeoxycholic acid (CDCA-TMA2), featuring two charged head groups, displayed intermediate behavior. In conclusion, the charge, hydration, and hydrophobicity of these cationic BA-based facial amphiphiles determined their interaction with cells and membrane translocation (Singh et al., 2013).
Brossard et al. (2010) utilized nitrogenous heterocycles as a fundamental component in synthesizing conjugate BA derivatives. They successfully synthesized new piperazinyl BA derivatives and examined in vitro activity in different human cancer cells. Among the synthesized derivatives, N-[4N-cinnamylpiperazin-1-yl]-3alpha,7beta-dihydroxy-5beta-cholan-24-amide (compound 7b) and N-[4N-cinnamyllpiperazin-1-yl]-3alpha,7alpha-dihydroxy-5beta-cholan-24-amide (compound 7c) demonstrated the most significant pro-apoptotic activity in these human cancer cells. These compounds induced nuclear and DNA fragmentation, indicating that 7b and 7c induce cell death through an apoptotic process. The findings suggest hybrid heterocycle-steroid compounds could serve as a new class of anticancer drugs with improved bioactivity. Additionally, the simple synthesis of these compounds highlighted their potential for future development as anticancer therapeutics (Brossard et al., 2010).
Králová et al. (2008) synthesized and utilized conjugates of porphyrin and BAs as ligands to specifically bind to saccharide cancer markers expressed by tumor cells. They found that these compounds possessed a high selectivity for saccharide cancer markers and cancer cells, indicating significant potential for targeted photodynamic therapy (Králová et al., 2008). LCA acetate inhibited hepatoblastoma, colon cancer and leukemia cell proliferation by binding to VDR (Adachi et al., 2005). Moreover, bile-acid-appended triazolyl aryl ketones (6af and 6cf) inhibited breast cancer cell viability (Agarwal et al., 2021).
5 Conclusion
This article comprehensively reviews the anticancer activities observed after treatment with both natural BAs and synthetic BA derivatives. These therapeutic approaches are attributed to the amphiphilic nature of BAs and their ability to activate additional targeted pathways that are not stimulated at physiological low concentrations. Additionally, the interaction between BAs and the gut microbiome, known as the BA/gut microbiome axis, may influence the association between BAs and cancer, facilitating BAs action (Song et al., 2020b).
Synthesized BA derivatives have strong ability to induce cell death in various human cancer cell lines. Consequently, these novel BA derivatives show promising results as potent agents to target different types of cancer cells by inducing apoptosis. These findings suggest that these derivatives are the potential candidates for developing novel alternative anticancer agents. Nonetheless, to better understand these agents, mechanistic insights of their activities remain to be substantially investigated. While there is currently no precise report on the cost-effectiveness of preparing BAs and their derivatives, we believe that through further research, the price of isolation, purification or synthesis expanse of BAs and derivatives can be reduced, potentially making it more affordable for a greater number of cancer patients.
Author contributions
WL: Writing–original draft. LZ: Writing–original draft. SH: Writing–review and editing. HM: Writing–review and editing. KL: Writing–review and editing. YG: Writing–review and editing, Conceptualization. YL: Writing–review and editing, Conceptualization, Writing–original draft. WW: Writing–review and editing, Conceptualization, Writing–original draft.
Funding
The author(s) declare financial support was received for the research, authorship, and/or publication of this article. This work was supported by the National Natural Science Foundation of China (Nos 82272620, 8227111000, and 82273016). Shanghai Science and Technology Innovation Action Plan (No. 22Y11908100). Open Project of State Key Laboratory of Oncogenes and Related Genes (No. KF2120), YL Expert Workstation Project of Yunnan Province (No. 202205AF150083), and the Innovative Research Team of High-level Local Universities in Shanghai.
Acknowledgments
The authors want to sincerely thank Professor Rong Shao for editing the manuscript and Figdraw for creating the figures.
Conflict of interest
The authors declare that the research was conducted in the absence of any commercial or financial relationships that could be construed as a potential conflict of interest.
Publisher’s note
All claims expressed in this article are solely those of the authors and do not necessarily represent those of their affiliated organizations, or those of the publisher, the editors and the reviewers. Any product that may be evaluated in this article, or claim that may be made by its manufacturer, is not guaranteed or endorsed by the publisher.
References
Abdel-Latif, M. M., Inoue, H., and Reynolds, J. V. (2016). Opposing effects of bile acids deoxycholic acid and ursodeoxycholic acid on signal transduction pathways in oesophageal cancer cells. Eur. J. Cancer Prev. 25, 368–379. doi:10.1097/CEJ.0000000000000198
Adachi, R., Honma, Y., Masuno, H., Kawana, K., Shimomura, I., Yamada, S., et al. (2005). Selective activation of vitamin D receptor by lithocholic acid acetate, a bile acid derivative. J. Lipid Res. 46, 46–57. doi:10.1194/jlr.M400294-JLR200
Agarwal, D. S., Anantaraju, H. S., Sriram, D., Yogeeswari, P., Nanjegowda, S. H., Mallu, P., et al. (2016). Synthesis, characterization and biological evaluation of bile acid-aromatic/heteroaromatic amides linked via amino acids as anti-cancer agents. Steroids 107, 87–97. doi:10.1016/j.steroids.2015.12.022
Agarwal, D. S., Mazumdar, S., Italiya, K. S., Chitkara, D., and Sakhuja, R. (2021). Bile-acid-appended triazolyl aryl ketones: design, synthesis, in vitro anticancer activity and pharmacokinetics in rats. Molecules 26, 5741. doi:10.3390/molecules26195741
Agarwal, D. S., Singh, R. P., Lohitesh, K., Jha, P. N., Chowdhury, R., and Sakhuja, R. (2018). Synthesis and evaluation of bile acid amides of [Formula: see text]-cyanostilbenes as anticancer agents. Mol. Divers 22, 305–321. doi:10.1007/s11030-017-9797-9
Alasmael, N., Mohan, R., Meira, L. B., Swales, K. E., and Plant, N. J. (2016). Activation of the Farnesoid X-receptor in breast cancer cell lines results in cytotoxicity but not increased migration potential. Cancer Lett. 370, 250–259. doi:10.1016/j.canlet.2015.10.031
Alpini, G., Kanno, N., Phinizy, J. L., Glaser, S., Francis, H., Taffetani, S., et al. (2004). Tauroursodeoxycholate inhibits human cholangiocarcinoma growth via Ca2+-PKC-and MAPK-dependent pathways. Am. J. Physiol. Gastrointest. Liver Physiol. 286, G973–G982. doi:10.1152/ajpgi.00270.2003
Baker, P. R., Wilton, J. C., Jones, C. E., Stenzel, D. J., Watson, N., and Smith, G. J. (1992). Bile acids influence the growth, oestrogen receptor and oestrogen-regulated proteins of MCF-7 human breast cancer cells. Br. J. Cancer 65, 566–572. doi:10.1038/bjc.1992.115
Batta, A. K., Salen, G., Holubec, H., Brasitus, T. A., Alberts, D., and Earnest, D. L. (1998). Enrichment of the more hydrophilic bile acid ursodeoxycholic acid in the fecal water-soluble fraction after feeding to rats with colon polyps. Cancer Res. 58, 1684–1687.
Bjedov, S., Jakimov, D., Pilipović, A., Poša, M., and Sakač, M. (2017). Antitumor activity of newly synthesized oxo and ethylidene derivatives of bile acids and their amides and oxazolines. Steroids 120, 19–25. doi:10.1016/j.steroids.2017.01.008
Brossard, D., El Kihel, L., Clement, M., Sebbahi, W., Khalid, M., Roussakis, C., et al. (2010). Synthesis of bile acid derivatives and in vitro cytotoxic activity with pro-apoptotic process on multiple myeloma (KMS-11), glioblastoma multiforme (GBM), and colonic carcinoma (HCT-116) human cell lines. Eur. J. Med. Chem. 45, 2912–2918. doi:10.1016/j.ejmech.2010.03.016
Brossard, D., Lechevrel, M., El Kihel, L., Quesnelle, C., Khalid, M., Moslemi, S., et al. (2014). Synthesis and biological evaluation of bile carboxamide derivatives with pro-apoptotic effect on human colon adenocarcinoma cell lines. Eur. J. Med. Chem. 86, 279–290. doi:10.1016/j.ejmech.2014.07.080
Brown, Z. J., Tsilimigras, D. I., Ruff, S. M., Mohseni, A., Kamel, I. R., Cloyd, J. M., et al. (2023). Management of hepatocellular carcinoma: a review. JAMA Surg. 158, 410–420. doi:10.1001/jamasurg.2022.7989
Carvajal, R. D., Sacco, J. J., Jager, M. J., Eschelman, D. J., Olofsson Bagge, R., Harbour, J. W., et al. (2023). Advances in the clinical management of uveal melanoma. Nat. Rev. Clin. Oncol. 20, 99–115. doi:10.1038/s41571-022-00714-1
Chiang, J. Y. (2009). Bile acids: regulation of synthesis. J. Lipid Res. 50, 1955–1966. doi:10.1194/jlr.R900010-JLR200
Chiang, J. Y. L., and Ferrell, J. M. (2019). Bile acids as metabolic regulators and nutrient sensors. Annu. Rev. Nutr. 39, 175–200. doi:10.1146/annurev-nutr-082018-124344
Chien, J. M., Chou, C. T., Liang, W. Z., Cheng, J. S., Chang, H. T., Tseng, H. W., et al. (2015). Effect of deoxycholic acid on Ca2+ movement, cell viability and apoptosis in human gastric cancer cells. Toxicol. Mech. Methods 25, 113–119. doi:10.3109/15376516.2014.990597
Choi, Y. H., Im, E. O., Suh, H., Jin, Y., Lee, W. H., Yoo, Y. H., et al. (2001). Apoptotic activity of novel bile acid derivatives in human leukemic T cells through the activation of caspases. Int. J. Oncol. 18, 979–984. doi:10.3892/ijo.18.5.979
Choi, Y. H., Im, E. O., Suh, H., Jin, Y., Yoo, Y. H., and Kim, N. D. (2003). Apoptosis and modulation of cell cycle control by synthetic derivatives of ursodeoxycholic acid and chenodeoxycholic acid in human prostate cancer cells. Cancer Lett. 199, 157–167. doi:10.1016/s0304-3835(03)00351-3
Chung, G. E., Yoon, J. H., Lee, J. H., Kim, H. Y., Myung, S. J., Yu, S. J., et al. (2011). Ursodeoxycholic acid-induced inhibition of DLC1 protein degradation leads to suppression of hepatocellular carcinoma cell growth. Oncol. Rep. 25, 1739–1746. doi:10.3892/or.2011.1239
Collins, S. L., Stine, J. G., Bisanz, J. E., Okafor, C. D., and Patterson, A. D. (2023). Bile acids and the gut microbiota: metabolic interactions and impacts on disease. Nat. Rev. Microbiol. 21, 236–247. doi:10.1038/s41579-022-00805-x
Di Ciaula, A., Garruti, G., Lunardi Baccetto, R., Molina-Molina, E., Bonfrate, L., Wang, D. Q. H., et al. (2017). Bile acid physiology. Ann. Hepatology 16, S4–S14. doi:10.5604/01.3001.0010.5493
DiNardo, C. D., Erba, H. P., Freeman, S. D., and Wei, A. H. (2023). Acute myeloid leukaemia. Lancet 401, 2073–2086. doi:10.1016/s0140-6736(23)00108-3
El Kihel, L., Clement, M., Bazin, M. A., Descamps, G., Khalid, M., and Rault, S. (2008). New lithocholic and chenodeoxycholic piperazinylcarboxamides with antiproliferative and pro-apoptotic effects on human cancer cell lines. Bioorg Med. Chem. 16, 8737–8744. doi:10.1016/j.bmc.2008.07.046
Fan, Y., Kim, H. J., Jung, Y. S., Na, S. Y., Radhakrishnan, K., and Choi, H. S. (2023). Chenodeoxycholic acid regulates fibroblast growth factor 23 gene expression via estrogen-related receptor γ in human hepatoma Huh7 cells. Steroids 197, 109257. doi:10.1016/j.steroids.2023.109257
Feldman, R., and Martinez, J. D. (2009). Growth suppression by ursodeoxycholic acid involves caveolin-1 enhanced degradation of EGFR. Biochim. Biophys. Acta 1793, 1387–1394. doi:10.1016/j.bbamcr.2009.05.003
Fimognari, C., Lenzi, M., Cantelli-Forti, G., and Hrelia, P. (2009). Apoptosis and modulation of cell cycle control by bile acids in human leukemia T cells. Ann. N. Y. Acad. Sci. 1171, 264–269. doi:10.1111/j.1749-6632.2009.04710.x
Fiorucci, S., Carino, A., Baldoni, M., Santucci, L., Costanzi, E., Graziosi, L., et al. (2021). Bile acid signaling in inflammatory bowel diseases. Dig. Dis. Sci. 66, 674–693. doi:10.1007/s10620-020-06715-3
Fu, J., Yu, M., Xu, W., and Yu, S. (2021). Research progress of bile acids in cancer. Front. Oncol. 11, 778258. doi:10.3389/fonc.2021.778258
Gafar, A. A., Draz, H. M., Goldberg, A. A., Bashandy, M. A., Bakry, S., Khalifa, M. A., et al. (2016). Lithocholic acid induces endoplasmic reticulum stress, autophagy and mitochondrial dysfunction in human prostate cancer cells. PeerJ 4, e2445. doi:10.7717/peerj.2445
Gallois, C., Shi, Q., Meyers, J. P., Iveson, T., Alberts, S. R., de Gramont, A., et al. (2023). Prognostic impact of early treatment and oxaliplatin discontinuation in patients with stage III colon cancer: an ACCENT/IDEA pooled analysis of 11 adjuvant trials. J. Clin. Oncol. 41, 803–815. doi:10.1200/JCO.21.02726
Geng, Y., Chen, S., Yang, Y., Miao, H., Li, X., Li, G., et al. (2022). Long-term exposure to genistein inhibits the proliferation of gallbladder cancer by downregulating the MCM complex. Sci. Bull. (Beijing) 67, 813–824. doi:10.1016/j.scib.2022.01.011
Giordano, C., Catalano, S., Panza, S., Vizza, D., Barone, I., Bonofiglio, D., et al. (2011). Farnesoid X receptor inhibits tamoxifen-resistant MCF-7 breast cancer cell growth through downregulation of HER2 expression. Oncogene 30, 4129–4140. doi:10.1038/onc.2011.124
Goldberg, A. A., Beach, A., Davies, G. F., Harkness, T. A., Leblanc, A., and Titorenko, V. I. (2011). Lithocholic bile acid selectively kills neuroblastoma cells, while sparing normal neuronal cells. Oncotarget 2, 761–782. doi:10.18632/oncotarget.338
Goldberg, A. A., Titorenko, V. I., Beach, A., and Sanderson, J. T. (2013). Bile acids induce apoptosis selectively in androgen-dependent and -independent prostate cancer cells. PeerJ 1, e122. doi:10.7717/peerj.122
Greten, T. F., Schwabe, R., Bardeesy, N., Ma, L., Goyal, L., Kelley, R. K., et al. (2023). Immunology and immunotherapy of cholangiocarcinoma. Nat. Rev. Gastroenterol. Hepatol. 20, 349–365. doi:10.1038/s41575-022-00741-4
Guan, W. L., He, Y., and Xu, R. H. (2023). Gastric cancer treatment: recent progress and future perspectives. J. Hematol. Oncol. 16, 57. doi:10.1186/s13045-023-01451-3
Halbrook, C. J., Lyssiotis, C. A., Pasca di Magliano, M., and Maitra, A. (2023). Pancreatic cancer: advances and challenges. Cell 186, 1729–1754. doi:10.1016/j.cell.2023.02.014
Hamdy, F. C., Donovan, J. L., Lane, J. A., Metcalfe, C., Davis, M., Turner, E. L., et al. (2023). Fifteen-year outcomes after monitoring, surgery, or radiotherapy for prostate cancer. N. Engl. J. Med. 388, 1547–1558. doi:10.1056/NEJMoa2214122
Horie, A., Akimoto, M., Tsumura, H., Makishima, M., Taketani, T., Yamaguchi, S., et al. (2008). Induction of differentiation of myeloid leukemia cells in primary culture in response to lithocholic acid acetate, a bile acid derivative, and cooperative effects with another differentiation inducer, cotylenin A. Leuk. Res. 32, 1112–1123. doi:10.1016/j.leukres.2007.12.004
Horowitz, N. S., Hua, J., Powell, M. A., Gibb, R. K., Mutch, D. G., and Herzog, T. J. (2007). Novel cytotoxic agents from an unexpected source: bile acids and ovarian tumor apoptosis. Gynecol. Oncol. 107, 344–349. doi:10.1016/j.ygyno.2007.07.072
Im, E., Choi, S. H., Suh, H., Choi, Y. H., Yoo, Y. H., and Kim, N. D. (2005). Synthetic bile acid derivatives induce apoptosis through a c-Jun N-terminal kinase and NF-kappaB-dependent process in human cervical carcinoma cells. Cancer Lett. 229, 49–57. doi:10.1016/j.canlet.2004.11.055
Im, E., and Martinez, J. D. (2004). Ursodeoxycholic acid (UDCA) can inhibit deoxycholic acid (DCA)-induced apoptosis via modulation of EGFR/Raf-1/ERK signaling in human colon cancer cells. J. Nutr. 134, 483–486. doi:10.1093/jn/134.2.483
Im, E. O., Choi, Y. H., Paik, K. J., Suh, H., Jin, Y., Kim, K. W., et al. (2001). Novel bile acid derivatives induce apoptosis via a p53-independent pathway in human breast carcinoma cells. Cancer Lett. 163, 83–93. doi:10.1016/s0304-3835(00)00671-6
Jeong, J. H., Park, J. S., Moon, B., Kim, M. C., Kim, J. K., Lee, S., et al. (2003). Orphan nuclear receptor Nur77 translocates to mitochondria in the early phase of apoptosis induced by synthetic chenodeoxycholic acid derivatives in human stomach cancer cell line SNU-1. Ann. N. Y. Acad. Sci. 1010, 171–177. doi:10.1196/annals.1299.029
Jiang, J., Han, F., Cai, K., Shen, Q., Yang, C., Gao, A., et al. (2023). Synthesis and biological evaluation of cholic acid-conjugated oxaliplatin as a new prodrug for liver cancer. J. Inorg. Biochem. 243, 112200. doi:10.1016/j.jinorgbio.2023.112200
Jin, Q., Noel, O., Nguyen, M., Sam, L., and Gerhard, G. S. (2018). Bile acids upregulate BRCA1 and downregulate estrogen receptor 1 gene expression in ovarian cancer cells. Eur. J. Cancer Prev. 27, 553–556. doi:10.1097/CEJ.0000000000000398
Kandell, R. L., and Bernstein, C. (1991). Bile salt/acid induction of DNA damage in bacterial and mammalian cells: implications for colon cancer. Nutr. Cancer 16, 227–238. doi:10.1080/01635589109514161
Katona, B. W., Anant, S., Covey, D. F., and Stenson, W. F. (2009). Characterization of enantiomeric bile acid-induced apoptosis in colon cancer cell lines. J. Biol. Chem. 284, 3354–3364. doi:10.1074/jbc.M805804200
Kayser, S., and Levis, M. J. (2023). The clinical impact of the molecular landscape of acute myeloid leukemia. Haematologica 108, 308–320. doi:10.3324/haematol.2022.280801
Kim, T. H., Yoo, Y. H., Kang, D. Y., Suh, H., Park, M. K., Park, K. J., et al. (2009). Efficacy on anaplastic thyroid carcinoma of valproic acid alone or in combination with doxorubicin, a synthetic chenodeoxycholic acid derivative, or lactacystin. Int. J. Oncol. 34, 1353–1362. doi:10.3892/ijo_00000262
Kim, Y. H., Kim, J. H., Kim, B. G., Lee, K. L., Kim, J. W., and Koh, S. J. (2019). Tauroursodeoxycholic acid attenuates colitis-associated colon cancer by inhibiting nuclear factor kappaB signaling. J. Gastroenterol. Hepatol. 34, 544–551. doi:10.1111/jgh.14526
Kim, E. K., Cho, J. H., Kim, E., and Kim, Y. J. (2017). Ursodeoxycholic acid inhibits the proliferation of colon cancer cells by regulating oxidative stress and cancer stem-like cell growth. PLoS One 12, e0181183. doi:10.1371/journal.pone.0181183
Kim, Y. J., Jeong, S. H., Kim, E. K., Kim, E. J., and Cho, J. H. (2017). Ursodeoxycholic acid suppresses epithelial-mesenchymal transition and cancer stem cell formation by reducing the levels of peroxiredoxin II and reactive oxygen species in pancreatic cancer cells. Oncol. Rep. 38, 3632–3638. doi:10.3892/or.2017.6045
Konstantinopoulos, P. A., and Matulonis, U. A. (2023). Clinical and translational advances in ovarian cancer therapy. Nat. Cancer 4, 1239–1257. doi:10.1038/s43018-023-00617-9
Kovács, P., Csonka, T., Kovács, T., Sári, Z., Ujlaki, G., Sipos, A., et al. (2019). Lithocholic acid, a metabolite of the microbiome, increases oxidative stress in breast cancer. Cancers (Basel) 11, 1255. doi:10.3390/cancers11091255
Králová, J., Koivukorpi, J., Kejík, Z., Poucková, P., Sievänen, E., Kolehmainen, E., et al. (2008). Porphyrin-bile acid conjugates: from saccharide recognition in the solution to the selective cancer cell fluorescence detection. Org. Biomol. Chem. 6, 1548–1552. doi:10.1039/b717528k
Langhi, C., Pedraz-Cuesta, E., Donate, Y., Marrero, P. F., Haro, D., and Rodríguez, J. C. (2013). Regulation of N-Myc downstream regulated gene 2 by bile acids. Biochem. Biophys. Res. Commun. 434, 102–109. doi:10.1016/j.bbrc.2013.03.058
Lee, J., Hong, E. M., Kim, J. H., Kim, J. H., Jung, J. H., Park, S. W., et al. (2022). Ursodeoxycholic acid inhibits epithelial-mesenchymal transition, suppressing invasiveness of bile duct cancer cells: an in vitro study. Oncol. Lett. 24, 448. doi:10.3892/ol.2022.13568
Lee, J., Hong, E. M., Kim, J. H., Kim, J. H., Jung, J. H., Park, S. W., et al. (2021). Ursodeoxycholic acid shows antineoplastic effects in bile duct cancer cells via apoptosis induction; p53 activation; and EGFR-ERK, COX-2, and PI3K-AKT pathway inhibition. Mol. Biol. Rep. 48, 6231–6240. doi:10.1007/s11033-021-06331-y
Lee, S., Cho, Y. Y., Cho, E. J., Yu, S. J., Lee, J. H., Yoon, J. H., et al. (2018). Synergistic effect of ursodeoxycholic acid on the antitumor activity of sorafenib in hepatocellular carcinoma cells via modulation of STAT3 and ERK. Int. J. Mol. Med. 42, 2551–2559. doi:10.3892/ijmm.2018.3807
Lee, W. S., Jung, J. H., Panchanathan, R., Yun, J. W., Kim, D. H., Kim, H. J., et al. (2017). Ursodeoxycholic acid induces death receptor-mediated apoptosis in prostate cancer cells. J. Cancer Prev. 22, 16–21. doi:10.15430/JCP.2017.22.1.16
Li, M., Zhang, Z., Li, X., Ye, J., Wu, X., Tan, Z., et al. (2014). Whole-exome and targeted gene sequencing of gallbladder carcinoma identifies recurrent mutations in the ErbB pathway. Nat. Genet. 46, 872–876. doi:10.1038/ng.3030
Li, Q., Luo, H., Dai, F. Q., Wang, R. T., Fan, X. Q., Luo, Y. Y., et al. (2023). SAMD9 promotes postoperative recurrence of esophageal squamous cell carcinoma by stimulating MYH9-mediated gsk3β/β-catenin signaling. Adv. Sci. (Weinh) 10, e2203573. doi:10.1002/advs.202203573
Li, T., and Chiang, J. Y. (2014). Bile acid signaling in metabolic disease and drug therapy. Pharmacol. Rev. 66, 948–983. doi:10.1124/pr.113.008201
Li, W., Wang, Z., Lin, R., Huang, S., Miao, H., Zou, L., et al. (2022). Lithocholic acid inhibits gallbladder cancer proliferation through interfering glutaminase-mediated glutamine metabolism. Biochem. Pharmacol. 205, 115253. doi:10.1016/j.bcp.2022.115253
Lim, S. C., Choi, J. E., Kang, H. S., and Han, S. I. (2010). Ursodeoxycholic acid switches oxaliplatin-induced necrosis to apoptosis by inhibiting reactive oxygen species production and activating p53-caspase 8 pathway in HepG2 hepatocellular carcinoma. Int. J. Cancer 126, 1582–1595. doi:10.1002/ijc.24853
Lim, S. C., Duong, H. Q., Choi, J. E., Lee, T. B., Kang, J. H., Oh, S. H., et al. (2011). Lipid raft-dependent death receptor 5 (DR5) expression and activation are critical for ursodeoxycholic acid-induced apoptosis in gastric cancer cells. Carcinogenesis 32, 723–731. doi:10.1093/carcin/bgr038
Lim, S. C., Duong, H. Q., Parajuli, K. R., and Han, S. I. (2012). Pro-apoptotic role of the MEK/ERK pathway in ursodeoxycholic acid-induced apoptosis in SNU601 gastric cancer cells. Oncol. Rep. 28, 1429–1434. doi:10.3892/or.2012.1918
Lim, S. C., and Han, S. I. (2015). Ursodeoxycholic acid effectively kills drug-resistant gastric cancer cells through induction of autophagic death. Oncol. Rep. 34, 1261–1268. doi:10.3892/or.2015.4076
Lin, R., Zhan, M., Yang, L., Wang, H., Shen, H., Huang, S., et al. (2020). Deoxycholic acid modulates the progression of gallbladder cancer through N(6)-methyladenosine-dependent microRNA maturation. Oncogene 39, 4983–5000. doi:10.1038/s41388-020-1349-6
Liu, H., Qin, C. K., Han, G. Q., Xu, H. W., Ren, W. H., and Qin, C. Y. (2008). Synthetic chenodeoxycholic acid derivative, HS-1200, induces apoptosis of human hepatoma cells via a mitochondrial pathway. Cancer Lett. 270, 242–249. doi:10.1016/j.canlet.2008.05.014
Liu, H., Qin, C. Y., Han, G. Q., Xu, H. W., Meng, M., and Yang, Z. (2007). Mechanism of apoptotic effects induced selectively by ursodeoxycholic acid on human hepatoma cell lines. World J. Gastroenterol. 13, 1652–1658. doi:10.3748/wjg.v13.i11.1652
Liu, H., Xu, H. W., Zhang, Y. Z., Huang, Y., Han, G. Q., Liang, T. J., et al. (2015). Ursodeoxycholic acid induces apoptosis in hepatocellular carcinoma xenografts in mice. World J. Gastroenterol. 21, 10367–10374. doi:10.3748/wjg.v21.i36.10367
Liu, J., Tong, S. J., Wang, X., and Qu, L. X. (2014). Farnesoid X receptor inhibits LNcaP cell proliferation via the upregulation of PTEN. Exp. Ther. Med. 8, 1209–1212. doi:10.3892/etm.2014.1894
Liu, J., Wei, Y., Jia, W., Can, C., Wang, R., Yang, X., et al. (2022). Chenodeoxycholic acid suppresses AML progression through promoting lipid peroxidation via ROS/p38 MAPK/DGAT1 pathway and inhibiting M2 macrophage polarization. Redox Biol. 56, 102452. doi:10.1016/j.redox.2022.102452
Liu, N., Zhao, J., Wang, J., Teng, H., Fu, Y., and Yuan, H. (2016). Farnesoid X receptor ligand CDCA suppresses human prostate cancer cells growth by inhibiting lipid metabolism via targeting sterol response element binding protein 1. Am. J. Transl. Res. 8, 5118–5124.
Liu, Z., Zhang, Z., Huang, M., Sun, X., Liu, B., Guo, Q., et al. (2018). Taurocholic acid is an active promoting factor, not just a biomarker of progression of liver cirrhosis: evidence from a human metabolomic study and in vitro experiments. BMC Gastroenterol. 18, 112. doi:10.1186/s12876-018-0842-7
Luu, T. H., Bard, J. M., Carbonnelle, D., Chaillou, C., Huvelin, J. M., Bobin-Dubigeon, C., et al. (2018). Lithocholic bile acid inhibits lipogenesis and induces apoptosis in breast cancer cells. Cell Oncol. (Dordr) 41, 13–24. doi:10.1007/s13402-017-0353-5
Mao, S. W., Chen, H., Yu, L. F., Lv, F., Xing, Y. J., Liu, T., et al. (2016). Novel 3,4-seco bile acid diamides as selective anticancer proliferation and migration agents. Eur. J. Med. Chem. 122, 574–583. doi:10.1016/j.ejmech.2016.04.055
Markov, A. V., Babich, V. O., Popadyuk, , Salomatina, O. V., Logashenko, E. B., Salakhutdinov, N. F., et al. (2019). Novel derivatives of deoxycholic acid bearing linear aliphatic diamine and aminoalcohol moieties and their cyclic analogs at the C3 position: synthesis and evaluation of their in vitro antitumor potential. Molecules 24, 2644. doi:10.3390/molecules24142644
Melloni, E., Marchesi, E., Preti, L., Casciano, F., Rimondi, E., Romani, A., et al. (2022). Synthesis and biological investigation of bile acid-paclitaxel hybrids. Molecules 27, 471. doi:10.3390/molecules27020471
Mikó, E., Vida, A., Kovács, T., Ujlaki, G., Trencsényi, G., Márton, J., et al. (2018). Lithocholic acid, a bacterial metabolite reduces breast cancer cell proliferation and aggressiveness. Biochim. Biophys. Acta Bioenerg. 1859, 958–974. doi:10.1016/j.bbabio.2018.04.002
Moon, B., Kim, M.-C., and Park, J.-s. (2004). Synthetic CDCA derivatives-induced apoptosis of stomach cancer cell line SNU-1 cells. Cancer Res. Treat. 36, 132–139. doi:10.4143/crt.2004.36.2.132
Nolan, E., Lindeman, G. J., and Visvader, J. E. (2023). Deciphering breast cancer: from biology to the clinic. Cell 186, 1708–1728. doi:10.1016/j.cell.2023.01.040
Palmela, I., Correia, L., Silva, R. F. M., Sasaki, H., Kim, K. S., Brites, D., et al. (2015). Hydrophilic bile acids protect human blood-brain barrier endothelial cells from disruption by unconjugated bilirubin: an in vitro study. Front. Neurosci. 9, 80. doi:10.3389/fnins.2015.00080
Pang, L., Zhao, X., Liu, W., Deng, J., Tan, X., and Qiu, L. (2015). Anticancer effect of ursodeoxycholic acid in human oral squamous carcinoma HSC-3 cells through the caspases. Nutrients 7, 3200–3218. doi:10.3390/nu7053200
Park, S. E., Choi, H. J., Yee, S. B., Chung, H. Y., Suh, H., Choi, Y. H., et al. (2004). Synthetic bile acid derivatives inhibit cell proliferation and induce apoptosis in HT-29 human colon cancer cells. Int. J. Oncol. 25, 231–236. doi:10.3892/ijo.25.1.231
Park, S. E., Lee, S. W., Hossain, M. A., Kim, M. Y., Kim, M. N., Ahn, E. Y., et al. (2008). A chenodeoxycholic derivative, HS-1200, induces apoptosis and cell cycle modulation via Egr-1 gene expression control on human hepatoma cells. Cancer Lett. 270, 77–86. doi:10.1016/j.canlet.2008.04.038
Park, K., Lee, G. Y., Park, R. W., Kim, I. S., Kim, S. Y., and Byun, Y. (2008). Combination therapy of heparin-deoxycholic acid conjugate and doxorubicin against squamous cell carcinoma and B16F10 melanoma. Pharm. Res. 25, 268–276. doi:10.1007/s11095-007-9366-5
Park, Y. H., Kim, J. A., Baek, J. H., Jung, E. J., Kim, T. H., Suh, H., et al. (1997). Induction of apoptosis in HepG2 human hepatocellular carcinoma cells by a novel derivative of ursodeoxycholic acid (UDCA). Arch. Pharm. Res. 20, 29–33. doi:10.1007/BF02974038
Patel, S., Challagundla, N., Rajput, R. A., and Mishra, S. (2022). Design, synthesis, characterization and anticancer activity evaluation of deoxycholic acid-chalcone conjugates. Bioorg Chem. 127, 106036. doi:10.1016/j.bioorg.2022.106036
Peiró-Jordán, R., Krishna-Subramanian, S., Hanski, M. L., Lüscher-Firzlaff, J., Zeitz, M., and Hanski, C. (2012). The chemopreventive agent ursodeoxycholic acid inhibits proliferation of colon carcinoma cells by suppressing c-Myc expression. Eur. J. Cancer Prev. 21, 413–422. doi:10.1097/CEJ.0b013e32834ef16f
Perino, A., Demagny, H., Velazquez-Villegas, L., and Schoonjans, K. (2021). Molecular physiology of bile acid signaling in health, disease, and aging. Physiol. Rev. 101, 683–731. doi:10.1152/physrev.00049.2019
Pourmanouchehri, Z., Ebrahimi, S., Limoee, M., Jalilian, F., Janfaza, S., Vosoughi, A., et al. (2022). Controlled release of 5-fluorouracil to melanoma cells using a hydrogel/micelle composites based on deoxycholic acid and carboxymethyl chitosan. Int. J. Biol. Macromol. 206, 159–166. doi:10.1016/j.ijbiomac.2022.02.096
Powell, A. A., LaRue, J. M., Batta, A. K., and Martinez, J. D. (2001). Bile acid hydrophobicity is correlated with induction of apoptosis and/or growth arrest in HCT116 cells. Biochem. J. 356, 481–486. doi:10.1042/0264-6021:3560481
Pyo, J. S., Ko, Y. S., Kang, G., Kim, D. H., Kim, W. H., Lee, B. L., et al. (2015). Bile acid induces MUC2 expression and inhibits tumor invasion in gastric carcinomas. J. Cancer Res. Clin. Oncol. 141, 1181–1188. doi:10.1007/s00432-014-1890-1
Qiao, D., Im, E., Qi, W., and Martinez, J. D. (2002). Activator protein-1 and CCAAT/enhancer-binding protein mediated GADD153 expression is involved in deoxycholic acid-induced apoptosis. Biochim. Biophys. Acta 1583, 108–116. doi:10.1016/s1388-1981(02)00190-7
Saeki, T., Yui, S., Hirai, T., Fujii, T., Okada, S., and Kanamoto, R. (2012). Ursodeoxycholic acid protects colon cancer HCT116 cells from deoxycholic acid-induced apoptosis by inhibiting apoptosome formation. Nutr. Cancer 64, 617–626. doi:10.1080/01635581.2012.669876
Saltzman, A. F., Cost, N. G., and Romao, R. L. P. (2023). Wilms tumor. Urol. Clin. North Am. 50, 455–464. doi:10.1016/j.ucl.2023.04.008
Schaff, L. R., and Mellinghoff, I. K. (2023). Glioblastoma and other primary brain malignancies in adults: a review. Jama 329, 574–587. doi:10.1001/jama.2023.0023
Shah, S. A., Volkov, Y., Arfin, Q., Abdel-Latif, M. M., and Kelleher, D. (2006). Ursodeoxycholic acid inhibits interleukin 1 beta [corrected] and deoxycholic acid-induced activation of NF-kappaB and AP-1 in human colon cancer cells. Int. J. Cancer 118, 532–539. doi:10.1002/ijc.21365
Shekels, L. L., Beste, J. E., and Ho, S. B. (1996). Tauroursodeoxycholic acid protects in vitro models of human colonic cancer cells from cytotoxic effects of hydrophobic bile acids. J. Lab. Clin. Med. 127, 57–66. doi:10.1016/s0022-2143(96)90166-3
Shen, Y., Lu, C., Song, Z., Qiao, C., Wang, J., Chen, J., et al. (2022). Ursodeoxycholic acid reduces antitumor immunosuppression by inducing CHIP-mediated TGF-β degradation. Nat. Commun. 13, 3419. doi:10.1038/s41467-022-31141-6
Shi, L., Jin, L., and Huang, W. (2023). Bile acids, intestinal barrier dysfunction, and related diseases. Cells 12, 1888. doi:10.3390/cells12141888
Singh, M., Bansal, S., Kundu, S., Bhargava, P., Singh, A., Motiani, R. K., et al. (2015). Synthesis, structure-activity relationship, and mechanistic investigation of lithocholic acid amphiphiles for colon cancer therapy. Medchemcomm 6, 192–201. doi:10.1039/C4MD00223G
Singh, M., Singh, A., Kundu, S., Bansal, S., and Bajaj, A. (2013). Deciphering the role of charge, hydration, and hydrophobicity for cytotoxic activities and membrane interactions of bile acid based facial amphiphiles. Biochimica Biophysica Acta (BBA) - Biomembr. 1828, 1926–1937. doi:10.1016/j.bbamem.2013.04.003
Song, W., Yang, H. B., Chen, P., Wang, S. M., Zhao, L. P., Xu, W. H., et al. (2013). Apoptosis of human gastric carcinoma SGC-7901 induced by deoxycholic acid via the mitochondrial-dependent pathway. Appl. Biochem. Biotechnol. 171, 1061–1071. doi:10.1007/s12010-013-0417-6
Song, X., Hu, Y., Li, Y., Shao, R., Liu, F., and Liu, Y. (2020a). Overview of current targeted therapy in gallbladder cancer. Signal Transduct. Target. Ther. 5, 230. doi:10.1038/s41392-020-00324-2
Song, X., Wang, X., Hu, Y., Li, H., Ren, T., Li, Y., et al. (2020b). A metagenomic study of biliary microbiome change along the cholecystitis-carcinoma sequence. Clin. Transl. Med. 10, e97. doi:10.1002/ctm2.97
Sreekanth, V., Bansal, S., Motiani, R. K., Kundu, S., Muppu, S. K., Majumdar, T. D., et al. (2013). Design, synthesis, and mechanistic investigations of bile acid-tamoxifen conjugates for breast cancer therapy. Bioconjug Chem. 24, 1468–1484. doi:10.1021/bc300664k
Sun, J., Mustafi, R., Cerda, S., Chumsangsri, A., Xia, Y. R., Li, Y. C., et al. (2008). Lithocholic acid down-regulation of NF-kappaB activity through vitamin D receptor in colonic cancer cells. J. Steroid Biochem. Mol. Biol. 111, 37–40. doi:10.1016/j.jsbmb.2008.01.003
Talebian, R., Hashem, O., and Gruber, R. (2020). Taurocholic acid lowers the inflammatory response of gingival fibroblasts, epithelial cells, and macrophages. J. Oral Sci. 62, 335–339. doi:10.2334/josnusd.19-0342
Tan, Y., Wang, Z., Xu, M., Li, B., Huang, Z., Qin, S., et al. (2023). Oral squamous cell carcinomas: state of the field and emerging directions. Int. J. Oral Sci. 15, 44. doi:10.1038/s41368-023-00249-w
Tang, Y., Blomenkamp, K. S., Fickert, P., Trauner, M., and Teckman, J. H. (2018). NorUDCA promotes degradation of α1-antitrypsin mutant Z protein by inducing autophagy through AMPK/ULK1 pathway. PLoS One 13, e0200897. doi:10.1371/journal.pone.0200897
Trah, J., Arand, J., Oh, J., Pagerols-Raluy, L., Trochimiuk, M., Appl, B., et al. (2020). Lithocholic bile acid induces apoptosis in human nephroblastoma cells: a non-selective treatment option. Sci. Rep. 10, 20349. doi:10.1038/s41598-020-77436-w
Vandewynckel, Y. P., Laukens, D., Devisscher, L., Paridaens, A., Bogaerts, E., Verhelst, X., et al. (2015). Tauroursodeoxycholic acid dampens oncogenic apoptosis induced by endoplasmic reticulum stress during hepatocarcinogen exposure. Oncotarget 6, 28011–28025. doi:10.18632/oncotarget.4377
Vogel, S. M., Bauer, M. R., Joerger, A. C., Wilcken, R., Brandt, T., Veprintsev, D. B., et al. (2012). Lithocholic acid is an endogenous inhibitor of MDM4 and MDM2. Proc. Natl. Acad. Sci. U. S. A. 109, 16906–16910. doi:10.1073/pnas.1215060109
Walz, A. L., Maschietto, M., Crompton, B., Evageliou, N., Dix, D., Tytgat, G., et al. (2023). Tumor biology, biomarkers, and liquid biopsy in pediatric renal tumors. Pediatr. Blood Cancer 70 (2), e30130. doi:10.1002/pbc.30130
Wang, Z., Li, W., Wang, X., Zhu, Q., Liu, L., Qiu, S., et al. (2023a). Isoliquiritigenin induces HMOX1 and GPX4-mediated ferroptosis in gallbladder cancer cells. Chin. Med. J. 136, 2210–2220. doi:10.1097/CM9.0000000000002675
Wang, Z., Qiang, X., Peng, Y., Wang, Y., Zhao, Q., and He, D. (2022). Design and synthesis of bile acid derivatives and their activity against colon cancer. RSC Med. Chem. 13, 1391–1409. doi:10.1039/d2md00220e
Wang, Z., Wang, S., Jia, Z., Hu, Y., Cao, D., Yang, M., et al. (2023b). YKL-40 derived from infiltrating macrophages cooperates with GDF15 to establish an immune suppressive microenvironment in gallbladder cancer. Cancer Lett. 563, 216184. doi:10.1016/j.canlet.2023.216184
Wu, Y. C., Chiu, C. F., Hsueh, C. T., and Hsueh, C. T. (2018). The role of bile acids in cellular invasiveness of gastric cancer. Cancer Cell Int. 18, 75. doi:10.1186/s12935-018-0569-0
Wu, Z., Lü, Y., Wang, B., Liu, C., and Wang, Z. R. (2003). Effects of bile acids on proliferation and ultrastructural alteration of pancreatic cancer cell lines. World J. Gastroenterol. 9, 2759–2763. doi:10.3748/wjg.v9.i12.2759
Yang, H. B., Song, W., Cheng, M. D., Fan, H. F., Gu, X., Qiao, Y., et al. (2015). Deoxycholic acid inhibits the growth of BGC-823 gastric carcinoma cells via a p53-mediated pathway. Mol. Med. Rep. 11, 2749–2754. doi:10.3892/mmr.2014.3004
Yang, M., Gu, Y., Li, L., Liu, T., Song, X., Sun, Y., et al. (2021). Bile acid-gut microbiota Axis in inflammatory bowel disease: from bench to bedside. Nutrients 13, 3143. doi:10.3390/nu13093143
Yao, Z., Zhang, X., Zhao, F., Wang, S., Chen, A., Huang, B., et al. (2020). Ursodeoxycholic acid inhibits glioblastoma progression via endoplasmic reticulum stress related apoptosis and synergizes with the proteasome inhibitor bortezomib. ACS Chem. Neurosci. 11, 1337–1346. doi:10.1021/acschemneuro.0c00095
Yee, S. B., Song, Y. S., Jeong, S. H., Lee, H. S., Seo, S. Y., Kim, J. H., et al. (2007). A novel chenodeoxycholic derivative HS-1200 enhances radiation-induced apoptosis in MCF-7 cells. Oncol. Rep. 17, 919–923. doi:10.3892/or.17.4.919
Yee, S. B., Yeo, W. J., Park, B. S., Kim, J. Y., Baek, S. J., Kim, Y. C., et al. (2005). Wilfoside K1N isolated from Cynanchum wilfordii inhibits angiogenesis and tumor cell invasion. Int. J. Oncol. 27, 653–659. doi:10.3892/ijo.26.6.1533
Yu, H., Fu, Q. R., Huang, Z. J., Lin, J. Y., Chen, Q. X., Wang, Q., et al. (2019). Apoptosis induced by ursodeoxycholic acid in human melanoma cells through the mitochondrial pathway. Oncol. Rep. 41, 213–223. doi:10.3892/or.2018.6828
Yu, M. K., Lee, D. Y., Kim, Y. S., Park, K., Park, S. A., Son, D. H., et al. (2007). Antiangiogenic and apoptotic properties of a novel amphiphilic folate-heparin-lithocholate derivative having cellular internality for cancer therapy. Pharm. Res. 24, 705–714. doi:10.1007/s11095-006-9190-3
Zeng, H., Botnen, J. H., and Briske-Anderson, M. (2009). Deoxycholic acid and selenium metabolite methylselenol exert common and distinct effects on cell cycle, apoptosis, and MAP kinase pathway in HCT116 human colon cancer cells. Nutr. Cancer 62, 85–92. doi:10.1080/01635580903191551
Zeng, H., Claycombe, K. J., and Reindl, K. M. (2015). Butyrate and deoxycholic acid play common and distinct roles in HCT116 human colon cell proliferation. J. Nutr. Biochem. 26, 1022–1028. doi:10.1016/j.jnutbio.2015.04.007
Zhang, D., Zhu, Y., Su, Y., Yu, M., Xu, X., Wang, C., et al. (2022). Taurochenodeoxycholic acid inhibits the proliferation and invasion of gastric cancer and induces its apoptosis. J. Food Biochem. 46, e13866. doi:10.1111/jfbc.13866
Zhu, L., Shan, L. J., Liu, Y. J., Chen, D., Xiao, X. G., and Li, Y. (2014). Ursodeoxycholic acid induces apoptosis of hepatocellular carcinoma cells in vitro. J. Dig. Dis. 15, 684–693. doi:10.1111/1751-2980.12191
Zhu, S., Yang, K., Yang, S., Zhang, L., Xiong, M., Zhang, J., et al. (2022). A high bile acid environment promotes apoptosis and inhibits migration in pancreatic cancer. Bioengineered 13, 6719–6728. doi:10.1080/21655979.2022.2045823
Keywords: bile acid biosynthesis, primary bile acid, secondary bile acid, anticancer activity, bile acid derivatives
Citation: Li W, Zou L, Huang S, Miao H, Liu K, Geng Y, Liu Y and Wu W (2024) The anticancer activity of bile acids in drug discovery and development. Front. Pharmacol. 15:1362382. doi: 10.3389/fphar.2024.1362382
Received: 28 December 2023; Accepted: 29 January 2024;
Published: 20 February 2024.
Edited by:
Qingbin Cui, University of Toledo College of Medicine and Life Sciences, United StatesReviewed by:
Yang Tian, University of Arkansas, United StatesXiaolin Qian, Southern Research Institute, United States
Copyright © 2024 Li, Zou, Huang, Miao, Liu, Geng, Liu and Wu. This is an open-access article distributed under the terms of the Creative Commons Attribution License (CC BY). The use, distribution or reproduction in other forums is permitted, provided the original author(s) and the copyright owner(s) are credited and that the original publication in this journal is cited, in accordance with accepted academic practice. No use, distribution or reproduction is permitted which does not comply with these terms.
*Correspondence: Yajun Geng, Z2VuZ3lhanVuQHJlbmppLmNvbQ==; Yingbin Liu, bGFvbml1bHliQHNoc211LmVkdS5jbg==; Wenguang Wu, d3V3ZW5ndWFuZzA4QDEyNi5jb20=
†These authors have contributed equally to this work