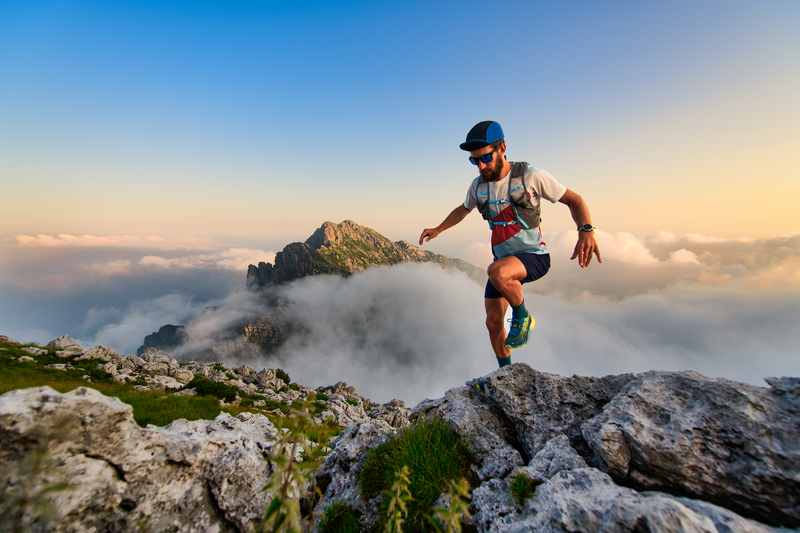
94% of researchers rate our articles as excellent or good
Learn more about the work of our research integrity team to safeguard the quality of each article we publish.
Find out more
REVIEW article
Front. Pharmacol. , 17 July 2023
Sec. Ethnopharmacology
Volume 14 - 2023 | https://doi.org/10.3389/fphar.2023.1214881
This article is part of the Research Topic Advances in Discoveries of Plant Phytochemicals View all 16 articles
Alzheimer’s disease (AD) is the most prevalent form of dementia affecting millions of people worldwide. It is a progressive, irreversible, and incurable neurodegenerative disorder that disrupts the synaptic communication between millions of neurons, resulting in neuronal death and functional loss due to the abnormal accumulation of two naturally occurring proteins, amyloid β (Aβ) and tau. According to the 2018 World Alzheimer’s Report, there is no single case of an Alzheimer’s survivor; even 1 in 3 people die from Alzheimer’s disease, and it is a growing epidemic across the globe fruits and vegetables rich in glucosinolates (GLCs), the precursors of isothiocyanates (ITCs), have long been known for their pharmacological properties and recently attracted increased interest for the possible prevention and treatment of neurodegenerative diseases. Epidemiological evidence from systematic research findings and clinical trials suggests that nutritional and functional dietary isothiocyanates interfere with the molecular cascades of Alzheimer’s disease pathogenesis and prevent neurons from functional loss. The aim of this review is to explore the role of glucosinolates derived isothiocyanates in various molecular mechanisms involved in the progression of Alzheimer’s disease and their potential in the prevention and treatment of Alzheimer’s disease. It also covers the chemical diversity of isothiocyanates and their detailed mechanisms of action as reported by various in vitro and in vivo studies. Further clinical studies are necessary to evaluate their pharmacokinetic parameters and effectiveness in humans.
In the past few decades, owing to healthy habits and general improvements in lifestyle and medication, life expectancy has substantially increased; however, the prominent upward shift in age distribution has increased the prevalence of chronic diseases, including Alzheimer’s disease (AD). AD slowly affects the brain and exhibits clear pathological changes in the hippocampus, the centre of memory and learning (Zhang et al., 2020). In AD, the propensity of neurotoxic proteins to form template or oligomers is higher and accelerates the conversion and aggregation of endogenous proteins, which eventually convert into fibrils (Schaffert and Carter, 2020). It can be sporadic or familial and AD cases are sporadic in most instances (Dorszewska et al., 2016). Disease modifying treatments primarily focused on reducing amyloid beta (senile plaques, Aβ) and tau (neurofibrillary tangles) load in the brain (Cammisuli et al., 2022). Despite many costly clinical trials ranging from pharmacological to hormonal treatments and immunotherapy, not even a single drug produced clinically significant results due to suboptimal dosing of drugs, unavailability of reliable biomarkers for early diagnosis and more specifically lack of detailed mechanistic approaches (Lashley et al., 2018; Loewenstein, 2022). The existing medication exert only moderate reduction of symptoms; therefore, AD remains symptomatic and can be controlled and prevented but uncured (Fernández and Ribeiro, 2018).
According to the World Alzheimer Report (2018), there are 50 million people living with dementia worldwide, of which 70–80 percent are AD patients, and by 2050 these numbers will be more than triple to 152 million (Patterson, 2018). From the data provided by the World Health Organization (WHO), it is an epidemic worldwide and has become a global burden (Cao et al., 2020). Death from AD has increased 123 percent between 2000–2005 and more than 60 percent cases are from low to middle income countries (Patterson, 2018). At the beginning of 21st century, AD remains a major biomedical challenge. Pharmaceutical companies and neurobiologists around the world are doing their efforts to develop novel FDA approved drugs such as acetyl cholinesterase (AChE) inhibitors (Donepezil, Rivastigmine and Galantamine) and NMDA (n-methyl D aspartate) receptor antagonist (Memantine) but they showed several side effects in phase II and III clinical trials. Common adverse effects of AChE inhibitors are diarrhea, nausea, vomiting, bradycardia, muscle twitching nightmares, etc., and memantine includes dizziness, headache, and lethargy (Ettcheto et al., 2018; Schneider, 2022).
The discovery of new natural pharmacologically active compounds is a widely growing field, as the synthesis of most the biomolecules is tough task (Ramawat and Arora, 2021). Consumption of antioxidant rich food and vegetables might improve brain function, minimize the possibilities of cognitive impairment, retard the process of aging, subsequent oxidation, and disease progression (Andrade et al., 2019). It is clinically proven that they enhance cellular metabolism and nourish brain cells; this safeguarding impact is more potent when isothiocyanates (ITCs) rich fruits and vegetables are specifically consumed (Esteve, 2020; Kamal et al., 2022). The propitious attributes of fruits and vegetables are related to their nutritional and functional components like minerals, vitamins, antioxidants and polyphenols. All of these molecules are found in cruciferous vegetables, however, the sulfurous compound GLCs that give them their distinctive pungent aroma and flavour set them apart. GLCs are stable chemically but biologically inactive and remain sequestered within plant compartment (Verkerk et al., 2009; Alexandre et al., 2020). Tissue damage and chewing are the main causes that lead to the formation of biologically active derivatives of GLCs such as ITCs by enzyme hydrolysis, which directly and indirectly regulate their activity and have been demonstrated to exert neuroprotective properties through multiple mechanisms (Tian et al., 2018).
Generally, there are three major hypothesis, i.e., AChE, amyloid, and tau, which are primarily implicated in Alzheimer’s disease management and prevention. Beside them, neuroinflammation is another important response target involving biochemical events activating resident cells of the central nervous system (CNS), which may induce the entire process of AD. It is initiated by aberrant astrocytes and microglial activation, which leads to the release of different inflammatory mediators such as nitric oxide (NO), prostaglandin E2 (PGE-2), reactive oxygen species (ROS), cytokines and chemokines (Kraft and Harry, 2011). Furthermore, it elevates the level of proinflammatory cytokines such as tumor necrosis factor (TNF-α), interleukin-1β (IL-1β) and interleukin-6 (IL-6), which are responsible for neuronal death (Xia et al., 2015). Controlling microglia and astrocytes activation can therefore be a therapeutic approach in the prevention and management of AD. Recently, it has been shown that ITCs possess neuroprotective effects through the modulation of different signalling pathways (Latronico et al., 2021). In oxidative stress and inflammation control, nuclear factor-kβ (NF-kβ) and nuclear erythroid related factor 2 (Nrf2) are two main regulators (Fão et al., 2019). They may primarily be attributed to their peculiar ability to activate the Nrf2/ARE pathway (Giacoppo et al., 2015). ITCs significantly decrease NF-kβ translocation with the inhibition of proinflammatory cytokines (Latronico et al., 2021). Hydrogen sulphide (H2S) is another important signal molecule in CNS; it could represent an intriguing strategy for the treatment of neurodegenerative diseases (Tabassum and Jeong, 2019; Sharif et al., 2023). Beside this, it also play a key role in many aspects of human health like in antiproliferation, cardioprotection, chemoprevention, etc. (Martelli et al., 2020). It also interacts with redox system regulating cellular oxidative stress and ROS (Kabil and Banerjee, 2010). There is a strong relationship between H2S and aging, as consistent significant decline of H2S levels has been observed in AD patients (Disbrow et al., 2021). H2S is a relevant player accounting for different biophysiological effects of Brassicaceae plants, for example, Allyl isothiocyanate (AITC) from black mustard (B. nigra), benzyl-ITC from garden cress (Lepidium sativum), erucin form Eruca sp., B. oleirecia, etc. and 4-hydorxybenzyl-ITC from white mustard (B. alba) are some important naturally occurring ITCs. Among these selected ITCs, benzyl ITC is the most effective H2S donor, exhibiting remarkable H2S release followed by AITC (Citi et al., 2014). Recently, available literature clearly demonstrated that the role of natural ITCs as H2S donor (Martelli et al., 2020). It is a pleiotropic mediator that affects different element in inflammatory cascade specially NF-kβ and Nrf2 signalling (Zhao et al., 2023).
Another important effect of ITCs is apoptotic suppression as they can intervene and arrest the mitochondrial apoptotic pathway (Dinkova-Kostova and Kostov, 2012). Deposition of Aβ and hyperphosphorylated tau proteins is a crucial event in AD as pathology several studies demonstrated the pharmacological potencies of ITCs against these two hallmarks and their toxicity by intervene in its cascade such as APP cleavage, BACE1 expression, oligomerization of seeded proteins, phosphorylation and dephosphorylation assembly, etc. (Morroni et al., 2018; Asif et al., 2022). ITCs could therefore be considered as a promising source of medicine and for the treatment and management of AD. This review focuses on the knowledge regarding the direct and indirect mechanisms by which GLCs-derived ITCs intervene in inhibition of AChE, neurotoxic proteins (Aβ and tau) and neuroinflammation cascade.
Glucosinolates (GLCs), a group of sulphur containing glycosides and their hydrolysis products, i.e., isothiocyanates (ITCs) are abundantly found in the family Brassicaceae which encompasses our daily vegetables including cabbage, broccoli, mustard, white radish, radish, kale, turnip, oilseed rape, collard greens, daikon, kohlrabi, wasabi, cauliflower, Brussels, etc. (Cancer et al., 2004; Shree et al., 2022). These metabolites distinguish them from other plant families and are responsible for pungent smell and bitter taste (Verkerk et al., 1998; Barba et al., 2016). Besides this, they are also found in Moringa oleifera (drumstick tree), a plant from the family Moringaceae; in contrast with other Brassicaceae plants, only aromatic GLCs have been identified in M. oleifera (Lopez-Rodriguez et al., 2020). More than 200 GLCs have already been characterized so far, although a small number of these compounds are present in closely related taxonomic groups and not all are present in plants that people consume (Fahey et al., 2001; Agerbirk and Olsen, 2012). Its content varies between different cultivars and plant species even in plant parts such as seeds, stems, roots, and leaves, while the highest amount is present in young tissues (Blažević and Mastelić, 2009). These variations arise from several factors (genetic, nutrient and environmental) and growth conditions (temperature, nutrient availability and water content).
GLCs are structurally thiohydroximates containing S-linked β-glucopyranosyl and O-linked sulfate residues with different side chains derived from amino acids (Agerbirk and Olsen, 2012). They are synthesized by different amino acid precursors such as phenylalanine, tryptophan, and methionine, which give rise to molecules with side chain R (Table 1; Figure 1). All known GLCs display structural homogeneity with different R groups in producing their corresponding ITCs responsible for various biological activities (Agerbirk and Olsen, 2012). On the basis of their side chain they are characteristically subdivided into three groups (Ali et al., 2018; Huke et al., 2021) as shown in Table 1: i) long chain length aliphatic; ii) short to medium chain length aliphatic (only C3 and C3 or C4 with C5) and iii) simple aryl aliphatic such as benzyl, phenyl, hydroxybenzyl GLCs; highly substituted aryl aliphatic such as dihydroxy, dimethoxy and trimethoxy benzyl GLCs. C3-C5 aliphatic GLCs are commonly found in Brassica species (Bennett et al., 2004).
TABLE 1. Trivial name, side chain structure and dietary plant source of Glucosinolates and Isothiocyanates.
ITCs are a specific type of compound derived from the hydrolysis of GLCs along with nitriles and thiocyanates. The entire conversion is catalyzed by endogenous myrosinase (thioglucoside glucohydrolase) enzyme released after chopping and chewing of raw vegetables or physical damage such as insect attack (Oliviero et al., 2018). Myrosinase reacts with GLCs by hydrolytically cleaving thio-linked glucose and forms active ITCs by an unstable intermediate thiohydroximate-O-sulfonate after immediate rearrangement depending on the corresponding substrate (GLCs), pH, temperature, epithiospecifier proteins (ESP), ferrous ions and thiocyanate forming proteins (TFP) (Sikorska-Zimny and Beneduce, 2021) as shown in Figure 2. Extraction and isolation of GLCs and their hydrolysis product ITCs are still challenging due to their sensitive nature. In recent years, different methods have been developed for the detection and quantification of GLCs and ITCs, mainly UHPLC-DAD-ESI-MS and HPLC-DAD-ESI-MS for GLCs (Devkota, 2020) and HPLC-DAD and UHPLC-HRMS/MS for ITCs (Karanikolopoulou et al., 2021). If myrosinase is denatured during ingestion, GLCs metabolism can also be triggered by gut microbiota (Luang-In et al., 2014). In such conditions, GLCs are absorbed in the stomach and then transit to the small intestine and colon where they hydrolyzed by microbiota (Barba et al., 2016). Long cooking time and high cooking temperature (>80°C) triggered myrosinase denaturation and significant GLCs and ITCs loss (more than 90%), but after ingestion, gut bacteria promote the conversion of GLCs into ITCs, which are then absorbed; therefore, a preferable method is steaming over boiling the raw food to minimize metabolite loss (Barba et al., 2016; Shakour et al., 2022).
FIGURE 2. Enzymatic hydrolysis reaction of GLCs and their corresponding breakdown products (ESP; epithiospecifier protein).
Bioavailability is an essential parameter that determines the action of metabolites. It represents absorption, distribution, metabolism, and excretion unlike drugs, where the oral concentration is predetermined. It depends on the number of food products, which is highly variable (Gupta and Robinson, 2017). It is evidently proved that ITCs are absorbed in higher amounts by passive diffusion from the gastrointestinal tract after ingestion into blood capillaries where they bind with free plasma proteins (thiocarbomylation) and pass into tissues cells where they affect their biophysiological mechanism (Kołodziejski et al., 2019). In a recent investigation, it was observed that broccoli converts gut microbiota to healthier profile, which coincides with myrosinase activity (Sikorska-Zimny and Beneduce, 2021). Most studies conducted among humans revealed that mercapturic acid pathway is involved in ITCs metabolism. One study using human urine explained that the ITCs can be absorbed indirectly through cylcocondensation determined by measuring plasma ITCs level after oral dose through high performance liquid chromatography with tandem mass spectrometry (HPLC-MS/MS) (Zhang and Zhang, 2017). Another study conducted on a rat model using radiolabel ITCs (14C) as an oral dose revealed the rapid absorption of ITCs, but the structure of individuals affects liposolubility (Chang et al., 2012). Both investigations observed that ITCs entered into enterocytes and glutathione S-transferase (GST) conjugated with glutathione favors internal accumulation and concentration gradient. Kidney and liver are involved in entire conversion because the liver contains high levels of glutathione and highest GST activity and plays a crucial role in xenobiotic detoxification by supporting accumulation of conjugated ITCs (Esteve, 2020). These conjugated ITCs are converted to mercapturic acid derivatives, which are implicated by the kidney due to the presence of γ-glutamyltranspeptidase (γ-GT), N-acetyltransferase (AT), and cysteinylglycinase (CGase), after they are excreted in urine (Shakour et al., 2022).
Neurons are the building blocks of the CNS, incapable of reproducing and replacing themselves. Several pathological disorders including AD are caused by the accumulation of reactive oxygen species (ROS) in cells (Deshmukh et al., 2017). The ability of a compound to possess anti-inflammatory, antioxidative, and/or antiapoptotic properties is currently used to establish neuroprotective and neuroinflammatory functions (Dinkova-Kostova and Kostov, 2012). ITCs were reported to play a protective effect in acute and chronic AD (Kamal et al., 2022). A variety of ITCs have been experimentally proven (Table 2) to reduce oxidative stress, inflammation, excitotoxicity, misfolded proteins, and mitochondrial dysfunction, and prevent programmed cell death (Connolly et al., 2021). Through the activation of ARE (antioxidant response element) driven genes, ITCs are strong Nrf-2 (nuclear factor erythroid factor 2) activators. They strongly suppress inflammation via NF-kβ (nuclear factor kappa light chain enhancer of activated β cells) pathway (Sita et al., 2016).
TABLE 2. The beneficial effects and mechanism of action of ITCs on various models of Alzheimer’s disease.
A deficient and non-equilibrium cholinergic neurotransmission is responsible for the pathophysiology of learning and memory resulting behavioral disturbance, progressive loss of cognition and daily routine function (Hoyer, 2004; Craig et al., 2011). In context with the cholinergic hypothesis, decreasing the amount of acetylcholine in the hippocampus and cerebral cortex leads to the dysregulation of ChAT and premature loss of basal forbidden cholinergic neurons (Burčul et al., 2018; Hampel et al., 2019). One of the most significant properties of ITCs is AChE inhibition implicated in acetylcholine neurotransmission (Figure 3). In one study, 11 different ITCs were evaluated for their AChE inhibitory and anti - inflammation properties; the most promising inhibitory activity among 11 ITCs was demonstrated by phenyl isothiocyanate and its derivatives. The most potent AChE inhibitory activity was shown by 2-methoxyphenyl ITC with IC50 value of 0.57 mM. Human COX-2 enzyme was also used to evaluate the anti-inflammatory activity, ranking phenyl ITC and 2-methoxy phenylITC as the most potent with 99% inhibition at 50 μM (Burčul et al., 2018). Moringine-specific benzyl ITC from Moringa Oleifera modulated the Nrf2/AER pathway, proinflammatory biomarkers, and apoptotic pathway in different mouse and rat models (Galuppo et al., 2014, (Galuppo et al., 2015). In another mouse model (LPS induced), it was found that ITCs effectively decreased TNF-α, IL-1β, IL-6 and inhibited NF-kβ (Sailaja et al., 2022). It also downregulated senescence as it promoted neuronal repair in in vitro Aβ induce SH5Y5Y cells (Silvestro et al., 2021).
FIGURE 3. The role of GLCs derived ITCs in AChE inhibition characterized by impaired acetylcholine neurotransmission.
Through different mechanisms (explained in Table 2), SFN prevented cognitive impairment, reduced the Aβ and tau biomarkers, oxidative stress, inflammation and neurodegeneration in experimental models (Kim, 2021). SFN was able to improve spatial and contextual memory through the Y-maze test and counteract the Aβ aggregate induced memory deficits induced by intracerebroventricular (ICV) injection in a mouse model (Kim, 2021). In the hippocampus and frontal cortex, SFN increased cholinacetyltransferase (ChAT) expression, decreased acetylcholine esterase (AChE) activity, and raised the level of acetylcholine (AChE) (Lee et al., 2014). In another study on a transgenic AD mouse model, it was observed that SFN not only reduced the production and deposition of Aβ plaques in the hippocampus and cerebral cortex but also it is associated with neurobehavioral deficit (Zhang et al., 2015; 2017). The neuroinflammatory inhibition is through the activation of Nrf2/HO-1 pathway and inhibition of JNK/AP-1/NF-Kβ by SFN. SFN significantly increased proteasome activity and enhance Nrf-2 pathway in murine neuroblastoma cell lines (Park et al., 2009). It also modulated the Nrf2/ARE pathway in an AlCl3-and D-galactose induced mice (Zhang et al., 2014).
Neurogenesis has been shown to be enhanced by AITC and PEITC. AChE inhibitory activity in AD revealed that PEITC inhibited the enzyme more effectively than benzyl ITC and AITC (Burčul et al., 2018). In another study, PEITC inhibited Akt activation, suppressed NO production through INF induction, and had an anti-inflammatory effect (Okubo et al., 2010). PEITC showed a protective effect by modulating the MAPK pathway (Ma et al., 2017). Experimental findings revealed that in LPS-induced inflammation model, AITC showed a neuroprotective effect mediated through downregulation of JNK/NF-kβ/TNF-α signaling (Subedi et al., 2017). It also activated ROS-dependent TRPA1 signaling, resulting in neurogenic inflammation reduction in cultured Schwann cells in vitro (De Logu et al., 2022a; De Logu et al., 2022b). PEITC decreased inflammation and activated the cytoprotective pathway in transgenic mice model by modulating Nrf2/AER pathway and restoring Nrf-2 expression (Boyanapalli et al., 2014; Dayalan Naidu et al., 2018). In another study using LPS-activated rat astrocyte culture, PEITC significantly downregulated MAPK/ERK signaling and influenced the inflammatory pathway (Latronico et al., 2021). Increasing evidences suggests that cytochrome p450 is fundamental for brain homeostasis and function while phase II enzyme such as glutathione S-transferase play a key role in redox homeostasis. Modulation of these enzymes can be achieved by ITCs, in the recent studies glucuronosyltranseferase expression increase by sulforaphane in HepG2 cells, in another study erucin and phenethyl ITC elevated glucuronosyltranseferase activity in rat liver slices (Abdull Razis and Mohd Noor, 2013).
Moringa oleifera extract (MOE) decreased the neuritis resulting from naturally occurring cellular injury, with the development of multipolar primary process (Hannan et al., 2014). It also suppressed oxidative stress, MDA, nitrite and TNF-α, increased SOD and inflammation and improved spatial memory and cholinergic neurotransmission by reducing AChE activity and loss of cortico-hippocampus neurons in rat model fed with M. oleifera seeds in dose dependent manner (Onasanwo et al., 2021). Moringa oleifera extract also scavenged free radicals produced by NO, iNOS and nitrotyrosine increase Nrf2 in LPS-activated macrophages and downregulated antioxidative genes; HO-1, GST-P1 and NQO- (Jaja-Chimedza et al., 2017). In another study, it significantly inhibited AChE and reduced neurodegeneration in an NDD/Al - induced temporocortical degenerated mice model (Ekong et al., 2017). Moringa oleifera - supplemented male Wistar rats showed improved memory when evaluated by the Morris water Maze test and significantly reduced AChE levels in brain tissues in a dose-dependent manner (Adebayo et al., 2021). In another observation, GMC-ITC treated neuronal cells (SH-SY5Y) significantly alleviate oxidative stress condition by reducing ROS level ((Jaafaru et al., 2019a). Glucomoringin ITC (GMC-ITC) isolated from M. oleifera seeds abrogated oxidative stress and showed neuroprotective activity against cytotoxic neuroblastoma cells (SH-SY5Y) induced by H2O2, gene expression study of detoxifying markers (phase II) by GMC-ITC revealed that all involved genes significantly express themselves. It also decreased the expression of NF-kβ and increased the expression of Ikβ, Nrf2, SOD-1, NQO1 and Nf-kβ respectively (Jaafaru et al., 2019b). Eruca sativa extract (ESE) with a high amount of erucin (ER) prevented cell death and degeneration induced by LPS in NSC-34 motor neurons exposed to LPS-stimulated macrophage cell culture medium by inhibiting FasL (tumor necrosis factor ligand superfamily number 6 expression) and suppressing pro-inflammatory mediators (attenuates TLR4, COX-2 expression of TNF-α level) (Gugliandolo et al., 2018). Erucin decreased inflammation in different cell line models, counteracted proinflammatory marker expression, and balanced Erk1/2, P38, and JNK signaling (Yehuda et al., 2012; Wagner et al., 2015). Indol 3 carbinol (I3C) is another promising candidate found in vegetables; it reduces the free radical production in neuronal cells (Mammana et al., 2019). It also showed the potent radical scavenging activity by chelating already produced free radical species (Giacoppo et al., 2015). In another study, it suppressed the expression of NO, COX-2, and iNOS in the brain, which prevented apoptosis and inflammation by inhibiting NF-kβ and IB phosphorylation (Kim et al., 2014). Furthermore, it decreased BDNF, GHS and increased TNF-α, IL1-β in mice model, it also helped in suppression of neurodegeneration (Huang et al., 2022). In another experiment, researchers explored the antioxidant and anti-inflammatory activity of SFN and ERN as H2S donor through the combination with rivastigmine in microglia and neuronal cell line (SH-SY5Y). Result revealed that both derivatives show significant antioxidant and anti inflammatory activities in microglial cell line, expression of antioxidant defense protein (GSH) was also induced in neuronal cell line. It significantly decreased the ROS production and NO release in microglial BV-2 cells. Further Erucin exerted a time dependent Nrf2 activation in SH-SY5Y cells (Sestito et al., 2019). When anti-inflammatory effect of erucin was evaluated in LPS-challenged umbilical vein endothelial cells (HUVECs), it significantly prevented the increase of ROS, TNF-α levels and decreased COX-2. It also induced NF-kβ (Ciccone et al., 2022).
The brain of people suffering from Alzheimer’s disease shows remarkable accumulations of two neurotoxic proteins Aβ and tau (Cao et al., 2020). So far, several Alzheimer’s plaque and tau inhibitors from different sources are available they can target different mechanistic steps of fibril formation. One of the inhibitors that are widely used to stop protein aggregation is GLCs derivatives ITCs as they are consumed as a part of our daily diet (Lopez-Rodriguez et al., 2020). In Table 3, we have discussed some of the GLCs derived ITCs, proposed as the potential inhibitor of misfolded Aβ and tau aggregation and their induced toxicity by different mechanisms and modulation of multiple pathways (Figures 4, 5) as described earlier (Grande et al., 2020). Recent investigations suggested that they may directly interact with misfolded proteins during very early stages of the aggregation cascade by binding and stabilizing unfolded proteins and redirecting the aggregation pathways to form amorphous nontoxic fibrils, blocking seeding and further conformational changes that result in neurotoxicity and cell death.
FIGURE 4. The potential role of ITCs in Aβ metabolism and related toxicity: sAPPα and C83 (membrane associated fragment) are formed by nonamyloidogenic pathway in which APP is cleaved by α-secretase, while in amyloidogenic pathway APP is cleaved by β-secretase producing S APPβ and C99 fragment, γ-secretase then processed the C99 and release Aβ. ITCs prevent from amyloidogenic cleavage by inhibiting β-secretase, further it inhibits nucleation, polymerization and plaques formation. It directly intervenes in Aβ induced neurotoxicity by altering Ca2+ homeostasis, downregulating cascade of caspase and in reducing inflammation.
FIGURE 5. The potential role of ITCs in disease modification, targeting tau protein and its aggregation. Defective microtubules resulting in impaired axonal transport due to kinases and phosphatase imbalance resulting destabilized microtubule formation. Detached hyperphosphorylated tau monomers oligomerized and form NFTs leads to cellular death.
6-(Methylsulfinyl) hexyl isothiocyanate (6-MSITC) from Wasabia japonica was evaluated against amyloidosis in a murine mice model in which 6-MSITC was induced by intra cerebroventricular injection of Aβ1-42 oligomers. Behavioral analysis revealed that it reduced Aβ1-42 induced memory impairment in hippocampus tissues, increased ROS, and decreased glutathione levels following Aβ1-42 injection (Morroni et al., 2018). In another study, the authors observed that Aβ25-35 induced mitochondrial dependent cell death was blocked by SFN through Nrf2-associated manner (Brasil et al., 2023). Clinically, it inhibited Aβ, reduced its burden, and increased the expression of p75NTR in an intransgenic mouse model (Zhang et al., 2015). In another investigation, SFN was found to suppress Aβ deposition, improve cognition, and locomotor function in aluminum and D-galactose-induced mouse model (Zhang et al., 2017). It modulated the Aβ expression related markers followed CDK5 overexpression inhibition in primary neurons, further it reduced Aβ1-42 induced neurotoxicity and its deposition in TgCRND8-transgenic mice brains. It also suppressed tau phosphorylation at specific sites (Yang et al., 2023). It reduced and altered hyperphosphorylated tau proteins in embryonic hippocampal rat astrocytes under hypoglycaemic condition at Th 181 and Sr 991/202 within astrocytes (Komiskey et al., 2022). It induced NDP52 by Nrf2 and cleared the phosphorylated tauproteins in mice model (Jo et al., 2014).Through high affinity molecular recognition by heteromeric interaction of Aβ plaques, I3C were found to strongly reduce Aβ fibril formation as observed in microscopic examination by TEM analysis (Cohen et al., 2006).
M.oleifera is profoundly used against chronic diseases including AD. Mitochondrial apoptotic genes profile through GMC-ITC pre-treated SH-SY5Y neuronal cells revealed that it protect the cells against oxidative stress via apoptotic pathway, it significantly downregulate the expression of Bax, CASP3, CASP8, CASP9, Apaf-1, cyt-c, p-53 genes and upregulate Bcl2 gene in mitochondrial apoptotic signalling pathway (Jaafaru et al., 2019a). In another study GMC-ITC from the seeds of M. oleifera significantly decreased the expression of BACE1, APP and increased the expression of MAPT tau genes in H2O2 induced cytotoxic neuroblastoma cell (SH-SY5Y) (Jaafaru et al., 2019b). It decreases Aβ production and enhance the synaptic proteins in HHcY induced AD model bydown regulating BACE1. It also played crucial role in Ca2+ homeostasis, as it deactivated calpain by decreasing intracellular Ca2+ resulting cytosolic protease calpain activity reduction in HHcY induced rat model (Mahaman et al., 2018). In another study conducted on MO-ZnONP treated Sprague Dawley rat model it reduced the Aβ accumulation and helped in sustained brain-Zn content (Dahran et al., 2023).
GLCs derived ICTs are important bioactive natural products that are found in many Brassicaceae plants and few plants from other families. In vitro and animal studies have reported their beneficial effects in neuroprotection and they are reported to enhance cellular metabolism, nourish brain cells, and reduce risk factors associated with neurodegeneration. ITCs inhibit inflammatory mediators, oxidative stress, cellular stress signaling, and improve behavioural measures. They also easily cross the blood brain barrier to interact with particular targets implicated in AD pathogenesis. However, there is no sufficient clinical evidence to prove these effects in humans. Future studies should focus to evaluate their pharmacokinetic parameters and effectiveness in humans.
JA contributed to the study conception and design. The first draft of the manuscript was written by FK. All tables and figures have been prepared by FK. AJ has performed literature survey. JA, HPD, AJ, VS, and VK revised and updated the manuscript. All authors read and approved the final manuscript.
The authors declare that the research was conducted in the absence of any commercial or financial relationships that could be construed as a potential conflict of interest.
All claims expressed in this article are solely those of the authors and do not necessarily represent those of their affiliated organizations, or those of the publisher, the editors and the reviewers. Any product that may be evaluated in this article, or claim that may be made by its manufacturer, is not guaranteed or endorsed by the publisher.
AD, Alzheimer’s disease; AITC, allylisothiocyanate; APP, amyloid precursor protein; Aβ, amyloid beta; BBB, blood brain barrier; ER, erucin; GLCs, glucosinolates; I3C, indole 3 carbinol; IL-1β, interleukin-1β; iNOS, inducible nitric oxide synthase; ITCs, isothiocyanates; MAPK, mitogen activated protein kinase; MO, Moringa oleifera; NF-kβ, nuclear factor kappa B; NFT, neurofibrillary tangles; PEITC, phenethylisothiocyanate; p-tau, phosphorylated tau; ROS, reactive oxygen species; SFN, sulforaphan; TNF-α, tumor necrosis factor- α.
Abdull Razis, A. F., De Nicola, G. R., Pagnotta, E., Iori, R., and Ioannides, C. (2013). A glucosinolate-rich extract of Japanese Daikon perturbs carcinogen-metabolizing enzyme systems in rat, being a potent inducer of hepatic glutathione S-transferase. Eur. J. Nutr. 52, 1279–1285. doi:10.1007/s00394-012-0397-2
Abdull Razis, A. F., and Mohd Noor, N. (2013). Cruciferous vegetables: Dietary phytochemicals for cancer prevention. Asian Pac. J. Cancer Prev. 14, 1565–1570. doi:10.7314/APJCP.2013.14.3.1565
Adebayo, O. G., Wopara, I., Aduema, W., Ebo, O. T., and Umoren, E. B. (2021). Long-term consumption of Moringa oleifera-supplemented diet enhanced neurocognition, suppressed oxidative stress, acetylcholinesterase activity and neuronal degeneration in rat’s hippocampus. Drug Metab. Pers. Ther. 36, 223–231. doi:10.1515/dmpt-2020-0189
Agerbirk, N., and Olsen, C. E. (2012). Glucosinolate structures in evolution. Phytochemistry 77, 16–45. doi:10.1016/j.phytochem.2012.02.005
Alexandre, E. M. C., Moreira, S. A., Pinto, C. A., Pintado, M., and Saraiva, J. A. (2020). “Analysis of glucosinolates content in food products,” in Glucosinolates: Properties, recovery, and applications (Elsevier), 213–250. doi:10.1016/B978-0-12-816493-8.00007-X
Ali, S. S., Ahmad, N., Jamal Gilani, S., and Ali Khan, N. (2018). Isothiocyanates: A review. Res. J. Pharmacogn. 5, 71–89. doi:10.22127/RJP.2018.58511
Alqahtani, W. S., and Albasher, G. (2021). Moringa oleifera Lam. extract rescues lead-induced oxidative stress, inflammation, and apoptosis in the rat cerebral cortex. J. Food Biochem. 45, e13579. doi:10.1111/jfbc.13579
Amarakoon, D., Lee, W., Tamia, G., and Lee, S. (2023). Indole-3-Carbinol: Occurrence, health-beneficial properties, and cellular/molecular mechanisms. Annu. Rev. Food Sci. Technol. 14, 347–366. doi:10.1146/annurev-food-060721-025531
An, Y. W., Jhang, K. A., Woo, S.-Y., Kang, J. L., and Chong, Y. H. (2016). Sulforaphane exerts its anti-inflammatory effect against amyloid-β peptide via STAT-1 dephosphorylation and activation of Nrf2/HO-1 cascade in human THP-1 macrophages. Neurobiol. Aging 38, 1–10. doi:10.1016/j.neurobiolaging.2015.10.016
Andrade, S., Ramalho, M. J., Loureiro, J. A., and Pereira, M. do C. (2019). Natural compounds for Alzheimer’s disease therapy: A systematic review of preclinical and clinical studies. Int. J. Mol. Sci. 20, 2313. doi:10.3390/ijms20092313
Asif, M., Kala, C., Gilani, S. J., Imam, S. S., Mohamad, T., Naaz, F., et al. (2022). Protective effects of isothiocyanates against Alzheimer’s disease. Curr. Tradit. Med. 8, 1–10. doi:10.2174/2215083807666211109121345
Avato, P., and Argentieri, M. P. (2015). Brassicaceae: A rich source of health improving phytochemicals. Phytochem. Rev. 14, 1019–1033. doi:10.1007/s11101-015-9414-4
Barba, F. J., Nikmaram, N., Roohinejad, S., Khelfa, A., Zhu, Z., and Koubaa, M. (2016). Bioavailability of glucosinolates and their breakdown products: Impact of processing. Front. Nutr. 3, 24. doi:10.3389/fnut.2016.00024
Bennett, R. N., Mellon, F. A., and Kroon, P. A. (2004). Screening crucifer seeds as sources of specific intact glucosinolates using ion-pair high-performance liquid chromatography negative ion electrospray mass spectrometry. J. Agric. Food Chem. 52, 428–438. doi:10.1021/jf030530p
Blažević, I., and Mastelić, J. (2009). Glucosinolate degradation products and other bound and free volatiles in the leaves and roots of radish (Raphanus sativus L). Food Chem. 113, 96–102. doi:10.1016/j.foodchem.2008.07.029
Boyanapalli, S. S. S., Paredes-Gonzalez, X., Fuentes, F., Zhang, C., Guo, Y., Pung, D., et al. (2014). Nrf2 knockout attenuates the anti-inflammatory effects of phenethyl isothiocyanate and curcumin. Chem. Res. Toxicol. 27, 2036–2043. doi:10.1021/tx500234h
Brasil, F. B., de Almeida, F. J. S., Luckachaki, M. D., Dall’Oglio, E. L., and de Oliveira, M. R. (2023). The isothiocyanate sulforaphane prevents mitochondrial impairment and neuroinflammation in the human dopaminergic SH-SY5Y and in the mouse microglial BV2 cells: Role for heme oxygenase-1. Metab. Brain Dis. 38, 419–435. doi:10.1007/s11011-022-00990-x
Burčul, F., Generalić Mekinić, I., Radan, M., Rollin, P., and Blažević, I. (2018). Isothiocyanates: Cholinesterase inhibiting, antioxidant, and anti-inflammatory activity. J. Enzyme Inhib. Med. Chem. 33, 577–582. doi:10.1080/14756366.2018.1442832
Caglayan, B., Kilic, E., Dalay, A., Altunay, S., Tuzcu, M., Erten, F., et al. (2019). Allyl isothiocyanate attenuates oxidative stress and inflammation by modulating Nrf2/HO-1 and NF-κB pathways in traumatic brain injury in mice. Mol. Biol. Rep. 46, 241–250. doi:10.1007/s11033-018-4465-4
Cammisuli, D. M., Cipriani, G., and Castelnuovo, G. (2022). Technological solutions for diagnosis, management and treatment of Alzheimer’s disease-related symptoms: A structured review of the recent scientific literature. Int. J. Environ. Res. Public Health 19, 3122. doi:10.3390/ijerph19053122
Cancer, I. A. for R. on, Strategies, I. W. G., theof, E. C.-P., and Organization, W. H. (2004). Cruciferous vegetables, isothiocyanates and indoles. IARC.
Cao, Q., Tan, C.-C., Xu, W., Hu, H., Cao, X.-P., Dong, Q., et al. (2020). The prevalence of dementia: A systematic review and meta-analysis. J. Alzheimer’s Dis. 73, 1157–1166. doi:10.3233/JAD-191092
Cartea, M. E., and Velasco, P. (2008). Glucosinolates in Brassica foods: Bioavailability in food and significance for human health. Phytochem. Rev. 7, 213–229. doi:10.1007/s11101-007-9072-2
Chang, Q., Wang, G.-N., Li, Y., Zhang, L., You, C., and Zheng, Y. (2012). Oral absorption and excretion of icaritin, an aglycone and also active metabolite of prenylflavonoids from the Chinese medicine Herba Epimedii in rats. Phytomedicine 19, 1024–1028. doi:10.1016/j.phymed.2012.05.017
Chen, J., Uto, T., Tanigawa, S., Yamada-Kato, T., Fujii, M., and Hou, D.-X. (2010). Microarray-based determination of anti-inflammatory genes targeted by 6-(methylsulfinyl) hexyl isothiocyanate in macrophages. Exp. Ther. Med. 1, 33–40. doi:10.3892/etm_00000006
Chilakala, R. R., Manchikalapudi, A. L., Kumar, A., and Sunkaria, A. (2020). Sulforaphane attenuates Aβ oligomers mediated decrease in phagocytic activity of microglial cells. Neuroscience 429, 225–234. doi:10.1016/j.neuroscience.2020.01.002
Ciccone, V., Piragine, E., Gorica, E., Citi, V., Testai, L., Pagnotta, E., et al. (2022). Anti-inflammatory effect of the natural H2S-donor erucin in vascular endothelium. Int. J. Mol. Sci. 23, 15593. doi:10.3390/ijms232415593
Citi, V., Martelli, A., Testai, L., Marino, A., Breschi, M. C., and Calderone, V. (2014). Hydrogen sulfide releasing capacity of natural isothiocyanates: Is it a reliable explanation for the multiple biological effects of brassicaceae? Planta Med. 80, 610–613. doi:10.1055/s-0034-1368591
Cohen, T., Frydman-Marom, A., Rechter, M., and Gazit, E. (2006). Inhibition of amyloid fibril formation and cytotoxicity by hydroxyindole derivatives. Biochemistry 45, 4727–4735. doi:10.1021/bi051525c
Connolly, E. L., Sim, M., Travica, N., Marx, W., Beasy, G., Lynch, G. S., et al. (2021). Glucosinolates from cruciferous vegetables and their potential role in chronic disease: Investigating the preclinical and clinical evidence. Front. Pharmacol. 12, 767975. doi:10.3389/fphar.2021.767975
Craig, L. A., Hong, N. S., and McDonald, R. J. (2011). Revisiting the cholinergic hypothesis in the development of Alzheimer’s disease. Neurosci. Biobehav. Rev. 35, 1397–1409. doi:10.1016/j.neubiorev.2011.03.001
Dahran, N., Abd-Elhakim, Y. M., Mohamed, A. A.-R., Abd-Elsalam, M. M., Said, E. N., Metwally, M. M. M., et al. (2023). Palliative effect of Moringa olifera-mediated zinc oxide nanoparticles against acrylamide-induced neurotoxicity in rats. Food Chem. Toxicol. 171, 113537. doi:10.1016/j.fct.2022.113537
Dayalan Naidu, S., Suzuki, T., Yamamoto, M., Fahey, J. W., and Dinkova-Kostova, A. T. (2018). Phenethyl isothiocyanate, a dual activator of transcription factors NRF2 and HSF1. Mol. Nutr. Food Res. 62, 1700908. doi:10.1002/mnfr.201700908
De Logu, F., De Siena, G., Landini, L., Marini, M., Souza Monteiro de Araujo, D., Albanese, V., et al. (2022a). Non-neuronal TRPA1 encodes mechanical allodynia associated with neurogenic inflammation and partial nerve injury in rats. Br. J. Pharmacol. 180, 1232–1246. doi:10.1111/bph.16005
De Logu, F., Nassini, R., Hegron, A., Landini, L., Jensen, D. D., Latorre, R., et al. (2022b). Schwann cell endosome CGRP signals elicit periorbital mechanical allodynia in mice. Nat. Commun. 13, 646–719. doi:10.1038/s41467-022-28204-z
Deshmukh, P., Unni, S., Krishnappa, G., and Padmanabhan, B. (2017). The keap1–nrf2 pathway: Promising therapeutic target to counteract ROS-mediated damage in cancers and neurodegenerative diseases. Biophys. Rev. 9, 41–56. doi:10.1007/s12551-016-0244-4
Devkota, H. P. (2020). “Analysis of glucosinolates,” in Recent advances in natural products analysis (Elsevier), 651–661. doi:10.1016/B978-0-12-816455-6.00020-2
Dinkova-Kostova, A. T., and Kostov, R. V. (2012). Glucosinolates and isothiocyanates in health and disease. Trends Mol. Med. 18, 337–347. doi:10.1016/j.molmed.2012.04.003
Disbrow, E., Stokes, K. Y., Ledbetter, C., Patterson, J., Kelley, R., Pardue, S., et al. (2021). Plasma hydrogen sulfide: A biomarker of Alzheimer’s disease and related dementias. Alzheimer’s Dement. 17, 1391–1402. doi:10.1002/alz.12305
Dorszewska, J., Prendecki, M., Oczkowska, A., Dezor, M., and Kozubski, W. (2016). Molecular basis of familial and sporadic Alzheimer’s disease. Curr. Alzheimer Res. 13, 952–963. doi:10.2174/1567205013666160314150501
Ekong, M. B., Ekpo, M. M., Akpanyung, E. O., and Nwaokonko, D. U. (2017). Neuroprotective effect of Moringa oleifera leaf extract on aluminium-induced temporal cortical degeneration. Metab. Brain Dis. 32, 1437–1447. doi:10.1007/s11011-017-0011-7
Esteve, M. (2020). Mechanisms underlying biological effects of cruciferous glucosinolate-derived isothiocyanates/indoles: A focus on metabolic syndrome. Front. Nutr. 7, 111. doi:10.3389/fnut.2020.00111
Ettcheto, M., Sánchez-López, E., Gómez-Mínguez, Y., Cabrera, H., Busquets, O., Beas-Zarate, C., et al. (2018). Peripheral and central effects of memantine in a mixed preclinical mice model of obesity and familial Alzheimer’s disease. Mol. Neurobiol. 55, 7327–7339. doi:10.1007/s12035-018-0868-4
Fahey, J. W., Zalcmann, A. T., and Talalay, P. (2001). The chemical diversity and distribution of glucosinolates and isothiocyanates among plants. Phytochemistry 56, 5–51. doi:10.1016/S0031-9422(00)00316-2
Fão, L., Mota, S. I., and Rego, A. C. (2019). Shaping the Nrf2-ARE-related pathways in Alzheimer’s and Parkinson’s diseases. Ageing Res. Rev. 54, 100942. doi:10.1016/j.arr.2019.100942
Fernández, S. S. M., and Ribeiro, S. M. L. (2018). Nutrition and alzheimer disease. Clin. Geriatr. Med. 34, 677–697. doi:10.1016/j.cger.2018.06.012
Galuppo, M., Giacoppo, S., De Nicola, G. R., Iori, R., Navarra, M., Lombardo, G. E., et al. (2014). Antiinflammatory activity of glucomoringin isothiocyanate in a mouse model of experimental autoimmune encephalomyelitis. Fitoterapia 95, 160–174. doi:10.1016/j.fitote.2014.03.018
Galuppo, M., Giacoppo, S., Iori, R., De Nicola, G. R., Bramanti, P., and Mazzon, E. (2015). Administration of 4-(α-L-rhamnosyloxy)-benzyl isothiocyanate delays disease phenotype in SOD1G93A rats: A transgenic model of amyotrophic lateral sclerosis. Biomed. Res. Int. 2015, 259417–259512. doi:10.1155/2015/259417
Ganguly, R., and Guha, D. (2008). Alteration of brain monoamines and EEG wave pattern in rat model of Alzheimer’s disease and protection by Moringa oleifera. Indian J. Med. Res. 128, 744–751.
Giacoppo, S., Galuppo, M., Montaut, S., Iori, R., Rollin, P., Bramanti, P., et al. (2015). An overview on neuroprotective effects of isothiocyanates for the treatment of neurodegenerative diseases. Fitoterapia 106, 12–21. doi:10.1016/j.fitote.2015.08.001
Grande, G., Qiu, C., and Fratiglioni, L. (2020). Prevention of dementia in an ageing world: Evidence and biological rationale. Ageing Res. Rev. 64, 101045. doi:10.1016/j.arr.2020.101045
Gugliandolo, A., Giacoppo, S., Ficicchia, M., Aliquò, A., Bramanti, P., and Mazzon, E. (2018). Eruca sativa seed extract: A novel natural product able to counteract neuroinflammation. Mol. Med. Rep. 17, 6235–6244. doi:10.3892/mmr.2018.8695
Gupta, P. K., and Robinson, J. R. (2017). ). Oral controlled-release delivery. Treatise Control. drug Deliv., 255–313.
Hampel, H., Mesulam, M.-M., Cuello, A. C., Khachaturian, A. S., Vergallo, A., Farlow, M. R., et al. (2019). Revisiting the cholinergic hypothesis in Alzheimer’s disease: Emerging evidence from translational and clinical research. J. Prev. Alzheimer’s Dis. 6, 2–15. doi:10.14283/jpad.2018.43
Hannan, M. A., Kang, J.-Y., Mohibbullah, M., Hong, Y.-K., Lee, H., Choi, J.-S., et al. (2014). Moringa oleifera with promising neuronal survival and neurite outgrowth promoting potentials. J. Ethnopharmacol. 152, 142–150. doi:10.1016/j.jep.2013.12.036
Hou, T.-T., Yang, H.-Y., Wang, W., Wu, Q.-Q., Tian, Y.-R., and Jia, J.-P. (2018). Sulforaphane inhibits the generation of amyloid-β oligomer and promotes spatial learning and memory in Alzheimer’s disease (PS1V97L) transgenic mice. J. Alzheimer’s Dis. 62, 1803–1813. doi:10.3233/JAD-171110
Hoyer, S. (2004). Glucose metabolism and insulin receptor signal transduction in Alzheimer disease. Eur. J. Pharmacol. 490, 115–125. doi:10.1016/j.ejphar.2004.02.049
Huang, C., Pan, S., Yang, R., Ma, Y.-Y., Lu, X., You, Q., et al. (2022). Indole-3-carbinol selectively prevents chronic stress-induced depression-but not anxiety-like behaviors via suppressing pro-inflammatory cytokine production and oxido-nitrosative stress in the brain. Front. Pharmacol. 331, 829966. doi:10.3389/fphar.2022.829966
Huke, C. D., Taylor, L. J., Argent, S. P., and Kays, D. L. (2021). Catalyst-free hydrophosphinylation of isocyanates and isothiocyanates under low-added-solvent conditions. ACS Sustain. Chem. Eng. 9, 10704–10709. doi:10.1021/acssuschemeng.1c02907
Jaafaru, M. S., Nordin, N., Rosli, R., Shaari, K., Bako, H. Y., Noor, N. M., et al. (2019a). Prospective role of mitochondrial apoptotic pathway in mediating GMG-ITC to reduce cytotoxicity in H2O2-induced oxidative stress in differentiated SH-SY5Y cells. Biomed. Pharmacother. 119, 109445. doi:10.1016/j.biopha.2019.109445
Jaafaru, M. S., Nordin, N., Rosli, R., Shaari, K., Bako, H. Y., Saad, N., et al. (2019b). Neuroprotective effects of glucomoringin-isothiocyanate against H2O2-Induced cytotoxicity in neuroblastoma (SH-SY5Y) cells. Neurotoxicology 75, 89–104. doi:10.1016/j.neuro.2019.09.008
Jaja-Chimedza, A., Graf, B. L., Simmler, C., Kim, Y., Kuhn, P., Pauli, G. F., et al. (2017). Biochemical characterization and anti-inflammatory properties of an isothiocyanate-enriched moringa (Moringa oleifera) seed extract. PLoS One 12, e0182658. doi:10.1371/journal.pone.0182658
Jeong, J. H., Kim, J.-J., Bak, D. H., Yu, K. S., Lee, J. H., Lee, N. S., et al. (2015). Protective effects of indole-3-carbinol-loaded poly (lactic-co-glycolic acid) nanoparticles against glutamate-induced neurotoxicity. J. Nanosci. Nanotechnol. 15, 7922–7928. doi:10.1166/jnn.2015.11219
Jhang, K. A., Park, J.-S., Kim, H.-S., and Chong, Y. H. (2018). Sulforaphane rescues amyloid-β peptide-mediated decrease in MerTK expression through its anti-inflammatory effect in human THP-1 macrophages. J. Neuroinflammation 15, 75–12. doi:10.1186/s12974-018-1112-x
Jo, C., Gundemir, S., Pritchard, S., Jin, Y. N., Rahman, I., and Johnson, G. V. W. (2014). Nrf2 reduces levels of phosphorylated tau protein by inducing autophagy adaptor protein NDP52. Nat. Commun. 5, 3496–3513. doi:10.1038/ncomms4496
Kabil, O., and Banerjee, R. (2010). Redox biochemistry of hydrogen sulfide. J. Biol. Chem. 285, 21903–21907. doi:10.1074/jbc.R110.128363
Kamal, R. M., Abdull Razis, A. F., Mohd Sukri, N. S., Perimal, E. K., Ahmad, H., Patrick, R., et al. (2022). Beneficial health effects of glucosinolates-derived isothiocyanates on cardiovascular and neurodegenerative diseases. Molecules 27, 624. doi:10.3390/molecules27030624
Karanikolopoulou, S., Revelou, P.-K., Xagoraris, M., Kokotou, M. G., and Constantinou-Kokotou, V. (2021). Current methods for the extraction and analysis of isothiocyanates and indoles in cruciferous vegetables. Analytica 2, 93–120. doi:10.3390/analytica2040011
Kim, H. W., Kim, J., Kim, J., Lee, S., Choi, B.-R., Han, J.-S., et al. (2014). 3, 3′-Diindolylmethane inhibits lipopolysaccharide-induced microglial hyperactivation and attenuates brain inflammation. Toxicol. Sci. 137, 158–167. doi:10.1093/toxsci/kft240
Kim, J. (2021). Pre-clinical neuroprotective evidences and plausible mechanisms of sulforaphane in Alzheimer’s Disease. Int. J. Mol. Sci. 22, 2929. doi:10.3390/ijms22062929
Kołodziejski, D., Piekarska, A., Hanschen, F. S., Pilipczuk, T., Tietz, F., Kusznierewicz, B., et al. (2019). Relationship between conversion rate of glucosinolates to isothiocyanates/indoles and genotoxicity of individual parts of Brassica vegetables. Eur. Food Res. Technol. 245, 383–400. doi:10.1007/s00217-018-3170-9
Komiskey, H., Peffley, D., Dang, T., McCain, J., McGallagher, D., Plascencia, M., et al. (2022). Effect of sulforaphane on hyperphosphorylation of tau.
Kraft, A. D., and Harry, G. J. (2011). Features of microglia and neuroinflammation relevant to environmental exposure and neurotoxicity. Int. J. Environ. Res. Public Health 8, 2980–3018. doi:10.3390/ijerph8072980
Lashley, T., Schott, J. M., Weston, P., Murray, C. E., Wellington, H., Keshavan, A., et al. (2018). Molecular biomarkers of Alzheimer’s disease: Progress and prospects. Dis. Model. Mech. 11, dmm031781. doi:10.1242/dmm.031781
Latronico, T., Larocca, M., Milella, S., Fasano, A., Rossano, R., and Liuzzi, G. M. (2021). Neuroprotective potential of isothiocyanates in an in vitro model of neuroinflammation. Inflammopharmacology 29, 561–571. doi:10.1007/s10787-020-00772-w
Lee, C., Park, G. H., Lee, S.-R., and Jang, J.-H. (2013). Attenuation of-amyloid-induced oxidative cell death by sulforaphane via activation of NF-E2-related factor 2. Oxid. Med. Cell. Longev. 2013, 313510. doi:10.1155/2013/313510
Lee, S., Kim, J., Seo, S. G., Choi, B.-R., Han, J.-S., Lee, K. W., et al. (2014). Sulforaphane alleviates scopolamine-induced memory impairment in mice. Pharmacol. Res. 85, 23–32. doi:10.1016/j.phrs.2014.05.003
Li, S., Jin, M., Liu, L., Dang, Y., Ostaszewski, B. L., and Selkoe, D. J. (2018). Decoding the synaptic dysfunction of bioactive human AD brain soluble Aβ to inspire novel therapeutic avenues for Alzheimer’s disease. Acta Neuropathol. Commun. 6, 121–216. doi:10.1186/s40478-018-0626-x
Loewenstein, R. J. (2022). Dissociation debates: Everything you know is wrong. Dialogues Clin. Neurosci. 20, 229–242. doi:10.31887/DCNS.2018.20.3/rloewenstein
Lopez-Rodriguez, N. A., Gaytán-Martínez, M., de la Luz Reyes-Vega, M., and Loarca-Piña, G. (2020). Glucosinolates and isothiocyanates from Moringa oleifera: Chemical and biological approaches. Plant Foods Hum. Nutr. 75, 447–457. doi:10.1007/s11130-020-00851-x
Luang-In, V., Narbad, A., Nueno-Palop, C., Mithen, R., Bennett, M., and Rossiter, J. T. (2014). The metabolism of methylsulfinylalkyl-and methylthioalkyl-glucosinolates by a selection of human gut bacteria. Mol. Nutr. Food Res. 58, 875–883. doi:10.1002/mnfr.201300377
Ma, Y.-S., Hsiao, Y.-T., Lin, J.-J., Liao, C.-L., Lin, C.-C., and Chung, J.-G. (2017). Phenethyl isothiocyanate (PEITC) and benzyl isothiocyanate (BITC) inhibit human melanoma A375. S2 cell migration and invasion by affecting MAPK signaling pathway in vitro. Anticancer Res. 37, 6223–6234. doi:10.21873/anticanres.12073
Mahaman, Y. A. R., Huang, F., Wu, M., Wang, Y., Wei, Z., Bao, J., et al. (2018). Moringa oleifera alleviates homocysteine-induced Alzheimer’s disease-like pathology and cognitive impairments. J. Alzheimer’s Dis. 63, 1141–1159. doi:10.3233/JAD-180091
Mahmoud, M. S., El-Kott, A. F., AlGwaiz, H. I. M., and Fathy, S. M. (2022). Protective effect of Moringa oleifera Lam. leaf extract against oxidative stress, inflammation, depression, and apoptosis in a mouse model of hepatic encephalopathy. Environ. Sci. Pollut. Res. 29, 83783–83796. doi:10.1007/s11356-022-21453-x
Mammana, S., Gugliandolo, A., Cavalli, E., Diomede, F., Iori, R., Zappacosta, R., et al. (2019). Human gingival mesenchymal stem cells pretreated with vesicular moringin nanostructures as a new therapeutic approach in a mouse model of spinal cord injury. J. Tissue Eng. Regen. Med. 13, 1109–1121. doi:10.1002/term.2857
Martelli, A., Citi, V., Testai, L., Brogi, S., and Calderone, V. (2020). Organic isothiocyanates as hydrogen sulfide donors. Antioxidants Redox Signal 32, 110–144. doi:10.1089/ars.2019.7888
Michalska, P., Buendia, I., Duarte, P., FernandezMendivil, C., Negredo, P., Cuadrado, A., et al. (2020). Melatonin-sulforaphane hybrid ITH12674 attenuates glial response in vivo by blocking LPS binding to MD2 and receptor oligomerization. Pharmacol. Res. 152, 104597. doi:10.1016/j.phrs.2019.104597
Mithen, R. F., Dekker, M., Verkerk, R., Rabot, S., and Johnson, I. T. (2000). The nutritional significance, biosynthesis and bioavailability of glucosinolates in human foods. J. Sci. Food Agric. 80, 967–984. doi:10.1002/(SICI)1097-0010(20000515)80:7<967::AID-JSFA597>3.0.CO;2-V
Morroni, F., Sita, G., Graziosi, A., Turrini, E., Fimognari, C., Tarozzi, A., et al. (2018). Protective effects of 6-(methylsulfinyl) hexyl isothiocyanate on Aβ1-42-induced cognitive deficit, oxidative stress, inflammation, and apoptosis in mice. Int. J. Mol. Sci. 19, 2083. doi:10.3390/ijms19072083
Okubo, T., Washida, K., and Murakami, A. (2010). Phenethyl isothiocyanate suppresses nitric oxide production via inhibition of phosphoinositide 3-kinase/Akt-induced IFN-γ secretion in LPS-activated peritoneal macrophages. Mol. Nutr. Food Res. 54, 1351–1360. doi:10.1002/mnfr.200900318
Oliviero, T., Verkerk, R., and Dekker, M. (2018). Isothiocyanates from Brassica vegetables—Effects of processing, cooking, mastication, and digestion. Mol. Nutr. Food Res. 62, 1701069. doi:10.1002/mnfr.201701069
Omotoso, O. D., Olumorin, O. I., Sunday, A., Aderemi, A. S., and Ogbonna, E. (2019). Neuroprotective properties of Moringa Oleifera in cadmium and herbal alcoholic beverage induced frontal cortex damage in Wistar Rats. Ip. Indian J. Neurosci. 5, 206–213. doi:10.18231/j.ijn.2019.034
Onasanwo, S. A., Adamaigbo, V. O., Adebayo, O. G., and Eleazer, S. E. (2021). Moringa oleifera-supplemented diet protect against cortico-hippocampal neuronal degeneration in scopolamine-induced spatial memory deficit in mice: Role of oxido-inflammatory and cholinergic neurotransmission pathway. Metab. Brain Dis. 36, 2445–2460. doi:10.1007/s11011-021-00855-9
Park, H.-M., Kim, J.-A., and Kwak, M.-K. (2009). Protection against amyloid beta cytotoxicity by sulforaphane: Role of the proteasome. Arch. Pharm. Res. 32, 109–115. doi:10.1007/s12272-009-1124-2
Qin, C.-Z., Zhang, X., Wu, L.-X., Wen, C.-J., Hu, L., Lv, Q.-L., et al. (2015). Advances in molecular signaling mechanisms of β-phenethyl isothiocyanate antitumor effects. J. Agric. Food Chem. 63, 3311–3322. doi:10.1021/jf504627e
Ramawat, K. G., and Arora, J. (2021). “Medicinal plants domestication, cultivation, improvement, and alternative technologies for the production of high value therapeutics: An overview,” in Medicinal plants, sustainable development and biodiversity. Editor H. M. Ekiert (Springer Nature Switzerland AG), 28, 1–29.
Sailaja, B. S., Hassan, S., Cohen, E., Tmenova, I., Farias-Pereira, R., Verzi, M. P., et al. (2022). Moringa isothiocyanate-1 inhibits LPS-induced inflammation in mouse myoblasts and skeletal muscle. PLoS One 17, e0279370. doi:10.1371/journal.pone.0279370
Schaffert, L.-N., and Carter, W. G. (2020). Do post-translational modifications influence protein aggregation in neurodegenerative diseases: A systematic review. Brain Sci. 10, 232. doi:10.3390/brainsci10040232
Schneider, L. S. (2022). A critical review of cholinesterase inhibitors as a treatment modality in Alzheimer’s disease. Dialogues Clin. Neurosci. 2, 111–128. doi:10.31887/DCNS.2000.2.2/lschneider
Sestito, S., Pruccoli, L., Runfola, M., Citi, V., Martelli, A., Saccomanni, G., et al. (2019). Design and synthesis of H2S-donor hybrids: A new treatment for Alzheimer’s disease? Eur. J. Med. Chem. 184, 111745. doi:10.1016/j.ejmech.2019.111745
Shakour, Z. T., Shehab, N. G., Gomaa, A. S., Wessjohann, L. A., and Farag, M. A. (2022). Metabolic and biotransformation effects on dietary glucosinolates, their bioavailability, catabolism and biological effects in different organisms. Biotechnol. Adv. 54, 107784. doi:10.1016/j.biotechadv.2021.107784
Sharif, A. H., Iqbal, M., Manhoosh, B., Gholampoor, N., Ma, D., Marwah, M., et al. (2023). Hydrogen sulphide-based therapeutics for neurological conditions: Perspectives and challenges. Neurochem. Res. 48, 1981–1996. doi:10.1007/s11064-023-03887-y
Shree, B., Kumar, S., Sharma, S., and Katoch, V. (2022). Functional significance of underutilized high value cruciferous vegetables-an exotic gleam in the gloomy guise of their functional importance. South Afr. J. Bot. 145, 420–437. doi:10.1016/j.sajb.2022.02.028
Sikorska-Zimny, K., and Beneduce, L. (2021). The metabolism of glucosinolates by gut microbiota. Nutrients 13, 2750. doi:10.3390/nu13082750
Silvestro, S., Chiricosta, L., Gugliandolo, A., Iori, R., Rollin, P., Perenzoni, D., et al. (2021). The moringin/α-CD pretreatment induces neuroprotection in an in vitro model of Alzheimer’s disease: A transcriptomic study. Curr. Issues Mol. Biol. 43, 197–214. doi:10.3390/cimb43010017
Sita, G., Hrelia, P., Tarozzi, A., and Morroni, F. (2016). Isothiocyanates are promising compounds against oxidative stress, neuroinflammation and cell death that may benefit neurodegeneration in Parkinson’s disease. Int. J. Mol. Sci. 17, 1454. doi:10.3390/ijms17091454
Subedi, L., Venkatesan, R., and Kim, S. Y. (2017). Neuroprotective and anti-inflammatory activities of allyl isothiocyanate through attenuation of JNK/NF-κB/TNF-α signaling. Int. J. Mol. Sci. 18, 1423. doi:10.3390/ijms18071423
Sutalangka, C., Wattanathorn, J., Muchimapura, S., and Thukham-mee, W. (2013). Moringa oleifera mitigates memory impairment and neurodegeneration in animal model of age-related dementia. Oxid. Med. Cell. Longev. 9, 695936, doi:10.1155/2013/695936
Tabassum, R., and Jeong, N. Y. (2019). Potential for therapeutic use of hydrogen sulfide in oxidative stress-induced neurodegenerative diseases. Int. J. Med. Sci. 16, 1386–1396. doi:10.7150/ijms.36516
Tian, S., Liu, X., Lei, P., Zhang, X., and Shan, Y. (2018). Microbiota: A mediator to transform glucosinolate precursors in cruciferous vegetables to the active isothiocyanates. J. Sci. Food Agric. 98, 1255–1260. doi:10.1002/jsfa.8654
Trio, P. Z., Fujisaki, S., Tanigawa, S., Hisanaga, A., Sakao, K., and Hou, D.-X. (2016). DNA microarray highlights Nrf2-mediated neuron protection targeted by Wasabi-derived isothiocyanates in IMR-32 cells. Gene Regul. Syst. Bio 10, 73–83. GRSB-S39440. doi:10.4137/GRSB.S39440
Uto, T., Fujii, M., and Hou, D.-X. (2005). Inhibition of lipopolysaccharide-induced cyclooxygenase-2 transcription by 6-(methylsulfinyl) hexyl isothiocyanate, a chemopreventive compound from Wasabia japonica (Miq) Matsumura, in mouse macrophages. Biochem. Pharmacol. 70, 1772–1784. doi:10.1016/j.bcp.2005.09.023
Verkerk, R., Dekker, M., and Jongen, W. M. F. (1998). Glucosinolates: Natural toxicants in food, 29–53. ed by Watson D.
Verkerk, R., Schreiner, M., Krumbein, A., Ciska, E., Holst, B., Rowland, I., et al. (2009). Glucosinolates in Brassica vegetables: The influence of the food supply chain on intake, bioavailability and human health. Mol. Nutr. Food Res. 53, S219. doi:10.1002/mnfr.200800065
Wagner, A. E., Boesch-Saadatmandi, C., Dose, J., Schultheiss, G., and Rimbach, G. (2012). Anti-inflammatory potential of allyl-isothiocyanate–role of Nrf2, NF-κB and microRNA-155. J. Cell. Mol. Med. 16, 836–843. doi:10.1111/j.1582-4934.2011.01367.x
Wagner, A. E., Sturm, C., Piegholdt, S., Wolf, I. M. A., Esatbeyoglu, T., De Nicola, G. R., et al. (2015). Myrosinase-treated glucoerucin is a potent inducer of the Nrf2 target gene heme oxygenase 1—studies in cultured HT-29 cells and mice. J. Nutr. Biochem. 26, 661–666. doi:10.1016/j.jnutbio.2015.01.004
Wang, Z., Chen, Q., Wang, J., Yu, L., and Chen, L. (2020). Sulforaphane mitigates LPS-induced neuroinflammation through modulation of Cezanne/NF-κB signalling. Life Sci. 262, 118519. doi:10.1016/j.lfs.2020.118519
Xia, Y., Kong, L., Yao, Y., Jiao, Y., Song, J., Tao, Z., et al. (2015). Osthole confers neuroprotection against cortical stab wound injury and attenuates secondary brain injury. J. Neuroinflammation 12, 155–211. doi:10.1186/s12974-015-0373-x
Yang, W., Liu, Y., Xu, Q.-Q., Xian, Y.-F., and Lin, Z.-X. (2020). Sulforaphene ameliorates neuroinflammation and hyperphosphorylated tau protein via regulating the PI3K/Akt/GSK-3β pathway in experimental models of Alzheimer’s disease. Oxid. Med. Cell. Longev. 2020, 4754195. doi:10.1155/2020/4754195
Yang, W., Xu, Q.-Q., Yuan, Q., Xian, Y.-F., and Lin, Z.-X. (2023). Sulforaphene, a CDK5 Inhibitor, attenuates cognitive deficits in a transgenic mouse model of Alzheimer’s disease via reducing Aβ Deposition, tau hyperphosphorylation and synaptic dysfunction. Int. Immunopharmacol. 114, 109504. doi:10.1016/j.intimp.2022.109504
Yehuda, H., Soroka, Y., Zlotkin-Frušić, M., Gilhar, A., Milner, Y., and Tamir, S. (2012). Isothiocyanates inhibit psoriasis-related proinflammatory factors in human skin. Inflamm. Res. 61, 735–742. doi:10.1007/s00011-012-0465-3
Zhang, J., Zhang, R., Zhan, Z., Li, X., Zhou, F., Xing, A., et al. (2017). Beneficial effects of sulforaphane treatment in Alzheimer’s disease may be mediated through reduced HDAC1/3 and increased P75NTR expression. Front. Aging Neurosci. 9, 121. doi:10.3389/fnagi.2017.00121
Zhang, L., Chen, C., Mak, M. S. H., Lu, J., Wu, Z., Chen, Q., et al. (2020). Advance of sporadic Alzheimer’s disease animal models. Med. Res. Rev. 40, 431–458. doi:10.1002/med.21624
Zhang, R., Miao, Q.-W., Zhu, C.-X., Zhao, Y., Liu, L., Yang, J., et al. (2015). Sulforaphane ameliorates neurobehavioral deficits and protects the brain from amyloid β deposits and peroxidation in mice with Alzheimer-like lesions. Am. J. Alzheimer’s Dis. Other Dementias® 30, 183–191. doi:10.1177/1533317514542645
Zhang, R., Zhang, J., Fang, L., Li, X., Zhao, Y., Shi, W., et al. (2014). Neuroprotective effects of sulforaphane on cholinergic neurons in mice with Alzheimer’s disease-like lesions. Int. J. Mol. Sci. 15, 14396–14410. doi:10.3390/ijms150814396
Zhang, S., and Zhang, S. (2017). Oral absorption, distribution, metabolism, and excretion of icaritin in rats by Q-TOF and UHPLC–MS/MS. Drug Test. Anal. 9, 1604–1610. doi:10.1002/dta.2188
Zhao, F., Zhang, J., and Chang, N. (2018). Epigenetic modification of Nrf2 by sulforaphane increases the antioxidative and anti-inflammatory capacity in a cellular model of Alzheimer’s disease. Eur. J. Pharmacol. 824, 1–10. doi:10.1016/j.ejphar.2018.01.046
Keywords: Alzheimer’s disease, isothiocyanates, amyloid β, phosphorylated tau, glucosinolates
Citation: Khan F, Joshi A, Devkota HP, Subramaniyan V, Kumarasamy V and Arora J (2023) Dietary glucosinolates derived isothiocyanates: chemical properties, metabolism and their potential in prevention of Alzheimer’s disease. Front. Pharmacol. 14:1214881. doi: 10.3389/fphar.2023.1214881
Received: 14 May 2023; Accepted: 04 July 2023;
Published: 17 July 2023.
Edited by:
Rajesh Chandra Misra, John Innes Centre, United KingdomReviewed by:
Ahmad Faizal Abdull Razis, University Putra Malaysia, MalaysiaCopyright © 2023 Khan, Joshi, Devkota, Subramaniyan, Kumarasamy and Arora. This is an open-access article distributed under the terms of the Creative Commons Attribution License (CC BY). The use, distribution or reproduction in other forums is permitted, provided the original author(s) and the copyright owner(s) are credited and that the original publication in this journal is cited, in accordance with accepted academic practice. No use, distribution or reproduction is permitted which does not comply with these terms.
*Correspondence: Jaya Arora, amF5YTg5MEBnbWFpbC5jb20=, amF5YWFyb3JhQG1sc3UuYWMuaW4=; Vinoth Kumarasamy, dmlub3RoQHVrbS5lZHUubXk=
†Present address: Vetriselvan Subramaniyan, Jeffrey Cheah School of Medicine and Health Sciences, Monash University, Petaling Jaya, Selangor, Malaysia
Disclaimer: All claims expressed in this article are solely those of the authors and do not necessarily represent those of their affiliated organizations, or those of the publisher, the editors and the reviewers. Any product that may be evaluated in this article or claim that may be made by its manufacturer is not guaranteed or endorsed by the publisher.
Research integrity at Frontiers
Learn more about the work of our research integrity team to safeguard the quality of each article we publish.