- 1Arthritis and Clinical Immunology Program, Oklahoma Medical Research Foundation, Oklahoma City, OK, United States
- 2Department of Microbiology and Immunology, University of Oklahoma Health Sciences Center, Oklahoma City, OK, United States
Epidemiological studies have revealed sex differences in the incidence and morbidity of respiratory virus infection in the human population, and often these observations are correlated with sex differences in the quality or magnitude of the immune response. Sex differences in immunity and morbidity also are observed in animal models of respiratory virus infection, suggesting differential dominance of specific immune mechanisms. Emerging research shows intrinsic sex differences in immune cell transcriptomes, epigenomes, and proteomes that may regulate human immunity when challenged by viral infection. Here, we highlight recent research into the role(s) of sex steroids and X chromosome complement in immune cells and describe how these findings provide insight into immunity during respiratory virus infection. We focus on the regulation of innate and adaptive immune cells by receptors for androgen and estrogens, as well as genes with a propensity to escape X chromosome inactivation. A deeper mechanistic knowledge of these pathways will help us to understand the often significant sex differences in immunity to endemic or pandemic respiratory pathogens such as influenza viruses, respiratory syncytial viruses and pathogenic coronaviruses.
Introduction
Respiratory virus infections present a global health burden. During the 2019–2020 influenza season, nearly 35 million cases and over 20,000 deaths occurred in the United States alone, with approximately $11.2 billion spent on healthcare (CDC, 2021). The COVID-19 pandemic continues to increase the numbers of respiratory infection cases. Epidemiological studies have shown human sex differences in infectious disease incidence, immune response quality, and treatment response, and in immunity upon vaccination against pathogens [reviewed in (Ursin and Klein, 2021; Wilkinson et al., 2022)]. While differential outcomes in respiratory immunity may be explained by cultural factors such as gender disparate workplaces, smoking history, or social habits, sex differences in immunity and morbidity also are observed in animal models of respiratory infection [reviewed in (Klein et al., 2012; Kadel and Kovats, 2018)]. These findings with rodent models suggest that many sex differences arise through biological mechanisms influenced by sex chromosome complement and/or sex steroid levels. Gender and sex differences in morbidity and/or immunity often present after the onset of sexual maturity, suggesting a role for higher levels of estrogens or androgens (Jacobsen and Klein, 2021). Investigation of pathways through which divergent sex steroid levels or sex steroid receptor activity influence overall morbidity and the immune cell-specific response to infectious disease has led to important new insights as detailed below. In addition, emerging research has shown that immunity is regulated by sex chromosome complement and the gene dosage effect resulting from escape of specific genes from X chromosome inactivation (Jiwrajka and Anguera, 2022). Mechanistically, the pathways downstream of sex steroid receptor activity may work in tandem with sex chromosome complement to influence the immune response to respiratory pathogens. Investigation of these pathways will increase our understanding of infectious disease pathogenesis and inform better treatment options based on biological sex and gender Box 1.
BOX 1 Sex and gender.
Sex and gender are distinct terms. While sex has historically been considered a strict binary variable, new information reveals that manifestation of biological sex is influenced by chromosomal complement, genetics, including alleles leading to disorders of sex development (DSDs), sex steroid concentrations and external/internal anatomy [discussed in (Ainsworth, 2015; Jacobsen and Klein, 2021)]. Gender is a social construct that does not exist in a binary, and its external manifestation may include manipulation of sex hormone levels, particularly by transgender and gender non-conforming individuals. In humans, sex steroid levels may vary greatly with health status, age (including milestones such as puberty, pregnancy, or menopause and andropause), and/or direct manipulation of levels through contraceptives, hormone replacement therapy, and/or gender affirming hormone treatments. Failure to measure and report these sex steroid levels in studies of humans inhibits our understanding of the mechanistic basis of observed sex differences in immune cell numbers or activation pathways. Thus, studies of human immunity will benefit from ascertainment of the gender identity, assigned sex at birth, sex chromosome complement, and sex steroid level of individuals, and integration of these variables into analyses of immune parameters and disease outcomes. Encouragingly, an increasing number of human studies do report gender and/or sex as a non-binary variable or include measurements of circulating sex steroid levels; for example (Furman et al., 2014; Ter Horst et al., 2016; Webb et al., 2018; Robinson et al., 2022). Here, unless otherwise noted, we refer to sex differences when discussing human population studies, as most studies report participants as male or female using definitions set by the study authors.
From the clinic: Epidemiological evidence for gender differences in the incidence and pathogenesis of respiratory infection
The incidence, morbidity and immune response to acute respiratory virus infection vary with sex and age. For example, sex differences in influenza incidence and severity depend on the age group, with increased morbidity and mortality associated with young (0–20 years) and elderly (>69 years) males, yet associated with females during reproductive age (20–49 years) (Kumar et al., 2009; WHO, 2010; Eshima et al., 2011; Hoffmann et al., 2015; Giurgea et al., 2022). Consistent with this, another study showed that the frequency of early life hospitalization for severe lower respiratory tract infection or bronchiolitis was elevated in male compared to female children (Regis et al., 2021). Infant and older adult males show increased incidence and/or severity of respiratory syncytial virus infection of the lower respiratory tract (De Jacobis et al., 2020; Orimadegun et al., 2020; Branche et al., 2022; Vila et al., 2022).
Epidemiological data show that males 45–79 years of age suffered greater morbidity and mortality when infected with pathogenic coronaviruses, such as SARS-CoV-1, MERS, and SARS-CoV-2 (Karlberg et al., 2004; Alghamdi et al., 2014; Gebhard et al., 2020; Alwani et al., 2021; Gomez et al., 2021; Scully et al., 2021). During the COVID-19 pandemic, reports of increased morbidity and mortality in elderly males have fueled new research in sex differences in immunity to infectious pathogens. Recent reports detail sex differences in immune system cells and related proteins in COVID-19 patients, and in some cases, these immune differences have been linked to sex-specific outcomes in morbidity or mortality (Takahashi et al., 2020; Hou et al., 2021; Ren et al., 2021; Butler-Laporte et al., 2022).
An open question is the extent to which biological sex (with inherent sex steroid levels and chromosome complement) shapes an individual’s intrinsic capacity for immune responses, and several recent studies provide evidence for this idea. Identification of innate immune endotypes predicting antibody responses through a pan-vaccine analysis revealed that biological sex and age explained small fractions of the variance in transcriptomic data collected (Fourati et al., 2022), suggesting that stratification of these types of data by biological sex may elucidate sex-divergent pathways in the human population. A study of transcriptional, epigenetic, and cellular changes in the human immune system revealed a sexual dimorphism in the profile of aging in peripheral blood mononuclear cells (PBMCs) (Marquez et al., 2020). The data, obtained using RNA-seq, ATAC-seq, and flow cytometry analyses of healthy adult men and women aged 22–93, showed an epigenomic signature of aging characterized by declining naïve T-cell functions and increased monocyte and cytotoxic lymphocyte functions. Genomic differences between sexes increased after age 65, with men showing acceleration of the aging signature, including higher innate and pro-inflammatory activity and reduced adaptive activity. These findings have implications for the quality of antiviral responses and may help to explain the increased morbidity often observed in elderly men. Another study showed that healthy younger (< age 50) males and elderly (> age 60) individuals of both sexes showed differences in immune cell proportions in blood and levels of circulating mediators that tend to correlate with the profile observed in severe COVID-19, suggesting both sex and age dependent immune variables that increase susceptibility to severe infectious disease (Kilic et al., 2021). Characterization of whole-genome autosomal DNA methylation in men and women aged 32–81 showed substantial differences that correlated with differential gene expression, thus defining sex-specific DNA methylation patterns that likely mechanistically underpin sex differences in immunity (Singmann et al., 2015). These findings are consistent with sex differences in inflammation and trained immunity upon BCG vaccination (Koeken et al., 2020).
Going forward, clinical data disaggregated by gender, sex, and age will inform our understanding of how biological sex and gender influence the incidence and pathogenesis of infectious disease. A barrier to studying human responses to infectious agents is the frequent absence of early ascertainment and sampling. For more common outbreaks, such as seasonal influenza, individuals often do not seek treatment for mild disease, which skews the sex outcome data towards more severe cases and hospitalizations. In the COVID-19 pandemic, early detection and reporting of SARS-CoV-2 infections has increased our understanding of the course of the disease, as well as how sex and gender may influence antiviral immunity and pathogenesis.
Sex steroid hormones and their receptors
Endogenous estrogens include estrone (E1), 17-β-estradiol (E2), and estriol (E3), with E2 predominant in adult females and males, albeit at distinct levels, and E3 elevated in pregnancy. Testosterone is synthesized in males and females and converted by 5α-reductase to the physiologically active dihydrotestosterone or by aromatase to E2 (Sathish et al., 2015). Sex steroids are synthesized in the gonads and adrenal cortex as well as peripheral tissues such as fat or liver (Labrie et al., 2005; Sathish et al., 2015; Huang et al., 2021). Activated lung macrophages expressing aromatase may regulate local E2 levels, but more information is needed about how this is impacted by respiratory infection (Sathish et al., 2015). In humans, levels of sex steroids vary with age (puberty, menopause, andropause) and/or due to external manipulation such as by contraceptives and/or other exogenous hormone therapy. While the major sex steroid surge is at puberty, neonatal males are exposed to high levels of testosterone in a minipuberty stage (Quigley, 2002; Renault et al., 2020). Furthermore, infection or inflammation can temporarily alter levels of androgens and estrogens (Robinson et al., 2011b; Vom Steeg et al., 2019; Tuku et al., 2020). Endocrine disrupting chemicals (EDCs), including phenols, parabens, and phthalates, also have been reported to modulate innate and adaptive immune function, although precise effects are difficult to ascertain since EDCs bioaccumulate over time (Popescu et al., 2021). EDCs are often partial estrogen receptor (ER) agonists that may modulate ER-mediated transcriptional or rapid signaling pathways. Thus, EDCs may have greater effects in females due to their to competition with endogenous estrogens for ER binding.
The sex steroid receptors for estrogens (ERα encoded by Esr1 and ERβ encoded by Esr2) and androgen (AR encoded by Ar) act as ligand-dependent transcription factors. Upon binding ligand in the cytoplasm, the receptor/ligand complex translocates to the nucleus, and, guided by the presence of pioneer factors such as FOXA1, binds to specific androgen or estrogen response elements (ARE or ERE, respectively) located at target genes. Once bound, recruitment of co-activators and/or repressors allows for modulation of gene transcription through epigenetic changes, such as modification of histone methylation or acetylation, leading to chromatin remodeling (Mann et al., 2011; Pihlajamaa et al., 2015; Liu et al., 2017). The expression of androgen or estrogen receptor RNA or protein on various immune cell types is summarized in (Kadel and Kovats, 2018) and is available in the Immunological Genome Project [Immgen.org] (Heng et al., 2008). For example, macrophages generally express Esr1 and Ar, while type 2 innate lymphocytes primarily express Ar. Levels of AR or ER expression may increase or decrease with cellular activation, as shown for AR in T cells (Guan et al., 2022). Therefore, the magnitude and/or complement of ER/AR expression on resident or infiltrating immune cells in the lung is likely to regulate their patterns of gene expression and influence functional responses. Furthermore, ER or AR signaling in hematopoietic progenitors may set up epigenetic patterns that are preserved in descendent immune cells (Igarashi et al., 2001; Carreras et al., 2008). Murine models to study mechanisms underlying sex differences are described in Box 2.
Sex steroids influence the course of disease through modulation of the overall inflammatory environment and through direct regulation of immune cells. Androgens are largely considered anti-inflammatory (Gilliver, 2010; Cutolo and Straub, 2020), while estrogens, such as E2, elicit both pro- and anti-inflammatory effects, depending on levels of sex steroids in vivo or in cultured cell experiments (Kovats, 2015; Cutolo and Straub, 2020). For example, murine gonadectomy with or without hormone replacement prior to influenza A virus (IAV) infection has shown that testosterone is protective in sublethal infections (Vermillion et al., 2018b; Vom Steeg et al., 2020). High levels of E2 in female mice were protective against lethal infection, which was associated with reduced levels of pro-inflammatory cytokines (Robinson et al., 2011b). Estrus-diestrus levels of E2 promote inflammatory DC differentiation by upregulating IRF4 in myeloid progenitors (Carreras et al., 2010). However, despite these advances in our understanding, differing levels of sex steroids in the human population have not been conclusively linked with infection outcome in the COVID-19 pandemic [reviewed in (Lott et al., 2022)].
Biological sex also may regulate other variables influencing pulmonary immunity. Sex differences in lung architecture secondary to sex steroid levels may contribute to differential immunity to respiratory virus infection (Carey et al., 2007). Sex-specific expression levels of viral entry receptors, such as ACE2 or TMPRSS2 for SARS-CoV-2, may impact the initiation or magnitude of infection (Lott et al., 2022).
Incomplete and variable X chromosome inactivation (XCI)
X chromosome inactivation (XCI) occurs early in embryonic development to compensate for the gene dosage imbalance resulting from the additional X chromosome(s) in females (Souyris et al., 2019; Jiwrajka and Anguera, 2022). Once XCI is initiated, X-inactivation specific transcript (XIST/Xist), a long non-coding RNA, is upregulated on the randomly chosen, future inactive X chromosome (Xi). The transcribed XIST/Xist RNA accumulates and condenses on the X chromosome, recruiting other complexes to epigenetically silence the chromosome. XCI is not permanent, and XIST must be continuously transcribed to maintain the inactivated state. However, not all genes on the Xi are silenced, as an estimated 15%–20% of genes on the X chromosome escape inactivation, with an additional 10%–12% displaying variable escape between individuals (Wang et al., 2016; Syrett and Anguera, 2019).
The X chromosome has the highest density of immune response genes, and many are known to escape XCI in humans or mice, such as TLR7, CXCR3, CD40LG, BTK, IRAK1, NEMO and CXORF21 (Wang et al., 2016; Souyris et al., 2018; Harris et al., 2019; Odhams et al., 2019). Although escape from XCI leads to biallelic expression of these genes, thus altering the gene-dose effect, it is not the only factor that contributes to altered expression of X-linked immune genes. Maintenance of the XIST RNA is variable during activation of lymphocyte populations (Wang et al., 2016). In naïve lymphocytes, the Xi is partially reactivated via decreased maintenance of the XIST RNA, and this phenotype changes to full inactivation upon lymphocyte interaction with antigen and subsequent functional activation. This transition from partial reactivation to fully inactive contributes to the differences in biallelic gene expression observed in naïve versus activated lymphocytes. While the contributions of X chromosome complement (including aneuploidy), XCI, and escape from XCI have been primarily studied in the context of autoimmunity, these findings have implications for antiviral immunity (Abramowitz et al., 2014).
BOX 2 Murine models to study mechanisms underlying sex differences.
Murine models to study the influence of sex steroids range from simple physiological manipulations to global or conditional disruption of Esr or Ar genes. Gonadectomy with or without hormone replacement is often used to study the role of sex steroids in infectious disease outcome and immune responses [for example (Laffont et al., 2014; Vom Steeg et al., 2016; Laffont et al., 2017; Vermillion et al., 2018a)]. Mice with global Esr1 deficiency have been used to investigate the role of ERα activity; however, these data are often confounded by the abnormally high levels of testosterone in both female and male Esr1−/− mice, as well as high levels of estradiol in female mice (Sims et al., 2002). ARTfm (testicular feminization) mice, with a spontaneous mutation in the Ar gene that leads to androgen insensitivity, have been used to understand how a global absence of AR activity modulates immune responses (He et al., 1991); yet a potential caveat to such studies is that Tfm mice have low levels of serum testosterone (Jones et al., 2003), which may lead to changes in estradiol levels. While these approaches increase understanding of the systemic impact of sex steroids, they do not identify cell intrinsic effects of ER or AR activity. Models that delete sex steroid receptor genes in specific immune cell types have elucidated ER or AR mediated cell intrinsic mechanisms without disruption of systemic hormone levels or synthesis pathways (Griesbeck et al., 2015; Casali et al., 2020; Ejima et al., 2022). A unique mouse model that enables assessment of the roles of sex chromosomes and sex steroids separately is the four core genotypes (FCG) model. In FCG mice, the testis-determining Sry gene is located on an autosome, so that XX and XY mice can either be gonadally male (express SRY) or female (do not express SRY). With the four genotypes available (XXF, XYF, XXM, XYM), comparison of these mice determines if sex differences are a result of gonads/sex steroids, sex chromosomes, or a combination of both (Arnold and Chen, 2009; Robinson et al., 2011a).
Sex differences in innate antiviral immunity
Upon infection with a single-stranded RNA (ssRNA) virus, such as respiratory syncytial virus (RSV), influenza A or B virus (IAV, IBV), or SARS-CoV-2, cells within the respiratory tract initiate the immune response by producing antiviral molecules and proinflammatory cytokines (Mettelman et al., 2022). Type I interferons (IFN-I) are produced after ssRNA recognition and signaling via endosomal TLR7 and other cytosolic nucleic acid sensors, such as MDA5 (Diebold et al., 2004; Okude et al., 2020). IFN-I stimulates the transcription of numerous genes involved in both viral clearance and enhancement of the innate immune response (Schoggins and Rice, 2011). IFN-I is initially produced by pulmonary epithelial cells as well as lung resident macrophages and conventional dendritic cells (cDCs), which also secrete chemotactic molecules to quickly recruit other innate immune cells from blood, such as neutrophils, monocytes, natural killer (NK) cells, and plasmacytoid dendritic cells (pDCs) (Figure 1, Table 1) (Mettelman et al., 2022).
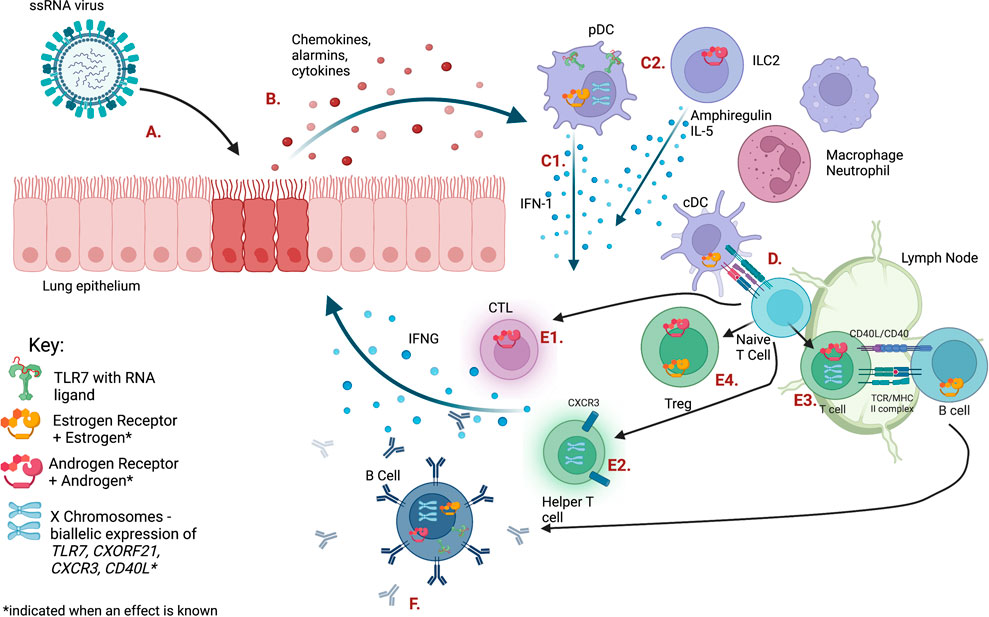
FIGURE 1. The immune response to ssRNA viral infection is regulated by sex steroid receptors and gene dosage on the X chromosome. Letters indicating each cell type or pathway are defined in Table 1. Figure created with BioRender.com.
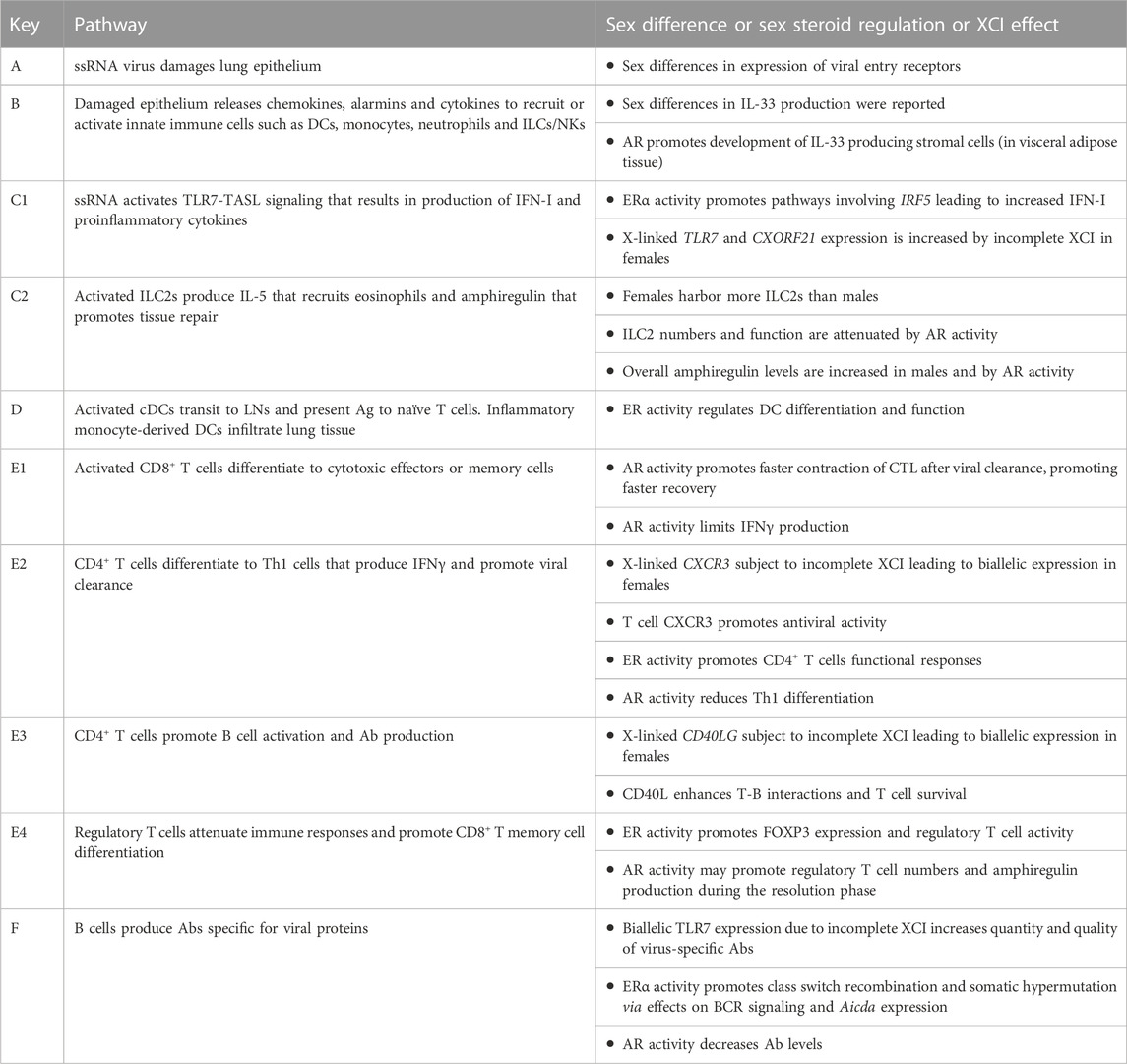
TABLE 1. Pathways shown in Figure 1.
Recent clinical studies show that females have an increased propensity to generate robust antiviral responses involving IFN-I. Stimulation of PBMCs from male and female teenagers with live virus, or TLR7 or TLR9 stimuli, showed that females produced higher levels of IFN-I, IFNγ and IFN-induced chemokines compared to males (Regis et al., 2021). A recent report on sex differences in SARS-CoV-2 infection in young military recruits followed longitudinally showed that females had higher pre- and post-infection expression of antiviral interferon-stimulated genes (ISGs) (Sauerwald et al., 2022), which correlated with increased illness symptoms, yet reduced viral load. A heightened state of antiviral innate immunity prior to infection in females is consistent with our understanding of sex-specific mechanisms regulating IFN-I pathways, as outlined below.
A body of evidence shows that estradiol acting via ERα promotes the production of IFN-I, often by regulation of genes in innate sensing pathways such as TLR8, Unc93b1, Aim2, Trim21 or Irf5, whose activity leads to the induction of the Ifna or Ifnb genes [reviewed in (Kovats, 2015)]. X linked genes, such as TLR7, that escape dosage compensation also contribute to increased IFN-I production in female cells. These mechanisms lead to sex differences in production of IFN-I or IFN-responsive pathways (Figure 2), which has important implications for antiviral responses, as recently reviewed (Pujantell and Altfeld, 2022).
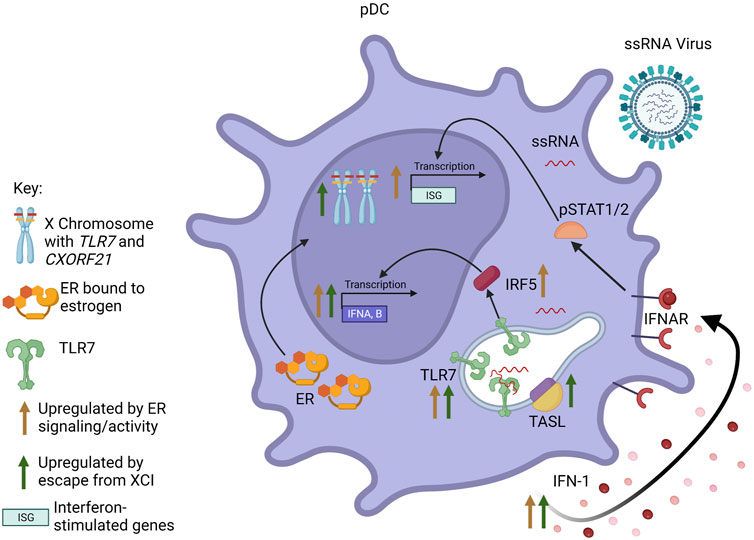
FIGURE 2. Interaction between sex steroid receptors and escape from XCI in pDCs. Signaling through ER results in increased pDC responsiveness to TLR7 signaling and TLR7-mediated IFN-I production. Additionally, ER activity increases transcription of IFNA/B and interferon-responsive genes (ISGs), both through increased production of IFN-I leading to signaling through the IFNAR, as well as increased expression of IRF5. Escape from XCI by TLR7 and CXORF21 results in biallelic transcription of the genes. Increased levels of TLR7 and TASL (the protein encoded by CXORF21) also result in increased transcription of IFNA/B and increased production of IFN-I. Figure created with BioRender.com.
Human and murine pDCs express high levels of both ESR1 and ESR2 (Phiel et al., 2005; Laffont et al., 2014). pDCs rapidly produce significant amounts of IFN-I in response to ssRNA viruses. Mouse models show that estrogens enhance the responsiveness of pDCs to TLR7 and TLR9 signaling, and treatment of postmenopausal women with estradiol restored cytokine production specifically in pDCs, indicating a cell-specific mechanism (Seillet et al., 2012). A later study using mice reconstituted with human CD34+ cells from cord blood showed this enhancement of TLR7-induced IFN-I production to be dependent on estrogen in the host, regardless of the sex of the donor, although X chromosome dosage independently contributed to the sex bias (Laffont et al., 2014). Esr1 deficiency restricted to either the hematopoietic compartment or DCs in mice revealed that the enhanced TLR7 signaling in female pDCs is likely due to direct regulation of IRF5 expression by ERα (Griesbeck et al., 2015). Increased basal IRF5 expression perpetuated by ERα signaling in pDCs, and perhaps other cells, may contribute to the elevated IRF5-dependent IFN-I production observed in females compared to males.
The sex differences in pDCs also are influenced by sex chromosome complement (Souyris et al., 2018). TLR7 is encoded on the X chromosome and is confirmed to escape XCI in immune cells, including monocytes and pDCs, from both female (46, XX) and Klinefelter syndrome (KS) male (47, XXY) individuals. Stimulated PBMCs from women and KS men produced similar amounts of TLR7 mRNA, which was greater than that produced by PBMCs from (46, XY) men and (45, XO) Turner syndrome (TS) women (Sarmiento et al., 2019). Furthermore, within PBMCs treated with a TLR7 agonist, pDCs from (46, XX) women and (46, XX) transgender men produced more type I IFN and had higher expression of CD86 (a surface marker of pDC activation) than (46, XY) men and (46, XY) transgender women, indicating the sex difference seen was more dependent on sex chromosome complement than sex steroid concentration (Webb et al., 2018). A recent study of COVID-19 patients showed that while TLR7 is expressed similarly in non-ICU patients of both sexes, TLR7 expression in blood cells is significantly downregulated in male patients admitted to the ICU as compared to female patients (Gomez-Carballa et al., 2022). Additionally, analysis of DNA methylation patterns on the TLR7 gene revealed four differentially methylated positions that were significantly different between men with severe and mild COVID-19, whereas there were no significant TLR7 methylation differences between females. The reduced TLR7 expression in males with severe disease suggests that these individuals had decreased early antiviral responses.
As IFN-I is critical for the initiation of the antiviral immune response, sex divergent production kinetics could lead to differences in disease outcome. Studies using mouse models of SARS-CoV-1 indicate that a delayed IFN-I response contributes to immunopathology, as the initial paucity of IFN-I and increased viral load leads to the recruitment of inflammatory macrophage-monocytes (IMM) and neutrophils into the lungs of infected mice (Channappanavar et al., 2016). In both MERS-CoV and IAV infections, the timing of the IFN-I response is vital to viral clearance, as early treatment with either rIFNβ or rIFNɑ provided total protection, while delayed treatment after the peak of viral replication exacerbated disease (Davidson et al., 2016; Channappanavar et al., 2019). This scenario also likely occurs upon SARS-CoV-2 infection in humans (Sette and Crotty, 2021). In the murine model of sublethal SARS-CoV-1 infection, males were more likely to show significant morbidity and mortality compared to females, particularly when older (8–20 months) (Channappanavar et al., 2017). While viral titers were higher in males, levels of Ifnb RNA were comparable. However, males showed increased levels of proinflammatory mediators (CCL2, CXCL1, IL-6) and lung infiltration of IMMs and neutrophils, suggesting a more exuberant inflammatory response and perhaps altered kinetics of IFN-I production or accumulation. Ovariectomy and the ER antagonist ICI182,780 decreased survival. These experiments showed that ER signaling protects the female mice during infection by decreasing IMM recruitment and viral load. In contrast, orchiectomy, or treatment with the AR antagonist flutamide did not alter morbidity or mortality in males.
Sex differences in lymphocyte mediated immunity
Lymphocyte immunity involves innate lymphocytes producing cytokines or other cytotoxic mediators (e.g., ILCs, NK or MAIT cells), or B and T cells implementing antigen-specific adaptive immunity. After acquiring viral antigens at the site of viral infection, DCs migrate to the draining lymph nodes where they present antigen to naïve T cells (Mettelman et al., 2022). Naïve T cells differentiate into cytotoxic (CD8+) or helper (CD4+) subsets based on peptide recognition and/or environmental cytokines. During respiratory viral infections, CD8+ cytotoxic T cells promote viral clearance through cytokine release (IFN𝛄, TNFα, IL-2), degranulation, and contact-dependent apoptosis of infected cells. CD4+ T helper and regulatory cells release cytokines that recruit innate immune cells, regulate CD8+ T cell activity, suppress the inflammatory environment, and promote B cell differentiation and antibody production. As T cell responses are vital to the immune response, dysregulation in numbers and/or function can negatively impact disease outcome.
Adaptive T and B cell responses in COVID-19 have been extensively reviewed elsewhere, for example in (Sette and Crotty, 2021). The majority of studies on T cells in COVID-19 patients did not report significant sex differences in the extent of T cell activation or numbers of antigen-specific T cells; however, a few studies described here did define differences that could be followed up. Among COVID-19 patients, males harbored a lower CD4+:CD8+ T cell ratio (Zhao et al., 2021). A decrease in activated T cells was correlated to increased age and mortality in male, but not female, patients with severe disease (Takahashi et al., 2020; Zhao et al., 2021). To date T cell epitope mapping studies have not revealed sex differences in epitope selection (Sette and Crotty, 2021).
Sex differences in the total number and activity of mucosal-associated invariant T (MAIT) cells also correlate with COVID-19 severity of infection (Yu et al., 2021). MAIT cells respond to viral infection through the release of IFNγ, TNFα, and the cytotoxic molecules perforin and granzyme B. Analyses of T cell populations of COVID-19 patients revealed that female patients had a lower proportion of MAIT cells in the blood, but higher numbers in the bronchoalveolar lavage fluid, indicating that MAIT cells may better extravasate to the pulmonary site of infection in females. MAIT cells in females showed a more immunologically active transcriptome, while MAIT cells in males showed an exhausted, pro-apoptotic phenotype, possibly contributing to the increase in immunopathology observed in males.
Sex steroids acting via ER or AR elicit both direct and indirect effects on CD4+ and CD8+ T cell function in diverse models of infection, autoimmunity and cancer. IAV infection of gonadectomized males showed that testosterone causes earlier contraction of IAV-specific IFN𝛄+CD8+ T cells in the lung after viral clearance, thereby reducing the overall inflammatory environment and increasing the rate of recovery, although this has not yet been linked to T cell intrinsic AR expression (Vom Steeg et al., 2020). Testosterone exposure led to direct binding of AR to the phosphatase gene Ptpn1, and the resulting increased transcription of Ptpn1 led to inhibition of IL-12 induced STAT4 phosphorylation and Th1 differentiation (Kissick et al., 2014). These studies are consistent with new reports showing that AR activity limits T cell effector function and promotes T cell exhaustion in solid tumors. AR bound directly to the Ifng gene, and blockade of AR activity or deletion of Ar in T cells led to increased IFN𝛄 production by CD8+ T cells (Guan et al., 2022; Kwon et al., 2022). Thus, AR activity may limit functional responses of activated antiviral T cells.
In contrast, multiple reports show that ER activity promotes T cell functional responses. ERα action in T cells induces their activation and proliferation in an autoimmune colitis model (Mohammad et al., 2018). Disruption of ERα signaling in a globally deficient Esr1−/− murine lupus model led to attenuated T cell activation, loss of spontaneous germinal centers, and loss of anti-chromatin autoantibodies, suggesting ERα regulates lymphocyte activation in this model, although a role for elevated testosterone in the Esr1−/− mice cannot be excluded (Graham et al., 2020). Healthy PBMCs from women expressed higher levels (compared to men) of the CD2 gene, and CD2 expression was upregulated by E2 exposure in vitro (Fernandez Lahore et al., 2021). ER-mediated regulation of the costimulatory molecule CD2 governed T cell activation in murine autoimmune models, in which female specific differences in CD2 expression on CD4+ T cells were attributed to the action of E2 and the binding of ERα to a polymorphic estrogen receptor binding site near the Cd2 gene (Fernandez Lahore et al., 2021). Sex differences in the capacity of human and murine CD4+ T cells to proliferate and produce IFNγ upon TCR stimulation was linked to levels of PPARα, which represses NF-κB and c-JUN important for T cell activation (Dunn et al., 2007; Zhang et al., 2012). The increased capacity of female CD4+ T cells to produce IFNγ was associated with lower levels of PPARα and elevated levels of PPARγ compared to male CD4+ T cells. In males, endogenous androgens increased PPARα and reduced PPARγ levels in CD4+ T cells, suggesting that sex differences in CD4+ T cell functional responses are regulated by sex hormones in vivo.
Biallelic expression of X-linked genes that escape XCI also impacts T cell function. Cxcr3 is located on the X chromosome, and during inflammation is upregulated on macrophages, dendritic cells, and CD8+ and CD4+ T cells, promoting their migration to sites of infection (Oghumu et al., 2019). The Cxcr3 gene escapes XCI, and biallelic expression of Cxcr3 enhances the Th1 anti-viral response and is associated with increased CD69 expression and production of IFNγ and IL-2 (Wang et al., 2016; Oghumu et al., 2019). CD40LG (encoding CD40L) expressed by T cells is another X-linked gene reported to escape XCI (Wang et al., 2016; Sarmiento et al., 2019). CD40L promotes T cell survival and regulates B cell activation and differentiation. Stimulated CD3+ T cells from (46, XX) women and (47, XXY) KS men showed significantly more CD40L protein density and mRNA as compared to cells from (46, XY) men and (45, XO) TS women (Sarmiento et al., 2019). Biallelic expression of CD40L enhances the adaptive immune response, however it has primarily been studied in the context of autoimmunity (Sarmiento et al., 2019; Syrett and Anguera, 2019).
During respiratory virus infection, B cell production of antibodies is an important mechanism of virus neutralization and clearance (Lam and Baumgarth, 2019). In addition, generation of memory B cells capable of producing high affinity antiviral antibodies upon infection or vaccination limits viral load and pathology upon subsequent infection with homo- or heterosubtypic viruses (Dhakal et al., 2021; Ursin et al., 2022). Sex differences in antibody production, particularly after vaccination, have been well studied in small mammal models and humans. Females produce a larger quantity and repertoire of antibodies specific for IAV and SARS-CoV-2 proteins (Lorenzo et al., 2011; Fink et al., 2018; Voigt et al., 2019; Dhakal et al., 2021; Ursin et al., 2022). An elegant study showed that enhanced seroconversion of HA-inhibiting antibodies was positively associated with the levels of estradiol in both adult and aged women, and that treatment of gonadectomized mice with either estradiol (females) or testosterone (males) led to an increase or decrease in antibody response, respectively (Potluri et al., 2019). These data confirm findings from an earlier study that showed levels of testosterone negatively correlate to antibody responses in males after vaccination against IAV (Furman et al., 2014). Due to their expression of both ERɑ and ERβ (Hill et al., 2011), B cells are likely to be directly regulated by estrogens during viral infection.
Much of our information about mechanisms by which estrogens and ERα regulate B cells function comes from studies of female sex-biased autoimmune diseases such as systemic lupus erythematosus, in which B cells produce high levels of autoantibodies (Dodd and Menon, 2022). ERα acts to modulate BCR signaling and promote the loss of B-cell tolerance, specifically in females, in murine lupus models (Grimaldi et al., 2001; Hill et al., 2011; Graham et al., 2020). Other work showed that ER activity enhances activated B cell expression of AID (encoded by Aicda), which is required for class switch recombination and somatic hypermutation (Muramatsu et al., 2000; Mai et al., 2010). Aicda expression is induced by the synergistic binding of HOXC4 and NF-κB to regulatory elements (Park et al., 2009), and ERɑ promotes this interaction by binding to the HoxC4 promoter (Mai et al., 2010). In addition, AID expression can be silenced through multiple mechanisms including binding of miR-26a to the 3′UTR of the Aicda mRNA. Using both global (Esr1−/−) or B cell specific Esr1 deficient (Aicda-cre-Esr1−/−) mice, researchers determined that ERɑ downregulated the expression of miR-26a through both direct and indirect binding to the genes responsible for miR-26a expression. The downregulation of miR-26a decreased AID silencing, thereby increasing somatic hypermutation (Casali et al., 2020). This mechanism may contribute to the enhanced antibody production seen in females in infection or vaccination.
B cells also display sex differences based upon their sex chromosome complement. Maintenance of XIST in naïve T and B cells is altered until they are activated, allowing for greater biallelic expression of some X-linked genes (Wang et al., 2016). One such gene is CXORF21, which encodes for the protein TASL (Heinz et al., 2020). TASL acts synergistically with TLR7, enhancing the downstream immune responses of TLR7 signaling and type 1 IFN production (Harris et al., 2019; Odhams et al., 2019). In B cells, TLR7 signaling promotes isotype switching, maturation, and antibody production (Jego et al., 2003). Biallelic expression of TLR7 in B cells is associated with greater antiviral antibody responses. In mice, vaccination with whole or subunit inactivated IAV was linked to greater expression of Tlr7 in females as compared to males, leading to a higher quantity and quality of IAV-specific antibodies (Fink et al., 2018). Human studies also show that after vaccination with the trivalent inactivated influenza vaccine, females of reproductive age generate higher antibody titers than males (Potluri et al., 2019). Elevated expression of TLR7 is also linked to the production of autoantibodies in autoimmune diseases, such as systemic lupus erythematosus (Souyris et al., 2018).
Lessons from regulatory and type 2 immunity
Often studied in the context of type 2 inflammation, such as asthma or helminth infections, regulatory T cells (Tregs) and innate type 2 lymphocytes (ILC2s) contribute to viral infections by promoting resolution and repair. In response to viral insult, infected epithelial cells release the alarmin IL-33 (Le Goffic et al., 2011), which in turn activates ILC2s and Tregs [reviewed in (Liew et al., 2016)]. Activated ILC2s secrete large amounts of IL-5, IL-4, and IL-13 (Kabata et al., 2018) that activate other immune cells, whereas Tregs most notably release high amounts of IL-10 that quell the inflammatory environment (Schmidt et al., 2012). Several reports in mouse models of asthma have indicated that both cell-intrinsic and extrinsic signaling through sex steroid receptors may regulate the function and/or development of both cell types.
ILC2s express high levels of Ar mRNA with little to no expression of Esr1 or Esr2 (Heng et al., 2008; Laffont et al., 2017), with the exception of those in the uterus (Bartemes et al., 2018). Androgens negatively regulate the transition of ILC precursors in the bone marrow to mature ILC2s in the lungs of male mice, which correlates to a two-fold increase in total numbers in the female lung compared to age matched males (Kadel et al., 2018). This difference in ILC2 numbers is mirrored in the blood of asthmatic patients (Cephus et al., 2017). Orchiectomy of male mice abolished the sex differences observed in asthma (Laffont et al., 2017), and androgen treatment of female mice after ovariectomy resulted in ILC2 numbers and functional responses typical of intact males (Blanquart et al., 2022). While these studies show that ILC2 development and number are influenced by sex steroids, mice bearing AR deficiency, specifically in ILC2s, will help delineate the cell intrinsic mechanisms that drive these phenotypes.
Tregs were reported to express quite low levels of Ar, Esr1 and Esr2 RNA (Heng et al., 2008), yet studies show evidence for cell-intrinsic ER or AR activity in Tregs. AR signaling reduces the number of pro-inflammatory Tregs in asthmatic male mice by decreasing their expression of IL-33R and activation by IL-33 (Gandhi et al., 2022). In contrast, physiological and pharmaceutical levels of E2 increase expansion of natural Tregs and induce the expression of FOXP3 in CD4+CD25– T cells in an ERα-dependent manner (Polanczyk et al., 2004; Tai et al., 2008). E2 acting via ERβ augments Treg expression of FOXP3, CD25 and GATA3, and E2-exposed Tregs promote resolution of bacterial pneumonia (Xiong et al., 2021). As reduction of inflammation is essential for recovery from viral infections, sex steroid regulation of Treg numbers and function may contribute to the sex differences observed in immunopathology following viral infection.
Taken together, these data highlight a role for the sex steroid regulation of Treg and ILC2 numbers and function in the respiratory tract. Notably, both ILC2s and Tregs produce high levels of the growth factor amphiregulin (Arpaia et al., 2015; Kabata et al., 2018), and loss of this function exacerbates immunopathology and delays tissue repair in murine IAV infection (Monticelli et al., 2011; Arpaia et al., 2015). IAV infected males produce higher levels of amphiregulin, which correlates with improved pulmonary function compared to females in the recovery phase (Vermillion et al., 2018b). While treatment of gonadectomized males with testosterone improved IAV outcomes, direct regulation of amphiregulin by AR activity in ILC2s or Tregs was not reported. Androgens have been shown to increase the number of epithelial cells that release IL-33 in the visceral adipose tissue and increase the number of immunosuppressive Tregs in males (Vasanthakumar et al., 2020), consistent with the elevated numbers of stable Tregs observed in males with asthma (Gandhi et al., 2022). In contrast, lungs in naïve 8 week old females were shown to contain higher amounts of IL-33 compared to male lungs (Matha et al., 2019). These AR-mediated mechanisms may contribute to the protection observed in males after IAV infection (Vom Steeg et al., 2019; Vom Steeg et al., 2020). As Tregs are required for the development of CD8+ memory T cells (de Goer de Herve et al., 2012), Treg expansion promoted by estradiol may lead to the enhanced protection against secondary viral infections observed in females (Ursin and Klein, 2021).
Male sex and AR regulation of type 2 immunity has implications for viral infection and secondary links to asthma susceptibility that differs before and after puberty. In a murine model of neonatal RSV infection, female pups showed better virus control with higher levels of IFNβ not seen in male pups (Malinczak et al., 2019). This led to persistent immune cell skewing in male offspring, including elevated Th2 and Th17 cells, ILC2s, and type 2 cytokines such as IL-33 and TSLP that correlated with exacerbation of allergic responses, which are often preferentially observed in young boys. However, in murine models of asthma after sexual maturity, AR activity decreases effector Th2 and Th17 cells, which correlates with epidemiological data showing that asthma propensity is decreased in males after adolescence. RNA-sequencing analyses of AR-deficient T cells show an increase in the production of type 2 cytokines in males with little effect on females, further highlighting an anti-inflammatory role of androgens in males (Ejima et al., 2022). Mouse models of house dust mite-induced asthma show that cell intrinsic AR activity attenuated IL-17A+ Th17 cells, leading to a decrease in neutrophil infiltration, while endogenous androgens indirectly decreased the number of pro-inflammatory IL-13+ Th2 cells by suppressing IL-4 production (Fuseini et al., 2018). Future research will determine if common pathways are activated or attenuated by intrinsic AR activity in Th1 cells prominent in IFN-centered respiratory virus infections and/or in Th2 and Th17 cells important in asthma and extracellular bacterial infections.
Conclusion
Sex steroids and X chromosome complement act in tandem or independently to mediate the sex differences seen in human disease and animal models (Figure 2). Herein, we have summarized evidence that sex steroids bound to their receptors and/or gene dosage on the X chromosome regulate immune responses in respiratory virus infection in humans and animal models. We have discussed how our understanding of immune mechanisms in infection may be informed by studies of sex differences in inflammatory pathways in autoimmune and other immune-mediated diseases. Taken together, these data reinforce the idea that multi-faceted immune responses to infection may be governed at various points by factors related to sex differences. For example, AR signaling may promote resolution of immune responses by attenuating T cell responses, while ER signaling may promote type I IFN production by innate cells. Secondly, a sex difference in response to or outcome of infection may be due to the presence or absence of sex-specific factors. For example, a particular pathway outcome in males may be due either to direct effects of AR signaling or the absence of robust ER signaling.
Ideally, an increased understanding of sex specific mechanisms underlying sex differences in pulmonary immunity would lead to improved treatment and precision medicine options for respiratory infection (Stachenfeld and Mazure, 2022). In the COVID-19 pandemic, observations of a male bias in mortality led to clinical trials involving estrogen or selective ER modulator administration or androgen blockade. To date, these clinical trials for COVID-19 have been inconclusive, and the field currently lacks consensus as to whether disparate sex steroid levels drive susceptibility or infection outcomes in the human population [reviewed in (Lott et al., 2022)]. Human studies that ascertain hormonal and chromosomal sex will benefit the field, as valuable data may be lost when sex steroid levels and sex chromosome complement are not considered and/or assumed to match overt gender identity. The challenge is to understand more fully how cell intrinsic sex steroid receptor expression and X chromosome complement regulate the epigenome, transcriptome, and proteome in immune cells, either prior to or during infection. This information will increase our understanding of the sex disparate pathways underlying immune function in antiviral responses.
Author contributions
RM and AW wrote the first draft of the manuscript. SK added content and edited the manuscript. AW prepared the figures. All authors approved the submitted version.
Funding
The writing of this review was made possible through funds from the Presbyterian Health Foundation and NIH EB025596. RM was supported by an OMRF predoctoral fellowship.
Conflict of interest
The authors declare that the research was conducted in the absence of any commercial or financial relationships that could be construed as a potential conflict of interest.
Publisher’s note
All claims expressed in this article are solely those of the authors and do not necessarily represent those of their affiliated organizations, or those of the publisher, the editors and the reviewers. Any product that may be evaluated in this article, or claim that may be made by its manufacturer, is not guaranteed or endorsed by the publisher.
References
Abramowitz, L. K., Olivier-Van Stichelen, S., and Hanover, J. A. (2014). Chromosome imbalance as a driver of sex disparity in disease. J. Genomics 2, 77–88. doi:10.7150/jgen.8123
Alghamdi, I. G., Hussain, , Almalki, S. S., Alghamdi, M. S., Alghamdi, M. M., and El-Sheemy, M. A. (2014). The pattern of Middle East respiratory syndrome coronavirus in Saudi arabia: A descriptive epidemiological analysis of data from the Saudi ministry of health. Int. J. Gen. Med. 7, 417–423. doi:10.2147/IJGM.S67061
Alwani, M., Yassin, A., Al-Zoubi, R. M., Aboumarzouk, O. M., Nettleship, J., Kelly, D., et al. (2021). Sex-based differences in severity and mortality in COVID-19. Rev. Med. Virol. 31, e2223. doi:10.1002/rmv.2223
Arnold, A. P., and Chen, X. (2009). What does the "four core genotypes" mouse model tell us about sex differences in the brain and other tissues? Front. Neuroendocrinol. 30, 1–9. doi:10.1016/j.yfrne.2008.11.001
Arpaia, N., Green, J. A., Moltedo, B., Arvey, A., Hemmers, S., Yuan, S., et al. (2015). A distinct function of regulatory T cells in tissue protection. Cell 162, 1078–1089. doi:10.1016/j.cell.2015.08.021
Bartemes, K., Chen, C. C., Iijima, K., Drake, L., and Kita, H. (2018). IL-33-Responsive group 2 innate lymphoid cells are regulated by female sex hormones in the uterus. J. Immunol. 200, 229–236. doi:10.4049/jimmunol.1602085
Blanquart, E., Mandonnet, A., Mars, M., Cenac, C., Anesi, N., Mercier, P., et al. (2022). Targeting androgen signaling in ILC2s protects from IL-33-driven lung inflammation, independently of KLRG1. J. Allergy Clin. Immunol. 149, 237–251 e12. doi:10.1016/j.jaci.2021.04.029
Branche, A. R., Saiman, L., Walsh, E. E., Falsey, A. R., Jia, H., Barrett, A., et al. (2022). Change in functional status associated with respiratory syncytial virus infection in hospitalized older adults. Influenza Other Respir. Viruses 16, 1151–1160. doi:10.1111/irv.13043
Butler-Laporte, G., Gonzalez-Kozlova, E., Su, C. Y., Zhou, S., Nakanishi, T., Brunet-Ratnasingham, E., et al. (2022). The dynamic changes and sex differences of 147 immune-related proteins during acute COVID-19 in 580 individuals. Clin. Proteomics 19, 34. doi:10.1186/s12014-022-09371-z
Carey, M. A., Card, J. W., Voltz, J. W., Germolec, D. R., Korach, K. S., and Zeldin, D. C. (2007). The impact of sex and sex hormones on lung physiology and disease: Lessons from animal studies. Am. J. Physiol. Lung Cell Mol. Physiol. 293, L272–L278. doi:10.1152/ajplung.00174.2007
Carreras, E., Turner, S., Frank, M. B., Knowlton, N., Osban, J., Centola, M., et al. (2010). Estrogen receptor signaling promotes dendritic cell differentiation by increasing expression of the transcription factor IRF4. Blood 115, 238–246. doi:10.1182/blood-2009-08-236935
Carreras, E., Turner, S., Paharkova-Vatchkova, V., Mao, A., Dascher, C., and Kovats, S. (2008). Estradiol acts directly on bone marrow myeloid progenitors to differentially regulate GM-CSF or Flt3 ligand-mediated dendritic cell differentiation. J. Immunol. 180, 727–738. doi:10.4049/jimmunol.180.2.727
Casali, P., Shen, T., Xu, Y., Qiu, Z., Chupp, D. P., Im, J., et al. (2020). Estrogen reverses HDAC inhibitor-mediated repression of Aicda and class-switching in antibody and autoantibody responses by downregulation of miR-26a. Front. Immunol. 11, 491. doi:10.3389/fimmu.2020.00491
CDC (2021). Estimated flu-related illnesses, medical visits, hospitalizations, and deaths in the United States - 2019-2020 flu season.
Cephus, J. Y., Stier, M. T., Fuseini, H., Yung, J. A., Toki, S., Bloodworth, M. H., et al. (2017). Testosterone attenuates group 2 innate lymphoid cell-mediated airway inflammation. Cell Rep. 21, 2487–2499. doi:10.1016/j.celrep.2017.10.110
Channappanavar, R., Fehr, A. R., Vijay, R., Mack, M., Zhao, J., Meyerholz, D. K., et al. (2016). Dysregulated type I interferon and inflammatory monocyte-macrophage responses cause lethal pneumonia in SARS-CoV-infected mice. Cell Host Microbe 19, 181–193. doi:10.1016/j.chom.2016.01.007
Channappanavar, R., Fehr, A. R., Zheng, J., Wohlford-Lenane, C., Abrahante, J. E., Mack, M., et al. (2019). IFN-I response timing relative to virus replication determines MERS coronavirus infection outcomes. J. Clin. Invest. 129, 3625–3639. doi:10.1172/JCI126363
Channappanavar, R., Fett, C., Mack, M., Ten Eyck, P. P., Meyerholz, D. K., and Perlman, S. (2017). Sex-based differences in susceptibility to severe acute respiratory syndrome coronavirus infection. J. Immunol. 198, 4046–4053. doi:10.4049/jimmunol.1601896
Cutolo, M., and Straub, R. H. (2020). Sex steroids and autoimmune rheumatic diseases: State of the art. Nat. Rev. Rheumatol. 16, 628–644. doi:10.1038/s41584-020-0503-4
Davidson, S., Mccabe, T. M., Crotta, S., Gad, H. H., Hessel, E. M., Beinke, S., et al. (2016). IFNλ is a potent anti-influenza therapeutic without the inflammatory side effects of IFNα treatment. EMBO Mol. Med. 8, 1099–1112. doi:10.15252/emmm.201606413
De Goer De Herve, M. G., Jaafoura, S., Vallee, M., and Taoufik, Y. (2012). FoxP3⁺ regulatory CD4 T cells control the generation of functional CD8 memory. Nat. Commun. 3, 986. doi:10.1038/ncomms1992
De Jacobis, I. T., Vona, R., Straface, E., Gambardella, L., Ceglie, G., De Gennaro, F., et al. (2020). Sex differences in blood pro-oxidant status and platelet activation in children admitted with respiratory syncytial virus bronchiolitis: A pilot study. Ital. J. Pediatr. 46, 29. doi:10.1186/s13052-020-0792-x
Dhakal, S., Ruiz-Bedoya, C. A., Zhou, R., Creisher, P. S., Villano, J. S., Littlefield, K., et al. (2021). Sex differences in lung imaging and SARS-CoV-2 antibody responses in a COVID-19 golden Syrian hamster model. mBio 12, e0097421. doi:10.1128/mBio.00974-21
Diebold, S. S., Kaisho, T., Hemmi, H., Akira, S., and Reis E Sousa, C. (2004). Innate antiviral responses by means of TLR7-mediated recognition of single-stranded RNA. Science 303, 1529–1531. doi:10.1126/science.1093616
Dodd, K. C., and Menon, M. (2022). Sex bias in lymphocytes: Implications for autoimmune diseases. Front. Immunol. 13, 945762. doi:10.3389/fimmu.2022.945762
Dunn, S. E., Ousman, S. S., Sobel, R. A., Zuniga, L., Baranzini, S. E., Youssef, S., et al. (2007). Peroxisome proliferator-activated receptor (PPAR)alpha expression in T cells mediates gender differences in development of T cell-mediated autoimmunity. J. Exp. Med. 204, 321–330. doi:10.1084/jem.20061839
Ejima, A., Abe, S., Shimba, A., Sato, S., Uehata, T., Tani-Ichi, S., et al. (2022). Androgens alleviate allergic airway inflammation by suppressing cytokine production in Th2 cells. J. Immunol. 209, 1083–1094. doi:10.4049/jimmunol.2200294
Eshima, N., Tokumaru, O., Hara, S., Bacal, K., Korematsu, S., Tabata, M., et al. (2011). Sex- and age-related differences in morbidity rates of 2009 pandemic influenza A H1N1 virus of swine origin in Japan. PLoS One 6, e19409. doi:10.1371/journal.pone.0019409
Fernandez Lahore, G., Forster, M., Johannesson, M., Sabatier, P., Lonnblom, E., Aoun, M., et al. (2021). Polymorphic estrogen receptor binding site causes Cd2-dependent sex bias in the susceptibility to autoimmune diseases. Nat. Commun. 12, 5565. doi:10.1038/s41467-021-25828-5
Fink, A. L., Engle, K., Ursin, R. L., Tang, W. Y., and Klein, S. L. (2018). Biological sex affects vaccine efficacy and protection against influenza in mice. Proc. Natl. Acad. Sci. U. S. A. 115, 12477–12482. doi:10.1073/pnas.1805268115
Fourati, S., Tomalin, L. E., Mule, M. P., Chawla, D. G., Gerritsen, B., Rychkov, D., Henrich, E., Miller, H. E. R., Hagan, T., Diray-Arce, J., Dunn, P., Levy, O., Gottardo, R., Sarwal, M. M., Tsang, J. S., Suarez-Farinas, M., Pulendran, B., Kleinstein, S. H., and Sekaly, R. P.C. (2022). Pan-vaccine analysis reveals innate immune endotypes predictive of antibody responses to vaccination. Nat. Immunol. 23, 1777–1787. doi:10.1038/s41590-022-01329-5
Furman, D., Hejblum, B. P., Simon, N., Jojic, V., Dekker, C. L., Thiebaut, R., et al. (2014). Systems analysis of sex differences reveals an immunosuppressive role for testosterone in the response to influenza vaccination. Proc. Natl. Acad. Sci. U. S. A. 111, 869–874. doi:10.1073/pnas.1321060111
Fuseini, H., Yung, J. A., Cephus, J. Y., Zhang, J., Goleniewska, K., Polosukhin, V. V., et al. (2018). Testosterone decreases house dust mite-induced type 2 and IL-17a-mediated airway inflammation. J. Immunol. 201, 1843–1854. doi:10.4049/jimmunol.1800293
Gandhi, V. D., Cephus, J. Y., Norlander, A. E., Chowdhury, N. U., Zhang, J., Ceneviva, Z. J., et al. (2022). Androgen receptor signaling promotes Treg suppressive function during allergic airway inflammation. J. Clin. Invest. 132, e153397. doi:10.1172/JCI153397
Gebhard, C., Regitz-Zagrosek, V., Neuhauser, H. K., Morgan, R., and Klein, S. L. (2020). Impact of sex and gender on COVID-19 outcomes in Europe. Biol. Sex. Differ. 11, 29. doi:10.1186/s13293-020-00304-9
Gilliver, S. C. (2010). Sex steroids as inflammatory regulators. J. Steroid Biochem. Mol. Biol. 120, 105–115. doi:10.1016/j.jsbmb.2009.12.015
Giurgea, L. T., Cervantes-Medina, A., Walters, K. A., Scherler, K., Han, A., Czajkowski, L. M., et al. (2022). Sex differences in influenza: The challenge study experience. J. Infect. Dis. 225, 715–722. doi:10.1093/infdis/jiab422
Gomez, J. M. D., Du-Fay-De-Lavallaz, J. M., Fugar, S., Sarau, A., Simmons, J. A., Clark, B., et al. (2021). Sex differences in COVID-19 hospitalization and mortality. J. Womens Health (Larchmt) 30, 646–653. doi:10.1089/jwh.2020.8948
Gomez-Carballa, A., Pardo-Seco, J., Pischedda, S., Rivero-Calle, I., Butler-Laporte, G., Richards, J. B., et al. (2022). Sex-biased expression of the TLR7 gene in severe COVID-19 patients: Insights from transcriptomics and epigenomics. Environ. Res. 215, 114288. doi:10.1016/j.envres.2022.114288
Graham, J. H., Yoachim, S. D., and Gould, K. A. (2020). Estrogen receptor alpha signaling is responsible for the female sex bias in the loss of tolerance and immune cell activation induced by the lupus susceptibility locus Sle1b. Front. Immunol. 11, 582214. doi:10.3389/fimmu.2020.582214
Griesbeck, M., Ziegler, S., Laffont, S., Smith, N., Chauveau, L., Tomezsko, P., et al. (2015). Sex differences in plasmacytoid dendritic cell levels of IRF5 drive higher IFN-alpha production in women. J. Immunol. 195, 5327–5336. doi:10.4049/jimmunol.1501684
Grimaldi, C. M., Michael, D. J., and Diamond, B. (2001). Cutting edge: Expansion and activation of a population of autoreactive marginal zone B cells in a model of estrogen-induced lupus. J. Immunol. 167, 1886–1890. doi:10.4049/jimmunol.167.4.1886
Guan, X., Polesso, F., Wang, C., Sehrawat, A., Hawkins, R. M., Murray, S. E., et al. (2022). Androgen receptor activity in T cells limits checkpoint blockade efficacy. Nature 606, 791–796. doi:10.1038/s41586-022-04522-6
Harris, V. M., Koelsch, K. A., Kurien, B. T., Harley, I. T. W., Wren, J. D., Harley, J. B., et al. (2019). Characterization of cxorf21 provides molecular insight into female-bias immune response in SLE pathogenesis. Front. Immunol. 10, 2160. doi:10.3389/fimmu.2019.02160
He, W. W., Kumar, M. V., and Tindall, D. J. (1991). A frame-shift mutation in the androgen receptor gene causes complete androgen insensitivity in the testicular-feminized mouse. Nucleic Acids Res. 19, 2373–2378. doi:10.1093/nar/19.9.2373
Heinz, L. X., Lee, J., Kapoor, U., Kartnig, F., Sedlyarov, V., Papakostas, K., et al. (2020). TASL is the SLC15A4-associated adaptor for IRF5 activation by TLR7-9. Nature 581, 316–322. doi:10.1038/s41586-020-2282-0
Heng, T. S., Painter, M. W., and Immunological Genome Project, C. (2008). The immunological genome Project: Networks of gene expression in immune cells. Nat. Immunol. 9, 1091–1094. doi:10.1038/ni1008-1091
Hill, L., Jeganathan, V., Chinnasamy, P., Grimaldi, C., and Diamond, B. (2011). Differential roles of estrogen receptors alpha and beta in control of B-cell maturation and selection. Mol. Med. 17, 211–220. doi:10.2119/molmed.2010.00172
Hoffmann, J., Otte, A., Thiele, S., Lotter, H., Shu, Y., and Gabriel, G. (2015). Sex differences in H7N9 influenza A virus pathogenesis. Vaccine 33, 6949–6954. doi:10.1016/j.vaccine.2015.08.044
Hou, Y., Zhou, Y., Gack, M. U., Lathia, J. D., Kallianpur, A., Mehra, R., et al. (2021). Multimodal single-cell omics analysis identifies epithelium-immune cell interactions and immune vulnerability associated with sex differences in COVID-19. Signal Transduct. Target Ther. 6, 292. doi:10.1038/s41392-021-00709-x
Huang, A., Kandhi, S., and Sun, D. (2021). Roles of genetic predisposition in the sex bias of pulmonary pathophysiology, as a function of estrogens: Sex matters in the prevalence of lung diseases. Adv. Exp. Med. Biol. 1303, 107–127. doi:10.1007/978-3-030-63046-1_7
Igarashi, H., Kouro, T., Yokota, T., Comp, P. C., and Kincade, P. W. (2001). Age and stage dependency of estrogen receptor expression by lymphocyte precursors. Proc. Natl. Acad. Sci. U. S. A. 98, 15131–15136. doi:10.1073/pnas.011513098
Jacobsen, H., and Klein, S. L. (2021). Sex differences in immunity to viral infections. Front. Immunol. 12, 720952. doi:10.3389/fimmu.2021.720952
Jego, G., Palucka, A. K., Blanck, J. P., Chalouni, C., Pascual, V., and Banchereau, J. (2003). Plasmacytoid dendritic cells induce plasma cell differentiation through type I interferon and interleukin 6. Immunity 19, 225–234. doi:10.1016/s1074-7613(03)00208-5
Jiwrajka, N., and Anguera, M. C. (2022). The X in seX-biased immunity and autoimmune rheumatic disease. J. Exp. Med. 219, e20211487. doi:10.1084/jem.20211487
Jones, R. D., Pugh, P. J., Hall, J., Channer, K. S., and Jones, T. H. (2003). Altered circulating hormone levels, endothelial function and vascular reactivity in the testicular feminised mouse. Eur. J. Endocrinol. 148, 111–120. doi:10.1530/eje.0.1480111
Kabata, H., Moro, K., and Koyasu, S. (2018). The group 2 innate lymphoid cell (ILC2) regulatory network and its underlying mechanisms. Immunol. Rev. 286, 37–52. doi:10.1111/imr.12706
Kadel, S., Ainsua-Enrich, E., Hatipoglu, I., Turner, S., Singh, S., Khan, S., et al. (2018). A major population of functional KLRG1(-) ILC2s in female lungs contributes to a sex bias in ILC2 numbers. Immunohorizons 2, 74–86. doi:10.4049/immunohorizons.1800008
Kadel, S., and Kovats, S. (2018). Sex hormones regulate innate immune cells and promote sex differences in respiratory virus infection. Front. Immunol. 9, 1653. doi:10.3389/fimmu.2018.01653
Karlberg, J., Chong, D. S., and Lai, W. Y. (2004). Do men have a higher case fatality rate of severe acute respiratory syndrome than women do? Am. J. Epidemiol. 159, 229–231. doi:10.1093/aje/kwh056
Kilic, G., Bulut, O., Jaeger, M., Ter Horst, R., Koeken, V., Moorlag, S., et al. (2021). The immunological factors predisposing to severe covid-19 are already present in healthy elderly and men. Front. Immunol. 12, 720090. doi:10.3389/fimmu.2021.720090
Kissick, H. T., Sanda, M. G., Dunn, L. K., Pellegrini, K. L., On, S. T., Noel, J. K., et al. (2014). Androgens alter T-cell immunity by inhibiting T-helper 1 differentiation. Proc. Natl. Acad. Sci. U. S. A. 111, 9887–9892. doi:10.1073/pnas.1402468111
Klein, S. L., Hodgson, A., and Robinson, D. P. (2012). Mechanisms of sex disparities in influenza pathogenesis. J. Leukoc. Biol. 92, 67–73. doi:10.1189/jlb.0811427
Koeken, V. A., De Bree, L. C. J., Mourits, V. P., Moorlag, S. J., Walk, J., Cirovic, B., et al. (2020). BCG vaccination in humans inhibits systemic inflammation in a sex-dependent manner. J. Clin. Invest. 130, 5591–5602. doi:10.1172/JCI133935
Kovats, S. (2015). Estrogen receptors regulate innate immune cells and signaling pathways. Cell Immunol. 294, 63–69. doi:10.1016/j.cellimm.2015.01.018
Kumar, A., Zarychanski, R., Pinto, R., Cook, D. J., Marshall, J., Lacroix, J., et al. (2009). Critically ill patients with 2009 influenza A(H1N1) infection in Canada. JAMA 302, 1872–1879. doi:10.1001/jama.2009.1496
Kwon, H., Schafer, J. M., Song, N. J., Kaneko, S., Li, A., Xiao, T., et al. (2022). Androgen conspires with the CD8(+) T cell exhaustion program and contributes to sex bias in cancer. Sci. Immunol. 7, eabq2630. doi:10.1126/sciimmunol.abq2630
Labrie, F., Luu-The, V., Belanger, A., Lin, S. X., Simard, J., Pelletier, G., et al. (2005). Is dehydroepiandrosterone a hormone? J. Endocrinol. 187, 169–196. doi:10.1677/joe.1.06264
Laffont, S., Blanquart, E., Savignac, M., Cenac, C., Laverny, G., Metzger, D., et al. (2017). Androgen signaling negatively controls group 2 innate lymphoid cells. J. Exp. Med. 214, 1581–1592. doi:10.1084/jem.20161807
Laffont, S., Rouquie, N., Azar, P., Seillet, C., Plumas, J., Aspord, C., et al. (2014). X-Chromosome complement and estrogen receptor signaling independently contribute to the enhanced TLR7-mediated IFN-alpha production of plasmacytoid dendritic cells from women. J. Immunol. 193, 5444–5452. doi:10.4049/jimmunol.1303400
Lam, J. H., and Baumgarth, N. (2019). The multifaceted B cell response to influenza virus. J. Immunol. 202, 351–359. doi:10.4049/jimmunol.1801208
Le Goffic, R., Arshad, M. I., Rauch, M., L'Helgoualc'H, A., Delmas, B., Piquet-Pellorce, C., et al. (2011). Infection with influenza virus induces IL-33 in murine lungs. Am. J. Respir. Cell Mol. Biol. 45, 1125–1132. doi:10.1165/rcmb.2010-0516OC
Liew, F. Y., Girard, J. P., and Turnquist, H. R. (2016). Interleukin-33 in health and disease. Nat. Rev. Immunol. 16, 676–689. doi:10.1038/nri.2016.95
Liu, S., Kumari, S., Hu, Q., Senapati, D., Venkadakrishnan, V. B., Wang, D., et al. (2017). A comprehensive analysis of coregulator recruitment, androgen receptor function and gene expression in prostate cancer. Elife 6, e28482. doi:10.7554/eLife.28482
Lorenzo, M. E., Hodgson, A., Robinson, D. P., Kaplan, J. B., Pekosz, A., and Klein, S. L. (2011). Antibody responses and cross protection against lethal influenza A viruses differ between the sexes in C57BL/6 mice. Vaccine 29, 9246–9255. doi:10.1016/j.vaccine.2011.09.110
Lott, N., Gebhard, C. E., Bengs, S., Haider, A., Kuster, G. M., Regitz-Zagrosek, V., et al. (2022). Sex hormones in SARS-CoV-2 susceptibility: Key players or confounders? Nat. Rev. Endocrinol., 1–15. doi:10.1038/s41574-022-00780-6
Mai, T., Zan, H., Zhang, J., Hawkins, J. S., Xu, Z., and Casali, P. (2010). Estrogen receptors bind to and activate the HOXC4/HoxC4 promoter to potentiate HoxC4-mediated activation-induced cytosine deaminase induction, immunoglobulin class switch DNA recombination, and somatic hypermutation. J. Biol. Chem. 285, 37797–37810. doi:10.1074/jbc.M110.169086
Malinczak, C. A., Fonseca, W., Rasky, A. J., Ptaschinski, C., Morris, S., Ziegler, S. F., et al. (2019). Sex-associated TSLP-induced immune alterations following early-life RSV infection leads to enhanced allergic disease. Mucosal Immunol. 12, 969–979. doi:10.1038/s41385-019-0171-3
Mann, M., Cortez, V., and Vadlamudi, R. K. (2011). Epigenetics of estrogen receptor signaling: Role in hormonal cancer progression and therapy. Cancers (Basel) 3, 1691–1707. doi:10.3390/cancers3021691
Marquez, E. J., Chung, C. H., Marches, R., Rossi, R. J., Nehar-Belaid, D., Eroglu, A., et al. (2020). Sexual-dimorphism in human immune system aging. Nat. Commun. 11, 751. doi:10.1038/s41467-020-14396-9
Matha, L., Shim, H., Steer, C. A., Yin, Y. H., Martinez-Gonzalez, I., and Takei, F. (2019). Female and male mouse lung group 2 innate lymphoid cells differ in gene expression profiles and cytokine production. PLoS One 14, e0214286. doi:10.1371/journal.pone.0214286
Mettelman, R. C., Allen, E. K., and Thomas, P. G. (2022). Mucosal immune responses to infection and vaccination in the respiratory tract. Immunity 55, 749–780. doi:10.1016/j.immuni.2022.04.013
Mohammad, I., Starskaia, I., Nagy, T., Guo, J., Yatkin, E., Vaananen, K., et al. (2018). Estrogen receptor alpha contributes to T cell-mediated autoimmune inflammation by promoting T cell activation and proliferation. Sci. Signal 11, eaap9415. doi:10.1126/scisignal.aap9415
Monticelli, L. A., Sonnenberg, G. F., Abt, M. C., Alenghat, T., Ziegler, C. G., Doering, T. A., et al. (2011). Innate lymphoid cells promote lung-tissue homeostasis after infection with influenza virus. Nat. Immunol. 12, 1045–1054. doi:10.1031/ni.2131
Muramatsu, M., Kinoshita, K., Fagarasan, S., Yamada, S., Shinkai, Y., and Honjo, T. (2000). Class switch recombination and hypermutation require activation-induced cytidine deaminase (AID), a potential RNA editing enzyme. Cell 102, 553–563. doi:10.1016/s0092-8674(00)00078-7
Odhams, C. A., Roberts, A. L., Vester, S. K., Duarte, C. S. T., Beales, C. T., Clarke, A. J., et al. (2019). Interferon inducible X-linked gene CXorf21 may contribute to sexual dimorphism in Systemic Lupus Erythematosus. Nat. Commun. 10, 2164. doi:10.1038/s41467-019-10106-2
Oghumu, S., Varikuti, S., Stock, J. C., Volpedo, G., Saljoughian, N., Terrazas, C. A., et al. (2019). Cutting edge: CXCR3 escapes X chromosome inactivation in T cells during infection: Potential implications for sex differences in immune responses. J. Immunol. 203, 789–794. doi:10.4049/jimmunol.1800931
Okude, H., Ori, D., and Kawai, T. (2020). Signaling through nucleic acid sensors and their roles in inflammatory diseases. Front. Immunol. 11, 625833. doi:10.3389/fimmu.2020.625833
Orimadegun, A. E., Adepoju, A. A., and Myer, L. (2020). A systematic review and meta-analysis of sex differences in morbidity and mortality of acute lower respiratory tract infections among african children. J. Pediatr. Rev. 8, 65–78. doi:10.32598/jpr.8.2.65
Park, S. R., Zan, H., Pal, Z., Zhang, J., Al-Qahtani, A., Pone, E. J., et al. (2009). HoxC4 binds to the promoter of the cytidine deaminase AID gene to induce AID expression, class-switch DNA recombination and somatic hypermutation. Nat. Immunol. 10, 540–550. doi:10.1038/ni.1725
Phiel, K. L., Henderson, R. A., Adelman, S. J., and Elloso, M. M. (2005). Differential estrogen receptor gene expression in human peripheral blood mononuclear cell populations. Immunol. Lett. 97, 107–113. doi:10.1016/j.imlet.2004.10.007
Pihlajamaa, P., Sahu, B., and Janne, O. A. (2015). Determinants of receptor- and tissue-specific actions in androgen signaling. Endocr. Rev. 36, 357–384. doi:10.1210/er.2015-1034
Polanczyk, M. J., Carson, B. D., Subramanian, S., Afentoulis, M., Vandenbark, A. A., Ziegler, S. F., et al. (2004). Cutting edge: Estrogen drives expansion of the CD4+CD25+ regulatory T cell compartment. J. Immunol. 173, 2227–2230. doi:10.4049/jimmunol.173.4.2227
Popescu, M., Feldman, T. B., and Chitnis, T. (2021). Interplay between endocrine disruptors and immunity: Implications for diseases of autoreactive etiology. Front. Pharmacol. 12, 626107. doi:10.3389/fphar.2021.626107
Potluri, T., Fink, A. L., Sylvia, K. E., Dhakal, S., Vermillion, M. S., Vom Steeg, L., et al. (2019). Age-associated changes in the impact of sex steroids on influenza vaccine responses in males and females. NPJ Vaccines 4, 29. doi:10.1038/s41541-019-0124-6
Pujantell, M., and Altfeld, M. (2022). Consequences of sex differences in Type I IFN responses for the regulation of antiviral immunity. Front. Immunol. 13, 986840. doi:10.3389/fimmu.2022.986840
Quigley, C. A. (2002). Editorial: The postnatal gonadotropin and sex steroid surge-insights from the androgen insensitivity syndrome. J. Clin. Endocrinol. Metab. 87, 24–28. doi:10.1210/jcem.87.1.8265
Regis, E., Fontanella, S., Lin, L., Howard, R., Haider, S., Curtin, J. A., et al. (2021). Sex differences in innate anti-viral immune responses to respiratory viruses and in their clinical outcomes in a birth cohort study. Sci. Rep. 11, 23741. doi:10.1038/s41598-021-03044-x
Ren, X., Wen, W., Fan, X., Hou, W., Su, B., Cai, P., et al. (2021). COVID-19 immune features revealed by a large-scale single-cell transcriptome atlas. Cell 184, 5838. doi:10.1016/j.cell.2021.10.023
Renault, C. H., Aksglaede, L., Wojdemann, D., Hansen, A. B., Jensen, R. B., and Juul, A. (2020). Minipuberty of human infancy - a window of opportunity to evaluate hypogonadism and differences of sex development? Ann. Pediatr. Endocrinol. Metab. 25, 84–91. doi:10.6065/apem.2040094.047
Robinson, D. P., Huber, S. A., Moussawi, M., Roberts, B., Teuscher, C., Watkins, R., et al. (2011a). Sex chromosome complement contributes to sex differences in coxsackievirus B3 but not influenza A virus pathogenesis. Biol. Sex. Differ. 2, 8. doi:10.1186/2042-6410-2-8
Robinson, D. P., Lorenzo, M. E., Jian, W., and Klein, S. L. (2011b). Elevated 17β-estradiol protects females from influenza A virus pathogenesis by suppressing inflammatory responses. PLoS Pathog. 7, e1002149. doi:10.1371/journal.ppat.1002149
Robinson, G. A., Peng, J., Peckham, H., Butler, G., Pineda-Torra, I., Ciurtin, C., et al. (2022). Investigating sex differences in T regulatory cells from cisgender and transgender healthy individuals and patients with autoimmune inflammatory disease: A cross-sectional study. Lancet Rheumatol. 4, e710–e724. doi:10.1016/S2665-9913(22)00198-9
Sarmiento, L., Svensson, J., Barchetta, I., Giwercman, A., and Cilio, C. M. (2019). Copy number of the X-linked genes TLR7 and CD40L influences innate and adaptive immune responses. Scand. J. Immunol. 90, e12776. doi:10.1111/sji.12776
Sathish, V., Martin, Y. N., and Prakash, Y. S. (2015). Sex steroid signaling: Implications for lung diseases. Pharmacol. Ther. 150, 94–108. doi:10.1016/j.pharmthera.2015.01.007
Sauerwald, N., Zhang, Z., Ramos, I., Nair, V. D., Soares-Schanoski, A., Ge, Y., et al. (2022). Pre-infection antiviral innate immunity contributes to sex differences in SARS-CoV-2 infection. Cell Syst. 13, 924–931 e4. doi:10.1016/j.cels.2022.10.005
Schmidt, A., Oberle, N., and Krammer, P. H. (2012). Molecular mechanisms of treg-mediated T cell suppression. Front. Immunol. 3, 51. doi:10.3389/fimmu.2012.00051
Schoggins, J. W., and Rice, C. M. (2011). Interferon-stimulated genes and their antiviral effector functions. Curr. Opin. Virol. 1, 519–525. doi:10.1016/j.coviro.2011.10.008
Scully, E. P., Schumock, G., Fu, M., Massaccesi, G., Muschelli, J., Betz, J., et al. (2021). Sex and gender differences in testing, hospital admission, clinical presentation, and drivers of severe outcomes from COVID-19. Open Forum Infect. Dis. 8, ofab448. doi:10.1093/ofid/ofab448
Seillet, C., Laffont, S., Tremollieres, F., Rouquie, N., Ribot, C., Arnal, J. F., et al. (2012). The TLR-mediated response of plasmacytoid dendritic cells is positively regulated by estradiol in vivo through cell-intrinsic estrogen receptor alpha signaling. Blood 119, 454–464. doi:10.1182/blood-2011-08-371831
Sette, A., and Crotty, S. (2021). Adaptive immunity to SARS-CoV-2 and COVID-19. Cell 184, 861–880. doi:10.1016/j.cell.2021.01.007
Sims, N. A., Dupont, S., Krust, A., Clement-Lacroix, P., Minet, D., Resche-Rigon, M., et al. (2002). Deletion of estrogen receptors reveals a regulatory role for estrogen receptors-beta in bone remodeling in females but not in males. Bone 30, 18–25. doi:10.1016/s8756-3282(01)00643-3
Singmann, P., Shem-Tov, D., Wahl, S., Grallert, H., Fiorito, G., Shin, S. Y., et al. (2015). Characterization of whole-genome autosomal differences of DNA methylation between men and women. Epigen. Chromatin 8, 43. doi:10.1186/s13072-015-0035-3
Souyris, M., Cenac, C., Azar, P., Daviaud, D., Canivet, A., Grunenwald, S., et al. (2018). TLR7 escapes X chromosome inactivation in immune cells. Sci. Immunol. 3, eaap8855. doi:10.1126/sciimmunol.aap8855
Souyris, M., Mejia, J. E., Chaumeil, J., and Guery, J. C. (2019). Female predisposition to TLR7-driven autoimmunity: Gene dosage and the escape from X chromosome inactivation. Semin. Immunopathol. 41, 153–164. doi:10.1007/s00281-018-0712-y
Stachenfeld, N. S., and Mazure, C. M. (2022). Precision medicine requires understanding how both sex and gender influence health. Cell 185, 1619–1622. doi:10.1016/j.cell.2022.04.012
Syrett, C. M., and Anguera, M. C. (2019). When the balance is broken: X-Linked gene dosage from two X chromosomes and female-biased autoimmunity. J. Leukoc. Biol. 106, 919–932. doi:10.1002/JLB.6RI0319-094R
Tai, P., Wang, J., Jin, H., Song, X., Yan, J., Kang, Y., et al. (2008). Induction of regulatory T cells by physiological level estrogen. J. Cell Physiol. 214, 456–464. doi:10.1002/jcp.21221
Takahashi, T., Ellingson, M. K., Wong, P., Israelow, B., Lucas, C., Klein, J., et al. (2020). Sex differences in immune responses that underlie COVID-19 disease outcomes. Nature 588, 315–320. doi:10.1038/s41586-020-2700-3
Ter Horst, R., Jaeger, M., Smeekens, S. P., Oosting, M., Swertz, M. A., Li, Y., et al. (2016). Host and environmental factors influencing individual human cytokine responses. Cell 167, 1111–1124. doi:10.1016/j.cell.2016.10.018
Tuku, B., Stanelle-Bertram, S., Sellau, J., Beck, S., Bai, T., Kouassi, N. M., et al. (2020). Testosterone protects against severe influenza by reducing the pro-inflammatory cytokine response in the murine lung. Front. Immunol. 11, 697. doi:10.3389/fimmu.2020.00697
Ursin, R. L., Dhakal, S., Liu, H., Jayaraman, S., Park, H. S., Powell, H. R., et al. (2022). Greater breadth of vaccine-induced immunity in females than males is mediated by increased antibody diversity in germinal center B cells. mBio 13, e0183922. doi:10.1128/mbio.01839-22
Ursin, R. L., and Klein, S. L. (2021). Sex differences in respiratory viral pathogenesis and treatments. Annu. Rev. Virol. 8, 393–414. doi:10.1146/annurev-virology-091919-092720
Vasanthakumar, A., Chisanga, D., Blume, J., Gloury, R., Britt, K., Henstridge, D. C., et al. (2020). Sex-specific adipose tissue imprinting of regulatory T cells. Nature 579, 581–585. doi:10.1038/s41586-020-2040-3
Vermillion, M. S., Ursin, R. L., Attreed, S. E., and Klein, S. L. (2018a). Estriol reduces pulmonary immune cell recruitment and inflammation to protect female mice from severe influenza. Endocrinology 159, 3306–3320. doi:10.1210/en.2018-00486
Vermillion, M. S., Ursin, R. L., Kuok, D. I. T., Vom Steeg, L. G., Wohlgemuth, N., Hall, O. J., et al. (2018b). Production of amphiregulin and recovery from influenza is greater in males than females. Biol. Sex. Differ. 9, 24. doi:10.1186/s13293-018-0184-8
Vila, J., Lera, E., Andres, C., Pinana, M., Rello-Saltor, V., Tobena-Rue, M., et al. (2022). The burden of non-SARS-CoV2 viral lower respiratory tract infections in hospitalized children in barcelona (Spain): A long-term, clinical, epidemiologic and economic study. Influenza Other Respir. Viruses 17, e13085. doi:10.1111/irv.13085
Voigt, E. A., Ovsyannikova, I. G., Kennedy, R. B., Grill, D. E., Goergen, K. M., Schaid, D. J., et al. (2019). Sex differences in older adults' immune responses to seasonal influenza vaccination. Front. Immunol. 10, 180. doi:10.3389/fimmu.2019.00180
Vom Steeg, L. G., Attreed, S. E., Zirkin, B., and Klein, S. L. (2019). Testosterone treatment of aged male mice improves some but not all aspects of age-associated increases in influenza severity. Cell Immunol. 345, 103988. doi:10.1016/j.cellimm.2019.103988
Vom Steeg, L. G., Dhakal, S., Woldetsadik, Y. A., Park, H. S., Mulka, K. R., Reilly, E. C., et al. (2020). Androgen receptor signaling in the lungs mitigates inflammation and improves the outcome of influenza in mice. PLoS Pathog. 16, e1008506. doi:10.1371/journal.ppat.1008506
Vom Steeg, L. G., Vermillion, M. S., Hall, O. J., Alam, O., Mcfarland, R., Chen, H., et al. (2016). Age and testosterone mediate influenza pathogenesis in male mice. Am. J. Physiol. Lung Cell Mol. Physiol. 311, L1234–L1244. doi:10.1152/ajplung.00352.2016
Wang, J., Syrett, C. M., Kramer, M. C., Basu, A., Atchison, M. L., and Anguera, M. C. (2016). Unusual maintenance of X chromosome inactivation predisposes female lymphocytes for increased expression from the inactive X. Proc. Natl. Acad. Sci. U. S. A. 113, E2029–E2038. doi:10.1073/pnas.1520113113
Webb, K., Peckham, H., Radziszewska, A., Menon, M., Oliveri, P., Simpson, F., et al. (2018). Sex and pubertal differences in the type 1 interferon pathway associate with both X chromosome number and serum sex hormone concentration. Front. Immunol. 9, 3167. doi:10.3389/fimmu.2018.03167
Wilkinson, N. M., Chen, H. C., Lechner, M. G., and Su, M. A. (2022). Sex differences in immunity. Annu. Rev. Immunol. 40, 75–94. doi:10.1146/annurev-immunol-101320-125133
Xiong, Y., Zhong, Q., Palmer, T., Benner, A., Wang, L., Suresh, K., et al. (2021). Estradiol resolves pneumonia via ERβ in regulatory T cells. JCI Insight 6, e133251. doi:10.1172/jci.insight.133251
Yu, C., Littleton, S., Giroux, N. S., Mathew, R., Ding, S., Kalnitsky, J., et al. (2021). Mucosal-associated invariant T cell responses differ by sex in COVID-19. Med. (N Y) 2, 755–772 e5. doi:10.1016/j.medj.2021.04.008
Zhang, M. A., Rego, D., Moshkova, M., Kebir, H., Chruscinski, A., Nguyen, H., et al. (2012). Peroxisome proliferator-activated receptor (PPAR)α and -γ regulate IFNγ and IL-17A production by human T cells in a sex-specific way. Proc. Natl. Acad. Sci. U. S. A. 109, 9505–9510. doi:10.1073/pnas.1118458109
Keywords: respiratory virus, pulmonary immunity, sex differences, estrogen, androgen, X chromosome inactivation
Citation: Miller RAJ, Williams AP and Kovats S (2023) Sex chromosome complement and sex steroid signaling underlie sex differences in immunity to respiratory virus infection. Front. Pharmacol. 14:1150282. doi: 10.3389/fphar.2023.1150282
Received: 24 January 2023; Accepted: 08 March 2023;
Published: 30 March 2023.
Edited by:
Yanira Vasquez, King’s College London, United KingdomReviewed by:
Elisabetta Vegeto, University of Milan, ItalyAnne Schumacher, Helmholtz Association of German Research Centres (HZ), Germany
Copyright © 2023 Miller, Williams and Kovats. This is an open-access article distributed under the terms of the Creative Commons Attribution License (CC BY). The use, distribution or reproduction in other forums is permitted, provided the original author(s) and the copyright owner(s) are credited and that the original publication in this journal is cited, in accordance with accepted academic practice. No use, distribution or reproduction is permitted which does not comply with these terms.
*Correspondence: Susan Kovats, U3VzYW4tS292YXRzQG9tcmYub3Jn
†These authors have contributed equally to this work