- 1Department of Breast and Thyroid Surgery. Renmin Hospital of Wuhan University, Wuhan, China
- 2School of Science, University of Sydney, Sydney, New South Wales, NSW, Australia
Ferritinophagy, a form of autophagy, is also an important part of ferroptosis, a type of regulated cell death resulting from abnormal iron metabolism involving the production of reactive oxygen species. As ferroptosis, autophagy and cancer have been revealed, ferritinophagy has attracted increasing attention in cancer development. In this review, we discuss the latest research progress on ferroptosis, autophagy-associated ferroptosis led by ferritinophagy, the regulators of ferritinophagy and promising cancer treatments that target ferritinophagy. Ferritinophagy is at the intersection of ferroptosis and autophagy and plays a significant role in cancer development. The discussed studies provide new insights into the mechanisms of ferritinophagy and promising related treatments for cancer.
1 Introduction
Ferroptosis is a form of regulated cell death that, is highly related to reactive oxygen species (ROS) and lipid metabolism (Lambeth and Neish, 2014). Autophagy, a self-degradative process that is, important for cellular homeostasis, is divided into three main types: macroautophagy, microautophagy, and chaperone-mediated autophagy (Santana-Codina and Mancias, 2018). Researchers have found that ferroptosis is strongly correlated with autophagy and cancer, and previous studies have revealed ferritinophagy; this review places an emphasis on ferritinophagy (Kang and Tang, 2017). Ferritinophagy is a new autophagy process associated with ferroptosis in which the binding of nuclear receptor coactivator 4 (NCOA4) and ferritin in autophagosomes (Dixon et al., 2012) causes autophagic degradation (Tang et al., 2018). Research related to cancer and ferritinophagy conducted over the past few years has shown new intracellular processes and mechanisms connecting iron and cancer (Manz et al., 2016). An increasing number of studies have shown that ferritinophagy can influence the growth of tumors (Su et al., 2020; Chen et al., 2021). In this review, we mainly discuss the process of ferritinophagy, its regulators and probable treatments for cancers that target ferritinophagy.
2 Latest progress in ferroptosis
2.1 Ferroptosis regulates different diseases
Ferroptosis is an iron-induced, lipid peroxide-driven form of programmed cell death (Stockwell et al., 2017). Ferroptosis is distinguished from other forms of cell death by morphological features, including smaller, abnormally shaped mitochondria with decreased and flat cristae, condensed mitochondrial membranes, and a ruptured outer membrane (Gao et al., 2019). Membrane oxidative damage is the final step of ferroptosis, and triggers of this process have been identified. Lethal lipid peroxide (LPO), which is cytotoxic, is the primary cause of ferroptosis (Li et al., 2021a). Over the past decades, different diseases, including cardiovascular diseases, ischemia–reperfusion injury, acute kidney injury and neural diseases, particularly cancer, have been found to be closely associated with ferroptosis (Hambright et al., 2017; Chen et al., 2020; Lee et al., 2020; Wang et al., 2020; Shao et al., 2021; Wang et al., 2021).
2.2 Typical GPX4-mediated ferroptosis
Ferroptosis was first identified by the discovery of erastin and RSL3 (Dixon et al., 2012). Cell death induced by the compounds occurs in the absence of the core apoptosis machinery caspases BAX and BAK. The knockdown of RIPK1/RIPK3, CASP1/4 and PARP1 also has no influence on ferroptosis, which means that this type of cell death is distinct from apoptosis, necroptosis, pyroptosis and parthanatos (Yagoda et al., 2007; Yang and Stockwell, 2008; Friedmann Angeli et al., 2014). Further studies have demonstrated that the function of erastin in this process is to inhibit the xc-system and that RSL3 inhibits GPX4; the former is a cysteine/glutamate transporter, and the latter is known as a phospholipid hydroperoxidase (Yang et al., 2014). Recent studies have identified more ferroptotic inducer targets, such as erastin2, IKE, sorafenib, sulfasalazine, glutamate, FIN56, ML162, ML210, FINO2 and DPIs. Targeting xc-/GPX4 has had a great impact on diseases related to ferroptosis (Louandre et al., 2013; Gaschler et al., 2018; Zhang et al., 2019; Sun et al., 2021a; Koppula et al., 2021; Moosmayer et al., 2021; Zhou et al., 2021). Moreover, typical signaling pathways, such as p62-Keap1-Nrf2, have been proven to regulate the xc-system, which offers a target (Pietsch et al., 2003; Shin et al., 2018; Dodson et al., 2019; Qiang et al., 2020) (Figure 1).
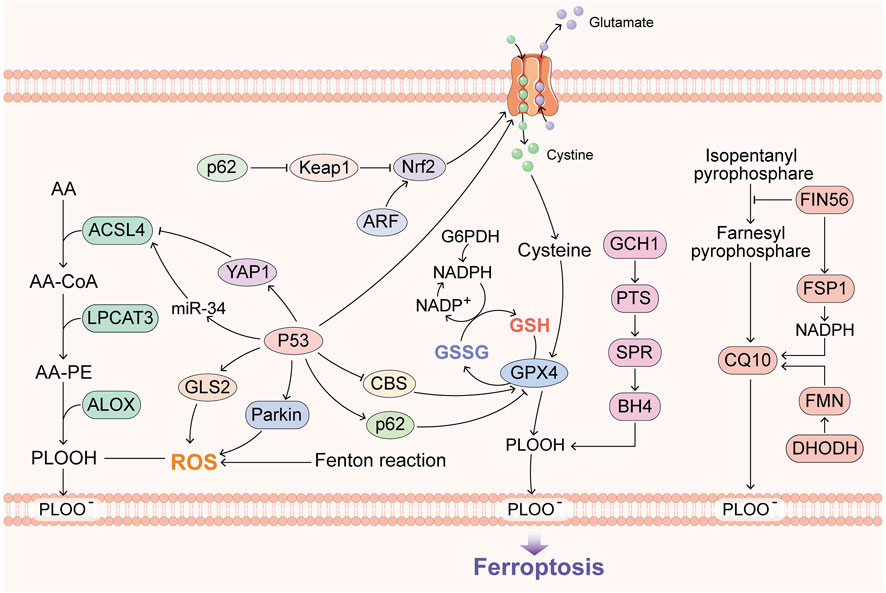
FIGURE 1. Overview of the recent research progress on ferroptosis. GPX4, BH4, FSP1 and DHODH constitute the main defense system of ferroptosis. ACSL4 and the Fenton reaction promote ferroptosis, and p53 has dual roles in ferroptosis.
2.3 GPX4-independent ferroptosis
Specifically, GPX4, FSP1 and BH4 are considered to constitute three major ferroptosis defense systems (Doll et al., 2019). FSP1 is a GPX4-independent ferroptosis suppressor, and FSP1 is also called apoptosis-inducing factor mitochondria-associated 2 (AIFM2), which confers protection against ferroptosis in the presence of GPX4 deletion (Shimada et al., 2016; Bersuker et al., 2019). Further research has demonstrated that its suppression is mediated by CoQ10. FSP1 can catalyze the regeneration of CoQ10 using NAD(p)H to ensure lipid peroxidation (Bersuker et al., 2019; Kraft et al., 2020). BH4 is also independent of the two other pathways, and its antioxidant capacity is shown by capturing free radicals and transferring them to BH2 (Soula et al., 2020). GCH1 catalyzes the rate-limiting step in the production of BH4, and GCH1-knockout cells are not sensitive to erastin treatment or other ROS-inducing compounds, which means that BH4 specifically confronts lipid peroxidation (Soula et al., 2020). Subsequently, an increasing number of discoveries on GPX4-independent ferroptosis have been revealed.
Recently, the mechanisms of p53 in inducing ferroptosis have been revealed, and both the canonical (GPX4-dependent) and noncanonical (GPX4-independent) ferroptosis pathways, as typical transcription factors, provide room for the imagination of ferroptosis and cancer (Jiang et al., 2015). DHODH was also found to operate in parallel with mitochondrial GPX4, independent of cytosolic GPX4 and FSP1, for reducing CoQ to CoQ10. The DHODH inhibitor brequinar has been applied to low-GPX4 tumors, and combined treatment with brequinar and sulfasalazine can inhibit high-GPX4 tumors (Mao et al., 2021).
2.4 Redox lipid death: Fenton reaction and PUFAS
Iron plays an important role in mammalian cells because it participates in cell metabolism, proliferation and growth (Ye et al., 2021). These processes are conducted by a variety of proteins that contain iron and hemoglobin, including many proteins that are important for cell growth (Federico et al., 2022). Iron in the human body exists in two oxidation states: ferrous iron and ferric iron (Smith et al., 2022). Iron can gain and lose electrons, which enables it to trigger the Fenton reaction, and during this reaction, Fe2+ donates an electron to hydrogen peroxide (H2O2) to form the hydroxyl radical (•HO), which is an ROS (Thomas et al., 2009). Elevated iron can cause the generation of ROS, which can damage lipids, proteins, RNA and DNA, and eventually leads to tumor growth (Zhou et al., 2011). Various types of cancers, such as lung cancer, breast cancer, prostate cancer, pancreatic cancer, colorectal cancer, hepatocellular cancer, renal cell carcinoma, and melanoma, are promoted by iron (Yan et al., 2021a). Additionally, iron can promote DNA replication and repair (Zhou et al., 2018). In addition, in 2016, ACSL4, which can enrich cellular membranes with PUFAs and alter the cellular lipid composition, was found to sensitize breast cancer cells to ferroptosis during RSL3 treatment (Doll et al., 2017). Thus, decreasing the cellular iron levels and targeting iron metabolic pathways and lipid metabolism may provide new tools for cancer therapy (Bordini et al., 2020).
3 Different types of ferroptosis evasion processes promote cancer development
3.1 The typical GPX4 pathway regulates cancer development
Ferroptosis is regulated by oncogenes, and the most frequently mutated gene, p53, as noted above, has been proven to regulate ferroptosis in dual manners (Jiang et al., 2015; Xie et al., 2017). In addition, BAP1, KEAP1, KRAS and ARF can downregulate SLC7A11 through NRF2 or NRF2-independent pathways (Chen et al., 2017; Dodson et al., 2019; Lim et al., 2019; Hu et al., 2020) and thus promote tumor metabolism.
3.2 The GPX4-independent pathway regulates cancer development
GCH1 is highly expressed in lung, breast and liver cancer, according to its defense against ferroptosis (Kraft et al., 2020; Liu et al., 2022). CoQH2 has also been shown to have a similar ability (Stefely and Pagliarini, 2017; Wei et al., 2021). Whether DHODH is the same remains unclear.
3.3 Tumor lipid metabolism and the microenvironment regulate tumor growth by ferroptosis
iPLA2β, which is overexpressed in human cancers, was recently shown to regulate ferroptosis by downregulating PUFAs (Sun et al., 2021b). ACSL3-mediated ferroptosis evasion was found in the lymphatic environment of cancer cells (Ubellacker et al., 2020). Interestingly, dysregulated cholesterol homeostasis resulting from 27 H C increases breast cancer growth by ferroptosis evasion (Liu et al., 2021), which shows that targeting the tumor microenvironment is promising in lipid metabolism because IFNγ downregulates SLC7A11 (Wang et al., 2019). When the tumor microenvironment changes, TFR1 and STEAP3 are upregulated in cancer cells, which increases iron accumulation and thus leads to ROS accumulation (Yan et al., 2021b; Ye et al., 2022).
4 Ferroptosis is strongly related to autophagy
Researchers have recently found that the inhibition of lysosome-dependent cell death by the pharmacological blockade of cathepsin activity and vacuolar-type Hb-ATPase limits erastin-induced ferroptosis, which implies that ferroptosis is a lysosome-dependent autophagic cell death process (Gao et al., 2016; Shui et al., 2021). Subsequently, further discoveries of the link between autophagy and ferroptosis have emerged (Figure 2). The cellular redox state has a great impact on autophagy (Li et al., 2021b). Lipid peroxidation and oxidized lipids can cause MAP1-LC3 turnover as well as autophagosome formation (Hill et al., 2008; Krohne et al., 2010). Growing evidence shows that excessive autophagy or lysosome activity can lead to ferroptosis through increased iron or lipid peroxidation, which means that autophagy and ferroptosis promote each other (Kang and Tang, 2017). NCOA4 is a transcriptional coactivator of nuclear hormone receptors and undergoes gene rearrangement in cancer; this coactivator mediates ferritin degradation to lead to iron accumulation in cells and thus activate the Fenton reaction (Bellelli et al., 2014). Ferritinophagy is at the intersection of ferroptosis and autophagy, and its relationship with cancer is also expected.
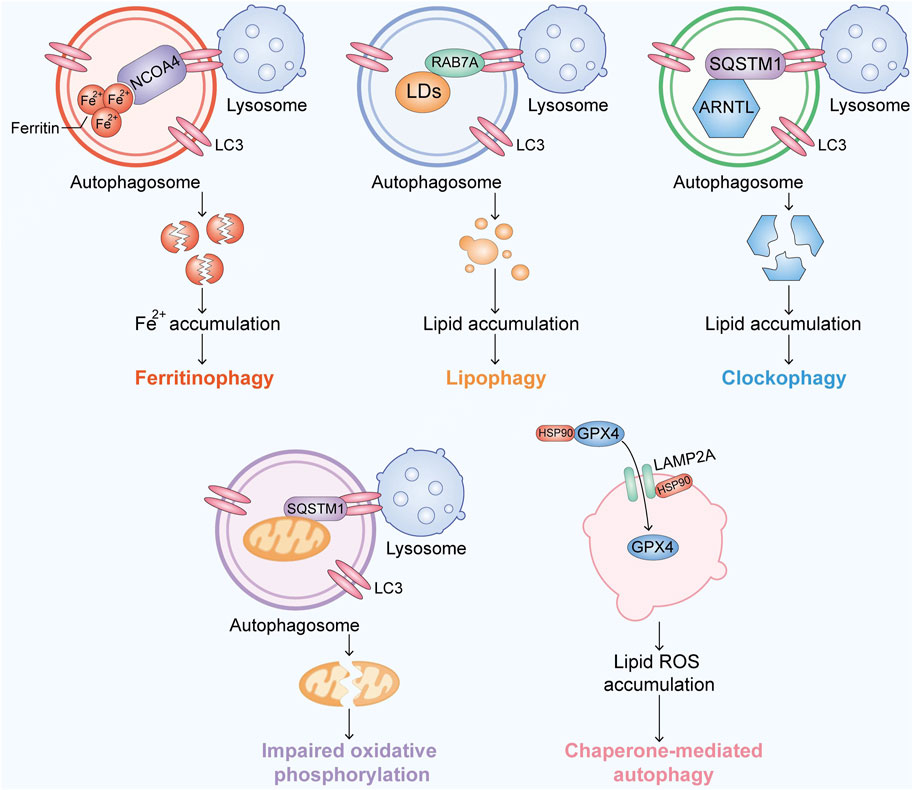
FIGURE 2. Overview of the recent research progress on autophagy-associated ferroptosis. Ferroptosis is activated by accumulating Fe, lipids or free radicals in an autophagic manner.
4.1 NCOA4-associated ferritinophagy at the intersection of autophagy and ferroptosis
Ferritin is the major storage protein of iron, and its degradation is mainly carried out by lysosomes, which causes the release of iron and induces oxidative injury (Senol et al., 2008). NCOA4 is a selective cargo receptor involved in the autophagic turnover of ferritin by lysosomes (Mancias et al., 2014; Fang et al., 2021). Ferritinophagy is mediated by NCOA4, which binds FTH1, microtubule-associated protein one and LC3–PE on the developing autophagosome membrane to sequester ferritin complexes into autophagosomes (Kaur and Debnath, 2015). The flux of ferritinophagy is totally dependent on NCOA4 (Mancias et al., 2015), which is regulated by the intracellular Fe levels. The knockdown of NCOA4 or ATGs (e.g., ATG3, ATG5, ATG7, and ATG13) could suppress ferritin degradation, iron accumulation, and lipid peroxidation caused by erastin, which means that NCOA4 is possibly related to the xc-system (Hou et al., 2016). Bafilomycin A1, an inhibitor of vacuolar-type H + -ATPase (V-ATPase) in lysosomes, also inhibits ferritin degradation and ferroptosis (Zhang et al., 2018; Kong et al., 2019). These findings provide genetic evidence showing that ferroptosis is a process of selective autophagic cell death (Bellelli et al., 2016). Ferritinophagy has been shown to cause ferroptotic cell death in multiple cancer cell lines (Hou et al., 2016).
4.2 Non-ferritinophagy autophagy-associated ferroptosis
4.2.1 RAB7A-mediated lipophagy
Lipophagy is a type of selective autophagy that causes autophagic degradation of lipid droplets (LDs) (Liu and Czaja, 2013). Importantly, lipophagy-induced LD degradation can promote lipid peroxidation in ferroptosis, and knockdown of the LD cargo receptor RAB7A or ATG5 yields the opposite result (Schroeder et al., 2015). In contrast, increased lipid storage caused by the overexpression of TPD52 reduces RSL3-induced ferroptosis (Bai et al., 2019). These findings show that the balance between lipid storage and degradation can determine the ferroptotic level.
4.2.2 Clock protein ARNTL-mediated clockophagy
Clockophagy is accomplished by selective degradation of ARNTL, the core circadian clock protein, through autophagy. SQSTM1 is a freight receptor responsible for autophagic ARNTL degradation. ARNTL inhibits ferroptosis by inhibiting Egln2 transcription and thereby activating the transcription factor HIF1A, resulting in free lipid accumulation (Liu et al., 2019). The degradation of ARNTL promotes increase in the ferroptotic levels in cells.
4.2.3 PINK1-mediated mitophagy
Ubiquitin is phosphorylated by the ubiquitin kinase PINK1, which activates the ubiquitin ligase Parkin, and activated Parkin builds ubiquitin chains on mitochondrial outer membrane proteins, where they recruit autophagy receptors (Yan et al., 2020). A novel model in which PINK1 produces phosphoryl ubiquitin as a mitochondrial autophagy signal and Parkin then amplifies the signal has been proven to influence the redox biology in cells and thus leads to ferroptosis (Lazarou et al., 2015; Yan et al., 2020; Chen et al., 2021).
4.2.4 HSP90-mediated CMA
HSP90 has been suggested as a promising anticancer drug target due to its significance in regulating the activity and stability of many proteins in human cancers (Lanneau et al., 2007). In addition, HSP90 plays important roles in the mitochondria-dependent pathway and necroptosis (Li et al., 2015; Jacobsen et al., 2016; Zhao et al., 2016). More recently, ferroptosis has been found to be related to HSP90 (Wu et al., 2019a). Mechanistically, HSP90 regulates the stability of LAMP2A, which is an isoform of LAMP2 and a receptor for CMA (De Domenico et al., 2006; Wu et al., 2019a). LAMP2A- and HSPA8/HSC70-mediated CMA result in GPX4 degradation (Wu et al., 2019a; Lahiri et al., 2019). CDDO and tanespimycin have been shown to reduce erastin/glutamate-mediated ferroptosis in HT-22 cells. Importantly, the knockdown of HSP90 by siRNA reverses erastin/glutamate-induced ferroptosis, which means that HSP90 plays an important role in this process (Lanneau et al., 2007; Wu et al., 2019a). These results, along with the finding that HSPA5 enhances GPX4 protein stabilization, imply that HSPs play a significant role in ferroptosis.
4.2.5 BECN1-mediated system xc−inhibition
The BECN1 interactome is widely known to regulate autophagy, apoptosis and many other cellular processes (Kang et al., 2011). The formation of a BECN1-SLC7A11 complex can promote the activation of ferroptosis activators such as erastin but has no influence on RSL3 or FIN56 (Kang et al., 2018; Song et al., 2018). Moreover, the phosphorylation of BECN1 enhances BECN1-SLC7A11 complex formation, inhibition of system xc-, and subsequent ferroptotic cancer cell death (Song et al., 2018). These findings imply that BECN1 is a direct inhibitor of the xc−system that induces ferroptosis.
4.2.6 STAT3-mediated lysosomal dysfunction
Lysosomal dysfunction is a type of RCD (Kroemer and Jaattela, 2005). Recent studies indicate that lysosomes may play a potential role in promoting ferroptosis (Torii et al., 2016; Gao et al., 2018). Mechanistically, CTSB expression and release mediated by STAT3 are essential for ferroptosis. Importantly, integrin ITGA6-and ITGB4-mediated STAT3 activation enhances cell survival in response to erastin, which shows that different pathways can converge on STAT3 to induce ferroptosis (Gao et al., 2018). RSL3-or erastin-induced ROS generation is inhibited by lysosome inhibitors, which implies that ferroptosis is dependent on lysosomal function (Torii et al., 2016).
5 Ferritinophagy is regulated by many factors
5.1 The HERC2-FBXL5-IPR2 axis plays a main role in regulating ferritinophagy
NCOA4, the speed-limiting factor of ferritinophagy, has been proven to be mainly regulated by the cellular iron levels (Mancias et al., 2015). Research with the 293T, U2OS, HCT116, and K562 cell lines shows that NCOA4 binds the ubiquitin E3 ligase HERC2 and is targeted for degradation through the ubiquitin proteasome system when the cellular iron levels are high (Mancias et al., 2015) (Figure 3). This finding ensures that the NCOA4 levels are low under high iron pressure (Yao et al., 2022), which can decrease ferritinophagy and increase ferritin iron storage. In contrast, when cellular iron is low, NCOA4 and HERC2 binding is decreased, causing elevated NCOA4 levels and promoting ferritinophagic flux to restore the cellular iron levels (Ryu et al., 2018). This finding suggests that in the context of long-term NCOA4 depletion in vivo, the sensitivity to ferroptosis may actually be enhanced (De Domenico et al., 2006). In response to a high cellular iron level, HERC2 promotes NCOA4 turnover through the C‐terminal domain in NCOA4 and a CUL7‐homology domain in HERC2, which prevents ferritinophagy and excess liberation of iron from ferritin (Zhou et al., 2020). Additionally, HERC2 regulates the proteasomal degradation of FBXL5, which targets IRP2 for its degradation under iron overload (Moroishi et al., 2014a; Endo et al., 2017). IRP2 plays a significant role in the maintenance of cellular iron homeostasis, and the degradation of IRP2 causes a decrease in Fe2+, which thus increases the flux of ferritinophagy (Yao et al., 2021). The inhibition of HERC2-FBXL5 binding or the knockout of endogenous HERC2 by RNA interference results in the stabilization of FBXL5 and an increase in its abundance (Moroishi et al., 2014a). Thus, HERC2 ubiquitination and the FBXL5‐IRP2 axis play important roles in the NCOA4‐ferritinophagy process and control iron metabolism (Moroishi et al., 2014b), but many unanswered questions, including whether the binding of HERC2 and NCOA4 influences the binding of ferritin and NCOA4, whether other E3 ligases target NCOA4, and whether regulators of HERC2 exist, remain, and focusing on these questions will contribute to the field.
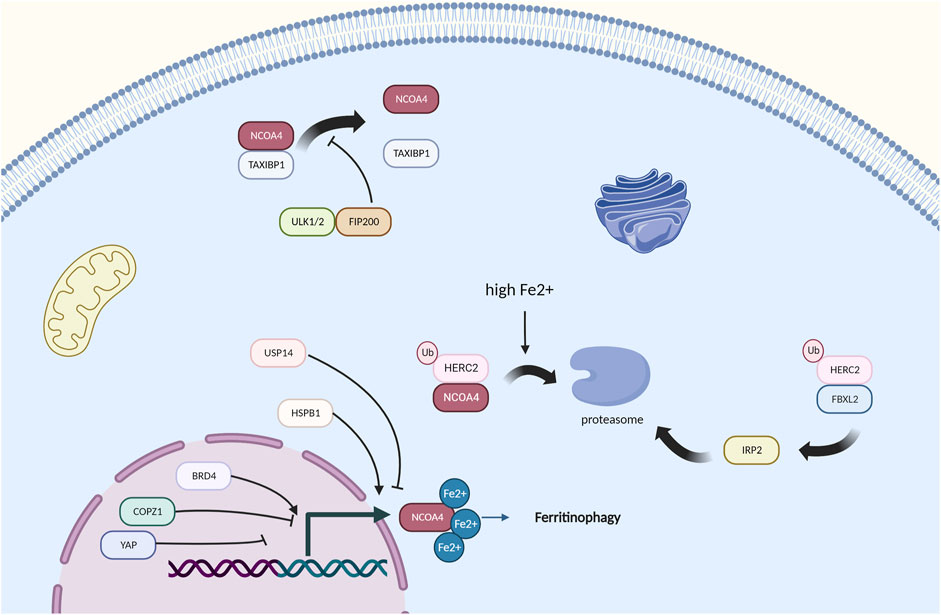
FIGURE 3. Regulators of NCOA4-mediated ferritinophagy, HERC2 and high iron pressure play a main role in the regulation of NCOA4.
5.2 Ferritinophagy regulation by hypoxia
Iron is essential for Fe-S cluster formation and heme synthesis and is therefore critical for maintaining the mitochondrial respiratory chain and the citric acid cycle (Subramanian et al., 2011). However, free iron overload causes severe oxidative damage (Garcia-Bermudez et al., 2018). Under hypoxia, HIF is known to regulate iron regulatory proteins. HIF regulates not only TfR and DMT1, which are responsible for iron import but also FPN, which is the only proven iron exporter (Ma et al., 2019). FTH and FTMT have ferroxidase activity, which allows the storage of Fe3+ instead of reactive Fe2+ (Hilton et al., 2012). FTMT expression is associated with the transport of iron from the cytosol to mitochondria (Campanella et al., 2009). In addition, its expression is dependent on HIF under hypoxia. Macrophages often suffer hypoxia during inflammation and in the tumor microenvironment. Furthermore, they are widely known for their essential role in iron metabolism, although the regulation of macrophage ferritinophagy remains undefined. The overexpression of FTMT protects SH-SY5Y neuroblastoma cells from ferroptosis (Wang et al., 2016; Mendsaikhan et al., 2018). Researchers have found that the increase in FTMT in hypoxic macrophages is related to decreased NCOA4 expression caused by damaged transcriptional regulation under hypoxia, which means that ferritinophagy is directly related to hypoxia (Fuhrmann et al., 2020).
5.3 Identification of more regulators of NCOA4 and ferritinophagy
Wu et al. (2019b) found that curcumol reduces the expression of NCOA4 via Yes-associated protein (YAP), which is a key regulator in the Hippo signaling pathway and affects the formation of autophagosomes (Qi et al., 2021). COPZ1 knockdown also leads to an increase in NCOA4, resulting in ferritin degradation, an increase in intracellular ferrous iron and ultimately ferroptosis (Zhang et al., 2021). The knockdown of NCOA4 could protect cells from ART‐induced cell death, which suggests that ART is a modulator of ferritinophagy (Goodwin et al., 2017). Ferritinophagy requires ATG9A, FIP200, VPS34, and TAX1BP1, and TAX1BP1 directly binds NCOA4 and is needed for the lysosomal trafficking of ferritin under different conditions (Dowdle et al., 2014; Goodwin et al., 2017). NCOA4 is also strongly related to ubiquitination. Coimmunoprecipitation experiments have shown that TRIM11 interacts with and binds UBE2N. Mechanistically, TRIM11 promotes suppression of ferritinophagy and gemcitabine resistance through the UBE2N-TAX1BP1 pathway, and this finding demonstrates that TRIM11 is a key modulator of TAX1BP1 signaling in ferritinophagy and gemcitabine resistance, which has seen treatment effects in pancreatic ductal adenocarcinoma (Dong et al., 2019). USP14 has also been demonstrated to influence NCOA4 and has been proven to improve ischemic stroke (Hangauer et al., 2017). The absence of DNMT-1, which is significant in DNA methylation, has been proven to reduce NCOA-4-mediated ferritinophagy (Li et al., 2021c).
BRD4 and HSPB1 have been found to upregulate ferritinophagy, and BRD4 and HSPB1 are highly expressed in many cancer types (Sui et al., 2019) (Sun et al., 2022). However, how these factors actually work remains unclear. Thus, we wonder whether the targets of selective autophagy mentioned above will interact with NCOA4, and focusing on this topic will be a good research direction.
6 Treatments targeting ferritinophagy in cancer
Cancer cells with dedifferentiated and mesenchymal characteristics have been proven to be more susceptible to ferroptosis than noncancerous cells (Hangauer et al., 2017; Tsoi et al., 2018). Particularly in lung and breast cancer, cells are more sensitive to ferroptosis (Lei et al., 2021). Because the cancer treatments targeting ferroptosis are limited mainly to SLC7A11, treatments focusing on ferritinophagy are receiving increased attention.
Moreover, in HUVECs and EA. hy926 cells, ferritinophagy is needed for the induction of ferroptosis by ZnONPs, which are nanoparticles. Because NCOA4 knockdown alleviates ZnONP-induced cell death (Qin et al., 2021), nanoparticles provide us with an interesting and broader way of thinking.
Apart from nanoparticles, other treatments are still being investigated in the laboratory. DpdtC, a novel iron chelator, was proven to promotes ferritinophagy in HepG2 cells, and this effect has also been verified in gastric cancer (Grignano et al., 2020; Gonciarz et al., 2021). Cancer cells with dedifferentiated and mesenchymal characteristics have been proven to be more susceptible to ferroptosis than noncancerous cells (Hangauer et al., 2017; Tsoi et al., 2018). Tetrandrine citrate has also been shown to suppress breast cancer by activating NCOA4 (Yin et al., 2022). Polybrominated diphenyl ether quinone induces NCOA4-mediated ferritinophagy, and this compound may play a role in subsequent research (Yin et al., 2022). Studies in cancer cells have shown the great potential of ferritinophagic treatments in cancer. But drugs targeting the ferroptosis pathway are still in the preclinical stage, we hope to see more drugs targeting NCOA4 and HERC2 in the future.
7 Future therapies and challenges
Ferritinophagy has become increasingly important in ferroptosis and cancer. In this review, we describe the origin and development of ferroptosis, and the discovery of the relationships between ferroptosis and autophagy has led to the emergence of ferritinophagy. As a part of ferroptosis, this process is regulated by the cellular iron levels and is caused by iron accumulation, and as a part of autophagy, this process mediates the binding of ferritin and autophagosomes. Ferritinophagy is distinguished from other ferroptosis pathways as well as other autophagy pathways, and targeting this process has led to achievements in many types of diseases and will play a more significant role in the discovery of the mechanisms of RCD. Therefore, we note various regulators and treatments for cancer, which show the great potential of ferritinophagy in cancer treatment, and we hope to see more outcomes, which will help us better understand the mechanisms of ferritinophagy in cancer.
Author contributions
KS and CL were in charge of searching all the relative papers and writing this manuscript, CL, XY, YO, ZW, ZL, and YF gave their valuable and professional suggestions and guide in organizing and drafting this manuscript.
Funding
This work was partly supported by a grant from the National Major Scientific Instruments and Equipment Development Projects (No. 2012YQ160203) to FY as well as a National Natural Science Foundation of China (NSFC) grant (No. 82203374) and a grant from the Fundamental Research Funds for the Central Universities (No. 2042022kf1081) to ZW.
Conflict of interest
The authors declare that the research was conducted in the absence of any commercial or financial relationships that could be construed as a potential conflict of interest.
Publisher’s note
All claims expressed in this article are solely those of the authors and do not necessarily represent those of their affiliated organizations, or those of the publisher, the editors and the reviewers. Any product that may be evaluated in this article, or claim that may be made by its manufacturer, is not guaranteed or endorsed by the publisher.
Abbreviations
GPX4, Phospholipid hydroperoxide glutathione peroxidase; System xc-, Polyhydroxyalkanoic acid system protein; BAX, BCL2-associated X protein; BAK, Bcl-2 homologous antagonist; RIPK1/RIPK3, Receptor-interacting serine/threonine-protein kinase1/3; CASP1/4, Caspase-1/4; FSP1, Apoptosis-inducing factor 2; DHODH, Dihydroorotate dehydrogenase; ACSL4, Long-chain fatty acid-CoA ligase 4; GCH1, GTP cyclohydrolase 1; IPLA2β, Inositol 1,4,5-trisphosphate receptor-like protein 2β; MAP1-LC3, Microtubule-associated proteins 1A/1B light chain 3; RAB7A, Ras-related protein Rab-7a; ARNTL, Aryl hydrocarbon receptor nuclear translocator-like protein 1; HIF1A, Hypoxia-inducible factor 1-alpha; PINK1, Serine/threonine-protein kinase PINK1; PARKIN, E3 ubiquitin-protein ligase parkin; HSP90, Heat shock protein 90; BECN1, Beclin-1; HERC2, E3 ubiquitin-protein ligase HERC2; FBXL5, F-box/LRR-repeat protein 5; DMT1, Charged multivesicular body protein 2b; FTMT, Ferritin.
References
Bai, Y., Meng, L., Han, L., Jia, Y., Zhao, Y., Gao, H., et al. (2019). Lipid storage and lipophagy regulates ferroptosis. Biochem. Biophys. Res. Commun. 508 (4), 997–1003. doi:10.1016/j.bbrc.2018.12.039
Bellelli, R., Castellone, M. D., Guida, T., Limongello, R., Dathan, N. A., Merolla, F., et al. (2014). NCOA4 transcriptional coactivator inhibits activation of DNA replication origins. Mol. Cell 55 (1), 123–137. doi:10.1016/j.molcel.2014.04.031
Bellelli, R., Federico, G., Matte', A., Colecchia, D., Iolascon, A., Chiariello, M., et al. (2016). NCOA4 deficiency impairs systemic iron homeostasis. Cell Rep. 14 (3), 411–421. doi:10.1016/j.celrep.2015.12.065
Bersuker, K., Hendricks, J. M., Li, Z., Magtanong, L., Ford, B., Tang, P. H., et al. (2019). The CoQ oxidoreductase FSP1 acts parallel to GPX4 to inhibit ferroptosis. Nature 575 (7784), 688–692. doi:10.1038/s41586-019-1705-2
Bordini, J., Morisi, F., Elia, A. R., Santambrogio, P., Pagani, A., Cucchiara, V., et al. (2020). Iron induces cell death and strengthens the efficacy of antiandrogen therapy in prostate cancer models. Clin. Cancer Res. 26 (23), 6387–6398. doi:10.1158/1078-0432.CCR-20-3182
Campanella, A., Rovelli, E., Santambrogio, P., Cozzi, A., Taroni, F., and Levi, S. (2009). Mitochondrial ferritin limits oxidative damage regulating mitochondrial iron availability: Hypothesis for a protective role in friedreich ataxia. Hum. Mol. Genet. 18 (1), 1–11. doi:10.1093/hmg/ddn308
Chen, D., Tavana, O., Chu, B., Erber, L., Chen, Y., Baer, R., et al. (2017). NRF2 is a major target of ARF in p53-independent tumor suppression. Mol. Cell 68 (1), 224–232. doi:10.1016/j.molcel.2017.09.009
Chen, G. Q., Benthani, F. A., Wu, J., Liang, D., Bian, Z. X., and Jiang, X. (2020). Artemisinin compounds sensitize cancer cells to ferroptosis by regulating iron homeostasis. Cell Death Differ. 27 (1), 242–254. doi:10.1038/s41418-019-0352-3
Chen, X., Li, J., Kang, R., Klionsky, D. J., and Tang, D. (2021). Ferroptosis: Machinery and regulation. Autophagy 17 (9), 2054–2081. doi:10.1080/15548627.2020.1810918
De Domenico, I., Vaughn, M. B., Li, L., Bagley, D., Musci, G., Ward, D. M., et al. (2006). Ferroportin-mediated mobilization of ferritin iron precedes ferritin degradation by the proteasome. EMBO J. 25 (22), 5396–5404. doi:10.1038/sj.emboj.7601409
Dixon, S. J., Lemberg, K. M., Lamprecht, M. R., Skouta, R., Zaitsev, E. M., Gleason, C. E., et al. (2012). Ferroptosis: An iron-dependent form of nonapoptotic cell death. Cell 149 (5), 1060–1072. doi:10.1016/j.cell.2012.03.042
Dodson, M., Castro-Portuguez, R., and Zhang, D. D. (2019). NRF2 plays a critical role in mitigating lipid peroxidation and ferroptosis. Redox Biol. 23, 101107. doi:10.1016/j.redox.2019.101107
Doll, S., Freitas, F. P., Shah, R., Aldrovandi, M., da Silva, M. C., Ingold, I., et al. (2019). FSP1 is a glutathione-independent ferroptosis suppressor. Nature 575 (7784), 693–698. doi:10.1038/s41586-019-1707-0
Doll, S., Proneth, B., Tyurina, Y. Y., Panzilius, E., Kobayashi, S., Ingold, I., et al. (2017). ACSL4 dictates ferroptosis sensitivity by shaping cellular lipid composition. Nat. Chem. Biol. 13 (1), 91–98. doi:10.1038/nchembio.2239
Dong, W., Tan, Y., Qin, Q., Yang, B., Zhu, Q., Xu, L., et al. (2019). Polybrominated diphenyl ethers quinone induces NCOA4-mediated ferritinophagy through selectively autophagic degradation of ferritin. Chem. Res. Toxicol. 32 (12), 2509–2516. doi:10.1021/acs.chemrestox.9b00350
Dowdle, W. E., Nyfeler, B., Nagel, J., Elling, R. A., Liu, S., Triantafellow, E., et al. (2014). Selective VPS34 inhibitor blocks autophagy and uncovers a role for NCOA4 in ferritin degradation and iron homeostasis in vivo. Nat. Cell Biol. 16 (11), 1069–1079. doi:10.1038/ncb3053
Endo, S., Nakata, K., Ohuchida, K., Takesue, S., Nakayama, H., Abe, T., et al. (2017). Autophagy is required for activation of pancreatic stellate cells, associated with pancreatic cancer progression and promotes growth of pancreatic tumors in mice. Gastroenterology 152 (6), 1492–1506. doi:10.1053/j.gastro.2017.01.010
Fang, Y., Chen, X., Tan, Q., Zhou, H., Xu, J., and Gu, Q. (2021). Inhibiting ferroptosis through disrupting the NCOA4-FTH1 interaction: A new mechanism of action. ACS Cent. Sci. 7 (6), 980–989. doi:10.1021/acscentsci.0c01592
Federico, G., Carrillo, F., Dapporto, F., Chiariello, M., Santoro, M., Bellelli, R., et al. (2022). NCOA4 links iron bioavailability to DNA metabolism. Cell Rep. 40 (7), 111207. doi:10.1016/j.celrep.2022.111207
Friedmann Angeli, J. P., Schneider, M., Proneth, B., Tyurina, Y. Y., Tyurin, V. A., Hammond, V. J., et al. (2014). Inactivation of the ferroptosis regulator Gpx4 triggers acute renal failure in mice. Nat. Cell Biol. 16 (12), 1180–1191. doi:10.1038/ncb3064
Fuhrmann, D. C., Mondorf, A., BeifuB, J., Jung, M., and Brune, B. (2020). Hypoxia inhibits ferritinophagy, increases mitochondrial ferritin, and protects from ferroptosis. Redox Biol. 36, 101670. doi:10.1016/j.redox.2020.101670
Gao, H., Bai, Y., Jia, Y., Zhao, Y., Kang, R., Tang, D., et al. (2018). Ferroptosis is a lysosomal cell death process. Biochem. Biophys. Res. Commun. 503 (3), 1550–1556. doi:10.1016/j.bbrc.2018.07.078
Gao, M., Monian, P., Pan, Q., Zhang, W., Xiang, J., and Jiang, X. (2016). Ferroptosis is an autophagic cell death process. Cell Res. 26 (9), 1021–1032. doi:10.1038/cr.2016.95
Gao, M., Yi, J., Zhu, J., Minikes, A. M., Monian, P., Thompson, C. B., et al. (2019). Role of mitochondria in ferroptosis. Mol. Cell 73 (2), 354–363. doi:10.1016/j.molcel.2018.10.042
Garcia-Bermudez, J., Baudrier, L., La, K., Zhu, X. G., Fidelin, J., Sviderskiy, V. O., et al. (2018). Aspartate is a limiting metabolite for cancer cell proliferation under hypoxia and in tumours. Nat. Cell Biol. 20 (7), 775–781. doi:10.1038/s41556-018-0118-z
Gaschler, M. M., Andia, A. A., Liu, H., Csuka, J. M., Hurlocker, B., Vaiana, C. A., et al. (2018). FINO2 initiates ferroptosis through GPX4 inactivation and iron oxidation. Nat. Chem. Biol. 14 (5), 507–515. doi:10.1038/s41589-018-0031-6
Gonciarz, R. L., Collisson, E. A., and Renslo, A. R. (2021). Ferrous iron-dependent Pharmacology. Trends Pharmacol. Sci. 42 (1), 7–18. doi:10.1016/j.tips.2020.11.003
Goodwin, J. M., Dowdle, W. E., DeJesus, R., Wang, Z., Bergman, P., Kobylarz, M., et al. (2017). Autophagy-independent lysosomal targeting regulated by ULK1/2-fip200 and ATG9. Cell Rep. 20 (10), 2341–2356. doi:10.1016/j.celrep.2017.08.034
Grignano, E., Birsen, R., Chapuis, N., and Bouscary, D. (2020). From iron chelation to overload as a therapeutic strategy to induce ferroptosis in leukemic cells. Front. Oncol. 10, 586530. doi:10.3389/fonc.2020.586530
Hambright, W. S., Fonseca, R. S., Chen, L., Na, R., and Ran, Q. (2017). Ablation of ferroptosis regulator glutathione peroxidase 4 in forebrain neurons promotes cognitive impairment and neurodegeneration. Redox Biol. 12, 8–17. doi:10.1016/j.redox.2017.01.021
Hangauer, M. J., Viswanathan, V. S., Ryan, M. J., Bole, D., Eaton, J. K., Matov, A., et al. (2017). Drug-tolerant persister cancer cells are vulnerable to GPX4 inhibition. Nature 551 (7679), 247–250. doi:10.1038/nature24297
Hill, B. G., Haberzettl, P., Ahmed, Y., Srivastava, S., and Bhatnagar, A. (2008). Unsaturated lipid peroxidation-derived aldehydes activate autophagy in vascular smooth-muscle cells. Biochem. J. 410 (3), 525–534. doi:10.1042/BJ20071063
Hilton, R. J., David Andros, N., and Watt, R. K. (2012). The ferroxidase center is essential for ferritin iron loading in the presence of phosphate and minimizes side reactions that form Fe(III)-phosphate colloids. Biometals 25 (2), 259–273. doi:10.1007/s10534-011-9500-z
Hou, W., Xie, Y., Song, X., Sun, X., Lotze, M. T., Zeh, H. J., et al. (2016). Autophagy promotes ferroptosis by degradation of ferritin. Autophagy 12 (8), 1425–1428. doi:10.1080/15548627.2016.1187366
Hu, K., Li, K., Lv, J., Feng, J., Chen, J., Wu, H., et al. (2020). Suppression of the SLC7A11/glutathione axis causes synthetic lethality in KRAS-mutant lung adenocarcinoma. J. Clin. Invest. 130 (4), 1752–1766. doi:10.1172/JCI124049
Jacobsen, A. V., Lowes, K. N., Tanzer, M. C., Lucet, I. S., Hildebrand, J. M., Petrie, E. J., et al. (2016). HSP90 activity is required for MLKL oligomerisation and membrane translocation and the induction of necroptotic cell death. Cell Death Dis. 7, e2051. doi:10.1038/cddis.2015.386
Jiang, L., Kon, N., Li, T., Wang, S. J., Su, T., Hibshoosh, H., et al. (2015). Ferroptosis as a p53-mediated activity during tumour suppression. Nature 520 (7545), 57–62. doi:10.1038/nature14344
Kang, R., and Tang, D. (2017). Autophagy and ferroptosis - what's the connection? Curr. Pathobiol. Rep. 5 (2), 153–159. doi:10.1007/s40139-017-0139-5
Kang, R., Zeh, H. J., Lotze, M. T., and Tang, D. (2011). The Beclin 1 network regulates autophagy and apoptosis. Cell Death Differ. 18 (4), 571–580. doi:10.1038/cdd.2010.191
Kang, R., Zhu, S., Zeh, H. J., Klionsky, D. J., and Tang, D. (2018). BECN1 is a new driver of ferroptosis. Autophagy 14 (12), 2173–2175. doi:10.1080/15548627.2018.1513758
Kaur, J., and Debnath, J. (2015). Autophagy at the crossroads of catabolism and anabolism. Nat. Rev. Mol. Cell Biol. 16 (8), 461–472. doi:10.1038/nrm4024
Kong, Z., Liu, R., and Cheng, Y. (2019). Artesunate alleviates liver fibrosis by regulating ferroptosis signaling pathway. Biomed. Pharmacother. 109, 2043–2053. doi:10.1016/j.biopha.2018.11.030
Koppula, P., Zhuang, L., and Gan, B. (2021). Cystine transporter slc7a11/xCT in cancer: Ferroptosis, nutrient dependency, and cancer therapy. Protein Cell 12 (8), 599–620. doi:10.1007/s13238-020-00789-5
Kraft, V. A. N., Bezjian, C. T., Pfeiffer, S., Ringelstetter, L., Muller, C., Zandkarimi, F., et al. (2020). GTP cyclohydrolase 1/tetrahydrobiopterin counteract ferroptosis through lipid remodeling. ACS Cent. Sci. 6 (1), 41–53. doi:10.1021/acscentsci.9b01063
Kroemer, G., and Jaattela, M. (2005). Lysosomes and autophagy in cell death control. Nat. Rev. Cancer 5 (11), 886–897. doi:10.1038/nrc1738
Krohne, T. U., Stratmann, N. K., Kopitz, J., and Holz, F. G. (2010). Effects of lipid peroxidation products on lipofuscinogenesis and autophagy in human retinal pigment epithelial cells. Exp. Eye Res. 90 (3), 465–471. doi:10.1016/j.exer.2009.12.011
Lahiri, V., Hawkins, W. D., and Klionsky, D. J. (2019). Watch what you (self-) eat: Autophagic mechanisms that modulate metabolism. Cell Metab. 29 (4), 803–826. doi:10.1016/j.cmet.2019.03.003
Lambeth, J. D., and Neish, A. S. (2014). Nox enzymes and new thinking on reactive oxygen: A double-edged sword revisited. Annu. Rev. Pathol. 9, 119–145. doi:10.1146/annurev-pathol-012513-104651
Lanneau, D., de Thonel, A., Maurel, S., Didelot, C., and Garrido, C. (2007). Apoptosis versus cell differentiation: Role of heat shock proteins HSP90, HSP70 and HSP27 Prion 1 (1), 53–60. doi:10.4161/pri.1.1.4059
Lazarou, M., Sliter, D. A., Kane, L. A., Sarraf, S. A., Wang, C., Burman, J. L., et al. (2015). The ubiquitin kinase PINK1 recruits autophagy receptors to induce mitophagy. Nature 524 (7565), 309–314. doi:10.1038/nature14893
Lee, J., You, J. H., Kim, M. S., and Roh, J. L. (2020). Epigenetic reprogramming of epithelial-mesenchymal transition promotes ferroptosis of head and neck cancer. Redox Biol. 37, 101697. doi:10.1016/j.redox.2020.101697
Lei, G., Zhang, Y., Hong, T., Zhang, X., Liu, X., Mao, C., et al. (2021). Ferroptosis as a mechanism to mediate p53 function in tumor radiosensitivity. Oncogene 40 (20), 3533–3547. doi:10.1038/s41388-021-01790-w
Li, C., Zhang, Y., Liu, J., Kang, R., Klionsky, D. J., and Tang, D. (2021). Mitochondrial DNA stress triggers autophagy-dependent ferroptotic death. Autophagy 17 (4), 948–960. doi:10.1080/15548627.2020.1739447
Li, D., Xu, T., Cao, Y., Wang, H., Li, L., Chen, S., et al. (2015). A cytosolic heat shock protein 90 and cochaperone CDC37 complex is required for RIP3 activation during necroptosis. Proc. Natl. Acad. Sci. U. S. A. 112 (16), 5017–5022. doi:10.1073/pnas.1505244112
Li, J., Liu, J., Xu, Y., Wu, R., Chen, X., Song, X., et al. (2021). Tumor heterogeneity in autophagy-dependent ferroptosis. Autophagy 17 (11), 3361–3374. doi:10.1080/15548627.2021.1872241
Li, W., Wang, Y., Leng, Y., and Xia, Z. (2021). Inhibition of DNMT-1 alleviates ferroptosis through NCOA4 mediated ferritinophagy during diabetes myocardial ischemia/reperfusion injury. Cell Death Discov. 7 (1), 267. doi:10.1038/s41420-021-00656-0
Lim, J. K. M., Delaidelli, A., Minaker, S. W., Zhang, H. F., Colovic, M., Yang, H., et al. (2019). Cystine/glutamate antiporter xCT (SLC7A11) facilitates oncogenic RAS transformation by preserving intracellular redox balance. Proc. Natl. Acad. Sci. U. S. A. 116 (19), 9433–9442. doi:10.1073/pnas.1821323116
Liu, J., Yang, M., Kang, R., Klionsky, D. J., and Tang, D. (2019). Autophagic degradation of the circadian clock regulator promotes ferroptosis. Autophagy 15 (11), 2033–2035. doi:10.1080/15548627.2019.1659623
Liu, K., and Czaja, M. J. (2013). Regulation of lipid stores and metabolism by lipophagy. Cell Death Differ. 20 (1), 3–11. doi:10.1038/cdd.2012.63
Liu, W., Chakraborty, B., Safi, R., Kazmin, D., Chang, C. Y., and McDonnell, D. P. (2021). Dysregulated cholesterol homeostasis results in resistance to ferroptosis increasing tumorigenicity and metastasis in cancer. Nat. Commun. 12 (1), 5103. doi:10.1038/s41467-021-25354-4
Liu, Y., Zhai, E., Chen, J., Qian, Y., Zhao, R., Ma, Y., et al. (2022). m(6 A-mediated regulation of PBX1-GCH1 axis promotes gastric cancer proliferation and metastasis by elevating tetrahydrobiopterin levels. Cancer Commun. 42 (4), 327–344. doi:10.1002/cac2.12281
Louandre, C., Ezzoukhry, Z., Godin, C., Barbare, J. C., Maziere, J. C., Chauffert, B., et al. (2013). Iron-dependent cell death of hepatocellular carcinoma cells exposed to sorafenib. Int. J. Cancer 133 (7), 1732–1742. doi:10.1002/ijc.28159
Ma, X., Das, N. K., Castillo, C., Gourani, A., Perekatt, A. O., Verzi, M. P., et al. (2019). SMAD family member 3 (SMAD3) and SMAD4 repress HIF2α-dependent iron-regulatory genes J. Biol. Chem. 294 (11), 3974–3986. doi:10.1074/jbc.RA118.005549
Mancias, J. D., Pontano Vaites, L., Nissim, S., Biancur, D. E., Kim, A. J., Wang, X., et al. (2015). Ferritinophagy via NCOA4 is required for erythropoiesis and is regulated by iron dependent HERC2-mediated proteolysis. Elife 4, 10308. doi:10.7554/eLife.10308
Mancias, J. D., Wang, X., Gygi, S. P., Harper, J. W., and Kimmelman, A. C. (2014). Quantitative proteomics identifies NCOA4 as the cargo receptor mediating ferritinophagy. Nature 509 (7498), 105–109. doi:10.1038/nature13148
Manz, D. H., Blanchette, N. L., Paul, B. T., Torti, F. M., and Torti, S. V. (2016). Iron and cancer: Recent insights. Ann. N. Y. Acad. Sci. 1368 (1), 149–161. doi:10.1111/nyas.13008
Mao, C., Liu, X., Zhang, Y., Lei, G., Yan, Y., Lee, H., et al. (2021). DHODH-mediated ferroptosis defence is a targetable vulnerability in cancer. Nature 593 (7860), 586–590. doi:10.1038/s41586-021-03539-7
Mendsaikhan, A., Takeuchi, S., Walker, D. G., and Tooyama, I. (2018). Differences in gene expression profiles and phenotypes of differentiated SH-SY5Y neurons stably overexpressing mitochondrial ferritin. Front. Mol. Neurosci. 11, 470. doi:10.3389/fnmol.2018.00470
Moosmayer, D., Hilpmann, A., Hoffmann, J., Schnirch, L., Zimmermann, K., Badock, V., et al. (2021). Crystal structures of the selenoprotein glutathione peroxidase 4 in its apo form and in complex with the covalently bound inhibitor ML162. Acta Crystallogr. D. Struct. Biol. 77 (2), 237–248. doi:10.1107/S2059798320016125
Moroishi, T., Yamauchi, T., Nishiyama, M., and Nakayama, K. I. (2014). HERC2 targets the iron regulator FBXL5 for degradation and modulates iron metabolism. J. Biol. Chem. 289 (23), 16430–16441. doi:10.1074/jbc.M113.541490
Moroishi, T., Yamauchi, T., Nishiyama, M., and Nakayama, K. I. (2014). HERC2 targets the iron regulator FBXL5 for degradation and modulates iron metabolism. J. Biol. Chem. 289 (23), 16430–16441. doi:10.1074/jbc.M113.541490
Pietsch, E. C., Chan, J. Y., Torti, F. M., and Torti, S. V. (2003). Nrf2 mediates the induction of ferritin H in response to xenobiotics and cancer chemopreventive dithiolethiones. J. Biol. Chem. 278 (4), 2361–2369. doi:10.1074/jbc.M210664200
Qi, X., Song, A., Ma, M., Wang, P., Zhang, X., Lu, C., et al. (2021). Curcumol inhibits ferritinophagy to restrain hepatocyte senescence through YAP/NCOA4 in non-alcoholic fatty liver disease. Cell Prolif. 54 (9), e13107. doi:10.1111/cpr.13107
Qiang, Z., Dong, H., Xia, Y., Chai, D., Hu, R., and Jiang, H. (2020). Nrf2 and STAT3 alleviates ferroptosis-mediated IIR-ALI by regulating SLC7A11. Oxid. Med. Cell. Longev. 2020, 5146982. doi:10.1155/2020/5146982
Qin, X., Zhang, J., Wang, B., Xu, G., Yang, X., Zou, Z., et al. (2021). Ferritinophagy is involved in the zinc oxide nanoparticles-induced ferroptosis of vascular endothelial cells. Autophagy 17, 4266–4285. doi:10.1080/15548627.2021.1911016
Ryu, M. S., Duck, K. A., and Philpott, C. C. (2018). Ferritin iron regulators, PCBP1 and NCOA4, respond to cellular iron status in developing red cells. Blood Cells Mol. Dis. 69, 75–81. doi:10.1016/j.bcmd.2017.09.009
Santana-Codina, N., and Mancias, J. D. (2018). The role of NCOA4-mediated ferritinophagy in Health and disease. Pharm. (Basel) 11 (4), E114. doi:10.3390/ph11040114
Schroeder, B., Schulze, R. J., Weller, S. G., Sletten, A. C., Casey, C. A., and McNiven, M. A. (2015). The small GTPase Rab7 as a central regulator of hepatocellular lipophagy. Hepatology 61 (6), 1896–1907. doi:10.1002/hep.27667
Senol, E., Ersoy, A., Erdinc, S., Sarandol, E., and Yurtkuran, M. (2008). Oxidative stress and ferritin levels in haemodialysis patients. Nephrol. Dial. Transpl. 23 (2), 665–672. doi:10.1093/ndt/gfm588
Shao, Y., Jia, H., Li, S., Huang, L., Aikemu, B., Yang, G., et al. (2021). Comprehensive analysis of ferroptosis-related markers for the clinical and biological value in gastric cancer. Oxid. Med. Cell. Longev. 2021, 7007933. doi:10.1155/2021/7007933
Shimada, K., Skouta, R., Kaplan, A., Yang, W. S., Hayano, M., Dixon, S. J., et al. (2016). Global survey of cell death mechanisms reveals metabolic regulation of ferroptosis. Nat. Chem. Biol. 12 (7), 497–503. doi:10.1038/nchembio.2079
Shin, D., Kim, E. H., Lee, J., and Roh, J. L. (2018). Nrf2 inhibition reverses resistance to GPX4 inhibitor-induced ferroptosis in head and neck cancer. Free Radic. Biol. Med. 129, 454–462. doi:10.1016/j.freeradbiomed.2018.10.426
Shui, S., Zhao, Z., Wang, H., Conrad, M., and Liu, G. (2021). Non-enzymatic lipid peroxidation initiated by photodynamic therapy drives a distinct ferroptosis-like cell death pathway. Redox Biol. 45, 102056. doi:10.1016/j.redox.2021.102056
Smith, G. L., Srivastava, A. K., Reutovich, A. A., Hunter, N. J., Arosio, P., Melman, A., et al. (2022). Iron mobilization from ferritin in yeast cell lysate and physiological implications. Int. J. Mol. Sci. 23 (11), 6100. doi:10.3390/ijms23116100
Song, X., Zhu, S., Chen, P., Hou, W., Wen, Q., Liu, J., et al. (2018). AMPK-mediated BECN1 phosphorylation promotes ferroptosis by directly blocking system xc(-) activity. Curr. Biol. 28 (15), 2388–2399. doi:10.1016/j.cub.2018.05.094
Soula, M., Weber, R. A., Zilka, O., Alwaseem, H., La, K., Yen, F., et al. (2020). Metabolic determinants of cancer cell sensitivity to canonical ferroptosis inducers. Nat. Chem. Biol. 16 (12), 1351–1360. doi:10.1038/s41589-020-0613-y
Stefely, J. A., and Pagliarini, D. J. (2017). Biochemistry of mitochondrial coenzyme Q biosynthesis. Trends biochem. Sci. 42 (10), 824–843. doi:10.1016/j.tibs.2017.06.008
Stockwell, B. R., Friedmann Angeli, J. P., Bayir, H., Bush, A. I., Conrad, M., Dixon, S. J., et al. (2017). Ferroptosis: A regulated cell death nexus linking metabolism, redox biology, and disease. Cell 171 (2), 273–285. doi:10.1016/j.cell.2017.09.021
Su, Y., Zhao, B., Zhou, L., Zhang, Z., Shen, Y., Lv, H., et al. (2020). Ferroptosis, a novel pharmacological mechanism of anti-cancer drugs. Cancer Lett. 483, 127–136. doi:10.1016/j.canlet.2020.02.015
Subramanian, P., Rodrigues, A. V., Ghimire-Rijal, S., and Stemmler, T. L. (2011). Iron chaperones for mitochondrial Fe-S cluster biosynthesis and ferritin iron storage. Curr. Opin. Chem. Biol. 15 (2), 312–318. doi:10.1016/j.cbpa.2011.01.003
Sui, S., Zhang, J., Xu, S., Wang, Q., Wang, P., and Pang, D. (2019). Ferritinophagy is required for the induction of ferroptosis by the bromodomain protein BRD4 inhibitor (+)-JQ1 in cancer cells. Cell Death Dis. 10 (5), 331. doi:10.1038/s41419-019-1564-7
Sun, W., Yan, J., Ma, H., Wu, J., and Zhang, Y. (2022). Autophagy-dependent ferroptosis-related signature is closely associated with the prognosis and tumor immune escape of patients with glioma. Int. J. Gen. Med. 15, 253–270. doi:10.2147/IJGM.S343046
Sun, W. Y., Tyurin, V. A., Mikulska-Ruminska, K., Shrivastava, I. H., Anthonymuthu, T. S., Zhai, Y. J., et al. (2021). Phospholipase iPLA2β averts ferroptosis by eliminating a redox lipid death signal. Nat. Chem. Biol. 17 (4), 465–476. doi:10.1038/s41589-020-00734-x
Sun, Y., Berleth, N., Wu, W., Schlutermann, D., Deitersen, J., Stuhldreier, F., et al. (2021). Fin56-induced ferroptosis is supported by autophagy-mediated GPX4 degradation and functions synergistically with mTOR inhibition to kill bladder cancer cells. Cell Death Dis. 12 (11), 1028. doi:10.1038/s41419-021-04306-2
Tang, M., Chen, Z., Wu, D., and Chen, L. (2018). Ferritinophagy/ferroptosis: Iron-related newcomers in human diseases. J. Cell. Physiol. 233 (12), 9179–9190. doi:10.1002/jcp.26954
Thomas, C., Mackey, M. M., Diaz, A. A., and Cox, D. P. (2009). Hydroxyl radical is produced via the Fenton reaction in submitochondrial particles under oxidative stress: Implications for diseases associated with iron accumulation. Redox Rep. 14 (3), 102–108. doi:10.1179/135100009X392566
Torii, S., Shintoku, R., Kubota, C., Yaegashi, M., Torii, R., Sasaki, M., et al. (2016). An essential role for functional lysosomes in ferroptosis of cancer cells. Biochem. J. 473 (6), 769–777. doi:10.1042/BJ20150658
Tsoi, J., Robert, L., Paraiso, K., Galvan, C., Sheu, K. M., Lay, J., et al. (2018). Multi-stage differentiation defines melanoma subtypes with differential vulnerability to drug-induced iron-dependent oxidative stress. Cancer Cell 33 (5), 890–904. doi:10.1016/j.ccell.2018.03.017
Ubellacker, J. M., Tasdogan, A., Ramesh, V., Shen, B., Mitchell, E. C., Martin-Sandoval, M. S., et al. (2020). Lymph protects metastasizing melanoma cells from ferroptosis. Nature 585 (7823), 113–118. doi:10.1038/s41586-020-2623-z
Wang, J., Deng, B., Liu, Q., Huang, Y., Chen, W., Li, J., et al. (2020). Pyroptosis and ferroptosis induced by mixed lineage kinase 3 (MLK3) signaling in cardiomyocytes are essential for myocardial fibrosis in response to pressure overload. Cell Death Dis. 11 (7), 574. doi:10.1038/s41419-020-02777-3
Wang, W., Green, M., Choi, J. E., Gijon, M., Kennedy, P. D., Johnson, J. K., et al. (2019). CD8(+) T cells regulate tumour ferroptosis during cancer immunotherapy. Nature 569 (7755), 270–274. doi:10.1038/s41586-019-1170-y
Wang, Y. Q., Chang, S. Y., Wu, Q., Gou, Y. J., Jia, L., Cui, Y. M., et al. (2016). The protective role of mitochondrial ferritin on erastin-induced ferroptosis. Front. Aging Neurosci. 8, 308. doi:10.3389/fnagi.2016.00308
Wang, Y., Quan, F., Cao, Q., Lin, Y., Yue, C., Bi, R., et al. (2021). Quercetin alleviates acute kidney injury by inhibiting ferroptosis. J. Adv. Res. 28, 231–243. doi:10.1016/j.jare.2020.07.007
Wei, J. L., Wu, S. Y., Yang, Y. S., Xiao, Y., Jin, X., Xu, X. E., et al. (2021). GCH1 induces immunosuppression through metabolic reprogramming and Ido1 upregulation in triple-negative breast cancer. J. Immunother. Cancer 9 (7), e002383. doi:10.1136/jitc-2021-002383
Wu, J., Minikes, A. M., Gao, M., Bian, H., Li, Y., Stockwell, B. R., et al. (2019). Intercellular interaction dictates cancer cell ferroptosis via NF2-YAP signalling. Nature 572 (7769), 402–406. doi:10.1038/s41586-019-1426-6
Wu, Z., Geng, Y., Lu, X., Shi, Y., Wu, G., Zhang, M., et al. (2019). Chaperone-mediated autophagy is involved in the execution of ferroptosis. Proc. Natl. Acad. Sci. U. S. A. 116 (8), 2996–3005. doi:10.1073/pnas.1819728116
Xie, Y., Zhu, S., Song, X., Sun, X., Fan, Y., Liu, J., et al. (2017). The tumor suppressor p53 limits ferroptosis by blocking DPP4 activity. Cell Rep. 20 (7), 1692–1704. doi:10.1016/j.celrep.2017.07.055
Yagoda, N., von Rechenberg, M., Zaganjor, E., Bauer, A. J., Yang, W. S., Fridman, D. J., et al. (2007). RAS-RAF-MEK-dependent oxidative cell death involving voltage-dependent anion channels. Nature 447 (7146), 864–868. doi:10.1038/nature05859
Yan, C., Gong, L., Chen, L., Xu, M., Abou-Hamdan, H., Tang, M., et al. (2020). PHB2 (prohibitin 2) promotes PINK1-PRKN/Parkin-dependent mitophagy by the PARL-PGAM5-PINK1 axis. Autophagy 16 (3), 419–434. doi:10.1080/15548627.2019.1628520
Yan, Q., Zhang, W., Lin, M., Teymournejad, O., Budachetri, K., Lakritz, J., et al. (2021). Iron robbery by intracellular pathogen via bacterial effector-induced ferritinophagy. Proc. Natl. Acad. Sci. U. S. A. 118 (23), e2026598118. doi:10.1073/pnas.2026598118
Yan, Y., Liang, Q., Xu, Z., Huang, J., Chen, X., Cai, Y., et al. (2021). Downregulated ferroptosis-related gene STEAP3 as a novel diagnostic and prognostic target for hepatocellular carcinoma and its roles in immune regulation. Front. Cell Dev. Biol. 9, 743046. doi:10.3389/fcell.2021.743046
Yang, W. S., SriRamaratnam, R., Welsch, M. E., Shimada, K., Skouta, R., Viswanathan, V. S., et al. (2014). Regulation of ferroptotic cancer cell death by GPX4. Cell 156 (1-2), 317–331. doi:10.1016/j.cell.2013.12.010
Yang, W. S., and Stockwell, B. R. (2008). Synthetic lethal screening identifies compounds activating iron-dependent, nonapoptotic cell death in oncogenic-RAS-harboring cancer cells. Chem. Biol. 15 (3), 234–245. doi:10.1016/j.chembiol.2008.02.010
Yao, F., Cui, X., Zhang, Y., Bei, Z., Wang, H., Zhao, D., et al. (2021). Iron regulatory protein 1 promotes ferroptosis by sustaining cellular iron homeostasis in melanoma. Oncol. Lett. 22 (3), 657. doi:10.3892/ol.2021.12918
Yao, F., Peng, J., Zhang, E., Ji, D., Gao, Z., Tang, Y., et al. (2022). Pathologically high intraocular pressure disturbs normal iron homeostasis and leads to retinal ganglion cell ferroptosis in glaucoma. Cell Death Differ. Epub ahead of print. doi:10.1038/s41418-022-01046-4
Ye, C. L., Du, Y., Yu, X., Chen, Z. Y., Wang, L., Zheng, Y. F., et al. (2022). STEAP3 affects ferroptosis and progression of renal cell carcinoma through the p53/xCT pathway. Technol. Cancer Res. Treat. 21, 15330338221078728. doi:10.1177/15330338221078728
Ye, Z., Zhuo, Q., Hu, Q., Xu, X., Liu, M., Zhang, Z., et al. (2021). FBW7-NRA41-SCD1 axis synchronously regulates apoptosis and ferroptosis in pancreatic cancer cells. Redox Biol. 38, 101807. doi:10.1016/j.redox.2020.101807
Yin, J., Lin, Y., Fang, W., Zhang, X., Wei, J., Hu, G., et al. (2022). Tetrandrine citrate suppresses breast cancer via depletion of glutathione peroxidase 4 and activation of nuclear receptor coactivator 4-mediated ferritinophagy. Front. Pharmacol. 13, 820593. doi:10.3389/fphar.2022.820593
Zhang, Y., Kong, Y., Ma, Y., Ni, S., Wikerholmen, T., Xi, K., et al. (2021). Loss of COPZ1 induces NCOA4 mediated autophagy and ferroptosis in glioblastoma cell lines. Oncogene 40 (8), 1425–1439. doi:10.1038/s41388-020-01622-3
Zhang, Y., Tan, H., Daniels, J. D., Zandkarimi, F., Liu, H., Brown, L. M., et al. (2019). Imidazole ketone erastin induces ferroptosis and slows tumor growth in a mouse lymphoma model. Cell Chem. Biol. 26 (5), 623–633. doi:10.1016/j.chembiol.2019.01.008
Zhang, Z., Yao, Z., Wang, L., Ding, H., Shao, J., Chen, A., et al. (2018). Activation of ferritinophagy is required for the RNA-binding protein ELAVL1/HuR to regulate ferroptosis in hepatic stellate cells. Autophagy 14 (12), 2083–2103. doi:10.1080/15548627.2018.1503146
Zhao, X. M., Chen, Z., Zhao, J. B., Zhang, P. P., Pu, Y. F., Jiang, S. H., et al. (2016). Hsp90 modulates the stability of MLKL and is required for TNF-induced necroptosis. Cell Death Dis. 7, e2089. doi:10.1038/cddis.2015.390
Zhou, B., Liu, J., Kang, R., Klionsky, D. J., Kroemer, G., and Tang, D. (2020). Ferroptosis is a type of autophagy-dependent cell death. Semin. Cancer Biol. 66, 89–100. doi:10.1016/j.semcancer.2019.03.002
Zhou, L., Chen, J., Li, R., Wei, L., Xiong, H., Wang, C., et al. (2021). Metal-polyphenol-Network coated prussian blue nanoparticles for synergistic ferroptosis and apoptosis via triggered GPX4 inhibition and concurrent in situ bleomycin toxification. Small 17 (47), e2103919. doi:10.1002/smll.202103919
Zhou, R., Yazdi, A. S., Menu, P., and Tschopp, J. (2011). A role for mitochondria in NLRP3 inflammasome activation. Nature 469 (7329), 221–225. doi:10.1038/nature09663
Keywords: ferroptosis, ferritinophagy, cancer, iron, autophagy
Citation: Sun K, Li C, Liao S, Yao X, Ouyang Y, Liu Y, Wang Z, Li Z and Yao F (2022) Ferritinophagy, a form of autophagic ferroptosis: New insights into cancer treatment. Front. Pharmacol. 13:1043344. doi: 10.3389/fphar.2022.1043344
Received: 13 September 2022; Accepted: 12 October 2022;
Published: 21 October 2022.
Edited by:
Lian Xiang Luo, Guangdong Medical University, ChinaReviewed by:
Pengwei Ren, Yale University, United StatesXun Li, University of Maryland, Baltimore, United States
Binsen Li, UCLA Health System, United States
Copyright © 2022 Sun, Li, Liao, Yao, Ouyang, Liu, Wang, Li and Yao. This is an open-access article distributed under the terms of the Creative Commons Attribution License (CC BY). The use, distribution or reproduction in other forums is permitted, provided the original author(s) and the copyright owner(s) are credited and that the original publication in this journal is cited, in accordance with accepted academic practice. No use, distribution or reproduction is permitted which does not comply with these terms.
*Correspondence: Zhong Wang, emhvbmd3YW5nY2huQHdodS5lZHUuY24=; Zhiyu Li, bGl6aGl5dUB3aHUuZWR1LmNu; Feng Yao, eWFvZmVuZ3JtaEAxNjMuY29t
†These authors have contributed equally to this work