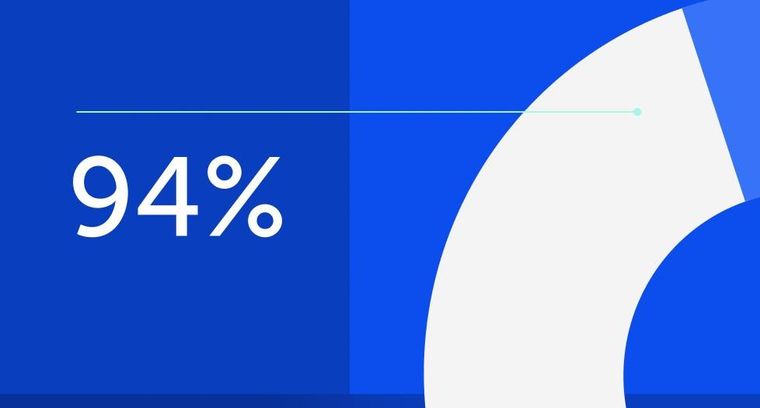
94% of researchers rate our articles as excellent or good
Learn more about the work of our research integrity team to safeguard the quality of each article we publish.
Find out more
REVIEW article
Front. Pediatr., 30 September 2021
Sec. Pediatric Neurology
Volume 9 - 2021 | https://doi.org/10.3389/fped.2021.753215
This article is part of the Research TopicUpdates on the Neuropathology of Sudden Unexplained Perinatal Death and other Neurodevelopmental DisordersView all 15 articles
Neonatal survival requires precise control of breathing and cardiovascular action, with fatal consequences or severe injury without support. Prematurity presents multiple opportunities to disrupt cardiorespiratory regulation, leading to expressions of apnea of prematurity, periodic breathing, and inappropriate cardiovascular responses to apnea. Failed breathing control can result from altered breathing drives, typically arising from untimely development of sensory or motor coordination processes. Some drives, such as temperature, are a special concern in neonates with low body mass, enhancing susceptibility to rapid body cooling. Chemical drives, such as pH or CO2 or O2, may be inadequately developed; in some conditions, such as congenital central hypoventilation syndrome (CCHS), breathing responses to CO2 or low O2 may be reduced or absent, and coupling of cardiovascular responses to breathing changes are abolished. Sleep states exert profound influences on both chemical and temperature drives, with rapid eye movement (REM) sleep potentially modifying descending temperature influences, and state transitions significantly altering respiratory responses to chemical stimuli. In addition, neonates spend the majority of time in REM sleep, a state which induces a generalized inhibition of skeletal muscle activity that abolishes muscle tone to upper airway and thoracic wall muscles, enhancing the likelihood for obstructive sleep apnea. Although disrupted regulatory drives can often be replaced by positive (or negative) pressure ventilation, such as continuous positive airway pressure or enhanced by manipulating neurotransmitter action via caffeine, those approaches may exert negative consequences in the long term; the lungs of neonates, especially premature infants, are fragile, and easily injured by positive pressure. The consequences of caffeine use, acting directly on neural receptors, although seemingly innocuous in the near-term, may have long-term concerns and disrupts the integrity of sleep. The developmental breathing field needs improved means to support ventilation when one or more drives to respiration fail, and when the cardiovascular system, depending heavily on interactions with breathing, is compromised. Neuromodulatory procedures which manipulate the vestibular system to stabilize breathing or use tactile or proprioceptive stimuli to activate long-established reflexive mechanisms coupling limb movement with respiratory efforts can provide support for central and obstructive apnea, as well as for periodic breathing and cardiovascular action, particularly during sleep.
The rate of breathing and extent of air exchange in infants is normally controlled by sensing CO2 and O2, by core body temperature, and by voluntary breathing efforts, all of which are partially modulated by sensory feedback of lung and muscle stretch as well as by afferent signals from airflow. However, some respiratory drives, such as those from CO2 or O2 sensing, can be diminished, lost, or shifted in timing by altered development, or by genetic malformations, as in congenital central hypoventilation syndrome CCHS (1), or by neural trauma in the neonatal period (2). CCHS subjects show loss of CO2 and O2 sensing (3), as well as impaired temperature control on breathing. Other disease processes, such as Covid-19, can be accompanied by loss of the perception of dyspnea, inappropriate responses to CO2 or low O2, resulting in “tolerant tachypnea” and “happy hypoxemia” (4, 5).
Some instances of impaired breathing, particularly during sleep, result from disrupted cerebellar interactions with other regulatory structures, particularly the parabrachial pons. Stroke, especially with cerebellar injury, or other developmental injury with cerebellar involvement, e.g., Joubert's syndrome, is frequently accompanied by airway obstruction (2, 6), an outcome often a consequence of a loss of coordination, a principal cerebellar function, between reduced drives to upper airway muscles but continued diaphragmatic action, i.e., flaccid oropharyngeal muscles in the presence of negative thoracic pressures from a descending diaphragm. The loss of motor influences to upper airway muscles during the paralysis of REM sleep can thus result in obstructive sleep apnea (OSA). The parabrachial pons exerts a pivotal role in these sequences, coordinating signals from the cerebellum, vagal and thoracic afferents, integrating temperature and other input from more-rostral brain influences, while providing respiratory phase switching and state arousal actions (7). The state arousal functions are critical in recovery from some forms of apnea in developing infants.
The impact of sleep on reduced or distorted breathing drives extends to state influences on chemical (8), or temperature influences on breathing in early development, and can contribute to apnea of prematurity. Distortion in timing of respiratory drive influences, such as altered timing between central and peripheral chemoreceptor integration, can lead to periodic breathing. Some drives supporting breathing are lost during sleep, including voluntary breathing efforts, and the so-called “wakefulness stimulus to breathe” (9). Temperature influences on breathing, an important consideration in infants with low body mass and thus susceptible to low or high environmental temperatures, are a concern. Temperature drives are diminished during REM sleep in young or adult feline preparations (10, 11). Those REM-related effects apparently differ in premature human infants (12).
Premature infants are at high risk for cerebellar injury (hemorrhages, infarctions, and intermittent hypoxic exposure), which can compromise cerebellar growth and function (13, 14). Such cerebellar injury, seizures, or hypoxic damage can alter respiratory motor timing circuitry, or delay circulation, leading to a mismatch of central and peripheral chemoreception and modify timing of inspiratory signaling to respiratory muscles, all of which are heavily dependent on the integrity of deep cerebellar nuclei and control influences on those nuclei (15). These functional distortions can result in periodic breathing, a start-stop respiratory patterning leading to intermittent hypoxia exposure that is injurious to brain tissue (16). Such disturbed breathing patterns are common in premature infants, and can appear in full-term neonates. Severe inflammatory processes and white matter injury can occur as well (17). Appropriate and sustained inspiratory and expiratory timing must be restored to minimize periodic breathing, together with reclamation or replacements for breathing drives; that timing is heavily dependent on intact cerebellar and parabrachial pontine processes (7).
The potential contributors to enhanced breathing efforts with exercise has been a target of respiratory physiologists for over a century. Increased ventilation to accommodate the additional metabolic demands is one possibility, but timing of the enhanced efforts before metabolic demands have built up argue against that interpretation. The range of potential mechanisms has been reviewed in detail (18), and that review described multiple processes that may be operating, with no single process providing a unifying, definitive mechanism.
A loss of drive from chemoreceptor sources, i.e., high CO2 or low pH, or from low O2, or insufficient temperature influences or loss of the “waking stimulus” during sleep can be overcome by recruiting a phylogenetically old reflex which couples limb locomotion with increased breathing-muscle activity. The coordination between limb movement and breathing can be easily observed in animals; a popular respiratory tale is that the distinguished French physiologist, DeJours, who loved horse racing, even in cold weather, observed synchrony between the visible expired air of horses with forward movement of the hoofs during running. Such coupling of ventilation to exercise has repeatedly been demonstrated (19, 20). In humans, children with CCHS, who express a mutation in PHOX2B leading to loss of sensitivity to CO2 and O2 (3), as well as poor temperature control (21), provide an “Experiment of Nature” which illustrates the role of movement to enhance breathing. Left to sit passively, as in watching television or playing a video game, CCHS patients fail to breathe and will rapidly lose oxygenation until provoked to do so by their caretaker to voluntarily breathe (voluntary breathing drives remain intact in CCHS children). However, if such children actively move about, as in playing soccer, they ventilate adequately (22). The limb movement can be passive; simple back and forth movement of the foot will sustain breathing, and does so, even during sleep (20, 23).
The coupling of movement with breathing provides species-survival benefits. The reflex immediately augments breathing in response to locomotion when the organism is threatened, and time is not available to enhance CO2 or other chemical processes usually used to increase ventilation. The independence from CO2 stimulation is an important aspect in premature infants with apnea of prematurity, because ventilatory responses to increasing CO2 may be poorly developed, secondary to diminished central sensitivity to CO2.
The action of both foot and limb movements can enhance breathing efforts; reflexive coupling of breathing with limb movements developed early phylogenetically, and is present in all four limbs used for locomotion. Thus, in humans, movement of both the upper and lower limbs can participate in enhancing ventilation, an aspect of importance in intervening for hypoventilation in spinal cord injured patients, where proprioceptive signaling from lower limbs may be lost (24). Proprioceptive signals from muscles and tendons of the hand and feet travel via peripheral neural pathways to the spinal cord, and then travel up the cord via spinocerebellar pathways to the brain stem and cerebellum (25).
Although selected neurons in the brainstem have respiratory pacemaker qualities to influence breathing rate, the timing and extent of those breathing signals depend heavily on multiple inputs, including descending forebrain signals, which include temperature influences from the hypothalamus and affective signals from the amygdala (26), as well as afferent signals from the lung, vascular system, and thoracic wall (18). Timing and extent of those signals are coordinated by cerebellar and pontine processes which integrate signaling to nuclei mediating breathing in the medulla and pons (20, 25, 27–29). Nuclei within the medulla, in turn, send signals to the phrenic motor pools C3, C4, and C5 of the spinal cord that innervate the diaphragm, cervical and thoracic spinal nerves serving ancillary respiratory intercostal muscles, and to the hypoglossal nuclei supplying the genioglossal fibers, the protruder (dilating) muscles of the tongue (30). The processes which maintain regularity in breathing, as well as momentary processes in respiratory effort depend on multiple inputs from forebrain and brainstem sites, as well as peripheral thoracic and oral airway sensory input. The different inputs require synchronization between inputs, and timely integration to maintain sufficient drive to maintain breathing, cessation of efforts when appropriate, and appropriate interactions with cardiovascular action to maintain appropriate perfusion.
Several major issues confront breathing control in neonatal sleep; one is to prevent obstructive sleep apnea (OSA). A set of oro-pharyngeal muscles participate in OSA, with the tongue genioglossal muscles being principal dilators maintaining upper airway patency, and thus, a key target for OSA intervention (30). For neonatal breathing, maintaining airway patency is key to preventing OSA, but such maintenance is a concern with the generalized somatic muscle paralysis of REM sleep. The REM paralysis leads to flaccid upper airway musculature; however, diaphragmatic movements are maintained in REM sleep, producing high negative airway pressure, with the danger of collapsing the upper airway musculature, resulting in airway occlusion. Prevention of upper airway occlusion during REM sleep is thus dependent on maintaining upper airway muscle tone as well as supplying appropriate phasic timing of that tone to the muscles, i.e., dilating the airway slightly ahead of diaphragmatic descent and its resulting negative pressure.
A second concern in newborn breathing is protection against loss of temperature influences during the REM sleep reduction of descending hypothalamic influences. Respiratory rate is partially dependent on core temperature, which provides a significant drive to breathing in an infant with low body mass, making it susceptible to rapid core cooling when unclothed or placed in other low temperature conditions. These issues have been largely addressed in neonatal intensive care units with use of servo-controlled incubators as well as heated and humidified airflow to maintain normothermia; however, in less-developed or less-supportive circumstances, temperature control remains an issue.
A third concern is the alteration in impact of chemical sensing that accompanies state changes (8). In premature infants with apnea of prematurity, ventilatory responses to increasing CO2 are immature, secondary to diminished central sensitivity to CO2 (31–33). In some conditions, such as CCHS, chemical sensing of CO2 is sufficiently reduced that affected children need to be mechanically ventilated during sleep. Non-CCHS children also undergo less extreme state-related breathing responses to CO2 sensing, but the potential for impaired sensitivity with very early development remains a concern. Timing of inspiratory and expiratory efforts involves a complex interaction of integrating descending signals from rostral brain areas, such as affective regions of the amygdala and temperature influences from the hypothalamus, and afferent signals from stretch receptors of the lung. The cerebellum and parabrachial pons exert a coordination role in integration of these multiple inputs, especially through amygdala projections to the parabrachial pons, descending hypothalamic influences, and lung afferent projections.
Periodic breathing patterns result in successive exposure to intermittent hypoxia with each stopped-breath episode; in infants, the resulting hypoxia leads to desaturations to very low levels, with rapid restoration to normoxia with onset of the next burst of respiratory efforts. Although periodic breathing is sometimes ignored in neonates, the consequences to the brain and other structures can be severe. The repeated desaturation/reoxygenation sequence is injurious to brain, visceral and pancreatic tissue in animal models, since periodic breathing patterns are essentially intermittent hypoxia exposures; exposure to repeated intermittent hypoxia even for short periods of a few hours results in severe cerebellar injury (16), and damage to medullary and peripheral cardiovascular integrative sites (34). In addition, reduced bone development occurs (35), as well as damage to structures mediating glucose control (36). Intermittent hypoxia episodes in human neonates lead to acute and chronic morbidities, including retinopathy of prematurity, impaired growth and cardiovascular regulation, bronchopulmonary dysplasia, sleep disordered breathing and neurodevelopmental disabilities (37–41).
Interventions to maintain ventilation in the neonatal period are few, and have the potential to impose injurious consequences. Continuous positive airway pressure (CPAP) procedures in infants pose risks, especially in the long term due to the fragility of the lungs in neonates (42) as well as concurrent bone distortion from facial masks on facial structures in early development (43). The consequences of using CPAP to manage periodic breathing in adults raise other concerns, although these aspects have not been fully explored in neonates. In adults, CPAP is often ineffective to stop Cheyne-Stokes breathing in heart failure patients (44, 45). In sleep-disordered breathing patients, major cardiovascular issues arise from use of servo-controlled positive pressure ventilation in those with periodic or Cheyne-Stokes breathing; positive pressure ventilation use leads to increased mortality in those with such breathing patterns [for rationale, see (46)]. Although adult conditions may not exactly parallel those encountered in infants, the absence of data during early development should not reduce concern of consequences of such interventions.
A common intervention to support neonatal breathing is to use methylxanthines, including caffeine, aminophylline, or theophylline, to enhance breathing drives, principally through neurotransmitter arousal processes. The benefits of caffeine, especially with early use reducing bronchopulmonary dysplasia, have been described (47). However, the long-term consequences of such use remain unclear; although a large study (48), found little change of sleep characteristics or obstructive sleep apnea in later childhood following treatment of caffeine or positive pressure ventilation to premature infants. However, concomitant arousal effects of caffeine enhance the risk for diminished sleep state integrity in prematurity, with unpredictable consequences to later cognitive development (49, 50). Thus, interventions to support breathing in premature infants might consider options other than caffeine or positive pressure ventilation to address issues of maintenance of respiratory muscle tone to prevent airway collapse, stimulation to respiratory drives to avoid central apnea, as well as synchronization of control systems to prevent periodic breathing.
Several types of impaired breathing must be considered in neonates and young infants. The first relates to loss of one or more critical drives that lead to hypoventilation or central apnea, a pattern typically found with loss of chemical sensing or temperature drive, a second relates to airway obstruction from loss of drive to the upper airway muscles with continued diaphragmatic efforts, a pattern commonly found in REM sleep or with cerebellar injury (2), and a third type of periodic breathing which stems from a loss of coordination or timing of central drive on both upper airway and diaphragmatic musculature. A solution to the first two of these issues would be to substitute a missing drive by enhancing the action by another, perhaps less commonly used influence. Correction of periodic breathing can be accomplished by assisting cerebellar-pontine mechanisms in coordination actions to overcome the stop-start patterns of periodic breathing, and stabilizing the regularity of breathing patterns. There are rather simple means to implement both solutions.
The evidence for coupling of breathing and limb movement processes derives from both animal physiological studies and human functional magnetic resonance studies (fMRI). We used fMRI procedures to validate in humans that lower limb proprioceptive stimulation activates cerebellar and parabrachial pontine structures (51) (Figure 1, left and right circled areas). In addition, the cortical area representing foot movement (Figure 1A) is activated, as is the cortical cervical motor area for the diaphragm (Figure 1B) (cervical nerves 3, 4, and 5 innervate the diaphragm).
Figure 1. Cerebellar and parabrachial pontine nuclei are activated by limb proprioceptive sensory stimulation. Functional MRI images following passive right foot movement in 14 children activated the parabrachial pons (Left circled area) and medial cerebellum (right circled area), sites involved in respiratory timing and blood pressure control. The sensorimotor cortex for the foot (A) and for the cervical region (for diaphragm), (B) are also activated, indicating that locomotion (foot) and breathing motor sites are concurrently recruited by the proprioceptive stimuli. From (51).
Any intervention to support breathing must consider both the drive to upper airway muscles as well as timing of drive to those muscles. The drive aspect requires the full array of influences from airflow sensors, temperature influences, lung stretch receptors and CO2 sensors for appropriate action, any of which can be poorly developed in infants. The timing aspects rely on appropriate development of cerebellar and pontine interactive circuitry, as well as chemical sensing from the periphery and central chemosensors, both of which can be distorted in timing during newborn development (52).
A long history of experimental evidence indicates that a variety of “non-classical respiratory” sensory inputs can stabilize breathing patterns. Those interventions can take the form of detecting apnea, and then introducing an arousing stimulus, typically through vibration to recruit the “wakefulness stimulus” and thus enhance breathing (53). Cutaneous stimulation through rubbing of peripheral limbs has been successfully used (54), and whole-body vibratory stimulation has also been applied intermittently through vibratory mattresses (55), and lessens apnea. Vestibular stimuli, applied by oscillating a water bed (56), or manual rocking (57) show remarkable effectiveness in stabilizing breathing. There are obvious logistic issues with continuous manual rubbing intervention, incorporating whole-bed vibration, or rocking, but the interventional studies indicate that efforts to restore breathing do not necessarily have to depend on manipulating chemosensitive processes or altering arousal-related neurotransmitters through methylxanthines, or using potentially injurious positive pressure ventilation techniques.
One solution to providing another breathing drive is to use a phylogenetically old reflex that links limb locomotion with increased breathing muscle activity; running imposes increased ventilatory demands for metabolic reasons, and breathing must often increase immediately, e.g., to escape from a predator, with no time available to build up CO2 signaling to breathe faster. This reflex thus bypasses the normal, but sometimes lost or reduced chemosensing and temperature drives that can occur in neonatal life or in genetic errors, such as CCHS. CCHS provides an “experiment of Nature” that allows evaluation of other means to support breathing; the condition, a consequence of PHOX2B mutation, shows an absence of CO2 and O2 sensitivity (3) and impaired temperature regulation, among other autonomic deficits (21). The neuromodulatory aspects of breathing support for diminished chemosensitivity were partially drawn from fMRI studies of CCHS patients who showed the reduced participation of defined cerebellar and parabrachial pontine areas to CO2 challenges (58).
Early recognition of the limb movement/breathing relationship in CCHS sprung from studies relating body movement or passive limb movement to breathing (22, 59); CCHS patients who would turn blue if watching television, would ventilate normally if playing soccer. Even passive foot movement during sleep was effective in supporting breathing (23). The locomotion-breathing reflex recruits not only the diaphragm but also the upper airway and thoracic wall muscles, and can overcome the other-than-diaphragm respiratory muscle paralysis of REM sleep as well as alterations in temperature and chemical senses of that sleep state. The recruitment of upper airway muscles in addition to the diaphragm overcomes the possibility of causing obstructive apnea—unlike phrenic nerve stimulation which can result in upper airway collapse by generating a too-high negative pressure (60).
The intervention also assists timing of breathing by directly activating the cerebellum and parabrachial pons, brain sites that coordinate proprioceptive and other input to synchronize activity of the respiratory muscles. This timing coordination is critical for resolving periodic breathing, which typically results from a temporal mismatch of CO2 sensing between the carotid and central chemoreceptors. CCHS patients show profound injury in cerebellar sites (21, 58, 61, 62), likely a consequence of the PHOX-2B relationships to that structure and unintended failure of ventilory support, triggering hypoxic episodes. The demonstration that foot movement can recruit enhanced breathing efforts is useful to show the principle of the phylogenetically old reflex, but that physical process of movement is obviously impractical for neonatal use. However, a variety of electrical or mechanical means can be used to simulate foot or hand movement, i.e., activate proprioceptive fibers to “trick” the brain into triggering the limb movement-breathing coupling reflex. Vibration of the foot or hand will activate proprioceptive fibers carried to the pontine and cerebellar nuclei, simultaneously recruiting action in breathing nuclei of the medulla and pons, and thus activating upper airway muscles, thoracic wall muscles, and the phrenic motor pool. These processes can thus provide a “drive” to those breathing muscles, and by activating upper airway muscles, overcome the potential for obstructive sleep apnea. In addition, the diaphragmatic and thoracic wall musculature which fail in central apnea can be excited (63, 64). The neuromodulatory procedure may have particular value in other conditions, such as drug-resistant epilepsy, where a concern exists for sudden unexpected death in epilepsy (SUDEP), an outcome typically dependent on the frequency of seizures, with such seizures often accompanied by hypoxic or hypotensive periods (65).
Recruitment of proprioceptive fibers to activate breathing requires only simple vibration to mechanical receptors of the limbs. Such a device is shown in Figure 2. The objective is to apply vibration to the sole of the foot (or palm of the hand) to activate proprioceptive nerve signals, normally activated by walking or running, that rise through spinal pathways to the pons and cerebellum (Figure 2A). The cerebellar and parabrachial pontine sites coordinate activation of oropharyngeal muscles, including the genioglossal fibers of the tongue and muscles of the diaphragm. The vibratory device consists of small vibratory motors (Figure 2B) that are taped to the sole of the foot by tape. The vibratory motors, attached to the leads, are powered by two alkaline batteries, fused for safety, from a power supply box (Figure 2C), providing 1.5 or 3.0 volts power (switchable). The motors provide two levels of vibration amplitude at 128 Hz, a standard vibratory signal used to elicit reflexes in neurological testing. The waveform and frequency characteristics were determined after extensive empirical trials, with attention to tolerance of vibratory levels (66).
Figure 2. (A) Placement of vibrator motor on the sole of the foot; since the limb movement/breathing reflex is operative on all four limbs (from early origins of the reflexive coupling), placement on the palm of the hand is also effective. The activated proprioceptive fibers project to the cerebellum and parabrachial pons, that in turn, integrate with motor action to the upper airway and diaphragm. (B) Vibrator motor with power lead. (C) Power supply containing two 1.5-volt fused AA batteries with two levels of vibratory stimulation. From (65).
By providing excitation to the cerebellum and parabrachial pons, timing and synchronizing effects are enhanced through proprioceptive stimulation of the limbs, and thus can reduce or abolish periodic breathing, a major concern in premature neonates, since such respiratory patterns result in serious intermittent hypoxia. The intervention can also improve cardiovascular aspects accompanying apnea, reducing bradycardia associated with the stopped-breathing periods, and assisting perfusion.
The usefulness of the intervention in managing periodic breathing can be seen in Figure 3, which shows breathing in an awake CCHS child with and without the vibratory device. The CCHS patient desaturates to low 70% values, but those values remain at full saturation with stimulation.
Figure 3. Stimulation of proprioceptive fibers of the foot of a pediatric (4 years) CCHS patient without (top) and with the device (Stim On). Note the decline in O2 saturation (shaded O2 saturation areas following cessation of breathing efforts) during no stimulation, and the complete abolition of periodic breathing and restored saturation with stimulation. Clinical data courtesy of Dr. M. Woo [Derived from (66)].
The device applies transcutaneous vibration to the soles of the foot and palms of the hand to elicit nerve signaling from pressure and other limb proprioceptor sensors to pontine, cerebellar, and medullary brain areas that coordinate limb movement and reflexively activate brain areas controlling breathing. The procedure enhances a reflexive drive to breathing when other breathing drives fail from disease processes or during sleep. The device is of use in obstructive sleep apnea, central apnea, periodic breathing, and hypoventilation, and will also normalize extremes of change in blood pressure to respiratory events (63, 64, 66).
It is important to note that the mode of action used by recruitment of reflexive breathing drives differs from the intuitive mechanism of arousing the apneic subject to restore the “wakefulness” drive to breathe. Such an approach can be effective for restarting breathing, but repeatedly arouses the subject, making a night's sleep not restful. Instead, the principle is to recruit an ancient reflexive drive that maintains sleep integrity (24, 63).
The safety, suitability and efficacy of the procedure has been shown in a premature infant trial (63), that demonstrates a significant reduction in number and duration of long breathing pauses and intermittent hypoxic events, as well as the number and duration of bradycardic events (Figures 4, 5). The intervention provides an ancillary drive to breathing which replaces missing drives during development, allowing stabilization of breathing, diminishing apnea, and reducing extreme changes in cardiovascular patterns accompanying breathing pauses.
Figure 4. Vibratory proprioceptive stimulation in 15 infants with apnea of prematurity reduced intermittent hypoxic events (IH) by 33% and breathing pauses by 40%. *p <0.05. From (63).
Figure 5. Limb proprioceptive stimulation in premature infants reduced the total number (A) and the total duration (B) of mild (<110 bpm) and moderate (<100 bpm) bradycardic episodes from pre-stimulation periods. A 3-fold reduction in total duration of both mild and moderate bradycardia episodes occurred. *p < 0.05. From (63).
The concerns for breathing support in neonates also extend to adults with sleep-disordered breathing. The principal intervention has been CPAP, which has serious deficiencies in patient adoption, since the devices are uncomfortable, are often noisy, are not compact, making travel difficult, has humidity control issues, and long-term support for blood pressure is not assured (67, 68), and can be dangerous for periodic breathing use (46). Mandibular positioning devices have been adopted for mild or moderate adult OSA, but are not considered for neonatal cases, and are often considered inadequate for severe adult apnea. Such devices pose a potential for temporal mandibular joint injury (from forward positioning of the mandible), and do nothing for central apnea (in conditions where patients lack central drive to breathe for all respiratory muscles, not just upper airway musculature). Genioglossal/hypoglossal nerve stimulation requires invasive surgical implantation of an electrical stimulation device, coupled with placing leads to the 12th cranial nerve or to genioglossal fibers of the tongue. The procedure is inappropriate for neonates. The surgery is expensive, is coupled with a risk for infection, damage to nerves and other tissue from stimulation leads, and may require multiple surgical interventions to restore the subcutaneous power supply of the implanted device. Mechanical positive pressure ventilation can be used, but also poses a concern of injury to delicate or compromised lungs, such as those in young or medically-compromised patients.
Disordered breathing in early life can lead to impaired oxygenation, often with intermittent hypoxia exposure, which can induce severe injury to multiple brain sites. Disordered breathing can result from failure of chemical, temperature, or state-related motoric drives; particular sleep states enhance the appearance of some of these failures. Alterations in drives can further compromise breathing by delayed timing or inappropriate coordination of afferent influences, or disrupt integration of sensory input with motor outflow to upper airway and diaphragmatic muscles. Multiple interventions have been used to demonstrate how potential injury from positive pressure procedures or bypassing caffeine administration can be avoided. These interventions attempt to modulate sensory input, include tactile and vibratory bed stimulation, and often rely on recruiting arousal actions to restore breathing. Vestibular input, applied through oscillatory water beds or rocking, has been employed successfully to exert excitatory vestibular/cerebellar processes to activate and synchronize breathing with motion input. Loss of breathing drives can be overcome by enhancing ancient reflexive interactions between limb locomotion and respiration, and that enhancement can be accomplished artificially by proprioceptive activation, “tricking” the brain into perceiving that the limbs are moving. If sufficient stimulation is provided, the proprioceptive signals can enhance cerebellar and pontine processing to improve coordination of integrative processes and abolish periodic breathing.
RH and KK contributed equally to the design and implementation of the research and findings outlined in this manuscript. Both authors contributed to the article and approved the submitted version.
This research was supported by grants from the UCLA Children's Discovery and Innovation Institute's Seed Grant award, Today's and Tomorrow's Children's Fund Award, 2016 and Little Giraffe Foundation NICU Support Grant, 2016 to KK and by the Fidelity Charitable Nancy Adams and Scott Schoen Fund and the Kraig and Linda Kupiec Family Trust to RH.
The University of California has been issued a patent for a device described in this review, listing RH as one of the inventors.
The remaining author declares that the research was conducted in the absence of any commercial or financial relationships that could be construed as a potential conflict of interest.
All claims expressed in this article are solely those of the authors and do not necessarily represent those of their affiliated organizations, or those of the publisher, the editors and the reviewers. Any product that may be evaluated in this article, or claim that may be made by its manufacturer, is not guaranteed or endorsed by the publisher.
1. Weese-Mayer DE, Berry-Kravis EM, Ceccherini I, Keens TG, Loghmanee DA, Trang H. An official ATS clinical policy statement: congenital central hypoventilation syndrome: genetic basis, diagnosis, and management. Am J Respir Crit Care Med. (2010) 181:626–44. doi: 10.1164/rccm.200807-1069ST
2. Chen ML, Witmans MB, Tablizo MA, Jubran RF, Turkel S. B, Tavaré CJ, et al. Disordered respiratory control in children with partial cerebellar resections. Pediatr Pulmonol. (2005) 40:88–91. doi: 10.1002/ppul.20225
3. Paton JY, Swaminathan S, Sargent CW, Keens TG. Hypoxic and hypercapnic ventilatory responses in awake children with congenital central hypoventilation syndrome. Am Rev Resp Dis. (1989) 140:368–72. doi: 10.1164/ajrccm/140.2.368
4. Couzin-Frankel J. The mystery of the pandemic's ‘happy hypoxia’ Science. (2020) 368:455–6. doi: 10.1126/science.368.6490.455
5. Tobin MJ, Laghi F, Jubran A. Why COVID-19 silent hypoxemia is baffling to physicians. Am J Respir Crit Care Med. (2020) 202:356–60. doi: 10.1164/rccm.202006-2157CP
6. Chokroverty S, Sachdeo R, Masdeu J. Autonomic dysfunction and sleep apnea in olivopontocerebellar degeneration. Arch Neurol. (1984) 41:926–31. doi: 10.1001/archneur.1984.04050200032014
7. Martelli D, Stanić D, Dutschmann M. The emerging role of the parabrachial complex in the generation of wakefulness drive and its implication for respiratory control. Respir Physiol Neurobiol. (2013) 188:318–23. doi: 10.1016/j.resp.2013.06.019
8. Nattie EE, Li A. CO2 dialysis in nucleus tractus solitarius region of rat increases ventilation in sleep and wakefulness. J Appl Physiol (1985). (2002) 92:2119–30. doi: 10.1152/japplphysiol.01128.2001
9. Orem J. The nature of the wakefulness stimulus for breathing. Prog Clin Biol Res. (1990) 345:23–30.
10. Ni H, Schechtman VL, Zhang J, Glotzbach SF, Harper RM. Respiratory responses to preoptic/anterior hypothalamic warming during sleep in kittens. Reprod Fertil Dev. (1996) 8:79–86. doi: 10.1071/rd9960079
12. Darnall RA, Ariagno RL. The effect of sleep state on active thermoreulation in the premature infant. Pediatric Res. (1982) 16:512–4. doi: 10.1203/00006450-198207000-00002
13. Pierson CR, Sufiani FA. Preterm birth and cerebellar neuropathology. Semin Fetal Neonatal Med. (2016) 21:305–11. doi: 10.1016/j.siny.2016.04.006
14. Welsh JP, Yuen G, Placantonakis DG, Vu TQ, Haiss F, O'Hearn E, et al. Why do Purkinje cells die so easily after global brain ischemia? Aldolase C, EAAT4, and the cerebellar contribution to posthypoxic myoclonus. Adv Neurol. (2002) 89:331–59.
15. Xu F, Frazier D. Role of the cerebellar deep nuclei in respiratory modulation. Cerebellum. (2002) 1:35–40. doi: 10.1080/147342202753203078
16. Pae EK, Chien P, Harper RM. Intermittent hypoxia damages cerebellar cortex and deep nuclei. Neurosci Lett. (2005) 375:123–8. doi: 10.1016/j.neulet.2004.10.091
17. Darnall RA, Chen X, Nemani KV, Sirieix CM, Gimi B, Knoblach S, et al. Early postnatal exposure to intermittent hypoxia in rodents is proinflammatory, impairs white matter integrity, and alters brain metabolism. Pediatr Res. (2017) 82:164–72. doi: 10.1038/pr.2017.102
18. Forster HV, Haouzi P, Dempsey JA. Control of breathing during exercise. Compr Physiol. (2012) 2:743–77. doi: 10.1002/cphy.c100045
19. Dejours P, LaBrousse Y, Teillac A. Etude du stimulus ventilatoire proprioceptif mis en jeu par l'activité motrice chez l'homme [Proprioceptive ventilatory stimulus initiated by motor activity in man]. C R Hebd Seances Acad Sci. (1959) 248:2129–31.
20. Ishida K, Yasuda Y, Miyamura M. Cardiorespiratory response at the onset of passive leg movements during sleep in humans. Europ J Appl Physiol. (1993) 66:507–13. doi: 10.1007/BF00634300
21. Patwari PP, Carroll MS, Rand CM, Kumar R, Harper R, Weese-Mayer DE. Congenital central hypoventilation syndrome and the PHOX2B gene: A model of respiratory and autonomic dysregulation. Respir Physiol Neurobiol. (2010) 173:322–35. doi: 10.1016/j.resp.2010.06.013
22. Paton JY, Swaminathan S, Sargent CW, Hawksworth A, Keens TG. Ventilatory response to exercise in children with congenital central hypoventilation syndrome. Am Rev Respir Dis. (1993) 147:1185–91. doi: 10.1164/ajrccm/147.5.1185
23. Gozal D, Simakajornboon N. Passive motion of the extremities modifies alveolar ventilation during sleep in patients with CCHS. Am J Resp Crit Care Med. (2000) 162:1747–51. doi: 10.1164/ajrccm.162.5.2005012
24. Woo MS, Valladares E, Montes L, Sarino M, Harper RM. Proprioceptor stimulation improves sleep disordered breathing in adolescents and young adults with Asia A paraplegia. Am J Respir Crit Care Med. (2014) 189:A1280.
25. Iwamoto E, Taito S, Kawae T, Sekikawa K, Takahashi M, Inamizu T. The neural influence on the occurrence of locomotor-respiratory coordination. Respir Physiol Neurobiol. (2010) 173:23–8. doi: 10.1016/j.resp.2010.06.002
26. Hopkins DA, Holstege G. Amygdaloid projections to the mesencephaion, pons and medulla oblongata in the cat. Exp Brain Res. (1978) 32:529–47. doi: 10.1007/BF00239551
27. Mitchell JH. Neural circulatory control during exercise: early insights. Exp Physiol. (2013) 98:867–78. doi: 10.1113/expphysiol.2012.071001
28. Potts JT, Rybak IA, Paton JFR. Respiratory rhythm entrainment by somatic afferent stimulation. J Neurosci. (2005) 25:1965–78. doi: 10.1523/JNEUROSCI.3881-04.2005
29. Shevtsova NA, Marchenko V, Bezdudnaya T. Modulation of respiratory system by limb muscle afferents in intact and injured spinal cord. Front Neurosci. (2019) 13:289. doi: 10.3389/fnins.2019.00289
30. Sauerland EK, Harper RM. The human tongue during sleep: electromyographic activity of the genioglossus muscle. Exp Neurol. (1976) 51:160–70. doi: 10.1016/0014-4886(76)90061-3
31. Frantz ID 3rd, Adler SM, Thach BT, Taeusch HW Jr. Maturational effects on respiratory responses to carbon dioxide in premature infants. J Appl Physiol. (1976) 41:41–5. doi: 10.1152/jappl.1976.41.1.41
32. Guthrie RD, Standaert TA, Hodson WA, Woodrum DE. Sleep and maturation of eucapnic ventilation and CO2 sensitivity in the premature primate. J Appl Physiol. (1980) 48:347–54. doi: 10.1152/jappl.1980.48.2.347
33. Darnall RA. The role of CO2 and central chemoreception in the control of breathing in the fetus and the neonate. Respir Physiol Neurobiol. (2010) 173:201–12. doi: 10.1016/j.resp.2010.04.009
34. Yan B, Li L, Harden SW, Gozal D, Lin Y, Wead WB, et al. Chronic intermittent hypoxia impairs heart rate responses to AMPA and NMDA and induces loss of glutamate receptor neurons in nucleus ambiguous of F344 rats. Am J Physiol Regul Integr Comp Physiol. (2009) 296:R299–R308. doi: 10.1152/ajpregu.90412.2008
35. Kim G, Elnabawi O, Shin D, Pae E-K. Transient intermittent hypoxia exposure disrupts neonatal bone strength. Front Pediatr. (2016) 4:15. doi: 10.3389/fped.2016.00015
36. Pae E-K, Ahuja B, Kim M, Kim G. Impaired glucose homeostasis after a transient intermittent hypoxic exposure in neonatal rats. Biochem Biophys Res Commun. (2013) 441:637–42. doi: 10.1016/j.bbrc.2013.10.102
37. Martin RJ, Wang K, Köroglu O, Di Fiore J, Kc P. Intermittent hypoxic episodes in preterm infants: do they matter? Neonatology. (2011) 100:303–10. doi: 10.1159/000329922
38. Martin RJ, Di Fiore JM, Walsh MC. Hypoxic episodes in bronchopulmonary dysplasia. Clin Perinatol. (2015) 42:825–38. doi: 10.1016/j.clp.2015.08.009
39. Cohen G, Lagercrantz H, Katz-Salamon M. Abnormal circulatory stress responses of preterm graduates. Pediatr Res. (2007) 61:329–34. doi: 10.1203/pdr.0b013e318030d0ef
40. Hibbs AM, Johnson NL, Rosen CL, Kirchner HL, Martin R, Storfer-Isser A, et al. Prenatal and neonatal risk factors for sleep disordered breathing in school-aged children born preterm. J Pediatr. (2008) 153:176–82. doi: 10.1016/j.jpeds.2008.01.040
41. Janvier A, Khairy M, Kokkotis A, Cormier C, Messmer D, Barrington KJ. Apnea is associated with neurodevelopmental impairment in very low birth weight infants. J Perinatol. (2004) 24:763–8. doi: 10.1038/sj.jp.7211182
42. Schmölzer GM, Te Pas AB, Davis PG, Morley CJ. Reducing lung injury during neonatal resuscitation of preterm infants. J Pediatr. (2008) 153:741–5. doi: 10.1016/j.jpeds.2008.08.016
43. Garland JS, Nelson DB, Rice T, Neu J. Increased risk of gastrointestinal perforations in neonates mechanically ventilated with either face mask or nasal prongs. Pediatrics. (1985) 76:406–10.
44. Buckle P, Millar T, Kryger M. The effect of short-term nasal CPAP on Cheyne-Stokes respiration in congestive heart failure. Chest. (1992) 102:31–5. doi: 10.1378/chest.102.1.31
45. Davies RJ, Harrington KJ, Omerod OJ, Stradling JR. Nasal continuous positive airway pressure in chronic heart failure with sleep-disordered breathing. Am Rev Respir Dis. (1993) 147:630–4. doi: 10.1164/ajrccm/147.3.630
46. Javaheri S. CPAP should not be used for central sleep apnea in congestive heart failure patients. J Clin Sleep Med. (2006) 2:399–402. doi: 10.5664/jcsm.26653
47. Dobson NR, Rhein LM, Darnall RA, Corwin MJ, Heeren TC, Eichenwald E, et al. Caffeine Study Group. Caffeine decreases intermittent hypoxia in preterm infants nearing term-equivalent age. J Perinatol. (2017) 37:1135–40. doi: 10.1038/jp.2017.82
48. Marcus CL, Meltzer LJ, Roberts RS, Traylor J, Dix J, D'ilario J, et al. Caffeine for apnea of prematurity-sleep study. Long-term effects of caffeine therapy for apnea of prematurity on sleep at school age. Am J Respir Crit Care Med. (2014) 190:791–9. doi: 10.1164/rccm.201410-1887LE
49. Hayes MJ, Akilesh MR, Fukumizu M, Gilles AA, Sallinen BA, Troese M, et al. Apneic preterms and methylxanthines: arousal deficits, sleep fragmentation and suppressed spontaneous movements. J Perinatol. (2007) 27:782–9. doi: 10.1038/sj.jp.7211820
50. Olini N, Kurth S, Huber R. The effects of caffeine on sleep and maturational markers in the rat. PLoS One. (2013) 8:e72539. doi: 10.1371/journal.pone.0072539
51. Harper RM, Kumar R, Macey PM, Woo MA. (2005) Neural responses to paced breathing in congenital central hypoventilation syndrome. In: Society for Neuroscience Annual Meeting (Washington, DC).
52. Harper RM. The cerebellum and respiratory control. Cerebellum. (2001) 1:1–2. doi: 10.1080/147342202753203032
53. Paydarfar D, Barbieri R, Indic PP, Kandah R, Niemi J, Osborne JP, et al. WO2013033433A2 - Systems and Methods for Inhibiting Apneic Events. World Intellectual Property Organization International Bureau (2013).
54. Kattwinkel J, Nearman HS, Fanaroff AA, Katona PG, Klaus MH. Comparative therapeutic effects of cutaneous stimulation and nasal continuous positive arirway pressure. J Pediatr. (1975) 86:588–92.
55. Bloch-Salisbury E, Indic P, Bednarek F, Paydarfar D. Stabilizing immature breathing patterns of preterm infants using stochastic mechanosensory stimulation. J Appl Physiol. (2009) 107:1017–27. doi: 10.1152/japplphysiol.00058.2009
56. Korner AF, Kraemer HC, Haffner E, Cosper LM. Effects of waterbed flotation on premature infants: a pilot study. Pediatrics. (1975) 56:361–7.
57. Sammon MP, Darnall RA. Entrainment of respiration to rocking in premature infants: coherence analysis. J Appl Physiol. (1994). 77:1548–54. doi: 10.1152/jappl.1994.77.3.1548
58. Harper RM, Macey PM, Woo MA, Macey KE, Keens TG, Gozal D, et al. Hypercapnic exposure in Congenital Central Hypoventilation Syndrome reveals central nervous system respiratory control mechanisms. J Neurophys. (2005) 93:1647–58. doi: 10.1152/jn.00863.2004
59. Gozal D, Marcus CL, Davidson Ward SL, Keens TG. Ventilatory responses to passive leg motion in children with congenital central hypoventilation syndrome. Am J Respir Crit Care Med. (1996) 153:761–8. doi: 10.1164/ajrccm.153.2.8564130
60. Wang A, Kun S, Diep B, Davidson-Ward SL, Keens TG, Perez IA. Obstructive sleep apnea in congenital central hypoventilation syndrome patients ventilated by diaphragm pacing without tracheostomy. J Clinic Sleep Med. (2018) 14:261–4. doi: 10.5664/jcsm.6948
61. Kumar R, Macey PM, Woo MA, Alger JR, Keens TG, Harper RM. Neuroanatomic deficits in congenital central hypoventilation syndrome. J Comp Neurol. (2005) 487:361–71. doi: 10.1002/cne.20565
62. Kumar R, Macey PM, Woo MA, Alger JR, Harper RM. Diffusion tensor imaging demonstrates brainstem and cerebellar abnormalities in congenital central hypoventilation syndrome. Pediatr Res. (2008) 64:275–80. doi: 10.1203/PDR.0b013e31817da10a
63. Kesavan K, Frank P, Cordero D, Benharash P, Harper RM. Neuromodulation of limb proprioceptive afferents decreases apnea of prematurity and accompanying intermittent hypoxia and bradycardia. PLoS One. (2016) 11:e0157349. doi: 10.1371/journal.pone.0157349
64. Harper RM, Harper RK, Snodgrass D, Feulner LC, Yan-Go F, Snodgrass D, et al. Non-invasive neuromodulation to maintain blood pressure and respiration in sleep-disturbed breathing. In: International Society for Autonomic Neuroscience, 11th Congress (Los Angeles) (2019).
65. Harper RM, Hertling D, Curtis A, Sauerland EK, De Giorgio CM. Pilot safety and feasibility study of non-invasive limb proprioceptive cerebellar stimulation for epilepsy. Front Neurol. (2021) 12:675947. doi: 10.3389/fneur.2021.675947
66. Harper RM, Woo MA, Shayboyan S, Macey PM. Device, System and Method for Facilitating Breathing via Simulation of Limb Movement. U.S. Patent No. 10,610,447, USPTO, Washington, DC (2015).
67. Lei Q, Lv Y, Li K, Ma L, Du G, Xiang Y, et al. Effects of continuous positive airway pressure on blood pressure in patients with resistant hypertension and obstructive sleep apnea: a systematic review and meta-analysis of six randomized controlled trials. J Bras Pneumol. (2017) 43:373–9. doi: 10.1590/s1806-37562016000000190
Keywords: neuromodulation, periodic breathing, apnea, cardiovascular, proprioception, prematurity
Citation: Harper RM and Kesavan K (2021) Neuromodulatory Support for Breathing and Cardiovascular Action During Development. Front. Pediatr. 9:753215. doi: 10.3389/fped.2021.753215
Received: 04 August 2021; Accepted: 03 September 2021;
Published: 30 September 2021.
Edited by:
Anna Maria Lavezzi, University of Milan, ItalyReviewed by:
Ana Paula Abdala, University of Bristol, United KingdomCopyright © 2021 Harper and Kesavan. This is an open-access article distributed under the terms of the Creative Commons Attribution License (CC BY). The use, distribution or reproduction in other forums is permitted, provided the original author(s) and the copyright owner(s) are credited and that the original publication in this journal is cited, in accordance with accepted academic practice. No use, distribution or reproduction is permitted which does not comply with these terms.
*Correspondence: Ronald M. Harper, cmhhcnBlckB1Y2xhLmVkdQ==
Disclaimer: All claims expressed in this article are solely those of the authors and do not necessarily represent those of their affiliated organizations, or those of the publisher, the editors and the reviewers. Any product that may be evaluated in this article or claim that may be made by its manufacturer is not guaranteed or endorsed by the publisher.
Research integrity at Frontiers
Learn more about the work of our research integrity team to safeguard the quality of each article we publish.