- 1School of Pharmacy, Changchun University of Chinese Medicine, Changchun, China
- 2Key Laboratory of Effective Components of Traditional Chinese Medicine, Changchun, China
- 3School of Clinical Medical, Changchun University of Chinese Medicine, Changchun, China
MicroRNAs are small non-coding RNAs that play important roles in gene regulation by influencing the translation and longevity of various target mRNAs and the expression of various target genes as well as by modifying histones and DNA methylation of promoter sites. Consequently, when dysregulated, microRNAs are involved in the development and progression of a variety of diseases, including cancer, by affecting cell growth, proliferation, differentiation, migration, and apoptosis. Preparations from the dried root and rhizome of Salvia miltiorrhiza Bge (Lamiaceae), also known as red sage or danshen, are widely used for treating cardiovascular diseases. Accumulating data suggest that certain bioactive constituents of this plant, particularly tanshinones, have broad antitumor effects by interfering with microRNAs and epigenetic enzymes. This paper reviews the evidence for the antineoplastic activities of S. miltiorrhiza constituents by causing or promoting cell cycle arrest, apoptosis, autophagy, epithelial-mesenchymal transition, angiogenesis, and epigenetic changes to provide an outlook on their future roles in the treatment of cancer, both alone and in combination with other modalities.
Introduction
Salvia miltiorrhiza is a common herbal beverage in Traditional Chinese Medicine (TCM). S. milthiorrhiza belongs to the Lamiaceae family, and its medicinal parts are the roots or rhizomes (Zhang et al., 2022). S. milthiorrhiza has been widely used in TCM for the treatment of cardiovascular disease (CVD) (Li et al., 2018; Ren et al., 2019). For instance, Danshen-Honghua is a commonly used herb pair for promoting blood circulation and removing blood stasis, and S. milthiorrhiza often appears in some TCM compounds used for cardiovascular and cerebrovascular diseases, such as Danhong Huayu Oral Liquid, Danhong Injection, and Xinning Tablets (Wang et al., 2019b).
S. milthiorrhiza improves microcirculation, protects the nervous system, and dilates blood vessels, and it also has antitumor properties (Tao et al., 2018). Research has shown that the compounds in S. milthiorrhiza are mainly divided into two categories as follows: fat-soluble, which mainly include diterpenoids, triterpenoids, flavonoids, nitrogenous compounds, lactones, and polysaccharides; and water-soluble, which mainly include phenolic acids (Su et al., 2015). Diterpenoids mainly include tanshinones, such as tanshinone I (tan I), tanshinone IIA (tan IIA), tanshinone IIB (tan IIB), cryptotanshinone (CPT), dihydrotanshinone I (DHT I), and isocryptotanshinone (Tian et al., 2021). The water-soluble compounds in S. milthiorrhiza are primarily salvianolic acid A (Sal A), salvianolic acid B (Sal B), salvinorin, caffeic acid, rosmarinic acid, and protocatechuic acid (Xin et al., 2020). Most of the triterpenoids are derivatives of oleanolic acid (OA) and ursolic acid (UA) (Tung et al., 2017). The flavonoids in S. milthiorrhiza are mainly luteolin (LUT) and its derivatives (Salinas-Arellano et al., 2020). The polysaccharides in S. milthiorrhiza are mainly composed of mannose, rhamnose, arabinose, glucose, and galactose (Han et al., 2019). The nitrogen-containing compounds that have been isolated from S. milthiorrhiza include neosalvianen, salvianen, and salviadione. In addition, S. milthiorrhiza also contains lactone compounds, such as Dan shen spiroketal lactone (Liang et al., 2020; Wang et al., 2020) (Figure 1). Cancer is a disease that seriously endangers human health. According to the latest data released by the World Health Organization’s International Agency for Research on Cancer (IARC), the number of new cancer cases worldwide in 2020 exceeded 19.3 million, and China ranks 68th in the world in cancer incidence rate (Sung et al., 2021). Many studies have reported that the chemical components in S. milthiorrhiza, such as tan IIA, have good antitumor activity. In addition to inhibiting the proliferation, blocking the cell cycle, inducing apoptosis, and inducing autophagic death of various types of tumor cells, tan IIA also significantly inhibits cell migration and invasion (Cao et al., 2018; Fang et al., 2020). Tan I and LUT also exert antitumor effects through mediating apoptosis and cell cycle arrest (Anson et al., 2018; Li et al., 2018a).
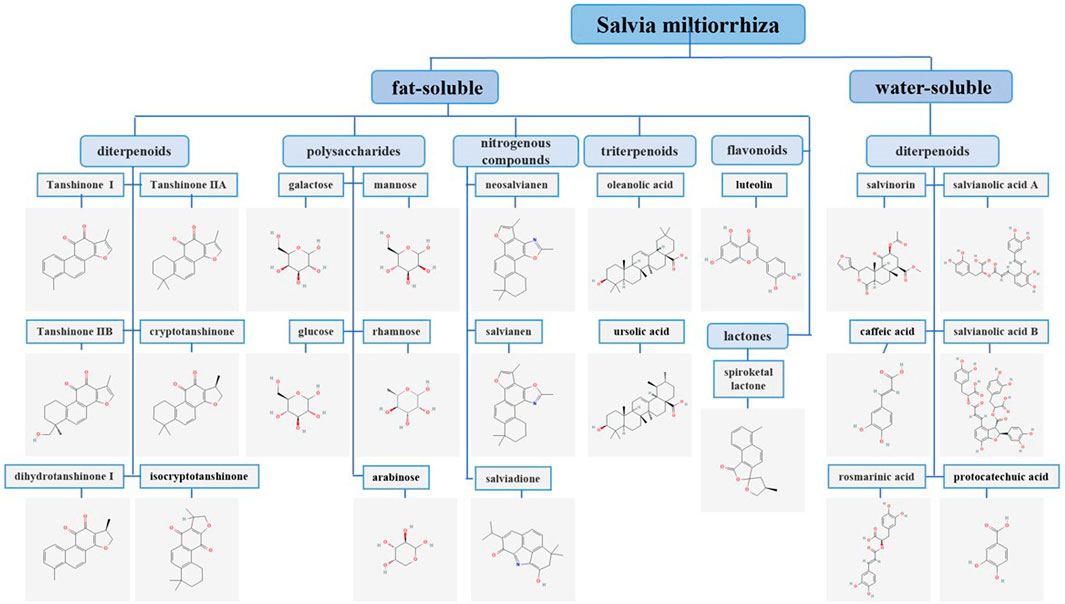
FIGURE 1. Classification and chemical structures of S. miltiorrhiza bioactive compounds. The chemical structures of the S. miltiorrhiza constituents included in this publication were obtained from PubChem and drawn with the ChemDraw program.
Antitumor activities of S. miltiorrhiza
Inhibition of proliferation
S. miltiorrhiza bioactive compounds, such as tan IIA and CPT, effectively inhibit tumor growth (Chen et al., 2014) and suppress tumor cell proliferation in vitro and in vivo (Lin et al., 2015; Zhang et al., 2019c). For example, tan IIA inhibits tumor growth in acute promyelocytic leukemia NB4 cell xenograft mice (Zhang et al., 2016c). Liu et al. (2020) reported that CPT inhibits the phosphatidylinositol-3-kinase (PI3K)/protein kinase B (Akt) pathway by inducing the expression of phosphatase and tensin homologue (PTEN), which represses tumor growth in 5637 cell xenograft mice. Furthermore, tanshinol represses U2-OS tumor growth in zebrafish larvae in a dose-dependent manner (Yu et al., 2022). In addition, previous studies have demonstrated that tan IIA suppresses the proliferation of different types of tumor cells in a time- and dose-dependent manner in vitro, including gastric cancer (GC; SNU-638, MKN1 and AGS cells) (Zhang et al., 2018) and non-small cell lung cancer (NSCLC; A549 and H292 cells) (Qi et al., 2022). Additionally, it has been reported that tan IIA reduces the phosphorylation of insulin-like growth factor 1 receptor (IGF-1R) and its downstream PI3K/Akt and extracellular signal-regulated kinase 1/2 (ERK1/2) pathways, resulting in the suppression of pheochromocytoma growth (PC12 cells) (Wang et al., 2018a). Other tanshinones also inhibit the development of tumors. For example, tan I suppresses colorectal cancer (CRC) cell proliferation by decreasing aurora A-mediated upregulation of p53 or downregulation of survivin expression to induce apoptosis in HCT116 and SW480 cells (Lu et al., 2016). In addition, CPT enhances the cytotoxicity of CD4+ T cells and prevents the development of H446 cells by upregulating the levels of phosphorylated JAK kinase 2 (p-JAK2) and phosphorylated signal transducer and activator of transcription 4 (p-STAT4) (Man et al., 2016). In addition, other components of S. miltiorrhiza have been reported to have antitumor properties. For example, LUT suppresses the viability of RPMI-8226 multiple myeloma cells by enhancing the expression of cleaved caspase 3 and microtubule-associated protein light chain 3 (LC3)-II/I (Chen et al., 2018). LUT also inhibits xenograft tumor growth by reducing Ki-67, p-LIMK, and p-cofilin expression (Zhang et al., 2021). Furthermore, salvianolic acid inhibits tumor cell proliferation. Sal A reduces the viability of NSCLC cells (Jin et al., 2021), and Sal B suppresses cell proliferation via regulating the Akt/mTOR pathway in DDP-resistant GC cells (Wang et al., 2021a).
The cell cycle is the foundation of cell proliferation, and blockade of the cell cycle prevents tumor development. It has been reported that tan IIA inhibits the proliferation of ovarian cancer (Zhou et al., 2020), cervical cancer (Pan et al., 2010), and GC (Chen et al., 2012) cells by arresting the cell cycle in the G2/M phase. In contrast, tan IIA arrests the cell cycle in lung cancer at the G0/G1 phase via upregulating the levels of p53 and p21 as well as downregulating the levels of cyclin D1, cyclin E1, and cyclin-dependent kinase 2 (CDK2) (Lee et al., 2016). Furthermore, tan IIA arrests the cell cycle in prostate cancer in the G0/G1 phase through increasing the expression of p21, p26, and p27 as well as decreasing the expression of cyclin D1, CDK2, and p-Rb (Chiu et al., 2013). Therefore, we propose that the different effects of tan IIA on the cell cycle may be related to cell types. Studies have indicated that other components of S. miltiorrhiza also block the cell cycle at different phases in various tumors cells. For example, LUT inhibits cell proliferation of bladder cancer and lung cancer through arresting the cell cycle in the G2/M phase and G1 phase, respectively (Iida et al., 2020; Zhang et al., 2021). LUT blocks the cell cycle in the G2/M phase through upregulating p21Waf1/Cip1 and p27Kip1 as well as downregulating cyclin A and D1 in bladder cancer T24 cells (Iida et al., 2020). In addition, LUT reduces the expression of cyclin D1 and cyclin D3, thereby inducing cycle arrest of lung cancer cells in the G1 phase (NCI-H1975 and NCI-H1650 cells) (Zhang et al., 2021). Moreover, OA reduces the levels of cyclin D1, CDK1, CDK2, CDK4, and CDK7 but increases the expression of p27, which in turn blocks the cell cycle of HeLa cells in the sub-G1 phase, thereby inhibiting cervical cancer cell growth (Edathara et al., 2021). Moreover, Sal B arrests the cell cycle of A549 cells in the G0/G1 phase by regulating cyclin B1 and p21, thereby repressing A549 cell proliferation (Han et al., 2022). Thus, these findings demonstrate that S. miltiorrhiza bioactive compounds suppress the proliferation of various cancers by driving cell cycle arrest, indicating the potential antitumor usage of S. miltiorrhiza bioactive compounds in the clinic.
Promotion of apoptosis
Apoptosis is an autonomous and orderly cell death process controlled by genes that occurs after cells are stimulated by physiological or pathological signals, and tumors have the ability to resist apoptosis via multiple strategies (Pistritto et al., 2016; Huang et al., 2021a). The antitumor effect of S. miltiorrhiza bioactive compounds has been linked to the induction of apoptosis in multiple studies (Sun et al., 2021). For example, tan IIA induces apoptosis of ovarian cancer A2780 cells by regulating the PI3K/Akt/c-Jun N-terminal kinase (JNK) signaling pathway, which increases the expression of caspase 3, caspase 8, and caspase 9 but decreases the expression of B cell lymphoma 2 (Bcl-2) family proteins (Bcl-w and Bcl-1L) (Zhang et al., 2019a). Tan IIA has also been shown to promote tumor necrosis factor (TNF)-related apoptosis-inducing ligand (TRAIL)-induced apoptosis in glioblastoma by upregulating death receptor 5 (DR5) and blocking STAT3-mediated survivin downregulation (Zhou et al., 2021). Moreover, tan I induces leukemia cell apoptosis via inactivation of the PI3K/Akt/survivin signaling pathway, which disrupts the membrane potential, increases Bcl-2-related X (Bax) expression, and activates caspase 3 (Liu et al., 2010). Tanshinone analog 2-((Glycine methyl ester) methyl)-naphtho (TC7) induces cell apoptosis by regulating apoptosis-associated genes, such as p53, ERK1, Bax, p38, Bcl-2, caspase 8, cleaved caspase 8, and poly (ADP-ribose) polymerase 1 (PARP1), as well as the phosphorylation levels of ERK1 and p38 (Wang et al., 2019). Additionally, LUT has been reported to promote apoptosis in a variety of tumor cells, including ovarian teratomas (Liu et al., 2019) and melanomas (Yao et al., 2019), by decreasing Bcl-2 expression, increasing Bax expression, and inhibiting the PI3K/Akt signaling pathway. Moreover, Sal B induces osteosarcoma MG63 cell apoptosis by increasing the expression of cleaved caspase 3, phosphorylated-p38 mitogen-activated protein kinase (p-p38 MAPK), and phosphorylated-p53 (p-p53) (Zeng et al., 2018).
S. miltiorrhiza bioactive compounds induce apoptosis of various tumor cells through the mitochondrial pathway (Ye et al., 2017). Tan IIA regulates mitochondrial fission through the JNK/mitochondrial fission factor (MFF) axis, which in turn upregulates mitochondrial pro-apoptotic proteins (Bax and Bad), activates caspase 9, and leads to colon cancer SW837 cell apoptosis (Jieensinue et al., 2018). Reactive oxygen species (ROS) interact with membrane receptors and transcription factors/repressors to activate endogenous and exogenous apoptotic signaling pathways, thereby inducing apoptosis (Su et al., 2019). DHT provokes mitochondrial dysfunction in the early stage by decreasing mitochondrial membrane permeability (MMP) and ROS levels, leading to cell death in colon cancer cells (Wang et al., 2013). Moreover, DHT I stimulates the release of both cytochrome c and apoptosis inducing factor (AIF) in HCT116 cells, which downregulates Bcl-xl and upregulates Bax, thereby inducing apoptosis (Wang et al., 2015). Another S. miltiorrhiza component, LUT, promotes apoptosis in canine osteosarcoma cells by generating ROS, increasing loss of MMP, and reducing cytosolic Ca2+ concentration (Ryu et al., 2019). In addition, CPT upregulates Bax and cleaved caspase3 but downregulates the anti-apoptotic protein, Bcl-2, which reduces the level of ROS, thereby inducing apoptosis in human melanoma A375 cells (Ye et al., 2016). Furthermore, Sal B induces mitochondria-mediated apoptosis in human colon cancer cells by reducing the mitochondrial potential and increasing mitochondrial cytochrome c release (Gong et al., 2016). Collectively, inducing mitochondria-dependent apoptosis is one of the important ways for S. miltiorrhiza bioactive compounds to exert an antitumor effect.
Inhibition of migration and invasion
Metastasis and invasion are the main manifestations of tumor malignancy, and they are key factors affecting cancer prognosis (Suhail et al., 2019). Tumor cell invasion and metastasis are complicated biological processes that involve the interplay of many growth factors, cytokines, enzyme systems, matrix metalloproteinases, signaling pathways, and epithelial-mesenchymal transition (EMT) (Patriarca et al., 2020). Many studies have reported that numerous S. miltiorrhiza bioactive compounds affect tumor metastasis and invasion via regulating angiogenesis, regulating tumor cell EMT, and blocking matrix metalloproteinases from hydrolyzing the basement membrane (Zhang et al., 2012). For instance, tan IIA blocks the nuclear factor kappa-B (NF-κB) signaling pathway to inhibit the activity of MMP2 and MMP9, thereby preventing the metastasis and invasion of human hepatoma cells and hepatocellular carcinoma (HCC) cells (Shan et al., 2009; Yuxian et al., 2009; Zhang et al., 2016d).
New blood vessels in tumor tissue provide a source of nutrients for cell proliferation and are also a channel for tumor cell migration (Jiang et al., 2020). Thus, inhibiting tumor angiogenesis has become one of the current antitumor strategies (Fu et al., 2020). Rapid tumor cell proliferation generates hypoxia in local tumor tissue, which activates and upregulates hypoxia-inducible factor 1α (HIF-1α), resulting in the upregulation of the expression of pro-angiogenic factors, such as vascular endothelial growth factor (VEGF), to promote angiogenesis (Keeley et al., 2010). VEGF is a potent angiogenic factor that increases vascular permeability, enabling endothelial cell migration and inducing angiogenesis (Melincovici et al., 2018). Many components of S. miltiorrhiza have been demonstrated to suppress tumor cell invasion and metastasis via regulating HIF-1α- and VEGF- mediated signaling pathways (Macklin et al., 2017). Tan IIA, tan I, and LUT inhibit the secretion of VEGF under hypoxia by downregulating HIF-1α expression, further inhibiting the growth of new blood vessels and the metastasis and invasion of HCC cells, CRC cells, breast cancer cells, human endothelial progenitor cells, and human umbilical vein endothelial cells (Nizamutdinova et al., 2008; Li et al., 2015; Lee et al., 2017; Sui et al., 2017; Fang et al., 2018; Li et al., 2019; Xue et al., 2019; Zhou et al., 2020c).
During EMT, cells transition from an epithelial state to a mesenchymal state, enabling tumor cells to invade and metastasize (Pastushenko and Blanpain 2019). In the EMT process, epithelial proteins are up- or downregulated, and these proteins are identified as EMT markers. The occurrence of EMT is indicated by decreased E-cadherin and keratin as well increased mesenchymal markers, including vimentin, fibronectin, N-cadherin, and α-SMA (Zeisberg and Neilson 2009). Salvinorin and DHT prevent EMT in colon cancer cells and triple-negative breast cancer (TNBC) cells by increasing E-cadherin levels and decreasing N-cadherin and vimentin levels (Kashyap et al., 2021; Cao et al., 2022).
Numerous studies have shown that S. miltiorrhiza bioactive compounds reverse the levels of EMT markers through a complex pathway, including transcription factors, which inhibit the EMT process in tumor cells (Pastushenko and Blanpain 2019). TGF-β is a key factor in inducing EMT in the process of tumor cell proliferation, and elevated levels of TGF-β regulate the expression of EMT markers, which in turn induces EMT (Ali et al., 2015). It has been reported that Sal B and Sal A inhibit the EMT of renal tubular epithelial cells and endothelial cells, respectively, by inhibiting the expression of TGF-β and reversing the expression of EMT markers (Wang et al., 2010; Zhao et al., 2017). YAP/TAZ, a co-activating transcription factor, translocates into the nucleus and binds to transcription factors, such as Twist and Snail, to promote gene transcription (Noguchi et al., 2018; Ma et al., 2019). LUT inhibits the EMT in TNBC cells by inhibiting the transcription activity of YAP/TAZ as well as preventing it from entering the nucleus, further upregulating the protein levels of E-cadherin and downregulating the protein levels of fibronectin, N-cadherin, and vimentin (Cao et al., 2020).
In addition, β-catenin, a cell adhesion regulator, combines with E-cadherin protein on the cell membrane to attach it to the actin cytoskeletal protein, thereby increasing cell adhesion (Shang et al., 2017). GSK-3β is an evolutionarily conserved serine/threonine (S/T) kinase that phosphorylates and degrades cytoplasmic β-catenin, which is regulated by β-arrestin1 and the PI3K/Akt pathway (Yu et al., 2021). Studies have shown that tan IIA increases the expression of GSK-3β by inhibiting the β-arrestin1 and PI3K/Akt signaling pathways, further reducing the nuclear localization of β-catenin and blocking the EMT in CRC and HCC cells (Zhang et al., 2019; Song et al., 2020). Moreover, adhesion factors, such as CCL2, are critical in the EMT process, and the reduction of adhesion factor expression leads to reduced adhesion between transitional cells and adjacent epithelial cells, which causes epithelial cells to lose apical basal cell polarity, thereby promoting the EMT process (Jin 2020; Zou et al., 2020). Studies have shown that tan IIA suppresses the EMT in bladder cancer cells by downregulating CCL2 via blocking the STAT3 pathway (Huang et al., 2017) (Figure 2).
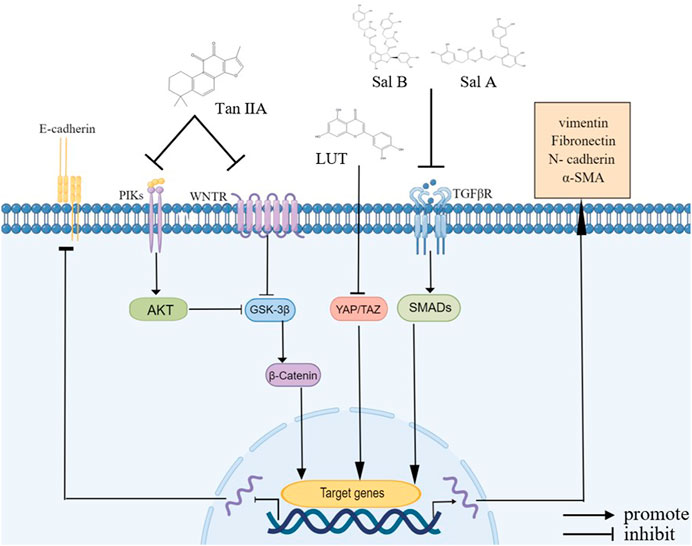
FIGURE 2. Bioactive compounds of S. miltiorrhiza involved in EMT regulation. Tan IIA, LUT, Sal A, and Sal B modulate various signaling pathways, including the NF-κB signaling pathway, VEGF signaling pathway, β-catenin signaling pathway, and PI3K/Akt signaling pathway, and they upregulate E-cadherin and downregulate N-cadherin, α-SMA, vimentin, and fibronectin.
In conclusion, S. miltiorrhiza bioactive compounds inhibit tumor cell metastasis and invasion through different pathways, including inhibiting the activity of MMP proteins, tumor angiogenesis, and the EMT process.
Regulation of autophagy
Autophagy is a type of programmed cell death, and it is a target in cancer treatment (Rodrigues et al., 2020). Investigations have revealed that S. miltiorrhiza bioactive compounds, such as tan IIA, tan I, CPT, DHT, and LUT, induce autophagy, thereby inhibiting cancer cell proliferation (Fu et al., 2020a).
One of the critical pathways to induce autophagy is the conversion of the soluble form of microtubule-associated protein light chain 3-I (LC3-I) to lipid-soluble LC3-II, which is involved in the formation of autophagosomes, thereby inducing autophagy (Wu et al., 2015). In vitro experiments have shown that CPT and DHT induce autophagy by increasing the accumulation of LC3-II in CRC, which induces tumor cell death (Hu et al., 2015).
In addition, the coordinated action of the unc-51-like kinase 1 (ULK1) complex and Beclin 1 functions in driving autophagy (Russell et al., 2013). Beclin 1 is one of the key regulators of autophagy, which participates in the initiation of autophagosome formation, thereby promoting autophagy (Funderburk et al., 2010). For example, LUT induces autophagy in lung cancer cells, HCC cells, and skin squamous cell carcinoma cells by upregulating Beclin 1 expression, subsequently inhibiting tumor cell growth (Verschooten et al., 2012; Park et al., 2013; Potočnjak et al., 2020). Moreover, the ULK1/2 complex is activated in the early stage of autophagy and cooperates with Beclin 1 to initiate autophagosome formation and induce autophagy (Parzych and Klionsky 2014). Furthermore, tan I activates the ULK1 complex and upregulates the expression level of Beclin 1, which subsequently induces autophagy and prevents the growth of breast and liver cancer cells (Zheng et al., 2020).
Mechanistic target of rapamycin (mTOR) is a S/T kinase, which exists in two distinct complexes called mTOR complex 1 (mTORC1) and 2 (mTORC2), and mTORC1 is a key regulator of autophagy (Hua et al., 2019). The activity of mTORC1 reflects the nutritional status of the cell (Kim et al., 2011). Under nutrient-rich conditions, mTORC1 inhibits the autophagy-promoting kinase activity of the ULK1 complex by mediating specific site phosphorylation of ULK1 (Ser637 and Ser757) (Karabiyik et al., 2021). During periods of starvation and cellular stress, mTORC1 activity is inhibited and dissociates from ULK1, resulting in dephosphorylation of ULK1 (Zachari and Ganley 2017). At the same time, the ULK1 complex becomes active through autophosphorylation at Thr180, initiating autophagy (Zachari and Ganley 2017). Studies have shown that tan I inhibits the activity of mTORC1 under starvation conditions, subsequently increasing the activity of the ULK1 complex and promoting the occurrence of autophagy in breast and liver cancer cells (Zheng et al., 2020).
The mTOR pathway is regulated by multiple signaling pathways in autophagy, such as the PI3K/Akt, MAPK/MEK/ERK1/2, and AMPK pathways (Querfurth and Lee 2021). The PI3K/Akt and MAPK/MEK/ERK1/2 signaling pathways have a positive regulatory effect on mTOR, and blocking the above pathways causes autophagy, eventually leading to tumor cell death (Xu et al., 2020a). It has been reported that tan I and tan IIA induce autophagy by inhibiting the PI3K/Akt/mTOR pathway, further inhibiting the proliferation of tumor cells (including ovarian cancer cells, glioma cells, acute promyelocytic leukemia NB4 cells, acute mononuclear leukemia cells, oral squamous cell carcinoma (OSCC) cells, and melanoma cells) (Ding et al., 2017; Li et al., 2017; Qiu et al., 2018; Zhang et al., 2019c; Zhou et al., 2020b; Pan et al., 2021). In addition, tan IIA also drives autophagy through inhibiting the MEK/ERK/mTOR signaling pathway, thereby suppressing the proliferation of colon cancer cells (Qian et al., 2020).
In contrast, the AMPK pathway negatively regulates mTOR. Phosphorylated AMPK inhibits the activity of mTORC1, which indirectly increases the activity of the ULK1 complex, thereby promoting the occurrence of autophagy (Li and Chen 2019). Tan IIA activates AMPK phosphorylation to inhibit mTOR phosphorylation, thereby inducing autophagic death of leukemia cells (Yun et al., 2014). Moreover, under starvation conditions, AMPK activation directly catalyzes the phosphorylation of ULK1 to promote autophagy (Karabiyik et al., 2021). According to a previous study, tan I activates the AMPK signaling pathway to active the ULK1 complex, which subsequently induces autophagy and prevents the growth of breast and liver cancer cells (Zheng et al., 2020).
In summary, tan IIA inhibits the mTOR pathway through different mechanisms and increases the expression of autophagy-related proteins, such as LC3-II and Beclin 1, thereby inducing autophagy in tumor cells (Figure 3).
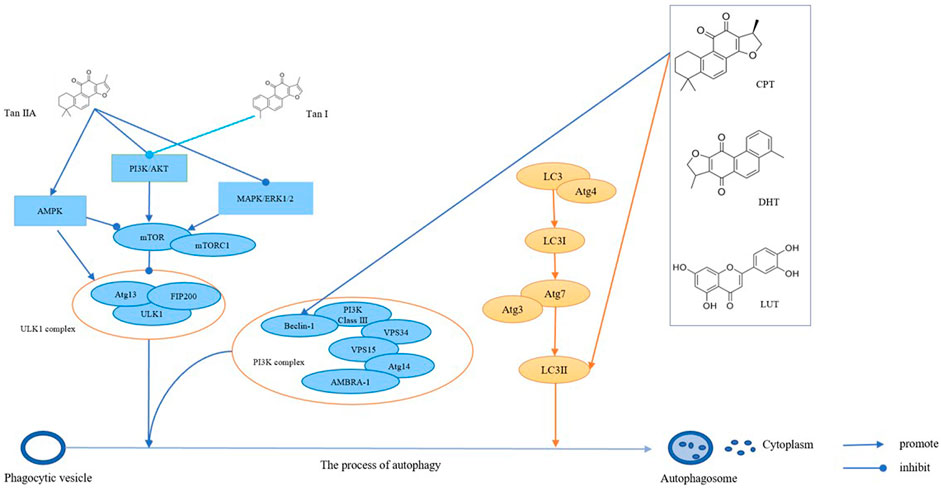
FIGURE 3. The bioactive compounds of S. miltiorrhiza promote autophagy in cancers. Tan IIA and Tan I regulate AMPK signaling, PI3K/AkT signaling, and MAPK/ERK1/2 signaling, thereby regulating mTOR and ULK1 complexes, which promotes the formation of autophagosomes. CPT, DHT, and LUT regulate the PI3K complex and LC3, which in turn promotes autophagosome formation.
Targeting microRNAs (miRNAs)
MiRNAs are a class of non-coding small RNAs with a length of 21–25 nucleotides, and they are widely present in animal and plant cells (Mohr and Mott 2015). MiRNAs bind to the 3′-untranslated region (UTR) of messenger RNA (mRNA) to inhibit mRNA translation or induce mRNA degradation, thereby silencing gene expression at the post-transcriptional level (Lee and Dutta 2009; Wu et al., 2021). In malignant tumors, aberrant amplification, deletion, mutation, and epigenetic silencing of miRNAs have been identified to exert oncogenic or tumor-suppressive effects depending on the target (Rupaimoole and Slack 2017). As an epigenetic modification, miRNAs up- or downregulate the expression of downstream genes through targeting the promoter region of the target gene, thereby mediating the progression of indicated tumors (Ali Syeda et al., 2020). Thus, miRNAs may be used as diagnostic, prognostic, and predictive biomarkers of tumors, and targeting microRNAs may be a potential therapeutic approach for cancers (Iorio and Croce 2012). Studies have demonstrated that S. miltiorrhiza bioactive compounds mediate tumorigenesis by regulating the biological behaviors of miRNAs in cell proliferation, apoptosis, invasion, and metastasis.
Targeting miRNAs to inhibit proliferation
It has been reported that miRNAs target proliferation-related genes and proteins, such as PKM, Akt, and MEF2D, to regulate tumor cell proliferation (Xu et al., 2020). Tan IIA suppresses the proliferation of AML cells (HL-60 and THP-1), glioma cells, esophageal cancer cells (EC109), breast cancer cells, and NSCLC cells through targeting miR-497-5p/Akt3, miR-122/PKM2, miR-16-5p/TLN1, miR-125b/STAR13, and let-7a-5p/aurora kinase A (AURKA), respectively (Zhang et al., 2016; Liu et al., 2020a; Liu et al., 2020; Nie et al., 2020; You et al., 2020; Li et al., 2022). Moreover, tan I, CPT, and tan IIA share common targets, including miR-137 and let-7a-5p, which function in inhibiting the proliferation of NSCLC by regulating the expression of ULK2/IBTK and AURKA, respectively; these common targets may be a result of highly similar chemical structures (Zhang et al., 2016; Liu et al., 2020a). It has been reported that tan I and CPT show inhibitory effects in NSCLC through targeting AURKA mediated by miR-32 (Ma et al., 2015). In addition, studies have reported that CPT inhibits the proliferation of NSCLC cells through targeting miR-146a-5p/EGFR (Qi et al., 2019). Furthermore, UA downregulates miR-499a-5/secreted frizzled related protein 4 (sFRP4) and miR-149-5p/myeloid differentiation primary response 88 (MyD88), thereby inhibiting the proliferation of NSCLC cells (Chen et al., 2020; Mandal et al., 2021). Another active ingredient of salvia, OA, suppresses the proliferation of A549 and GC cells through increasing the expression of miR-122/CCNG1/MEF2D and miR-98-5p/IL-6, respectively (Zhao et al., 2015; Xu et al., 2021). Additionally, studies have shown that LUT regulates the levels of miR-203/Ras/Raf/MEK/ERK, miR-8080/AR-V7 and miR-6809-5p/flotillin 1, which in turn inhibits the proliferation of breast cancer, castration-resistant prostate cancer (CRPC), and HCC, respectively (Gao et al., 2019; Yang et al., 2019; Naiki-Ito et al., 2020).
Researchers have also indicated that abnormal miRNAs promote tumor cell proliferation by regulating the expression of cell cycle-related proteins (Mens and Ghanbari 2018). Studies have shown that UA inhibits the proliferation of BGC-823 cells by upregulating miR-133a and inhibiting Akt1 expression, thereby promoting cell cycle arrest in the G phase (Xiang et al., 2014). Zhang et al. (2016b) reported that tan IIA induces the upregulation of miR-122 expression and inhibits the expression of pyruvate kinase M2 (PKM2) in human EC109 cells, which induces human EC109 tumor cells to arrest in S phase, thereby exerting an anticancer effect (Zhang et al., 2016).
Targeting microRNAs to promote apoptosis
Specific miRNAs directly or indirectly participate in the regulation of multiple apoptotic pathways by regulating the level of downstream genes, and they can play an oncogenic or tumor suppressor role (Bejarano et al., 2021). Tan IIA targets miR-125b/gasdermin D (GSDMD) and miR-145/caspase 1, which induces apoptosis of nasopharyngeal carcinoma (NPC) cells (HK1 cells) and cervical carcinoma cells (HeLa cells), respectively (Tong et al., 2020; Wang et al., 2021c). In addition, tan IIA targets miR30b, further upregulating p53 levels, which induces apoptosis and death of HCC cells (Ren et al., 2017). Studies have found that tan I and UA target miR135a-3p/DR5 (prostate cancer), miR-21 (glioma), and let7b (malignant mesothelioma), which mediates the activation of caspase 3, thereby inducing tumor cell apoptosis and proliferation (Wang et al., 2012; Shin et al., 2014; Sohn et al., 2016). It has been reported that UA increases the level of miR-4500, which suppresses the expression of p-STAT3 and cleaved PARP, thereby inducing CRC cell apoptosis and inhibiting CRC growth (Kim et al., 2018).
Targeting microRNAs to inhibit migration and invasion
Studies have found that miRNAs play an important role in tumor invasion and metastasis by affecting indicated targets and pathways (Wang and Chen 2019). Numerous studies have demonstrated that S. miltiorrhiza bioactive compounds regulate the levels of miRNAs, thereby inhibiting metastasis and invasion of tumor cells. LUT upregulates miR-203/Ras/Raf/MEK/ERK, which upregulates E-cadherin and downregulates N-cadherin, thereby inhibiting the progression of EMT and preventing tumor cell invasion and metastasis of breast cancer (Gao et al., 2019). Furthermore, LUT upregulates miR-384 and subsequently downregulates the expression of multivitamin trophic factor (PTN), which inhibits CRC and osteosarcoma (OS) cell migration and invasion (Yao et al., 2019; Qin et al., 2022). In addition, LUT inhibits migration- and invasion-related factors, including VEGF, MMP2, and MMP9, by upregulating miR-133a-3p levels, thereby suppressing invasion and metastasis of NSCLC (Pan et al., 2022). In addition, UA targets let 7b, which inhibits the expression of p-Akt, β-catenin, and Twist, thereby repressing the EMT process (Sohn et al., 2016).
In summary, S. miltiorrhiza bioactive compounds inhibit tumor cell proliferation, migration, and invasion as well as induce apoptosis by regulating the expression of miRNAs, thereby exerting antitumor effects (Table 1).
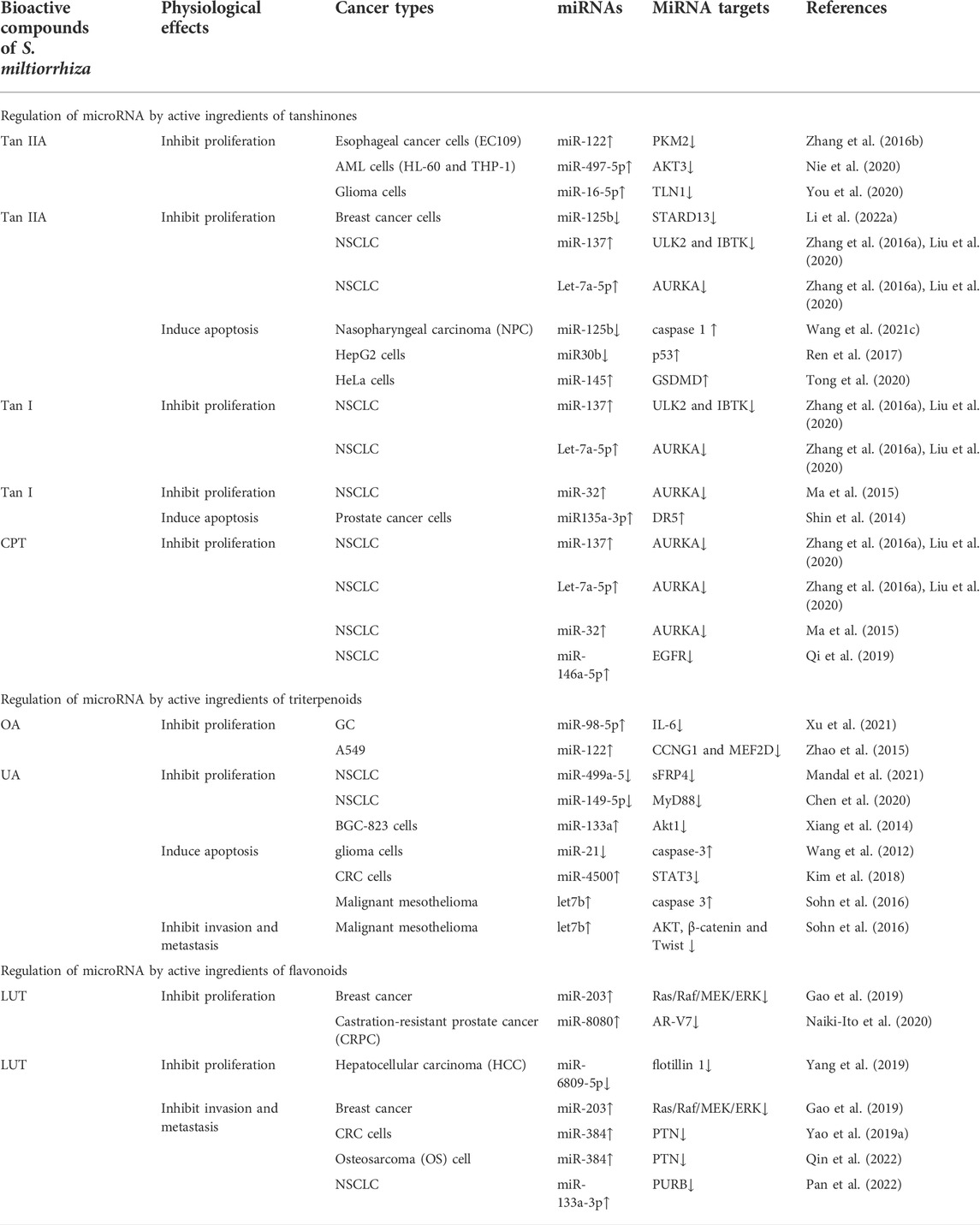
TABLE 1. Regulation of microRNAs by S. miltiorrhiza bioactive compounds in cancer progression inhibition.
Regulation of epigenetic enzymes
Epigenetic mechanisms refer to heritable changes in gene expression without altering the DNA sequence, and they include DNA methylation, chromatin remodeling, histone modifications, and non-coding RNAs (Kanwal et al., 2015; Huang et al., 2021b). Abnormal epigenetic alterations are often involved in tumor initiation, progression, and metastasis (Esteller 2008). S. miltiorrhiza bioactive compounds have been reported to inhibit tumorigenesis and progression by modulating indicated epigenetic mechanisms, including DNA methylation, histone acetylation, ubiquitination, and DNA topoisomerase (Tian et al., 2018; Bhattacharjee and Dashwood 2020). For example, DHT promotes Keap1-mediated degradation of nuclear factor erythroid 2-related factor 2 (Nrf2) ubiquitination, and it increases the accumulation of ROS, which in turn downregulates Bcl-2, cyclin B1, and Cdc2 levels as well as upregulates Bax levels in ovarian cancer cells in vivo and in vitro (Sun et al., 2022).
DNA methylation
In normal cells, DNA methylation occurs mainly in repetitive genomic regions and is responsible for silencing gene expression and maintaining genome stability (Sandoval et al., 2011). However, special DNA regions, such as promoters, especially CpG islands (CpGs), are often unmethylated (Bird 1986). Hypomethylation in tumor cells is mainly due to methylation deficiency in repetitive genomic regions, leading to genomic instability and activation of transposon promoters, whereas hypermethylation is the ab initio methylation of CpGs, which can lead to transcriptional silencing of growth regulatory genes (Lakshminarasimhan and Liang 2016). Cancer cells display genome-wide hypomethylation and site-specific CpG promoter hypermethylation (Rodríguez-Paredes and Esteller 2011). Abnormal increased DNA methylation leads to transcriptional repression and decreased gene expression (Pan et al., 2018). Studies have reported that S. miltiorrhiza bioactive compounds regulate the level of methylation in tumor cells and inhibit tumor development (Kim et al., 2016; Li et al., 2022). For example, tan IIA, LUT, and UA target the abnormal hypermethylation at the CpG methylation region in the Nrf2 promoter by reducing the expression of DNA methyltransferases (DNMTs), including DNMT1, DNMT3a, and DNMT3b, thereby inhibiting cell proliferation of skin and colon cancers (Wang et al., 2014; Kim et al., 2016; Zuo et al., 2018). Moreover, it has been reported that the inhibition of NRF2 promoter methylation is mediated by tan IIA via ten-eleven translocation (TET)-2 activation (Yang et al., 2020). In addition, OA decreases the promoter DNA demethylation of programmed death-ligand 1 (PD-L1) by inhibiting the expression of TET-3, which downregulates PD-L1, ultimately enhancing T cell-mediated killing of MKN-45 cells (Lu et al., 2021). Moreover, it has been reported that Sal B induces DNMT1-mediated demethylation of the PTCH1 gene, which in turn inhibits hepatic stellate cell (HSC) EMT in vivo and in vitro (Yu et al., 2015).
Histone methylation
Histone methylation is a major player in the regulation of gene expression and genetic stability, and dysfunction of histone methylation contributes to the development of various cancers (Rotili and Mai 2011). Methylation occurs primarily on lysine or arginine residues of histones H3 and H4, which is controlled by histone methyltransferases (HMTs) and histone demethylases (HDMs) (Janardhan et al., 2018). Polycomb repressive complex 2 (PRC2) is a complex that mediates gene silencing by regulating chromatin structure (Chan and Morey 2019). Enhancer of zeste homolog 2 (EZH2), a polycomb group (PcG) protein, is an enzymatic catalytic subunit of PRC2 that regulates gene expression through catalyzing primarily tri-methylation of histone H3 Lys-27 (H3K27), and it functions in the development and progression of a variety of cancers, such as breast cancer (Bachmann et al., 2006), GC (Gan et al., 2018), and NPC (Duan et al., 2020). It has been reported that tanshindiol C inhibits EZH2 activity, which in turn downregulates H3K27me3 levels, thereby inhibiting the proliferation of the Pfeiffer cell line, a type of B cell lymphoma (Woo et al., 2014). Furthermore, tan I directly binds to EZH2, which inhibits PRC2 activity, resulting in a decrease in tri-methylated modification of H3K27, and this decrease, in turn, upregulates MMP9 and ABCG2, ultimately inhibiting the proliferation of human leukemia cells in vitro and in vitro (Huang et al., 2021). Moreover, tan I reduces the expression of CCAAT-enhancer-binding protein β (C/EBPβ), which inhibits the mRNA levels of the JMJD2b histone H3K9 demethylase as well as cell cycle-related genes, further regulating the mitotic clonal expansion (MCE) process (Jung et al., 2020). Lysine specific demethylase 1 (LSD1) is a flavin adenine dinucleotide (FAD)-dependent amine oxidase (AO) that catalyzes the demethylation of mono- and di-methyl on H3K4 and H3K9, triggering transcriptional repression and activation, respectively (Karakaidos et al., 2019). Studies have demonstrated that CPT blocks the interaction of LSD1 and androgen receptor (AR) at the promoter of the AR target gene, prostate-specific antigen (PSA), which demethylates H3K9me1/2, thereby inhibiting the transcriptional activity of PSA and suppressing the proliferation of AR-positive prostate cancer cells (Wu et al., 2012). Moreover, tan IIA upregulates the level of H3K27me1 and H3K4m2 on the TP53 promoter by inhibiting LSD1, thereby activating the transcriptional activity of p53 (Zheng et al., 2018). It has been reported that the mRNA and protein levels of p53 are downregulated in several types of tumors, such as prostate cancer and colon cancer (Bossi and Sacchi 2007). Therefore, reactivating p53 is an important strategy for tumor therapy. Taken together, these findings suggest that tan IIA may increase the level of H3K27me1 and H3K4m2 on the p53 promoter by targeting LSD1, which activates p53, thereby promoting tumor cell cycle arrest and apoptosis.
Histone acetylation
Histone acetylation is a reversible and homeostatic process regulated by histone acetyltransferases (HATs) and histone deacetylases (HDACs) (Attoub et al., 2011). Studies have reported abnormal acetylation levels in certain tumors (Zhao and Shilatifard 2019). Histone lysine residues are hyperacetylated in drug-resistant prostate cancer, acute myeloid leukemia, gastrointestinal stromal tumors, lymphomas, and TNBC (He et al., 2021), whereas acetylation levels are decreased in ovarian cancer, colon cancer, liver cancer, and skin cancer (Wu et al., 2020). Overexpression of HDAC is present in tumors with low acetylation levels, indicating that targeting HDAC may be a strategy for treatment of these tumors (Li and Seto 2016). To date, numerous HDAC inhibitors are in various stages of clinical research; vorinostat (SAHA), beristatin (PXD101), and bilestat (LBH589) are approved for the clinical treatment of cutaneous T cell lymphoma, peripheral T cell lymphoma and multiple myeloma, respectively (Ho et al., 2020), and other HDAC inhibitors have been investigated in clinical trials for the treatment of various cancers (Ono et al., 2018; Xie et al., 2018). S. miltiorrhiza bioactive compounds have been found to target histone deacetyltransferases (HDACs) and identified as potential inhibitors of HDACs. Tan IIA suppresses the expression and enzymatic activity of HDAC1, HDAC3, HDAC4, and HDAC8, which increases histone 3 acetylation and RNA polymerase II recruitment to the Nrf2 transcription start site, thereby inhibiting TPA-induced JB6 P+ cell neoplastic transformation (Wang et al., 2014). In contrast, LUT increases the enrichment of H3K27ac in the promoter region of Nrf2 by downregulating HDAC1, HDAC2, HDAC3, HDAC6, and HDAC7 expression and enzymatic activity, further promoting the transcriptional activity of the Nrf2 gene, which in turn inhibits the cellular activity of HCT116 (Zuo et al., 2018).
The level of acetylation is closely related to tumor stage, metastasis, and invasion as well as prognosis (He et al., 2021). Aurora A is a mitotic S/T kinase involved in the process of cell division, and the expression of aurora A is elevated in various tumors (Meraldi et al., 2004). Tan I reduces the level of histone acetylation at the aurora A DNA promoter region, which downregulates aurora A and ultimately inhibits the growth of breast cancer cells (Gong et al., 2012). Furthermore, LUT reduces the level of H3K27ac and H3K56ac at the MMP9 promoter region by inactivating the Akt/mTOR signaling pathway, thereby inhibiting metastasis of AR-positive TNBC (Wu et al., 2021).
Ubiquitination
Ubiquitination is an important post-translational modification mediated by ubiquitin-activating enzymes (E1), ubiquitin-conjugating enzymes (E2), and ubiquitin ligases (E3) (Cockram et al., 2021). Abnormal ubiquitination alters intracellular physiological functions, leading to the initiation and development of cancer (Pellegrino et al., 2022). For example, overexpression of the E3 ligase, mouse double minute 2 (MDM2), mediates ubiquitination of p53 and promotes proteasomal degradation of p53, which inhibits p53 function, leading to tumorigenesis and cancer cell progression (Wang et al., 2017). It has been reported that tan IIA induces ubiquitous degradation of the anti-apoptotic protein, Mcl-1, through targeting the EGFR-Akt signaling pathway, which induces NSCC cell apoptosis (Gao et al., 2020). Tan IIA promotes the FBW7 E3 ligase-mediated ubiquitination degradation of c-Myc, which induces OSCC cell apoptosis (Chen et al., 2020a; Li et al., 2020). Moreover, tan I and DHT activate the Nrf2 pathway by reducing Nrf2 ubiquitination, thereby maintaining redox homeostasis of skin fibroblasts and HBE cells (Tao et al., 2013a; Tao et al., 2013).
In summary, S. miltiorrhiza bioactive compounds suppress tumor growth and development by regulating DNA methylation, histone methylation, acetylation, and ubiquitination to silence tumor suppressors or promote oncogenes (Figure 4).
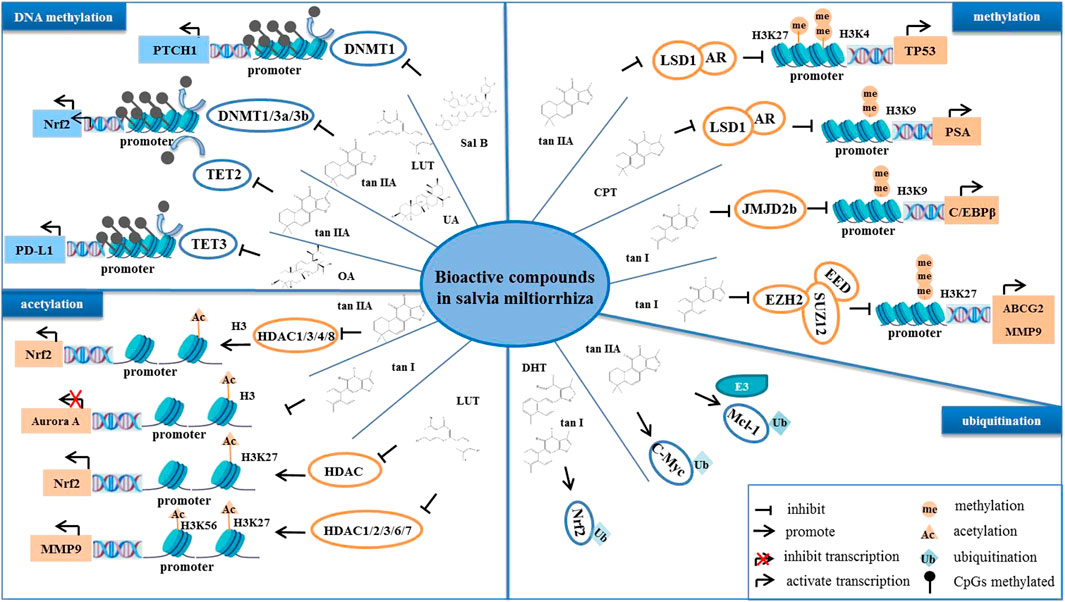
FIGURE 4. The bioactive compounds of S. miltiorrhiza regulate epigenetic modifications. S. miltiorrhiza bioactive compounds suppress the transcriptional activity and enzymatic activity of their target genes by regulating epigenetic modification-related enzymes.
Future prospects
Currently, chemotherapy and radiotherapy are the main methods for the clinical treatment of tumors, but these methods have serious shortcomings, such as resistance and toxic side effects. Thus, combination therapy may be important to improve tumor sensitivity. We previously demonstrated that the bioactive compounds of S. miltiorrhiza inhibit cancer cell proliferation, induce apoptosis, suppress metastasis, and suppress invasion. Thus, we speculate that S. miltiorrhiza bioactive compounds may be used as adjuvant drugs to improve the sensitivity of drug-resistant tumors.
At present, drug resistance is one of the major barriers to cancer treatment, which limits the efficacy of chemotherapy drugs, leading to tumor recurrence, metastasis, and cell death resistance (Haider et al., 2020). Doxorubicin (Dox) and paclitaxel are first-line treatments for patients with metastatic and early-stage breast cancer (Abu Samaan, Samec et al., 2019; Jamialahmadi et al., 2021). However, it has been reported that patients develop resistance to these drugs during the treatment of breast cancer, greatly affecting the efficacy of the drugs (Rivera and Gomez 2010). Moreover, platinum drugs, such as cisplatin and oxaliplatin (OXA), induce DNA damage in tumor cells; they are mainly used in the treatment of ovarian cancer, but their efficacy is severely limited due to drug resistance (Pujade-Lauraine et al., 2019). Studies have found that the bioactive compounds of S. miltiorrhiza have potential roles in reversing resistance and enhancing chemotherapeutic drug sensitivity. For example, tan IIA increases the sensitivity of OXA-resistant CRC cells to OXA by regulating the Akt/ERK signaling pathway, thereby enhancing the anti-proliferation effect and inducing apoptosis in SW480/OXA and HT29/OXA cells (Zhang et al., 2019b). Furthermore, CPT/DHT reduces the P-glycoprotein (P-gp) mRNA and protein levels as well as inhibits P-gp ATPase activity of SW620 Ad300 cells (Hu et al., 2014). Moreover, tan IIA reduces the accumulation of β-catenin in the nucleus by repressing β-catenin nuclear translocation, which enhances Dox inhibition of MCF-7/Dox cell proliferation and migration (Li et al., 2021). Adenosine triphosphate (ATP)-binding cassette (ABC) transporters, such as P-gp, breast cancer resistance protein (BCRP), and multidrug resistance-related protein 1 (MRP1), catalyze the transduction of structurally diverse compounds across cell membranes via ATP-dependent transport (Zahra et al., 2020). Because ABC transporters are overexpressed in tumor cells, they pump out the chemotherapeutic drugs, thereby inducing tumor cells to acquire drug resistance (Choi and Yu 2014). It has been reported that tan IIA downregulates the expression of P-gp, BCRP, and MRP1, further promoting intracellular accumulation of Dox, which induces apoptosis and suppresses proliferation of MCF7/Dox cells, thereby alleviating the inhibitory effect of Dox on the hematopoietic function and Dox-induced cardiotoxicity and nephrotoxicity (Li et al., 2019).
S. miltiorrhiza bioactive compounds not only increase the sensitivity of drug-resistant cells to chemotherapeutic drugs but also synergistically inhibit tumor development. Bai et al. reported that tan IIA combined with 5-FU represses the activation of NF-κB and inhibits CRC cell proliferation (Bai et al., 2016). Furthermore, tan IIA and Dox cotreatment decreases the activity of the VEGF/PI3K/Akt signaling pathway, which promotes apoptosis and induces cell cycle arrest, thereby suppressing proliferation, metastasis, and invasion of A549 cells (Xie et al., 2016). Moreover, tan I and paclitaxel cooperate to promote apoptosis and accelerate cell senescence, thereby inhibiting proliferation and metastasis of ovarian cancer cells (A2780 and ID-8 cells) (Zhou et al., 2020). In addition, the combination of LUT and OXA induces apoptosis and cell cycle arrest as well as inhibits proliferation of SGC-7901 gastric cancer cells (Ren et al., 2020).
Radiotherapy is an effective method of cancer treatment, but the resistance and side effects of radiation therapy affect its efficacy. Therefore, sensitizers that kill tumor cells improve the efficacy of radiotherapy (Chevalier 2020). Tan IIA combined with irradiation reduces histone H3 (S10) phosphorylation in ionizing radiation-resistant cell lines (CAL27-IR and SCC25-IR cells), and this combination enhances irradiation-induced DNA damage and promotes apoptosis, thereby inhibiting tumor growth in vivo and in vitro (Li et al., 2021). Moreover, tan IIA increases radiation-induced ROS production and induces autophagy, which in turn increases the sensitivity of OSCC cells (SCC090 cells) to radiation (Ding et al., 2016). Pro-oncogenic protein phosphoribosyl pyrophosphate aminotransferase is an essential enzyme that catalyzes the first step in purine biosynthesis and promotes the increase in purine metabolism in cancer cells, and its overexpression promotes cancer cell proliferation and invasion. Tan I downregulates the expression of PPAT, which in turn enhances the radiosensitivity of radiation-resistant lung cancer cells (H358-IR and H157-IR cells), thereby inhibiting cell proliferation (Yan et al., 2018).
In summary, the bioactive compounds of S. miltiorrhiza target miRNAs and epigenetic enzymes as well as function to inhibit cancer cell proliferation, induce cell cycle arrest, induce apoptosis, trigger autophagy, suppress metastasis, and suppress invasion (Figure 5). Moreover, S. miltiorrhiza bioactive compounds have multiple roles as adjuvant drugs in chemotherapy and radiotherapy as follows: 1) improve the sensitivity of drug-resistant cells to chemotherapeutic drugs; 2) work together with chemotherapeutic drugs to enhance the therapeutic effect; and 3) combine with radiotherapy to improve the effect of radiotherapy (Table 2). Thus, S. miltiorrhiza bioactive compounds have an antitumor effect and potential application prospects in tumor treatment and adjuvant medicine. In addition, one critical mechanism of S. miltiorrhiza bioactive compounds in suppressing cancer initiation and progression is through targeting miRNAs, including miR-125b, miRNA-122, and miRNA-384, which are present in various cancer cells, suggesting that these miRNAs may play important roles in cancer progression. The pharmacological activity and mechanism of S. miltiorrhiza bioactive compounds are continually being elucidated, thereby supporting their potential application in future clinical cancer therapies.
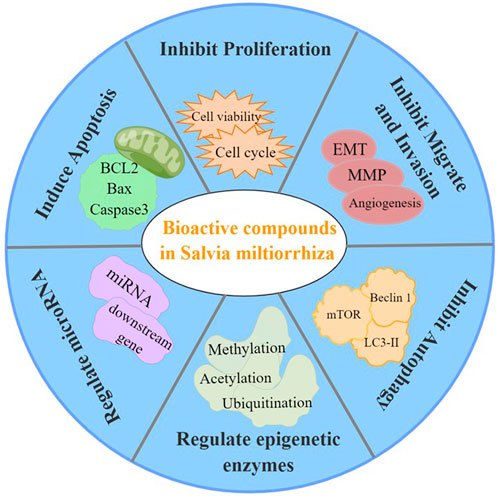
FIGURE 5. Pharmacological activities of S. miltiorrhiza bioactive compounds resulting in antitumor effects.
Author contributions
WG and DW designed the review; XL, ML, XW, XF, YZ, and HL contributed to the writing of the manuscirpt.
Funding
This work was supported by National Natural Science Foundation of China (Grant No. 81903876), Jilin Provincial Department of Education (JJKH20210982KJ), Jilin Province Youth Science and Technology Talent Support Project (2020025).
Acknowledgments
We would like to thank the Charlesworth Author Services (https://www.cwauthors.com.cn) for English language editing.
Conflict of interest
The authors declare that the research was conducted in the absence of any commercial or financial relationships that could be construed as a potential conflict of interest.
Publisher’s note
All claims expressed in this article are solely those of the authors and do not necessarily represent those of their affiliated organizations, or those of the publisher, the editors and the reviewers. Any product that may be evaluated in this article, or claim that may be made by its manufacturer, is not guaranteed or endorsed by the publisher.
References
Abu Samaan, T. M., Samec, M., Liskova, A., Kubatka, P., and Büsselberg, D. (2019). Paclitaxel's mechanistic and clinical effects on breast cancer. Biomolecules 9 (12), E789. doi:10.3390/biom9120789
Ali, A., Zhang, P., Liangfang, Y., Wenshe, S., Wang, H., Lin, X., et al. (2015). KLF17 empowers TGF-β/Smad signaling by targeting Smad3-dependent pathway to suppress tumor growth and metastasis during cancer progression. Cell Death Dis. 6 (3), e1681. doi:10.1038/cddis.2015.48
Ali Syeda, Z., Langden, S. S. S., Munkhzul, C., Lee, M., and Song, S. J. (2020). Regulatory mechanism of MicroRNA expression in cancer. Int. J. Mol. Sci. 21 (5), E1723. doi:10.3390/ijms21051723
Allegri, L., Capriglione, F., Maggisano, V., Damante, G., and Baldan, F. (2021). Effects of dihydrotanshinone I on proliferation and invasiveness of paclitaxel-resistant anaplastic thyroid cancer cells. Int. J. Mol. Sci. 22 (15), 8083. doi:10.3390/ijms22158083
Anson, D. M., Wilcox, R. M., Huseman, E. D., Stump, T. A., Paris, R. L., Darkwah, B. O., et al. (2018). Luteolin decreases epidermal growth factor receptor-mediated cell proliferation and induces apoptosis in glioblastoma cell lines. Basic Clin. Pharmacol. Toxicol. 123 (6), 678–686. doi:10.1111/bcpt.13077
Attoub, S., Hassan, A. H., Vanhoecke, B., Iratni, R., Takahashi, T., Gaben, A. M., et al. (2011). Inhibition of cell survival, invasion, tumor growth and histone deacetylase activity by the dietary flavonoid luteolin in human epithelioid cancer cells. Eur. J. Pharmacol. 651 (1-3), 18–25. doi:10.1016/j.ejphar.2010.10.063
Bachmann, I. M., Halvorsen, O. J., Collett, K., Stefansson, I. M., Straume, O., Haukaas, S. A., et al. (2006). EZH2 expression is associated with high proliferation rate and aggressive tumor subgroups in cutaneous melanoma and cancers of the endometrium, prostate, and breast. J. Clin. Oncol. 24 (2), 268–273. doi:10.1200/JCO.2005.01.5180
Bai, Y., Zhang, L., Fang, X., and Yang, Y. (2016). Tanshinone IIA enhances chemosensitivity of colon cancer cells by suppressing nuclear factor-κB. Exp. Ther. Med. 11 (3), 1085–1089. doi:10.3892/etm.2016.2984
Bejarano, F., Chang, C. H., Sun, K., Hagen, J. W., Deng, W. M., and Lai, E. C. (2021). A comprehensive in vivo screen for anti-apoptotic miRNAs indicates broad capacities for oncogenic synergy. Dev. Biol. 475, 10–20. doi:10.1016/j.ydbio.2021.02.010
Bhattacharjee, S., and Dashwood, R. H. (2020). Epigenetic regulation of NRF2/KEAP1 by phytochemicals. Antioxidants (Basel) 9 (9), E865. doi:10.3390/antiox9090865
Bird, A. P. (1986). CpG-rich islands and the function of DNA methylation. Nature 321 (6067), 209–213. doi:10.1038/321209a0
Bossi, G., and Sacchi, A. (2007). Restoration of wild-type p53 function in human cancer: Relevance for tumor therapy. Head. Neck 29 (3), 272–284. doi:10.1002/hed.20529
Cao, D., Zhu, G. Y., Lu, Y., Yang, A., Chen, D., Huang, H. J., et al. (2020). Luteolin suppresses epithelial-mesenchymal transition and migration of triple-negative breast cancer cells by inhibiting YAP/TAZ activity. Biomed. Pharmacother. 129, 110462. doi:10.1016/j.biopha.2020.110462
Cao, Y. F., Wang, S. F., Li, X., Zhang, Y. L., and Qiao, Y. J. (2018). The anticancer mechanism investigation of Tanshinone II(A) by pharmacological clustering in protein network. BMC Syst. Biol. 12 (1), 90. doi:10.1186/s12918-018-0606-6
Cao, Y., Lu, K., Xia, Y., Wang, Y., Wang, A., and Zhao, Y. (2022). Danshensu attenuated epithelial-mesenchymal transformation and chemoresistance of colon cancer cells induced by platelets. Front. Biosci. 27 (5), 160. doi:10.31083/j.fbl2705160
Chan, H. L., and Morey, L. (2019). Emerging roles for polycomb-group proteins in stem cells and cancer. Trends biochem. Sci. 44 (8), 688–700. doi:10.1016/j.tibs.2019.04.005
Chen, J., Ding, C., Chen, Y., Hu, W., Lu, Y., Wu, W., et al. (2020). ACSL4 promotes hepatocellular carcinoma progression via c-Myc stability mediated by ERK/FBW7/c-Myc axis. Oncogenesis 9 (4), 42. doi:10.1038/s41389-020-0226-z
Chen, J., Shi, D. Y., Liu, S. L., and Zhong, L. (2012). Tanshinone IIA induces growth inhibition and apoptosis in gastric cancer in vitro and in vivo. Oncol. Rep. 27 (2), 523–528. doi:10.3892/or.2011.1524
Chen, Q., Luo, J., Wu, C., Lu, H., Cai, S., Bao, C., et al. (2020a). The miRNA-149-5p/MyD88 axis is responsible for ursolic acid-mediated attenuation of the stemness and chemoresistance of non-small cell lung cancer cells. Environ. Toxicol. 35 (5), 561–569. doi:10.1002/tox.22891
Chen, T., Li, X. F., Wang, J. F., Zhou, S., and Fang, F. (2018). Effects of luteolin on proliferation and programmed cell death of human multiple myeloma cell RPMI-8226. Zhongguo Shi Yan Xue Ye Xue Za Zhi 26 (5), 1425–1429. doi:10.7534/j.issn.1009-2137.2018.05.028
Chen, X., Guo, J., Bao, J., Lu, J., and Wang, Y. (2014). The anticancer properties of salvia miltiorrhiza bunge (danshen): A systematic review. Med. Res. Rev. 34 (4), 768–794. doi:10.1002/med.21304
Chevalier, F. (2020). Counteracting radio-resistance using the optimization of radiotherapy. Int. J. Mol. Sci. 21 (5), E1767. doi:10.3390/ijms21051767
Chiu, S. C., Huang, S. Y., Chen, S. P., Su, C. C., Chiu, T. L., and Pang, C. Y. (2013). Tanshinone IIA inhibits human prostate cancer cells growth by induction of endoplasmic reticulum stress in vitro and in vivo. Prostate Cancer Prostatic Dis. 16 (4), 315–322. doi:10.1038/pcan.2013.38
Choi, Y. H., and Yu, A. M. (2014). ABC transporters in multidrug resistance and pharmacokinetics, and strategies for drug development. Curr. Pharm. Des. 20 (5), 793–807. doi:10.2174/138161282005140214165212
Cockram, P. E., Kist, M., Prakash, S., Chen, S. H., Wertz, I. E., and Vucic, D. (2021). Ubiquitination in the regulation of inflammatory cell death and cancer. Cell Death Differ. 28 (2), 591–605. doi:10.1038/s41418-020-00708-5
Ding, L., Ding, L., Wang, S., Wang, S., Wang, W., Wang, W., et al. (2017). Tanshinone IIA affects autophagy and apoptosis of glioma cells by inhibiting phosphatidylinositol 3-kinase/akt/mammalian target of rapamycin signaling pathway. Pharmacology 99 (3-4), 188–195. doi:10.1159/000452340
Ding, L., Wang, S., Qu, X., and Wang, J. (2016). Tanshinone IIA sensitizes oral squamous cell carcinoma to radiation due to an enhanced autophagy. Environ. Toxicol. Pharmacol. 46, 264–269. doi:10.1016/j.etap.2016.07.021
Duan, R., Du, W., and Guo, W. (2020). EZH2: A novel target for cancer treatment. J. Hematol. Oncol. 13 (1), 104. doi:10.1186/s13045-020-00937-8
Edathara, P. M., Chintalapally, S., Makani, V. K. K., Pant, C., Yerramsetty, S., D, R. M., et al. (2021). Inhibitory role of oleanolic acid and esculetin in HeLa cells involve multiple signaling pathways. Gene 771, 145370. doi:10.1016/j.gene.2020.145370
Esteller, M. (2008). Epigenetics in cancer. N. Engl. J. Med. 358 (11), 1148–1159. doi:10.1056/NEJMra072067
Fang, B., Chen, X., Wu, M., Kong, H., Chu, G., Zhou, Z., et al. (2018). Luteolin inhibits angiogenesis of the M2-like TAMs via the downregulation of hypoxia inducible factor-1α and the STAT3 signalling pathway under hypoxia. Mol. Med. Rep. 18 (3), 2914–2922. doi:10.3892/mmr.2018.9250
Fang, Z. Y., Zhang, M., Liu, J. N., Zhao, X., Zhang, Y. Q., and Fang, L. (2020). Tanshinone IIA: A review of its anticancer effects. Front. Pharmacol. 11, 611087. doi:10.3389/fphar.2020.611087
Fu, L., Han, B., Zhou, Y., Ren, J., Cao, W., Patel, G., et al. (2020). The anticancer properties of tanshinones and the pharmacological effects of their active ingredients. Front. Pharmacol. 11, 193. doi:10.3389/fphar.2020.00193
Fu, L. Q., Du, W. L., Cai, M. H., Yao, J. Y., Zhao, Y. Y., and Mou, X. Z. (2020a). The roles of tumor-associated macrophages in tumor angiogenesis and metastasis. Cell. Immunol. 353, 104119. doi:10.1016/j.cellimm.2020.104119
Funderburk, S. F., Wang, Q. J., and Yue, Z. (2010). The Beclin 1-VPS34 complex--at the crossroads of autophagy and beyond. Trends Cell Biol. 20 (6), 355–362. doi:10.1016/j.tcb.2010.03.002
Gan, L., Xu, M., Hua, R., Tan, C., Zhang, J., Gong, Y., et al. (2018). The polycomb group protein EZH2 induces epithelial-mesenchymal transition and pluripotent phenotype of gastric cancer cells by binding to PTEN promoter. J. Hematol. Oncol. 11 (1), 9. doi:10.1186/s13045-017-0547-3
Gao, F., Li, M., Liu, W., and Li, W. (2020). Inhibition of EGFR signaling and activation of mitochondrial apoptosis contribute to tanshinone IIA-mediated tumor suppression in non-small cell lung cancer cells. Onco. Targets. Ther. 13, 2757–2769. doi:10.2147/OTT.S246606
Gao, G., Ge, R., Li, Y., and Liu, S. (2019). Luteolin exhibits anti-breast cancer property through up-regulating miR-203. Artif. Cells Nanomed. Biotechnol. 47 (1), 3265–3271. doi:10.1080/21691401.2019.1646749
Gong, L., Di, C., Xia, X., Wang, J., Chen, G., Shi, J., et al. (2016). AKT/mTOR signaling pathway is involved in salvianolic acid B-induced autophagy and apoptosis in hepatocellular carcinoma cells. Int. J. Oncol. 49 (6), 2538–2548. doi:10.3892/ijo.2016.3748
Gong, Y., Li, Y., Abdolmaleky, H. M., Li, L., and Zhou, J. R. (2012). Tanshinones inhibit the growth of breast cancer cells through epigenetic modification of Aurora A expression and function. PLoS One 7 (4), e33656. doi:10.1371/journal.pone.0033656
Guo, Y., Li, Y., Wang, F. F., Xiang, B., Huang, X. O., Ma, H. B., et al. (2019). The combination of Nutlin-3 and Tanshinone IIA promotes synergistic cytotoxicity in acute leukemic cells expressing wild-type p53 by co-regulating MDM2-P53 and the AKT/mTOR pathway. Int. J. Biochem. Cell Biol. 106, 8–20. doi:10.1016/j.biocel.2018.10.008
Guo, Y., Li, Y., Xiang, B., Huang, X. O., Ma, H. B., Wang, F. F., et al. (2017). Nutlin-3 plus tanshinone IIA exhibits synergetic anti-leukemia effect with imatinib by reactivating p53 and inhibiting the AKT/mTOR pathway in Ph+ ALL. Biochem. J. 474 (24), 4153–4170. doi:10.1042/BCJ20170386
Haider, T., Pandey, V., Banjare, N., Gupta, P. N., and Soni, V. (2020). Drug resistance in cancer: Mechanisms and tackling strategies. Pharmacol. Rep. 72 (5), 1125–1151. doi:10.1007/s43440-020-00138-7
Han, C., Wei, Y., Wang, X., Ba, C., and Shi, W. (2019). Protective effect of Salvia miltiorrhiza polysaccharides on liver injury in chickens. Poult. Sci. 98 (9), 3496–3503. doi:10.3382/ps/pez153
Han, G., Wang, Y., Liu, T., Gao, J., Duan, F., Chen, M., et al. (2022). Salvianolic acid B acts against non-small cell lung cancer A549 cells via inactivation of the MAPK and Smad2/3 signaling pathways. Mol. Med. Rep. 25 (5), 184. doi:10.3892/mmr.2022.12700
He, Z. X., Wei, B. F., Zhang, X., Gong, Y. P., Ma, L. Y., and Zhao, W. (2021). Current development of CBP/p300 inhibitors in the last decade. Eur. J. Med. Chem. 209, 112861. doi:10.1016/j.ejmech.2020.112861
Ho, T. C. S., Chan, A. H. Y., and Ganesan, A. (2020). Thirty years of HDAC inhibitors: 2020 insight and hindsight. J. Med. Chem. 63 (21), 12460–12484. doi:10.1021/acs.jmedchem.0c00830
Hu, T., To, K. K., Wang, L., Zhang, L., Lu, L., Shen, J., et al. (2014). Reversal of P-glycoprotein (P-gp) mediated multidrug resistance in colon cancer cells by cryptotanshinone and dihydrotanshinone of Salvia miltiorrhiza. Phytomedicine 21 (11), 1264–1272. doi:10.1016/j.phymed.2014.06.013
Hu, T., Wang, L., Zhang, L., Lu, L., Shen, J., Chan, R. L., et al. (2015). Sensitivity of apoptosis-resistant colon cancer cells to tanshinones is mediated by autophagic cell death and p53-independent cytotoxicity. Phytomedicine 22 (5), 536–544. doi:10.1016/j.phymed.2015.03.010
Hua, H., Kong, Q., Zhang, H., Wang, J., Luo, T., and Jiang, Y. (2019). Targeting mTOR for cancer therapy. J. Hematol. Oncol. 12 (1), 71. doi:10.1186/s13045-019-0754-1
Huang, S. Y., Chang, S. F., Liao, K. F., and Chiu, S. C. (2017). Tanshinone IIA inhibits epithelial-mesenchymal transition in bladder cancer cells via modulation of STAT3-CCL2 signaling. Int. J. Mol. Sci. 18 (8), E1616. doi:10.3390/ijms18081616
Huang, Y., Yu, S. H., Zhen, W. X., Cheng, T., Wang, D., Lin, J. B., et al. (2021). Tanshinone I, a new EZH2 inhibitor restricts normal and malignant hematopoiesis through upregulation of MMP9 and ABCG2. Theranostics 11 (14), 6891–6904. doi:10.7150/thno.53170
Huang, Y., Yuan, K., Tang, M., Yue, J., Bao, L., Wu, S., et al. (2021a). Melatonin inhibiting the survival of human gastric cancer cells under ER stress involving autophagy and Ras-Raf-MAPK signalling. J. Cell. Mol. Med. 25 (3), 1480–1492. doi:10.1111/jcmm.16237
Huang, Y., Zhou, Z., Zhang, J., Hao, Z., He, Y., Wu, Z., et al. (2021b). lncRNA MALAT1 participates in metformin inhibiting the proliferation of breast cancer cell. J. Cell. Mol. Med. 25 (15), 7135–7145. doi:10.1111/jcmm.16742
Iida, K., Naiki, T., Naiki-Ito, A., Suzuki, S., Kato, H., Nozaki, S., et al. (2020). Luteolin suppresses bladder cancer growth via regulation of mechanistic target of rapamycin pathway. Cancer Sci. 111 (4), 1165–1179. doi:10.1111/cas.14334
Iorio, M. V., and Croce, C. M. (2012). MicroRNA dysregulation in cancer: Diagnostics, monitoring and therapeutics. A comprehensive review. EMBO Mol. Med. 4 (3), 143–159. doi:10.1002/emmm.201100209
Jamialahmadi, K., Zahedipour, F., and Karimi, G. (2021). The role of microRNAs on doxorubicin drug resistance in breast cancer. J. Pharm. Pharmacol. 73 (8), 997–1006. doi:10.1093/jpp/rgaa031
Janardhan, A., Kathera, C., Darsi, A., Ali, W., He, L., Yang, Y., et al. (2018). Prominent role of histone lysine demethylases in cancer epigenetics and therapy. Oncotarget 9 (76), 34429–34448. doi:10.18632/oncotarget.24319
Jiang, X., Wang, J., Deng, X., Xiong, F., Zhang, S., Gong, Z., et al. (2020). The role of microenvironment in tumor angiogenesis. J. Exp. Clin. Cancer Res. 39 (1), 204. doi:10.1186/s13046-020-01709-5
Jieensinue, S., Zhu, H., Li, G., Dong, K., Liang, M., and Li, Y. (2018). Tanshinone IIA reduces SW837 colorectal cancer cell viability via the promotion of mitochondrial fission by activating JNK-Mff signaling pathways. BMC Cell Biol. 19 (1), 21. doi:10.1186/s12860-018-0174-z
Jin, L., Chen, C., Huang, L., Bu, L., Zhang, L., and Yang, Q. (2021). Salvianolic acid A blocks vasculogenic mimicry formation in human non-small cell lung cancer via PI3K/Akt/mTOR signalling. Clin. Exp. Pharmacol. Physiol. 48 (4), 508–514. doi:10.1111/1440-1681.13464
Jin, W. (2020). Role of JAK/STAT3 signaling in the regulation of metastasis, the transition of cancer stem cells, and chemoresistance of cancer by epithelial-mesenchymal transition. Cells 9 (1), E217. doi:10.3390/cells9010217
Jung, D. Y., Kim, J. H., and Jung, M. H. (2020). Anti-obesity effects of tanshinone I from salvia miltiorrhiza bunge in mice fed a high-fat diet through inhibition of early adipogenesis. Nutrients 12 (5), E1242. doi:10.3390/nu12051242
Kan, S., Cheung, W. M., Zhou, Y., and Ho, W. S. (2014). Enhancement of doxorubicin cytotoxicity by tanshinone IIA in HepG2 human hepatoma cells. Planta Med. 80 (1), 70–76. doi:10.1055/s-0033-1360126
Kanwal, R., Gupta, K., and Gupta, S. (2015). Cancer epigenetics: An introduction. Methods Mol. Biol. 1238, 3–25. doi:10.1007/978-1-4939-1804-1_1
Karabiyik, C., Vicinanza, M., Son, S. M., and Rubinsztein, D. C. (2021). Glucose starvation induces autophagy via ULK1-mediated activation of PIKfyve in an AMPK-dependent manner. Dev. Cell 56 (13), 1961–1975.e5. doi:10.1016/j.devcel.2021.05.010
Karakaidos, P., Verigos, J., and Magklara, A. (2019). LSD1/KDM1A, a gate-keeper of cancer stemness and a promising therapeutic target. Cancers (Basel) 11 (12), E1821. doi:10.3390/cancers11121821
Kashyap, A., Umar, S. M., Dev, J. R. A., and Prasad, C. P. (2021). Dihydrotanshinone-I modulates epithelial mesenchymal transition (EMT) thereby impairing migration and clonogenicity of triple negative breast cancer cells. Asian pac. J. Cancer Prev. 22 (7), 2177–2184. doi:10.31557/APJCP.2021.22.7.2177
Keeley, E. C., Mehrad, B., and Strieter, R. M. (2010). CXC chemokines in cancer angiogenesis and metastases. Adv. Cancer Res. 106, 91–111. doi:10.1016/S0065-230X(10)06003-3
Kim, H., Ramirez, C. N., Su, Z. Y., and Kong, A. N. (2016). Epigenetic modifications of triterpenoid ursolic acid in activating Nrf2 and blocking cellular transformation of mouse epidermal cells. J. Nutr. Biochem. 33, 54–62. doi:10.1016/j.jnutbio.2015.09.014
Kim, J., Kundu, M., Viollet, B., and Guan, K. L. (2011). AMPK and mTOR regulate autophagy through direct phosphorylation of Ulk1. Nat. Cell Biol. 13 (2), 132–141. doi:10.1038/ncb2152
Kim, K., Shin, E. A., Jung, J. H., Park, J. E., Kim, D. S., Shim, B. S., et al. (2018). Ursolic acid induces apoptosis in colorectal cancer cells partially via upregulation of MicroRNA-4500 and inhibition of JAK2/STAT3 phosphorylation. Int. J. Mol. Sci. 20 (1), E114. doi:10.3390/ijms20010114
Kumar, V., Radin, D., and Leonardi, D. (2019). Studies examining the synergy between Dihydrotanshinone and Temozolomide against MGMT+ glioblastoma cells in vitro: Predicting interactions with the blood-brain barrier. Biomed. Pharmacother. 109, 386–390. doi:10.1016/j.biopha.2018.10.069
Lakshminarasimhan, R., and Liang, G. (2016). The role of DNA methylation in cancer. Adv. Exp. Med. Biol. 945, 151–172. doi:10.1007/978-3-319-43624-1_7
Lee, H. P., Liu, Y. C., Chen, P. C., Tai, H. C., Li, T. M., Fong, Y. C., et al. (2017). Tanshinone IIA inhibits angiogenesis in human endothelial progenitor cells in vitro and in vivo. Oncotarget 8 (65), 109217–109227. doi:10.18632/oncotarget.22649
Lee, W. D., Liang, Y. J., and Chen, B. H. (2016). Effects of tanshinone nanoemulsion and extract on inhibition of lung cancer cells A549. Nanotechnology 27 (49), 495101. doi:10.1088/0957-4484/27/49/495101
Lee, Y. S., and Dutta, A. (2009). MicroRNAs in cancer. Annu. Rev. Pathol. 4, 199–227. doi:10.1146/annurev.pathol.4.110807.092222
Li, C., Wang, Q., Shen, S., Wei, X., and Li, G. (2019). HIF-1α/VEGF signaling-mediated epithelial-mesenchymal transition and angiogenesis is critically involved in anti-metastasis effect of luteolin in melanoma cells. Phytother. Res. 33 (3), 798–807. doi:10.1002/ptr.6273
Li, G., Shan, C., Liu, L., Zhou, T., Zhou, J., Hu, X., et al. (2015). Tanshinone IIA inhibits HIF-1α and VEGF expression in breast cancer cells via mTOR/p70S6K/RPS6/4E-BP1 signaling pathway. PLoS One 10 (2), e0117440. doi:10.1371/journal.pone.0117440
Li, K., and Lai, H. (2017). TanshinoneIIA enhances the chemosensitivity of breast cancer cells to doxorubicin through down-regulating the expression of MDR-related ABC transporters. Biomed. Pharmacother. 96, 371–377. doi:10.1016/j.biopha.2017.10.016
Li, K., Liu, W., Zhao, Q., Wu, C., Fan, C., Lai, H., et al. (2019a). Combination of tanshinone IIA and doxorubicin possesses synergism and attenuation effects on doxorubicin in the treatment of breast cancer. Phytother. Res. 33 (6), 1658–1669. doi:10.1002/ptr.6353
Li, L., Zhang, Z. H., and Zhao, W. D. (2009). Apoptosis of MR2 cells induced by Tanshinone II A combined with arsenic trioxide. Sichuan Da Xue Xue Bao Yi Xue Ban. 40 (5), 812–816.
Li, M., Gao, F., Zhao, Q., Zuo, H., Liu, W., and Li, W. (2020). Tanshinone IIA inhibits oral squamous cell carcinoma via reducing Akt-c-Myc signaling-mediated aerobic glycolysis. Cell Death Dis. 11 (5), 381. doi:10.1038/s41419-020-2579-9
Li, M., Liu, H., Zhao, Q., Han, S., Zhou, L., Liu, W., et al. (2021). Targeting Aurora B kinase with Tanshinone IIA suppresses tumor growth and overcomes radioresistance. Cell Death Dis. 12 (2), 152. doi:10.1038/s41419-021-03434-z
Li, Q., Zhang, J., Liang, Y., Mu, W., Hou, X., Ma, X., et al. (2018). Tanshinone l exhibits anticancer effects in human endometrial carcinoma HEC-1-A cells via mitochondrial mediated apoptosis, cell cycle arrest and inhibition of JAK/STAT signalling pathway. J. buon 23 (4), 1092–1096.
Li, S., Wu, C., Fan, C., Zhang, P., Yu, G., and Li, K. (2021a). Tanshinone II A improves the chemosensitivity of breast cancer cells to doxorubicin by inhibiting β-catenin nuclear translocation. J. Biochem. Mol. Toxicol. 35 (1), e22620. doi:10.1002/jbt.22620
Li, S., Wu, R., Wang, L., Dina Kuo, H. C., Sargsyan, D., Zheng, X., et al. (2022). Triterpenoid ursolic acid drives metabolic rewiring and epigenetic reprogramming in treatment/prevention of human prostate cancer. Mol. Carcinog. 61 (1), 111–121. doi:10.1002/mc.23365
Li, X., Jia, Q., Zhou, Y., Jiang, X., Song, L., Wu, Y., et al. (2022a). Tanshinone IIA attenuates the stemness of breast cancer cells via targeting the miR-125b/STARD13 axis. Exp. Hematol. Oncol. 11 (1), 2. doi:10.1186/s40164-022-00255-4
Li, X., Li, Z., Li, X., Liu, B., and Liu, Z. (2017). Mechanisms of Tanshinone II a inhibits malignant melanoma development through blocking autophagy signal transduction in A375 cell. BMC Cancer 17 (1), 357. doi:10.1186/s12885-017-3329-y
Li, Y., and Chen, Y. (2019). AMPK and autophagy. Adv. Exp. Med. Biol. 1206, 85–108. doi:10.1007/978-981-15-0602-4_4
Li, Y., and Seto, E. (2016). HDACs and HDAC inhibitors in cancer development and therapy. Cold Spring Harb. Perspect. Med. 6 (10), a026831. doi:10.1101/cshperspect.a026831
Li, Z. M., Xu, S. W., and Liu, P. Q. (2018a). Salvia miltiorrhizaBurge (danshen): A golden herbal medicine in cardiovascular therapeutics. Acta Pharmacol. Sin. 39 (5), 802–824. doi:10.1038/aps.2017.193
Liang, Y. Y., Wan, X. H., Niu, F. J., Xie, S. M., Guo, H., Yang, Y. Y., et al. (2020). Salvia plebeia R. Br, an overview about its traditional uses, chemical constituents, pharmacology and modern applications. Biomed. Pharmacother. 121, 109589. doi:10.1016/j.biopha.2019.109589
Lin, H., Zheng, L., Li, S., Xie, B., Cui, B., Xia, A., et al. (2018). Cytotoxicity of Tanshinone IIA combined with Taxol on drug-resist breast cancer cells MCF-7 through inhibition of Tau. Phytother. Res. 32 (4), 667–671. doi:10.1002/ptr.6014
Lin, L. L., Hsia, C. R., Hsu, C. L., Huang, H. C., and Juan, H. F. (2015). Integrating transcriptomics and proteomics to show that tanshinone IIA suppresses cell growth by blocking glucose metabolism in gastric cancer cells. BMC Genomics 16 (1), 41. doi:10.1186/s12864-015-1230-0
Liu, J. J., Liu, W. D., Yang, H. Z., Zhang, Y., Fang, Z. G., Liu, P. Q., et al. (2010). Inactivation of PI3k/Akt signaling pathway and activation of caspase-3 are involved in tanshinone I-induced apoptosis in myeloid leukemia cells in vitro. Ann. Hematol. 89 (11), 1089–1097. doi:10.1007/s00277-010-0996-z
Liu, T., Xu, J., Yan, H. L., Cheng, F. C., and Liu, X. J. (2019). Luteolin suppresses teratoma cell growth and induces cell apoptosis via inhibiting bcl-2. Oncol. Res. 27 (7), 773–778. doi:10.3727/096504018X15208986577685
Liu, X., Zou, H., Zhao, Y., Chen, H., Liu, T., Wu, Z., et al. (2020). Tanshinone inhibits NSCLC by downregulating AURKA through let-7a-5p. Front. Genet. 11, 838. doi:10.3389/fgene.2020.00838
Liu, Y., Lin, F., Chen, Y., Wang, R., Liu, J., Jin, Y., et al. (2020a). Cryptotanshinone inhibites bladder cancer cell proliferation and promotes apoptosis via the PTEN/PI3K/AKT pathway. J. Cancer 11 (2), 488–499. doi:10.7150/jca.31422
Lu, M., Wang, C., and Wang, J. (2016). Tanshinone I induces human colorectal cancer cell apoptosis: The potential roles of Aurora A-p53 and survivin-mediated signaling pathways. Int. J. Oncol. 49 (2), 603–610. doi:10.3892/ijo.2016.3565
Lu, X., Li, Y., Yang, W., Tao, M., Dai, Y., Xu, J., et al. (2021). Inhibition of NF-κB is required for oleanolic acid to downregulate PD-L1 by promoting DNA demethylation in gastric cancer cells. J. Biochem. Mol. Toxicol. 35 (1), e22621. doi:10.1002/jbt.22621
Ma, S., Meng, Z., Chen, R., and Guan, K. L. (2019). The hippo pathway: Biology and pathophysiology. Annu. Rev. Biochem. 88, 577–604. doi:10.1146/annurev-biochem-013118-111829
Ma, Z. L., Zhang, B. J., Wang, D. T., Li, X., Wei, J. L., Zhao, B. T., et al. (2015). Tanshinones suppress AURKA through up-regulation of miR-32 expression in non-small cell lung cancer. Oncotarget 6 (24), 20111–20120. doi:10.18632/oncotarget.3933
Macklin, P. S., McAuliffe, J., Pugh, C. W., and Yamamoto, A. (2017). Hypoxia and HIF pathway in cancer and the placenta. Placenta 56, 8–13. doi:10.1016/j.placenta.2017.03.010
Man, Y., Yang, L., Zhang, D., and Bi, Y. (2016). Cryptotanshinone inhibits lung tumor growth by increasing CD4(+) T cell cytotoxicity through activation of the JAK2/STAT4 pathway. Oncol. Lett. 12 (5), 4094–4098. doi:10.3892/ol.2016.5123
Mandal, S., Gamit, N., Varier, L., Dharmarajan, A., and Warrier, S. (2021). Inhibition of breast cancer stem-like cells by a triterpenoid, ursolic acid, via activation of Wnt antagonist, sFRP4 and suppression of miRNA-499a-5p. Life Sci. 265, 118854. doi:10.1016/j.lfs.2020.118854
Melincovici, C. S., Boşca, A. B., Şuşman, S., Mărginean, M., Mihu, C., Istrate, M., et al. (2018). Vascular endothelial growth factor (VEGF) - key factor in normal and pathological angiogenesis. Rom. J. Morphol. Embryol. 59 (2), 455–467.
Mens, M. M. J., and Ghanbari, M. (2018). Cell cycle regulation of stem cells by MicroRNAs. Stem Cell Rev. Rep. 14 (3), 309–322. doi:10.1007/s12015-018-9808-y
Meraldi, P., Honda, R., and Nigg, E. A. (2004). Aurora kinases link chromosome segregation and cell division to cancer susceptibility. Curr. Opin. Genet. Dev. 14 (1), 29–36. doi:10.1016/j.gde.2003.11.006
Mohr, A. M., and Mott, J. L. (2015). Overview of microRNA biology. Semin. Liver Dis. 35 (1), 3–11. doi:10.1055/s-0034-1397344
Naiki-Ito, A., Naiki, T., Kato, H., Iida, K., Etani, T., Nagayasu, Y., et al. (2020). Recruitment of miR-8080 by luteolin inhibits androgen receptor splice variant 7 expression in castration-resistant prostate cancer. Carcinogenesis 41 (8), 1145–1157. doi:10.1093/carcin/bgz193
Nie, Z. Y., Zhao, M. H., Cheng, B. Q., Pan, R. F., Wang, T. R., Qin, Y., et al. (2020). Tanshinone IIA regulates human AML cell proliferation, cell cycle, and apoptosis through miR-497-5p/AKT3 axis. Cancer Cell Int. 20, 379. doi:10.1186/s12935-020-01468-5
Nizamutdinova, I. T., Lee, G. W., Lee, J. S., Cho, M. K., Son, K. H., Jeon, S. J., et al. (2008). Tanshinone I suppresses growth and invasion of human breast cancer cells, MDA-MB-231, through regulation of adhesion molecules. Carcinogenesis 29 (10), 1885–1892. doi:10.1093/carcin/bgn151
Noguchi, S., Saito, A., and Nagase, T. (2018). YAP/TAZ signaling as a molecular link between fibrosis and cancer. Int. J. Mol. Sci. 19 (11), E3674. doi:10.3390/ijms19113674
Ono, H., Sowa, Y., Horinaka, M., Iizumi, Y., Watanabe, M., Morita, M., et al. (2018). The histone deacetylase inhibitor OBP-801 and eribulin synergistically inhibit the growth of triple-negative breast cancer cells with the suppression of survivin, Bcl-xL, and the MAPK pathway. Breast Cancer Res. Treat. 171 (1), 43–52. doi:10.1007/s10549-018-4815-x
Pan, J., Cai, X., Zheng, X., Zhu, X., Feng, J., and Wang, X. (2022). Luteolin inhibits viability, migration, angiogenesis and invasion of non-small cell lung cancer vascular endothelial cells via miR-133a-3p/purine rich element binding protein B-mediated MAPK and PI3K/Akt signaling pathways. Tissue Cell 75, 101740. doi:10.1016/j.tice.2022.101740
Pan, T. L., Hung, Y. C., Wang, P. W., Chen, S. T., Hsu, T. K., Sintupisut, N., et al. (2010). Functional proteomic and structural insights into molecular targets related to the growth inhibitory effect of tanshinone IIA on HeLa cells. Proteomics 10 (5), 914–929. doi:10.1002/pmic.200900178
Pan, Y., Chen, L., Li, R., Liu, Y., Nan, M., and Hou, L. (2021). Tanshinone IIa induces autophagy and apoptosis via PI3K/Akt/mTOR Axis in acute promyelocytic leukemia NB4 cells. Evid. Based. Complement. Altern. Med. 2021, 3372403. doi:10.1155/2021/3372403
Pan, Y., Liu, G., Zhou, F., Su, B., and Li, Y. (2018). DNA methylation profiles in cancer diagnosis and therapeutics. Clin. Exp. Med. 18 (1), 1–14. doi:10.1007/s10238-017-0467-0
Park, S. H., Park, H. S., Lee, J. H., Chi, G. Y., Kim, G. Y., Moon, S. K., et al. (2013). Induction of endoplasmic reticulum stress-mediated apoptosis and non-canonical autophagy by luteolin in NCI-H460 lung carcinoma cells. Food Chem. Toxicol. 56, 100–109. doi:10.1016/j.fct.2013.02.022
Parzych, K. R., and Klionsky, D. J. (2014). An overview of autophagy: Morphology, mechanism, and regulation. Antioxid. Redox Signal. 20 (3), 460–473. doi:10.1089/ars.2013.5371
Pastushenko, I., and Blanpain, C. (2019). EMT transition states during tumor progression and metastasis. Trends Cell Biol. 29 (3), 212–226. doi:10.1016/j.tcb.2018.12.001
Patriarca, C., Pini, G. M., and Conti, G. (2020). Invasion and metastasis: A historical perspective. Pathologica 112 (4), 229–233. doi:10.32074/1591-951X-111
Pellegrino, N. E., Guven, A., Gray, K., Shah, P., Kasture, G., Nastke, M. D., et al. (2022). The next frontier: Translational development of ubiquitination, SUMOylation, and NEDDylation in cancer. Int. J. Mol. Sci. 23 (7), 3480. doi:10.3390/ijms23073480
Pistritto, G., Trisciuoglio, D., Ceci, C., Garufi, A., and D'Orazi, G. (2016). Apoptosis as anticancer mechanism: Function and dysfunction of its modulators and targeted therapeutic strategies. Aging (Albany NY) 8 (4), 603–619. doi:10.18632/aging.100934
Potočnjak, I., Šimić, L., Gobin, I., Vukelić, I., and Domitrović, R. (2020). Antitumor activity of luteolin in human colon cancer SW620 cells is mediated by the ERK/FOXO3a signaling pathway. Toxicol. Vitro 66, 104852. doi:10.1016/j.tiv.2020.104852
Pujade-Lauraine, E., Banerjee, S., and Pignata, S. (2019). Management of platinum-resistant, relapsed epithelial ovarian cancer and new drug perspectives. J. Clin. Oncol. 37 (27), 2437–2448. doi:10.1200/JCO.19.00194
Qi, H., Chen, Z., Qin, Y., Wang, X., Zhang, Z., and Li, Y. (2022). Tanshinone IIA inhibits cell growth by suppressing SIX1-induced aerobic glycolysis in non-small cell lung cancer cells. Oncol. Lett. 23 (6), 184. doi:10.3892/ol.2022.13304
Qi, P., Li, Y., Liu, X., Jafari, F. A., Zhang, X., Sun, Q., et al. (2019). Cryptotanshinone suppresses non-small cell lung cancer via microRNA-146a-5p/EGFR Axis. Int. J. Biol. Sci. 15 (5), 1072–1079. doi:10.7150/ijbs.31277
Qian, J., Cao, Y., Zhang, J., Li, L., Wu, J., Wei, G., et al. (2020). Tanshinone IIA induces autophagy in colon cancer cells through MEK/ERK/mTOR pathway. Transl. Cancer Res. 9 (11), 6919–6928. doi:10.21037/tcr-20-1963
Qin, T., Zhu, W., Kan, X., Li, L., and Wu, D. (2022). Luteolin attenuates the chemoresistance of osteosarcoma through inhibiting the PTN/β-catenin/MDR1 signaling axis by upregulating miR-384. J. Bone Oncol. 34, 100429. doi:10.1016/j.jbo.2022.100429
Qiu, Y., Li, C., Wang, Q., Zeng, X., and Ji, P. (2018). Tanshinone IIA induces cell death via Beclin-1-dependent autophagy in oral squamous cell carcinoma SCC-9 cell line. Cancer Med. 7 (2), 397–407. doi:10.1002/cam4.1281
Querfurth, H., and Lee, H. K. (2021). Mammalian/mechanistic target of rapamycin (mTOR) complexes in neurodegeneration. Mol. Neurodegener. 16 (1), 44. doi:10.1186/s13024-021-00428-5
Ren, J., Fu, L., Nile, S. H., Zhang, J., and Kai, G. (2019). Salvia miltiorrhiza in treating cardiovascular diseases: A review on its pharmacological and clinical applications. Front. Pharmacol. 10, 753. doi:10.3389/fphar.2019.00753
Ren, L. Q., Li, Q., and Zhang, Y. (2020). Luteolin suppresses the proliferation of gastric cancer cells and acts in synergy with oxaliplatin. Biomed. Res. Int. 2020, 9396512. doi:10.1155/2020/9396512
Ren, X., Wang, C., Xie, B., Hu, L., Chai, H., Ding, L., et al. (2017). Tanshinone IIA induced cell death via miR30b-p53-PTPN11/SHP2 signaling pathway in human hepatocellular carcinoma cells. Eur. J. Pharmacol. 796, 233–241. doi:10.1016/j.ejphar.2016.11.046
Rivera, E., and Gomez, H. (2010). Chemotherapy resistance in metastatic breast cancer: The evolving role of ixabepilone. Breast Cancer Res. 12 (2), S2. doi:10.1186/bcr2573
Rodrigues, C., B, L., Lallo, M. A., and Perez, E. C. (2020). The controversial role of autophagy in tumor development: A systematic review. Immunol. Invest. 49 (4), 386–396. doi:10.1080/08820139.2019.1682600
Rodríguez-Paredes, M., and Esteller, M. (2011). Cancer epigenetics reaches mainstream oncology. Nat. Med. 17 (3), 330–339. doi:10.1038/nm.2305
Rotili, D., and Mai, A. (2011). Targeting histone demethylases: A new avenue for the fight against cancer. Genes Cancer 2 (6), 663–679. doi:10.1177/1947601911417976
Rupaimoole, R., and Slack, F. J. (2017). MicroRNA therapeutics: Towards a new era for the management of cancer and other diseases. Nat. Rev. Drug Discov. 16 (3), 203–222. doi:10.1038/nrd.2016.246
Russell, R. C., Tian, Y., Yuan, H., Park, H. W., Chang, Y. Y., Kim, J., et al. (2013). ULK1 induces autophagy by phosphorylating Beclin-1 and activating VPS34 lipid kinase. Nat. Cell Biol. 15 (7), 741–750. doi:10.1038/ncb2757
Ryu, S., Park, S., Lim, W., and Song, G. (2019). Effects of luteolin on canine osteosarcoma: Suppression of cell proliferation and synergy with cisplatin. J. Cell. Physiol. 234 (6), 9504–9514. doi:10.1002/jcp.27638
Salinas-Arellano, E., Pérez-Vásquez, A., Rivero-Cruz, I., Torres-Colin, R., González-Andrade, M., Rangel-Grimaldo, M., et al. (2020). Flavonoids and terpenoids with PTP-1B inhibitory properties from the infusion of salvia amarissima ortega. Molecules 25 (15), E3530. doi:10.3390/molecules25153530
Sandoval, J., Heyn, H., Moran, S., Serra-Musach, J., Pujana, M. A., Bibikova, M., et al. (2011). Validation of a DNA methylation microarray for 450, 000 CpG sites in the human genome. Epigenetics 6 (6), 692–702. doi:10.4161/epi.6.6.16196
Shan, Y. F., Shen, X., Xie, Y. K., Chen, J. C., Shi, H. Q., Yu, Z. P., et al. (2009). Inhibitory effects of tanshinone II-A on invasion and metastasis of human colon carcinoma cells. Acta Pharmacol. Sin. 30 (11), 1537–1542. doi:10.1038/aps.2009.139
Shang, S., Hua, F., and Hu, Z. W. (2017). The regulation of β-catenin activity and function in cancer: Therapeutic opportunities. Oncotarget 8 (20), 33972–33989. doi:10.18632/oncotarget.15687
Shin, E. A., Sohn, E. J., Won, G., Choi, J. U., Jeong, M., Kim, B., et al. (2014). Upregulation of microRNA135a-3p and death receptor 5 plays a critical role in Tanshinone I sensitized prostate cancer cells to TRAIL induced apoptosis. Oncotarget 5 (14), 5624–5636. doi:10.18632/oncotarget.2152
Sohn, E. J., Won, G., Lee, J., Yoon, S. W., Lee, I., Kim, H. J., et al. (2016). Blockage of epithelial to mesenchymal transition and upregulation of let 7b are critically involved in ursolic acid induced apoptosis in malignant mesothelioma cell. Int. J. Biol. Sci. 12 (11), 1279–1288. doi:10.7150/ijbs.13453
Song, Q., Yang, L., Han, Z., Wu, X., Li, R., Zhou, L., et al. (2020). Tanshinone IIA inhibits epithelial-to-mesenchymal transition through hindering β-arrestin1 mediated β-catenin signaling pathway in colorectal cancer. Front. Pharmacol. 11, 586616. doi:10.3389/fphar.2020.586616
Su, C. C. (2012). Tanshinone IIA potentiates the efficacy of 5-FU in Colo205 colon cancer cells in vivo through downregulation of P-gp and LC3-II. Exp. Ther. Med. 3 (3), 555–559. doi:10.3892/etm.2011.441
Su, C. Y., Ming, Q. L., Rahman, K., Han, T., and Qin, L. P. (2015). Salvia miltiorrhiza: Traditional medicinal uses, chemistry, and pharmacology. Chin. J. Nat. Med. 13 (3), 163–182. doi:10.1016/S1875-5364(15)30002-9
Su, L. J., Zhang, J. H., Gomez, H., Murugan, R., Hong, X., Xu, D., et al. (2019). Reactive oxygen species-induced lipid peroxidation in apoptosis, autophagy, and ferroptosis. Oxid. Med. Cell. Longev. 2019, 5080843. doi:10.1155/2019/5080843
Suhail, Y., Cain, M. P., Vanaja, K., Kurywchak, P. A., Levchenko, A., Kalluri, R., et al. (2019). Systems biology of cancer metastasis. Cell Syst. 9 (2), 109–127. doi:10.1016/j.cels.2019.07.003
Sui, H., Zhao, J., Zhou, L., Wen, H., Deng, W., Li, C., et al. (2017). Tanshinone IIA inhibits β-catenin/VEGF-mediated angiogenesis by targeting TGF-β1 in normoxic and HIF-1α in hypoxic microenvironments in human colorectal cancer. Cancer Lett. 403, 86–97. doi:10.1016/j.canlet.2017.05.013
Sun, C., Han, B., Zhai, Y., Zhao, H., Li, X., Qian, J., et al. (2022). Dihydrotanshinone I inhibits ovarian tumor growth by activating oxidative stress through Keap1-mediated Nrf2 ubiquitination degradation. Free Radic. Biol. Med. 180, 220–235. doi:10.1016/j.freeradbiomed.2022.01.015
Sun, S., Zhu, L., Lai, M., Cheng, R., and Ge, Y. (2021). Tanshinone I inhibited growth of human chronic myeloid leukemia cells via JNK/ERK mediated apoptotic pathways. Braz J. Med. Biol. Res. 54 (8), e10685. doi:10.1590/1414-431X2020e10685
Sung, H., Ferlay, J., Siegel, R. L., Laversanne, M., Soerjomataram, I., Jemal, A., et al. (2021). Global cancer statistics 2020: GLOBOCAN estimates of incidence and mortality worldwide for 36 cancers in 185 countries. Ca. Cancer J. Clin. 71 (3), 209–249. doi:10.3322/caac.21660
Tao, L., Xu, M., Dai, X., Ni, T., Li, D., Jin, F., et al. (2018). Polypharmacological profiles underlying the antitumor property of salvia miltiorrhiza root (danshen) interfering with NOX-dependent neutrophil extracellular traps. Oxid. Med. Cell. Longev. 2018, 4908328. doi:10.1155/2018/4908328
Tao, S., Justiniano, R., Zhang, D. D., and Wondrak, G. T. (2013). The Nrf2-inducers tanshinone I and dihydrotanshinone protect human skin cells and reconstructed human skin against solar simulated UV. Redox Biol. 1 (1), 532–541. doi:10.1016/j.redox.2013.10.004
Tao, S., Zheng, Y., Lau, A., Jaramillo, M. C., Chau, B. T., Lantz, R. C., et al. (2013a). Tanshinone I activates the Nrf2-dependent antioxidant response and protects against As(III)-induced lung inflammation in vitro and in vivo. Antioxid. Redox Signal. 19 (14), 1647–1661. doi:10.1089/ars.2012.5117
Tian, H., Li, Y., Mei, J., Cao, L., Yin, J., Liu, Z., et al. (2021). Effects of Salvia miltiorrhiza extract on lung adenocarcinoma. Exp. Ther. Med. 22 (2), 794. doi:10.3892/etm.2021.10226
Tian, Q. T., Ding, C. Y., Song, S. S., Wang, Y. Q., Zhang, A., and Miao, Z. H. (2018). New tanshinone I derivatives S222 and S439 similarly inhibit topoisomerase I/II but reveal different p53-dependency in inducing G2/M arrest and apoptosis. Biochem. Pharmacol. 154, 255–264. doi:10.1016/j.bcp.2018.05.006
Tong, W., Guo, J., and Yang, C. (2020). Tanshinone II A enhances pyroptosis and represses cell proliferation of HeLa cells by regulating miR-145/GSDMD signaling pathway. Biosci. Rep. 40 (4), BSR20200259. doi:10.1042/BSR20200259
Tsai, K. J., Tsai, H. Y., Tsai, C. C., Chen, T. Y., Hsieh, T. H., Chen, C. L., et al. (2021). Luteolin inhibits breast cancer stemness and enhances chemosensitivity through the nrf2-mediated pathway. Molecules 26 (21), 6452. doi:10.3390/molecules26216452
Tung, N. H., Nakajima, K., Uto, T., Hai, N. T., Long, D. D., Ohta, T., et al. (2017). Bioactive triterpenes from the root of salvia miltiorrhiza bunge. Phytother. Res. 31 (9), 1457–1460. doi:10.1002/ptr.5877
Verschooten, L., Barrette, K., Van Kelst, S., Rubio Romero, N., Proby, C., De Vos, R., et al. (2012). Autophagy inhibitor chloroquine enhanced the cell death inducing effect of the flavonoid luteolin in metastatic squamous cell carcinoma cells. PLoS One 7 (10), e48264. doi:10.1371/journal.pone.0048264
Wang, C., Li, Q., Xiao, B., Fang, H., Huang, B., Huang, F., et al. (2021). Luteolin enhances the antitumor efficacy of oncolytic vaccinia virus that harbors IL-24 gene in liver cancer cells. J. Clin. Lab. Anal. 35 (3), e23677. doi:10.1002/jcla.23677
Wang, H., Luo, Y., Qiao, T., Wu, Z., and Huang, Z. (2018). Luteolin sensitizes the antitumor effect of cisplatin in drug-resistant ovarian cancer via induction of apoptosis and inhibition of cell migration and invasion. J. Ovarian Res. 11 (1), 93. doi:10.1186/s13048-018-0468-y
Wang, H., Su, X., Fang, J., Xin, X., Zhao, X., Gaur, U., et al. (2018a). Tanshinone IIA attenuates insulin like growth factor 1 -induced cell proliferation in PC12 cells through the PI3K/Akt and MEK/ERK pathways. Int. J. Mol. Sci. 19 (9), E2719. doi:10.3390/ijms19092719
Wang, J., Li, Y., Wang, X., and Jiang, C. (2012). Ursolic acid inhibits proliferation and induces apoptosis in human glioblastoma cell lines U251 by suppressing TGF-β1/miR-21/PDCD4 pathway. Basic Clin. Pharmacol. Toxicol. 111 (2), 106–112. doi:10.1111/j.1742-7843.2012.00870.x
Wang, J., Ma, Y., Guo, M., Yang, H., and Guan, X. (2021a). Salvianolic acid B suppresses EMT and apoptosis to lessen drug resistance through AKT/mTOR in gastric cancer cells. Cytotechnology 73 (1), 49–61. doi:10.1007/s10616-020-00441-4
Wang, J. Y., and Chen, L. J. (2019). The role of miRNAs in the invasion and metastasis of cervical cancer. Biosci. Rep. 39 (3), BSR20181377. doi:10.1042/BSR20181377
Wang, L., Hu, T., Shen, J., Zhang, L., Chan, R. L., Lu, L., et al. (2015). Dihydrotanshinone I induced apoptosis and autophagy through caspase dependent pathway in colon cancer. Phytomedicine 22 (12), 1079–1087. doi:10.1016/j.phymed.2015.08.009
Wang, L., Yeung, J. H., Hu, T., Lee, W. Y., Lu, L., Zhang, L., et al. (2013). Dihydrotanshinone induces p53-independent but ROS-dependent apoptosis in colon cancer cells. Life Sci. 93 (8), 344–351. doi:10.1016/j.lfs.2013.07.007
Wang, L., Zhang, C., Guo, Y., Su, Z. Y., Yang, Y., Shu, L., et al. (2014). Blocking of JB6 cell transformation by tanshinone IIA: Epigenetic reactivation of Nrf2 antioxidative stress pathway. Aaps J. 16 (6), 1214–1225. doi:10.1208/s12248-014-9666-8
Wang, M., Zeng, X., Li, S., Sun, Z., Yu, J., Chen, C., et al. (2019). A novel tanshinone analog exerts anti-cancer effects in prostate cancer by inducing cell apoptosis, arresting cell cycle at G2 phase and blocking metastatic ability. Int. J. Mol. Sci. 20 (18), E4459. doi:10.3390/ijms20184459
Wang, Q. L., Tao, Y. Y., Yuan, J. L., Shen, L., and Liu, C. H. (2010). Salvianolic acid B prevents epithelial-to-mesenchymal transition through the TGF-beta1 signal transduction pathway in vivo and in vitro. BMC Cell Biol. 11, 31. doi:10.1186/1471-2121-11-31
Wang, R., Luo, Z., Zhang, H., and Wang, T. (2019a). Tanshinone IIA reverses gefitinib-resistance in human non-small-cell lung cancer via regulation of VEGFR/Akt pathway. Onco. Targets. Ther. 12, 9355–9365. doi:10.2147/OTT.S221228
Wang, S., Zhao, Y., Aguilar, A., Bernard, D., and Yang, C. Y. (2017). Targeting the MDM2-p53 protein-protein interaction for new cancer therapy: Progress and challenges. Cold Spring Harb. Perspect. Med. 7 (5), a026245. doi:10.1101/cshperspect.a026245
Wang, X. P., Wang, P. F., Bai, J. Q., Gao, S., Wang, Y. H., Quan, L. N., et al. (2019b). Investigating the effects and possible mechanisms of danshen- honghua herb pair on acute myocardial ischemia induced by isoproterenol in rats. Biomed. Pharmacother. 118, 109268. doi:10.1016/j.biopha.2019.109268
Wang, X., Yang, Y., Liu, X., and Gao, X. (2020). Pharmacological properties of tanshinones, the natural products from Salvia miltiorrhiza. Adv. Pharmacol. 87, 43–70. doi:10.1016/bs.apha.2019.10.001
Wang, X., Zhang, L., Dai, Q., Si, H., Zhang, L., Eltom, S. E., et al. (2021b). Combined luteolin and indole-3-carbinol synergistically constrains erα-positive breast cancer by dual inhibiting estrogen receptor alpha and cyclin-dependent kinase 4/6 pathway in cultured cells and xenograft mice. Cancers (Basel) 13 (9), 2116. doi:10.3390/cancers13092116
Wang, Y., Jin, W., and Wang, J. (2021c). Tanshinone IIA regulates microRNA-125b/foxp3/caspase-1 signaling and inhibits cell viability of nasopharyngeal carcinoma. Mol. Med. Rep. 23 (5), 371. doi:10.3892/mmr.2021.12010
Wang, Y., Zhang, Y., Chen, X., Hong, Y., and Wu, Z. (2018b). Combined treatment with myo-inositol and luteolin selectively suppresses growth of human lung cancer A549 cells possibly by suppressing activation of PDK1 and Akt. Nan Fang. Yi Ke Da Xue Xue Bao 38 (11), 1378–1383. doi:10.12122/j.issn.1673-4254.2018.11.17
Woo, J., Kim, H. Y., Byun, B. J., Chae, C. H., Lee, J. Y., Ryu, S. Y., et al. (2014). Biological evaluation of tanshindiols as EZH2 histone methyltransferase inhibitors. Bioorg. Med. Chem. Lett. 24 (11), 2486–2492. doi:10.1016/j.bmcl.2014.04.010
Wu, C. Y., Hsieh, C. Y., Huang, K. E., Chang, C., and Kang, H. Y. (2012). Cryptotanshinone down-regulates androgen receptor signaling by modulating lysine-specific demethylase 1 function. Int. J. Cancer 131 (6), 1423–1434. doi:10.1002/ijc.27343
Wu, D., Qiu, Y., Jiao, Y., Qiu, Z., and Liu, D. (2020). Small molecules targeting HATs, HDACs, and BRDs in cancer therapy. Front. Oncol. 10, 560487. doi:10.3389/fonc.2020.560487
Wu, H. T., Lin, J., Liu, Y. E., Chen, H. F., Hsu, K. W., Lin, S. H., et al. (2021). Luteolin suppresses androgen receptor-positive triple-negative breast cancer cell proliferation and metastasis by epigenetic regulation of MMP9 expression via the AKT/mTOR signaling pathway. Phytomedicine. 81, 153437. doi:10.1016/j.phymed.2020.153437
Wu, J., Xu, H., Zhang, L., and Zhang, X. (2016). Radix astragali and tanshinone help carboplatin inhibit B16 tumor cell growth. Technol. Cancer Res. Treat. 15 (4), 583–588. doi:10.1177/1533034615588682
Wu, S., Sun, C., Tian, D., Li, Y., Gao, X., He, S., et al. (2015). Expression and clinical significances of Beclin1, LC3 and mTOR in colorectal cancer. Int. J. Clin. Exp. Pathol. 8 (4), 3882–3891.
Xiang, F., Pan, C., Kong, Q., Wu, R., Jiang, J., Zhan, Y., et al. (2014). Ursolic acid inhibits the proliferation of gastric cancer cells by targeting miR-133a. Oncol. Res. 22 (5-6), 267–273. doi:10.3727/096504015X14410238486685
Xie, J., Liu, J. H., Liu, H., Liao, X. Z., Chen, Y., Lin, M. G., et al. (2016). Tanshinone IIA combined with adriamycin inhibited malignant biological behaviors of NSCLC A549 cell line in a synergistic way. BMC Cancer 16 (1), 899. doi:10.1186/s12885-016-2921-x
Xie, R., Li, Y., Tang, P., and Yuan, Q. (2018). Design, synthesis and biological evaluation of novel 2-aminobenzamides containing dithiocarbamate moiety as histone deacetylase inhibitors and potent antitumor agents. Eur. J. Med. Chem. 143, 320–333. doi:10.1016/j.ejmech.2017.08.041
Xin, M., Hao, Y., Huang, G., Wang, X., Liang, Z., Miao, J., et al. (2020). The efficacy and safety of salvianolic acids on acute cerebral infarction treatment: A protocol for systematic review and meta analysis. Med. Baltim. 99 (23), e20059. doi:10.1097/MD.0000000000020059
Xu, P., Wu, Q., Lu, D., Yu, J., Rao, Y., Kou, Z., et al. (2020). A systematic study of critical miRNAs on cells proliferation and apoptosis by the shortest path. BMC Bioinforma. 21 (1), 396. doi:10.1186/s12859-020-03732-x
Xu, Q. F., Peng, H. P., Lu, X. R., Hu, Y., Xu, Z. H., and Xu, J. K. (2021). Oleanolic acid regulates the Treg/Th17 imbalance in gastric cancer by targeting IL-6 with miR-98-5p. Cytokine 148, 155656. doi:10.1016/j.cyto.2021.155656
Xu, Z., Chen, L., Xiao, Z., Zhu, Y., Jiang, H., Jin, Y., et al. (2018). Potentiation of the anticancer effect of doxorubicinin drug-resistant gastric cancer cells by tanshinone IIA. Phytomedicine 51, 58–67. doi:10.1016/j.phymed.2018.05.012
Xu, Z., Han, X., Ou, D., Liu, T., Li, Z., Jiang, G., et al. (2020a). Targeting PI3K/AKT/mTOR-mediated autophagy for tumor therapy. Appl. Microbiol. Biotechnol. 104 (2), 575–587. doi:10.1007/s00253-019-10257-8
Xue, J., Jin, X., Wan, X., Yin, X., Fang, M., Liu, T., et al. (2019). Effects and mechanism of tanshinone II A in proliferation, apoptosis, and migration of human colon cancer cells. Med. Sci. Monit. 25, 4793–4800. doi:10.12659/MSM.914446
Yan, Y., Su, W., Zeng, S., Qian, L., Chen, X., Wei, J., et al. (2018). Effect and mechanism of tanshinone I on the radiosensitivity of lung cancer cells. Mol. Pharm. 15 (11), 4843–4853. doi:10.1021/acs.molpharmaceut.8b00489
Yang, P. W., Lu, Z. Y., Pan, Q., Chen, T. T., Feng, X. J., Wang, S. M., et al. (2019). MicroRNA-6809-5p mediates luteolin-induced anticancer effects against hepatoma by targeting flotillin 1. Phytomedicine 57, 18–29. doi:10.1016/j.phymed.2018.10.027
Yang, Y., Liu, L., Zhang, X., Jiang, X., and Wang, L. (2020). Tanshinone IIA prevents rifampicin-induced liver injury by regulating BSEP/NTCP expression via epigenetic activation of NRF2. Liver Int. 40 (1), 141–154. doi:10.1111/liv.14262
Yao, X., Jiang, W., Yu, D., and Yan, Z. (2019). Luteolin inhibits proliferation and induces apoptosis of human melanoma cells in vivo and in vitro by suppressing MMP-2 and MMP-9 through the PI3K/AKT pathway. Food Funct. 10 (2), 703–712. doi:10.1039/c8fo02013b
Yao, Y., Rao, C., Zheng, G., and Wang, S. (2019a). Luteolin suppresses colorectal cancer cell metastasis via regulation of the miR-384/pleiotrophin axis. Oncol. Rep. 42 (1), 131–141. doi:10.3892/or.2019.7136
Ye, T., Zhu, S., Zhu, Y., Feng, Q., He, B., Xiong, Y., et al. (2016). Cryptotanshinone induces melanoma cancer cells apoptosis via ROS-mitochondrial apoptotic pathway and impairs cell migration and invasion. Biomed. Pharmacother. 82, 319–326. doi:10.1016/j.biopha.2016.05.015
Ye, Y. T., Zhong, W., Sun, P., Wang, D., Wang, C., Hu, L. M., et al. (2017). Apoptosis induced by the methanol extract of Salvia miltiorrhiza Bunge in non-small cell lung cancer through PTEN-mediated inhibition of PI3K/Akt pathway. J. Ethnopharmacol. 200, 107–116. doi:10.1016/j.jep.2016.12.051
You, S., He, X., Wang, M., Mao, L., and Zhang, L. (2020). Tanshinone IIA suppresses glioma cell proliferation, migration and invasion both in vitro and in vivo partially through miR-16-5p/talin-1 (TLN1) Axis. Cancer Manag. Res. 12, 11309–11320. doi:10.2147/CMAR.S256347
Yu, F., Lu, Z., Chen, B., Wu, X., Dong, P., and Zheng, J. (2015). Salvianolic acid B-induced microRNA-152 inhibits liver fibrosis by attenuating DNMT1-mediated Patched1 methylation. J. Cell. Mol. Med. 19 (11), 2617–2632. doi:10.1111/jcmm.12655
Yu, F., Yu, C., Li, F., Zuo, Y., Wang, Y., Yao, L., et al. (2021). Wnt/β-catenin signaling in cancers and targeted therapies. Signal Transduct. Target. Ther. 6 (1), 307. doi:10.1038/s41392-021-00701-5
Yu, S., Guo, L., Yan, B., Yuan, Q., Shan, L., Zhou, L., et al. (2022). Tanshinol suppresses osteosarcoma by specifically inducing apoptosis of U2-OS cells through p53-mediated mechanism. J. Ethnopharmacol. 292, 115214. doi:10.1016/j.jep.2022.115214
Yun, S. M., Jung, J. H., Jeong, S. J., Sohn, E. J., Kim, B., and Kim, S. H. (2014). Tanshinone IIA induces autophagic cell death via activation of AMPK and ERK and inhibition of mTOR and p70 S6K in KBM-5 leukemia cells. Phytother. Res. 28 (3), 458–464. doi:10.1002/ptr.5015
Yuxian, X., Feng, T., Ren, L., and Zhengcai, L. (2009). Tanshinone II-A inhibits invasion and metastasis of human hepatocellular carcinoma cells in vitro and in vivo. Tumori 95 (6), 789–795. doi:10.1177/030089160909500623
Zachari, M., and Ganley, I. G. (2017). The mammalian ULK1 complex and autophagy initiation. Essays Biochem. 61 (6), 585–596. doi:10.1042/EBC20170021
Zahra, R., Furqan, M., Ullah, R., Mithani, A., Saleem, R. S. Z., and Faisal, A. (2020). A cell-based high-throughput screen identifies inhibitors that overcome P-glycoprotein (Pgp)-mediated multidrug resistance. PLoS One 15 (6), e0233993. doi:10.1371/journal.pone.0233993
Zeisberg, M., and Neilson, E. G. (2009). Biomarkers for epithelial-mesenchymal transitions. J. Clin. Invest. 119 (6), 1429–1437. doi:10.1172/JCI36183
Zeng, Z., Zhang, H., Wang, X., Liu, K., Li, T., Sun, S., et al. (2018). Salvianolic acid B suppresses cell proliferation and induces apoptosis in osteosarcoma through p38-mediated reactive oxygen species generation. Oncol. Lett. 15 (2), 2679–2685. doi:10.3892/ol.2017.7609
Zhang, B., Ma, Z., Li, X., Zhang, C., Shao, Y., Liu, Z., et al. (2016a). Tanshinones suppress non-small cell lung cancer through up-regulating miR-137. Acta Biochim. Biophys. Sin. 48 (8), 768–770. doi:10.1093/abbs/gmw053
Zhang, G., Yang, Y. M., Meng, W. T., and Zhou, J. (2010). Apoptosis of NB4 cells induced by Tanshinone II A combined with arsenic trioxide. Sichuan Da Xue Xue Bao Yi Xue Ban. 41 (1), 57–61.
Zhang, H. S., Zhang, F. J., Li, H., Liu, Y., Du, G. Y., and Huang, Y. H. (2016b). Tanshinone ⅡA inhibits human esophageal cancer cell growth through miR-122-mediated PKM2 down-regulation. Arch. Biochem. Biophys. 598, 50–56. doi:10.1016/j.abb.2016.03.031
Zhang, K., Li, J., Meng, W., Xing, H., and Yang, Y. (2016c). Tanshinone IIA inhibits acute promyelocytic leukemia cell proliferation and induces their apoptosis in vivo. Blood Cells Mol. Dis. 56 (1), 46–52. doi:10.1016/j.bcmd.2015.10.007
Zhang, L., Lin, W., Chen, X., Wei, G., Zhu, H., and Xing, S. (2019). Tanshinone IIA reverses EGF- and TGF-β1-mediated epithelial-mesenchymal transition in HepG2 cells via the PI3K/Akt/ERK signaling pathway. Oncol. Lett. 18 (6), 6554–6562. doi:10.3892/ol.2019.11032
Zhang, L., Liu, Q., Huang, L., Yang, F., Liu, A., and Zhang, J. (2020). Combination of lapatinib and luteolin enhances the therapeutic efficacy of lapatinib on human breast cancer through the FOXO3a/NQO1 pathway. Biochem. Biophys. Res. Commun. 531 (3), 364–371. doi:10.1016/j.bbrc.2020.07.049
Zhang, M., Wang, R., Tian, J., Song, M., Zhao, R., Liu, K., et al. (2021). Targeting LIMK1 with luteolin inhibits the growth of lung cancer in vitro and in vivo. J. Cell. Mol. Med. 25 (12), 5560–5571. doi:10.1111/jcmm.16568
Zhang, R. W., Liu, Z. G., Xie, Y., Wang, L. X., Li, M. C., and Sun, X. (2016d). In vitro inhibition of invasion and metastasis in colon cancer cells by TanIIA. Genet. Mol. Res. 15 (3). doi:10.4238/gmr.15039008
Zhang, W., Liu, C., Li, J., Lu, Y., Li, H., Zhuang, J., et al. (2022). Tanshinone IIA: New perspective on the anti-tumor mechanism of A traditional natural medicine. Am. J. Chin. Med. 50 (1), 209–239. doi:10.1142/S0192415X22500070
Zhang, X., Zhou, Y., and Gu, Y. E. (2019a). Tanshinone IIA induces apoptosis of ovarian cancer cells in vitro and in vivo through attenuation of PI3K/AKT/JNK signaling pathways. Oncol. Lett. 17 (2), 1896–1902. doi:10.3892/ol.2018.9744
Zhang, Y., Ge, T., Xiang, P., Zhou, J., Tang, S., Mao, H., et al. (2019b). Tanshinone IIA reverses oxaliplatin resistance in human colorectal cancer via inhibition of ERK/Akt signaling pathway. Onco. Targets. Ther. 12, 9725–9734. doi:10.2147/OTT.S217914
Zhang, Y., Geng, Y., He, J., Wu, D., Zhang, T., Xue, L., et al. (2019c). Tanshinone IIA induces apoptosis and autophagy in acute monocytic leukemia via downregulation of PI3K/Akt pathway. Am. J. Transl. Res. 11 (5), 2995–3006.
Zhang, Y., Guo, S., Fang, J., Peng, B., Zhang, Y., and Cao, T. (2018). Tanshinone IIA inhibits cell proliferation and tumor growth by downregulating STAT3 in human gastric cancer. Exp. Ther. Med. 16 (4), 2931–2937. doi:10.3892/etm.2018.6562
Zhang, Y., Jiang, P., Ye, M., Kim, S. H., Jiang, C., and Lü, J. (2012). Tanshinones: Sources, pharmacokinetics and anti-cancer activities. Int. J. Mol. Sci. 13 (10), 13621–13666. doi:10.3390/ijms131013621
Zhao, W., Feng, H., Guo, S., Han, Y., and Chen, X. (2017). Danshenol A inhibits TNF-α-induced expression of intercellular adhesion molecule-1 (ICAM-1) mediated by NOX4 in endothelial cells. Sci. Rep. 7 (1), 12953. doi:10.1038/s41598-017-13072-1
Zhao, X., Liu, M., and Li, D. (2015). Oleanolic acid suppresses the proliferation of lung carcinoma cells by miR-122/Cyclin G1/MEF2D axis. Mol. Cell. Biochem. 400 (1-2), 1–7. doi:10.1007/s11010-014-2228-7
Zhao, Y. X., Luo, D., Zhang, Y. H., Shen, B., Wang, B. X., and Sun, Z. F. (2017a). The effect of tanshinone ⅡA potentiates the effects of Cisplatin in Fadu cells in vitro through downregulation of survivin. Lin. Chung Er Bi Yan Hou Tou Jing Wai Ke Za Zhi 31 (10), 781–784. doi:10.13201/j.issn.1001-1781.2017.10.011
Zhao, Z., and Shilatifard, A. (2019). Epigenetic modifications of histones in cancer. Genome Biol. 20 (1), 245. doi:10.1186/s13059-019-1870-5
Zheng, L., Guan, Z. J., Pan, W. T., Du, T. F., Zhai, Y. J., and Guo, J. (2018). Tanshinone suppresses arecoline-induced epithelial-mesenchymal transition in oral submucous fibrosis by epigenetically reactivating the p53 pathway. Oncol. Res. 26 (3), 483–494. doi:10.3727/096504017X14941825760362
Zheng, L., Zhang, Y., Liu, G., Cheng, S., Zhang, G., An, C., et al. (2020). Tanshinone I regulates autophagic signaling via the activation of AMP-activated protein kinase in cancer cells. Anticancer. Drugs 31 (6), 601–608. doi:10.1097/CAD.0000000000000908
Zhou, J., Jiang, Y. Y., Chen, H., Wu, Y. C., and Zhang, L. (2020). Tanshinone I attenuates the malignant biological properties of ovarian cancer by inducing apoptosis and autophagy via the inactivation of PI3K/AKT/mTOR pathway. Cell Prolif. 53 (2), e12739. doi:10.1111/cpr.12739
Zhou, J., Jiang, Y. Y., Wang, H. P., Chen, H., Wu, Y. C., Wang, L., et al. (2020a). Natural compound Tan-I enhances the efficacy of Paclitaxel chemotherapy in ovarian cancer. Ann. Transl. Med. 8 (12), 752. doi:10.21037/atm-20-4072
Zhou, J., Jiang, Y. Y., Wang, X. X., Wang, H. P., Chen, H., Wu, Y. C., et al. (2020b). Tanshinone IIA suppresses ovarian cancer growth through inhibiting malignant properties and angiogenesis. Ann. Transl. Med. 8 (20), 1295. doi:10.21037/atm-20-5741
Zhou, L., Sui, H., Wang, T., Jia, R., Zhang, Z., Fu, J., et al. (2020c). Tanshinone IIA reduces secretion of pro-angiogenic factors and inhibits angiogenesis in human colorectal cancer. Oncol. Rep. 43 (4), 1159–1168. doi:10.3892/or.2020.7498
Zhou, X., Lv, L., Tan, Y., Zhang, Z., Wei, S., and Xiao, S. (2021). Tanshinone IIA sensitizes TRAIL-induced apoptosis in glioblastoma through inducing the expression of death receptors (and suppressing STAT3 activation). Brain Res. 1766, 147515. doi:10.1016/j.brainres.2021.147515
Zou, S., Tong, Q., Liu, B., Huang, W., Tian, Y., and Fu, X. (2020). Targeting STAT3 in cancer immunotherapy. Mol. Cancer 19 (1), 145. doi:10.1186/s12943-020-01258-7
Keywords: Salvia miltiorrhiza, microRNA, apoptosis, histone modifications, combination medication, cancer
Citation: Lu M, Lan X, Wu X, Fang X, Zhang Y, Luo H, Gao W and Wu D (2022) Salvia miltiorrhiza in cancer: Potential role in regulating MicroRNAs and epigenetic enzymes. Front. Pharmacol. 13:1008222. doi: 10.3389/fphar.2022.1008222
Received: 31 July 2022; Accepted: 24 August 2022;
Published: 12 September 2022.
Edited by:
Peng Qu, National Institutes of Health (NIH), United StatesReviewed by:
Dennis Mans, Anton de Kom University of Suriname, SurinameXuejun Jin, Yanbian University, China
Ming Zhang, Shanghai Jiao Tong University, China
Copyright © 2022 Lu, Lan, Wu, Fang, Zhang, Luo, Gao and Wu. This is an open-access article distributed under the terms of the Creative Commons Attribution License (CC BY). The use, distribution or reproduction in other forums is permitted, provided the original author(s) and the copyright owner(s) are credited and that the original publication in this journal is cited, in accordance with accepted academic practice. No use, distribution or reproduction is permitted which does not comply with these terms.
*Correspondence: Wenyi Gao, Z2Fvd3lAY2N1Y20uZWR1LmNu; Donglu Wu, d3VkbDEwMjBAMTYzLmNvbQ==
†These authors have contributed equally to this work