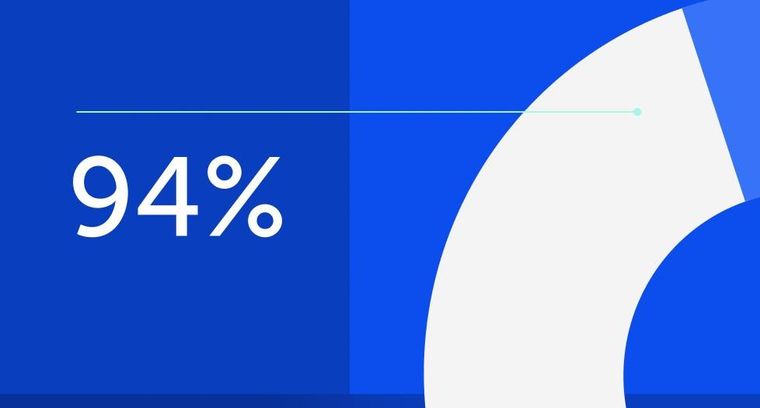
94% of researchers rate our articles as excellent or good
Learn more about the work of our research integrity team to safeguard the quality of each article we publish.
Find out more
REVIEW article
Front. Ophthalmol., 24 October 2023
Sec. Glaucoma
Volume 3 - 2023 | https://doi.org/10.3389/fopht.2023.1274797
This article is part of the Research TopicOcular Fibrosis: Molecular and Cellular Mechanisms and Treatment ModalitiesView all 5 articles
Primary open angle glaucoma (POAG) is a progressive and chronic disease exhibiting many of the features of fibrosis. The extracellular matrix (ECM) in the trabecular meshwork (TM) undergoes extensive remodeling and enhanced rigidity, resembling fibrotic changes. In addition, there are changes associated with myofibroblast activation and cell contractility that further drives tissue fibrosis and stiffening. This review discusses what is known about the integrins in the TM and their involvement in fibrotic processes.
The extracellular matrix (ECM) is a dynamic network composed of structural and nonstructural proteins that are assembled into a tissue-specific architectural 3D scaffold that provides not only structural support for tissues but directs cell motility, survival, proliferation, and even cell death. In the trabecular meshwork (TM) in the anterior segment of the human eye, the ECM is primarily composed of different collagens (types I, III, IV, V, and VI), the glycoproteins fibronectin and laminin, and the proteoglycan hyaluronan. It also contains elastic fibers composed of fibrillin and elastin as well as multiple matricellular proteins whose expression may be transient. These ECM proteins can be found distributed throughout the various layers of the TM (1) (Figure 1). The uveal and corneoscleral meshworks form the trabecular lamellae and consist of collagen beams surrounded by a monolayer of endothelial-like TM cells on top of a basement membrane. The juxtacanalicular tissue (JCT) consists of cells exhibiting both fibroblastic and smooth muscle-like qualities (2, 3) loosely embedded in an ECM composed of different collagens, elastin fibers, fibronectin, hyaluronan, and various proteoglycans. Directly adjacent to the JCT is a monolayer of endothelial-like cells on top of a basement membrane that forms the inner wall of Schlemm’s Canal (SC). These last two layers of the TM are considered to be the major sites involved in regulating the outflow of aqueous humor and intraocular pressure (IOP) (4) and are also the regions where profibrotic changes are thought to lead to the pathogenesis of primary open angle glaucoma (POAG) (5).
Figure 1 The trabecular meshwork/Schlemm’s canal (TM/SC) outflow pathway. (A) Aqueous humor (AH, green arrows) exits the anterior chamber through the uveoscleral meshwork (UM), corneoscleral meshwork (CM) and the juxtacanalicular tissue (JCT). It then crosses the basement membrane (BM) underlying the inner wall (IW) of Schlemm’s Canal to exit either paracellularly or transcellularly into the lumen of SC. The light and dark blue cells in the JCT indicate that the JCT consists of cells showing both fibroblastic and smooth muscle-like properties, respectively. The beams are connected to each other by cytoplasmic extensions between the TM cells surrounding the beams. The insert in the circle shows the profibrotic changes associated with POAG that may include the transition of TM cells in the JCT into myofibroblasts, the expression of the EDA+ isoform of fibronectin (FN), and the increased production of proteins in the BM of the IW wall. (B) Diagram of the whole eye showing the normal movement of aqueous humor (dashed arrow) from the ciliary body past the lens and iris into the anterior chamber and out through the TM/SC. (C) Diagram of the whole eye showing that profibrotic changes in the TM/SC shown in (A) would lead to a restriction in the movement of aqueous humor through the TM/SC (smaller arrow head) and an accumulation of more aqueous humor in the anterior chamber (larger arrowhead). This would result in increased pressure throughout the eye including in the vitreous chamber.
Remodeling of the ECM in the TM is considered to be important in maintaining normal homeostasis of aqueous humor outflow through the TM (6, 7) and IOP. However, excessive and prolonged remodeling of the ECM in the JCT and inner wall of Schlemm’s canal (Figure 1) leads to a restriction in the outflow of aqueous humor that results in a buildup of fluid in the anterior chamber and an elevation in IOP. This is believed to trigger a pro-fibrotic like state resembling fibrosis that leads to the pathogenesis of POAG (8, 9). Transition into this pro-fibrotic state can start with either an age-related remodeling of the ECM that causes a general stiffening of the TM, thickening of the beams, loss of beam cells (5) or elevated levels of TGFβ2 in aqueous humor (10, 11). These changes which alter the mechanical properties of the TM (5, 12) activate signaling cascades that could cause the transdifferentiation of quiescent TM cells into myofibroblast-like cells. This process, termed endothelial-to-mesenchymal transition (EndoMT), can be driven by a variety of autocrine and paracrine signaling molecules including TGFβ, Wnt/β-catenin, Notch and/or inflammatory cytokines (13). EndoMT is also driven by the expression and/or structural stiffness of an isoform of fibronectin called EDA+ in the ECM (14). Myofibroblasts display a greater capacity to produce ECM proteins and contract (15, 16). The increased contractile properties of myofibroblasts further enhances the deposition of the ECM, notedly collagen and fibronectin thereby creating a feedback loop that further increases the rigidity of the ECM and pro-fibrotic activity of the tissue. These changes in the mechanical properties of a tissue are due in part to the activity of a family of transmembrane receptors called integrins (17, 18).
Each integrin is a heterodimer composed of an α- and a β-subunit (19–21) (Figure 2). In humans, there are 18 α and 8 β subunits which mix and match to form 24 unique receptors that have tissue-specific biological properties and show specificity for different ECM ligands. For instance, α5β1 integrin only binds fibronectin whereas α4β1 integrin binds fibronectin and VCAM. Although all integrins have been shown to mediate cell attachment to the ECM, several integrins also have distinct biological functions. For example, α5β1 integrin is best known for regulating fibronectin fibrillogenesis (22, 23) and αvβ5 integrin regulates phagocytosis (24, 25). A more detailed discussion of integrin structure and function in the TM can be found in a recent review (21).
Figure 2 Integrins and their ligands found in the TM. (A) An integrin consists of an α- and β-subunit. The heterodimer functions to form a physical link between the extracellular matrix (ECM) and the cytoskeleton that acts as a signal transducer. The extracellular domain of the heterodimers bind to a variety of ECM proteins and growth factors found the TM/SC while the cytoplasmic tails of the heterodimer bind a variety of kinases (i.e. FAK, Src), adapter proteins (i.e. Talin, Hic-5) and members of the Rho family in a complex called a focal adhesion (FA). This complex of cytoplasmic proteins helps form a link between the tails of integrins and the actin cytoskeleton. It also connects integrins to signaling pathways including the Rho GTPase and TGFβ signaling pathways. (B) Integrins and their ligands found in the TM/SC. As shown, all integrins with the exception of α5β1 integrins bind multiple ligands. (C) The color-coded sequences in the box are the motifs that are recognized by each specific integrin in the various ligands. Fibronectin (FN), Thrombospondin-1 (TSP-1), tenascin-C (TN-C), Osteopontin (OPN), Vascular cell adhesion molecule (VCAM), galectin-8 (GAL-8), Vitronectin (VN), Latency-associated protein (LAP), Transforming growth factor β (TGFβ), Milk fat globule-EGF factor 8 (MFG-E8), Secreted protein acidic and rich in cysteine (SPARC), Fibrillin (FBN), Fibroblast growth factor (FGF), Connective tissue growth factor (CTGF), and Vascular endothelial growth factor receptor (VEGFR).
At least 20 different integrins have been identified in the cells associated with the TM in the outflow pathway by either RNA or protein analysis. These integrins show a broad distribution and are found along the trabecular beams, in the JCT and in SC cells along the inner wall demonstrating that multiple integrins are expressed on cells throughout the TM/SC (25–27). The major integrins found in the JCT of the TM/SC by scRNA analysis of human tissues (3) appear to be αvβ5 and α9β1, whereas SC cells contain predominantly αvβ3, αvβ1, α5β1, α9β1, and α10β1. A complete listing of all the integrins found in this study can be found at the Broad Institute of MIT and Harvard Single Cell Portal1.
Although integrins are best known for mediating cell attachment to the ECM, integrins are also key partners in a number of signaling pathways involved in fibrosis including TGFβ signaling (28), formation of a fibronectin matrix (22, 29), myofibroblast formation (30), and activation of Rho GTPases (31). Integrins participate in these pathways via either direct interaction with receptors or through an association with intracellular cytoskeleton elements assembled into a signaling complex called focal adhesions (FAs). This latter association occurs through a variety of cytoskeletal linker proteins and kinases (i.e., FAK, Src, talin, paxillin, vinculin, etc.) which form a physical linkage that directly connects intracellular and extracellular structures (Figure 2). This puts integrins in a unique situation in that they exhibit bidirectional signaling. Integrins can convert extracellular biochemical signals generated from the proteins in the ECM into intracellular biochemical signals. They can also convert extracellular mechanical forces derived from the pulsatile motions of the TM (32) into intracellular biochemical signals. Both sets of intracellular biochemical signals can drive the differentiation of cells into myofibroblasts (30) and promote excessive ECM deposition during fibrogenesis. Alternatively, integrins can transmit intracellular, myosin-generated contractile forces to the outside of the cell that alter the architecture and signaling properties of proteins in the ECM (17, 33). These intracellular signals may include, but are not limited to, tyrosine phosphorylation of proteins such as paxillin and p130CAS (34–36), activation of protein tyrosine kinases such as FAK and Src (37), and activation of serine/threonine kinases such as Erk or Akt (38, 39). Extracellular signals, on the other hand, could include changes in the conformation of fibronectin (40) needed for fibrillogenesis (41).
Among the important signaling pathways controlled by integrins that are involved in fibrosis is the Rho GTPase pathway (31, 42). Rho GTPase pathways involving RhoA, Rac, and Cdc42 are essential in regulating the contractile (43, 44) and phagocytic (25) properties of the human TM. They are also needed for the enhanced contractile properties of myofibroblasts and the deposition of proteins associated with fibrosis into the ECM (45, 46). Integrins control GTPase mediated-processes by directing the localization and activation of Rho GTPases at the membrane (47). This is done by controlling the activity of guanine nucleotide exchange factors (GEFs) and GTPase activating proteins (GAPs) that control Rho GTPase activities (48). For instance, in human TM cells, activation of αvβ3 integrin uses the GEF Tiam1 to trigger the activity of Rac1 which promotes the reorganization of actin and smooth muscle α-actin (α-SMA) into crosslinked actin networks (CLANs) (49, 50). This network is frequently observed in glaucomatous cells and tissues and is believed to alter TM contractility (51, 52) and hence the mechanical properties of the TM/SC. Activation of Rac1 by αvβ3 integrin also leads to an inhibition of Rho-mediated phagocytosis (25, 53). In this scenario, αvβ3 integrin uses the GEFs Tiam1 and RhoG/ILK/ELMO2 rather than Trio to trigger Rac1 activity. Once activated, Rac1 could inhibit RhoA activity by upregulating a 190 RhoGAP (54).
RhoA, another member of the Rho GTPase family, plays a prominent role in promoting the contractile properties of the actomyosin network in the TM that control IOP. In human TM cells, constitutively active RhoA causes a significant increase in the formation of actomyosin networks, and in rodent models of ocular hypertension it causes an increase in α-SMA expressing myofibroblast-like cells (55–59). Thus, studies have shown that inhibiting the Rho-associated protein kinase (ROCK), a downstream effector of RhoA activity, is an effective treatment for lowering IOP in POAG (58, 59).
RhoA also leads to an increase in the deposition of ECM proteins especially fibronectin in human TM cells (55). In these studies the activity of RhoA, as well as the TGFβ/SMAD pathways, was controlled by the αvβ3 integrin, together with the subsequent recruitment of Hic-5 to FAs (57). The activation of αvβ3 integrin or overexpression of Hic-5 induced the cytoskeleton changes attributed to RhoA activity while the knockdown of Hic-5 suppressed TGFβ2-induced fibrogenic activity (57). Interestingly these studies found that activation of RhoA and the TGFβ/SMAD pathways occurred in the absence of TGFβ2. This suggests that integrin-mediated signaling may play an essential role in the TGFβ2-mediated activation of RhoA during fibrosis. This is not totally unexpected since studies have shown that integrin engagement plays a critical role in growth factor signaling including TGFβ signaling (60–63) and the subsequent activation of RhoA.
Integrins play a critical role in fibrosis since they are responsible for the deposition and formation of fibronectin fibrils that direct and maintain the organization of the ECM. Fibronectin is a dimeric glycoprotein maintained by two disulfide bonds at its C-terminus that is composed of an array of repeating modular structures called repeats (Figure 3A). There are 12 type I repeats (FNI), 2 type II repeats (FNII), 15 type III repeats (FNIII) and a non-homologous variable (V) or type III connecting segment (IIICS) region. In addition, it can contain 2 additional alternatively spliced type III repeats referred to as EDA and EDB repeats. These repeating segments create functional domains that interact with multiple binding partners within the ECM that allow fibronectin to help mold and maintain the 3D-architecture of the ECM during fibrosis and incorporation of other proteins into the ECM (64–69).
Figure 3 Fibronectin (FN) matrix assembly model. (A) Fibronectin consists of three repeating numbered modules (types I, II, III) and three alternatively spliced sequences. The ECM proteins that use fibronectin to be incorporated into the ECM are indicated below their fibronectin binding sites. The major integrin binding site (RGD) is in the 10th FNIII repeat and binds the integrins indicated in the figure. Tenascin-C (TNC), Collagen/gelatin (Col/Gel), Thrombospondin (TSP), Fibrillin (FBN), Transglutaminase (TGC), Proteoglycans (PGs), Fibulin (FBLN), Transforming growth factor β (TGFβ), Fibroblast growth factor (FGF), Bone morphogenetic proteins (BMPs) and Vascular endothelial growth factor (VEGF). (B) Fibronectin, which is secreted as a globular dimer, binds an integrin in a focal adhesion (FA) on the cell surface (step 1). Contractile forces generated by the actomyosin cytoskeleton connected to the integrins (step 2) cause the fibronectin dimer to unfold. Fibronectin-fibronectin binding sites (purple ovals) mediate fibril formation (step 3). These fibronectin fibrils serve as a scaffold for the incorporation of other ECM components such as collagen fibrils (step 4).
Fibronectin fibrillogenesis plays a critical role in fibrosis and disruption of it attenuated fibrosis in multiple in vivo models of fibrosis including two different mouse models of kidney fibrosis (70, 71) and a model of liver fibrosis (72). It also prevented fibrosis during a mouse model of heart failure (73). In TM cells in culture, inhibition of fibronectin fibril formation also inhibited the incorporation of other matrix proteins such as type IV collagen, fibrillin and laminin into the ECM (74). Intriguingly, inhibition of fibronectin fibrillogenesis also appeared to promote the removal of existing fibronectin fibrils (74) and lowered IOP in vivo (75). This suggests that controlling this integrin mediated assembly of fibronectin fibrils may represent a way to control fibrosis in glaucoma.
The assembly of fibronectin into fibrils in TM cells is mediated by several fibronectin binding integrins (22, 41). The major integrin involved is the α5β1 integrin. α5β1 integrins promote fibril formation by binding the secreted soluble dimer and inducing a conformational change that exposes specific fibronectin–fibronectin binding sites (Figure 3A). These binding sites are needed for the assembly of fibronectin into an insoluble fibril that then acts as the scaffold for any subsequent matrix deposition (74). Among the sites involved in fibril formation are the amino terminus of fibronectin (76) and the heparin II binding domain (77) and blocking their binding activity has been shown to prevent fibril formation. Thus, inhibiting these interactions have proven an attractive mechanism to control fibril formation and fibrosis.
Integrins can control this process because they regulate the contractile forces of the actomyosin network that are used to unfold and stretch fibronectin so that fibronectin-fibronectin binding sites needed for fibril formation are exposed (Figure 3). In human TM cells as in other cell types, this process usually involves the GTPase RhoA (23, 40) which appears to be activated when the α5β1 integrin engages the fibronectin monomer. In TM cells activation of αvβ3 integrin, however, enhances the assembly of fibronectin into fibrils leading to an increase in the deposition of fibronectin fibrils. How activation of αvβ3 integrin enhances the α5β1 integrin-mediated process is unclear. Unlike the RhoA-mediated process involving α5β1 integrin, the αvβ3 integrin uses a RhoA/ROCK-independent process (23) since the process is unaffected by the ROCK inhibitor, Y27632. Thus, it is possible that activation of αvβ3 integrins may be activating pathways that are independent of RhoA/ROCK by either using the guanine nucleotide exchange factor GEF-H1/mDia (78) or the GTPase Rac1 (49, 79) to generate the contractile forces of the actomyosin network in the cells. Interestingly, GEF-H1 has been shown to regulate RhoA-dependent cell stiffening and rigidity (48) while the RhoA/ROCK pathway has been observed to control fibrotic activity in human TM cells in culture (56) and in vivo (57). This suggests that the two processes together may be increasing the contractile forces regulating fibril formation and targeting a specific GEF as well as ROCK could be an effective treatment for preventing any profibrotic changes during POAG (58, 59).
Intriguingly, fibronectin fibrils assembled by TM cells expressing constitutively activated αvβ3 integrin also contained higher levels of the alternatively spliced isoforms of fibronectin containing the EDA and EDB domains (23). These alternatively spliced domains are usually not expressed in adult tissue unless the ECM in the tissue is being remodeled. The inclusion of both the EDA+ and EDB+ alternatively spliced domains in fibronectin supports a more robust response to TGFβ signaling whereas fibrils containing only EDA+fibronectin promoted a weaker response to TGFβ (80). Inclusion of the EDA+ and EDB+ domains into fibronectin also affects the thickness, stiffness, and degree of branching of the fibril and the pore size of the fibronectin fibrillar network (80). The EDA domain is also involved in the transition of cells into myofibroblasts (14). These fibrils also exhibited an altered fibril conformation that resulted in the exposure of a buried domain known as the L8 epitope (Figure 3) which involves the GLN-690 in the first type III repeat (81). Hence changes in the expression or activity of integrins in TM cells are likely to profoundly affect the function and compliance of the ECM as well as modulate ECM-mediated signaling events (21, 80). This also suggests that during glucocorticoid-induced ocular hypertension or glaucoma, where αvβ3 integrins are likely to be overexpressed and active (82), the αvβ3 integrin may induce the formation of fibronectin fibrils that are more similar to a fibrotic-like ECM.
In many tissue types, fibrosis involves the formation of myofibroblasts. Myofibroblast-like cells have been observed in the TM of young human eyes (83). These cells express α-SMA, a marker of myofibroblasts and are randomly distributed throughout the TM. Interestingly, their prevalence decreases with age, but their levels increase in eyes following treatments with corticosteroids. Myofibroblasts develop pronounced α-SMA-myosin bundles (stress fibers in cultured cells) that have increased contractile properties and can connect with the ECM via integrins at sites of large focal adhesions (16). Although the formation of these α-SMA positive stress fibers is a distinguishing feature of myofibroblasts that provides the contractile properties that play an important role in the fibrotic process, α-SMA is not essential for the development of a myofibroblast phenotype (16, 84).
The transformation of a cell into a myofibroblast occurs in two stages (Figure 4). In the first stage, expression of the EDA+ isoform of fibronectin begins the transformation of cells into myofibroblasts by TGFβ1 (14). Recent studies in mice constitutively expressing EDA+ fibronectin support this idea and show that expression of EDA+ fibronectin enhances the TGFβ2-induced deposition of ECM in the TM causing an age-dependent elevation in IOP (85, 86). In fibroblasts this transformation appears to involve an interaction between the EDA domain in fibronectin and either the α4β1, α4β7 or α9β1 integrins (87, 88). Whether one of these integrins is also involved in the transformation of TM or SC cells into myofibroblasts remains to be determined.
Figure 4 Role of integrins in the transformation of a cell into a myofibroblast. (A) Multiple integrin mediated processes trigger the early stages of myofibroblast formation. These processes include: (1) binding of EDA+ fibronectin to α4β1 integrin triggers expression of α-SMA, and (2) integrin mediated mechanical transduction activates RhoA, leading to assembly of actin stress fibers and contractile forces that promote the release of TGFβ from the LAP-TGFβ complex. (3) activated TGFβ1 (freed from LAP) binds to the TGFβRI/II complex stimulating SMAD intracellular signaling that promotes further expression of α-SMA, EDA+ fibronectin, and CTGF. This signaling, which triggers a feedback loop, may occur within the focal adhesion (FA) and be mediated by the specific integrin associated with TGFβRI/II complex in the FA. (B) Activation of the integrin-mediated processes in (A), triggers the myofibroblast phenotype. The differentiated myofibroblast and its contractile properties are then sustained by the formation of supermature FAs containing αvβ3 integrin.
Other integrins such as αv-containing integrins may help promote the transformation of a cell into a myofibroblast by triggering the release of TGFβ1 from the surrounding ECM (28, 89). Finally in human TM cells, interactions between αvβ3 integrin and connective tissue growth factor (CTGF) may play a role in the transformation of the TGFβ-induced myofibroblast phenotype observed in TM cells (90–92). CTGF has been shown to be a downstream mediator of TGFβ1 induced myofibroblast differentiation in NRK cells (93) and in TGFβ2-induced myofibroblast differentiation in TM cells (90). Interactions between CTGF and αvβ3 integrin may be involved in the TGFβ2-induced myofibroblast differentiation, since cyclic RGD peptides that bind αvβ3 integrin suppressed CTGF-induced fibrosis in animals and in TM cells in culture (92). This suggests that a CTGF/αvβ3 integrin mediated signaling pathway may participate in the transformation of a cell into a myofibroblast. In summary, combinatorial signaling pathways involving multiple integrins may be responsible for the profibrotic phenotype of the TM in POAG (94).
In the second stage of myofibroblast maturation, stress fibers containing α-SMA form and develop the contractile properties that can lead to further increases in the release of TGFβ2 from the ECM and enhanced ECM rigidity due to the dependence of integrins in the activation and release of TGFβ stored within the ECM. The formation and maintenance of these stress fibers as well the contractile force generated by the activation of αvβ3, α5β1, and αvβ5 integrins (84) within FAs (30) contributes to the continued transformation of the myofibroblast.
Maturation of FAs in myofibroblasts starts with the activation of a single integrin, αvβ3 integrin (95). Interactions between αvβ3 integrin (and possibly α5β1integrin) with their ECM ligand promote the development of super mature FAs that lead to the phosphorylation of the kinases FAK and Src and the subsequent activation of mechanosensitive signaling molecules such as MAPK, RhoA, and ROCK. It is the activation of these molecules that trigger α-SMA-containing stress fiber formation. These integrin-containing FAs also contribute to the transformation of the myofibroblast by serving as hubs for signaling pathways for the TGFβ1 receptor complex (16, 96). For instance, the TGFβ1 receptor complex appears to laterally associate in the fibroblast membrane with αvβ5 integrins which in turn promotes the activation of extracellular LAP-TGF-β1 and release of TGFβ stored in the ECM (28, 89, 97).
Although TGβ1 is the most potent profibrotic cytokine known and the major driving force in the differentiation of myofibroblast phenotype (30), it does not appear to play a role in the pathogenesis of a fibrotic like state in POAG. Rather TGFβ2 appears to be predominantly involved in TGFβ induced POAG (11, 98) since numerous studies have now shown that sustained activation of ROCK by either TGFβ, CTGF or lysophosphatidic acid (LPA) in TM cells in culture or in vivo contributes significantly to creating the fibrogenic properties of the TM associated with POAG (11, 56, 57, 90, 91, 99–101). Not surprisingly, inhibition of ROCK signaling decreases ECM deposition in the TM and fibrosis but also increases aqueous humor outflow making it a therapeutic target for the treatment of POAG (46, 102).
In contrast to TGFβ1 where it is well established that the release of active TGFβ1 can be triggered by a variety of factors including αv-containing integrins and proteases (103–105), it is still unclear how TGFβ2 is activated. TGFβ2 is thought to be released by thrombospondin-1 (105) which is overexpressed in the TM from glaucomatous patients (106). Similar to the mechanisms governing the release of TGFβ1, release of TGFβ2 is also thought to involve a mechanical mechanism of TGFβ activation whereby integrin mediated contractile forces on the ECM could trigger the release of active TGFβ (103, 107), thereby possibly increasing TGFβ2 levels (Figure 4). This suggests a model whereby the combination of TGFβ, integrin-mediated contractility and changes in ECM expression could engage in a feed forward signaling loop (108) that leads to the pathogenesis of POAG. The enhanced ECM deposition by myofibroblasts leads to the production of a stiffer matrix with a higher mechanical load when compared with healthy ECM produced by fibroblasts (109). This mechanically stiffer ECM, in turn, leads to more efficient activation of TGFβ, and the increased levels of TGFβ further enhance myofibroblast differentiation leading to a positive feedback loop that sustains the profibrotic environment.
More recent studies in immortalized human TM cells in culture have suggested that the activation of the αvβ3 integrin leads to an increase in the expression of TGFβ2 mRNA and protein (110). Although, the mechanism behind this is still unclear, it appears that expression of TGFβ2 mRNA, like the β3 integrin subunit, may be a secondary glucocorticoid response modulated by calcineurin (CaN) and the transcription factor NFATc1 (82, 111). Activation of this CaN/NFATc1 pathway may be dependent on the TM cell cycle (111) since it was only activated when cells were in the proliferative state.
Finally, integrins could also play a role in TGFβ signaling by regulating the association of the two TGFβ receptors TGFβRI and TGFβRII into a complex (112) and their subsequent activation. In human lung fibroblasts, TGFBRI is enriched in FAs, while TGFBRII is selectively excluded. The oligomerization of the two receptors can be mediated by αvβ3 integrin which selectively recruits the TGFBRII receptor to interact with TGFβRI receptor in FAs (61). The αvβ3 integrin can also potentiate TGFβ signaling by controlling the activation state of TGFβRII. This is achieved through a direct interaction between αvβ3 integrin and the TGFβRII receptor that allows Src to phosphorylate and activate TGFβRII during the epithelial-mesenchymal transition of mammary epithelial cells (113). This interaction may explain the recent observation that a Src mediated TGFβ signaling pathway induced an elevation in IOP in a mouse model of ocular hypertension (114). Interestingly, recruitment of the α2β1 integrin to FAs could negatively regulate the tyrosine phosphorylation of TGFβRII through its recruitment of the phosphatase TCPTP into FAs (62). Thus, as with other growth factors, integrins have the capability of modulating TGFβ signaling (Figure 4).
In summary, integrins play crucial roles in many important biological steps in fibrosis from the deposition of the ECM to the bioavailability of TGFβ and the contractile properties of myofibroblasts. Targeting integrins and the signaling pathways that they regulate could therefore be an important long-term antifibrotic strategy in chronic fibrotic diseases to preserve the function of the TM and restore homeostasis. Potential approaches to alleviate fibrosis in the TM would be to disrupt fibronectin fibril formation. This approach has proven successful in vivo using small fibronectin peptides to prevent fibrosis in vitreoretinopathy (115), but has not been pursued in the TM. Novel studies using recombinant integrin blocking antibodies (116) may also be another approach to reduce ECM production and/or the contractile properties of the tissue.
JF: Writing – review & editing. MF: Writing – review & editing. DP: Conceptualization, Funding acquisition, Writing – original draft, Writing – review & editing.
The author(s) declare financial support was received for the research, authorship, and/or publication of this article. This work was supported by NEI grants EY017006, EY032905 (DP), and a Core grant to the Department of Ophthalmology and Visual Sciences (P30 EY016665).
The authors would like to acknowledge the assistance provided by Kassidy Johns in preparing the figures.
The authors declare that the research was conducted in the absence of any commercial or financial relationships that could be construed as a potential conflict of interest.
All claims expressed in this article are solely those of the authors and do not necessarily represent those of their affiliated organizations, or those of the publisher, the editors and the reviewers. Any product that may be evaluated in this article, or claim that may be made by its manufacturer, is not guaranteed or endorsed by the publisher.
1. Rohen JW, Lutjen-Drecoll E. Morphology of aqueous outflow pathways in normal and glaucomatous eyes. In: Ritch R, Shields MB, Krupin T, editors. The Glaucomas. St Louis: CV Mosby (1989).
2. Patel G, Fury W, Yang H, Gomez-Caraballo M, Bai Y, Yang T, et al. Molecular taxonomy of human ocular outflow tissues defined by single-cell transcriptomics. Proc Natl Acad Sci USA (2020) 117:12856–67. doi: 10.1073/pnas.2001896117
3. Van Zyl T, Yan W, McAdams A, Peng Y-R, Shekhar K, Regev A, et al. Cell atlas of aqueous humor outflow pathways in eyes of humans and four model species provides insight into glaucoma pathogenesis. Proc Natl Acad Sci USA (2020) 117:10339–49. doi: 10.1073/pnas.2001250117
4. Johnson M. What controls aqueous humour outflow resistance? Exp Eye Res (2006) 82:545–57. doi: 10.1016/j.exer.2005.10.011
5. Liu B, Mcnally S, Kilpatrick JI, Jarvis SP, O'Brien CJ. Aging and ocular tissue stiffness in glaucoma. Survey Opthalamol (2018) 63:56–74. doi: 10.1016/j.survophthal.2017.06.007
6. Keller KE, Aga M, Bradley JM, Kelley MJ, Acott TS. Extracellular matrix turnover and outflow resistance. Exp Eye Res (2009) 88:676–82. doi: 10.1016/j.exer.2008.11.023
7. Keller KE, Acott TS. The juxtacanalicular region of ocular trabecular meshwork: a tissue with a unique extracellular matrix and specialized function. J Ocul Biol (2013) 1:10. doi: 10.13188/2334-2838.1000003
8. Vranka JA, Kelley MJ, Acott TS, Keller KE. Extracellular matrix in the trabecular meshwork: intraocular pressure regulation and dysregulation in glaucoma. Exp Eye Res (2015) 133:112–25. doi: 10.1016/j.exer.2014.07.014
9. Keller KE, Peters DM. Pathogenesis of glaucoma: Extracellular matrix dysfunction in the trabecular meshwork—A review. Clin Experiment Ophthalmol (2022) 50:163–82. doi: 10.1111/ceo.14027
10. Tripathi RC, Chan WF, Li J, Tripathi BJ. Trabecular cells express the TGF-β2 gene and secrete the cytokine. Exp Eye Res (1994) 58:523–8. doi: 10.1006/exer.1994.1046
11. Fuchshofer R, Tamm ER. The role of TGF-β in the pathogenesis of primary open-angle glaucoma. Cell Tissue Res (2012) 347:279–90. doi: 10.1007/s00441-011-1274-7
12. Last JA, Pan T, Ding Y, Reilly CM, Keller KE, Acott TS, et al. Elastic modulus determination of normal and glaucomatous human trabecular meshwork. Invest Ophthamol Vis Sci (2011) 52:2147–52. doi: 10.1167/iovs.10-6342
13. Ma J, Sanchez-Duffhues G, Goumans M-J, Ten Dijke P. TGF-β-induced endothelial to mesenchymal transition in disease and tissue engineering. Front Cell Dev Biol (2020) 8:260. doi: 10.3389/fcell.2020.00260
14. Serini G, Bochaton-Piallat ML, Ropraz P, Geinoz A, Borsi L, Zardi L, et al. The fibronectin domain ED-A is crucial for myofibroblastic phenotype induction by transforming growth factor-β1. J Cell Biol (1998) 142:873–81. doi: 10.1083/jcb.142.3.873
15. Hinz B, Phan SH, Thannickal VJ, Prunotto M, Desmouliere A, Varga J, et al. Recent developments in myofibroblast biology: paradigms for connective tissue remodeling. Am J Path (2012) 180:1340–55. doi: 10.1016/j.ajpath.2012.02.004
16. Hinz B, McCulloch CA, Coelho NM. Mechanical regulation of myofibroblast phenoconversion and collagen contraction. Exp Cell Res (2019) 379:119–28. doi: 10.1016/j.yexcr.2019.03.027
17. Humphrey DJ, Dufresne ER, Schwartz MA. Mechanotransduction and extracellular matrix homeostasis. Nat Rev Mol Cell Biol (2014) 15:802–12. doi: 10.1038/nrm3896
18. Sun X, Fu Y, Gu M, Zhu Y. Activation of integrin α5 mediated by flow requires its translocation to membrane lipid rafts in vascular endothelial cells. Proc Natl Acad Sci USA (2016) 113:769–74. doi: 10.1073/pnas.1524523113
19. Luo B-H, Carman CV, Springer TA. Structural basis of integrin regulation and signaling. Ann Rev Immunol (2007) 25:619–47. doi: 10.1146/annurev.immunol.25.022106.141618
20. Gagen D, Faralli JA, Filla MS, Peters DM. The role of integrins in the trabecular meshwork. J Ocul Pharmacol Ther (2014) 30:110–20. doi: 10.1089/jop.2013.0176
21. Faralli JA, Filla MS, Peters DM. Integrin crosstalk and its effect on the biological functions of the trabecular meshwork/Schlemm's canal. Front Cell Dev Biol (2022) 10:886702. doi: 10.3389/fcell.2022.886702
22. Leiss M, Beckmann K, Giros A, Costell M, Fassler R. The role of integrin binding sites in fibronectin matrix assembly in vivo. Curr Opin Cell Biol (2008) 20:502–7. doi: 10.1016/j.ceb.2008.06.001
23. Filla M, Faralli JA, Desikan H, Peotter JL, Wannow AC, Peters DM. Activation of αvβ3 integrin alters fibronectin fibril formation in human trabecular meshwork cells in a ROCK-independent manner. Invest Ophthalmol Vis Sci (2019) 60:3897–913. doi: 10.1167/iovs.19-27171
24. Finnemann SC. Role of αvβ5 integrin in regulating phagocytosis by the retinal pigment epithelium. Adv Exp Med Biol (2003) 533:337–42. doi: 10.1007/978-1-4615-0067-4_42
25. Gagen D, Filla MS, Clark R, Liton P, Peters DM. Activated αvβ3 integrin regulates αvβ5 integrin-mediated phagocytosis in trabecular meshwork cells. Invest Ophthamol Vis Sci (2013) 54:5000-11. doi: 10.1167/iovs.13-12084
26. Zhou L, Zhang SR, Yue BY. Adhesion of human trabecular meshwork cells to extracellular matrix proteins. Roles and distribution of integrin receptors. Invest Ophthamol Vis Sci (1996) 37:104–13.
27. Zhou L, Maruyama I, Yuhong L, Cheng EL, Yue BYJT. Expression of integrin receptors in the human trabecular meshwork. Curr Eye Res (1999) 19:395–402. doi: 10.1076/ceyr.19.5.395.5297
28. Henderson NC, S D. Integrin-mediated regulation of TGFβ in fibrosis. Biochim Biophys Acta (2013) 1832:891–6. doi: 10.1016/j.bbadis.2012.10.005
29. Mao Y, Schwarzbauer J. Fibronectin fibrillogenesis, a cell-mediated matrix assembly process. Matrix Biol (2005) 24:389–99. doi: 10.1016/j.matbio.2005.06.008
30. Hinz B. Masters and servants of the force: the role of matrix adhesions in myofibroblast force perception and transmission. Euro J Cell Biol (2006) 85:175–81. doi: 10.1016/j.ejcb.2005.09.004
31. Ridley A. Rho GTPases: integrating integrin signaling. J Cell Biol (2000) 150:F107–9. doi: 10.1083/jcb.150.4.F107
32. Du R, Xin C, Xu J, Hu J, Wang H, Wang N, et al. Pulsatile trabecular meshwork motion: an indicator of intraocular pressure control in primary open-angle glaucoma. J Clin Med (2022) 11:2696. doi: 10.3390/jcm11102696
33. Sun Z, Guo SS, Fassler R. Integrin-mediated mechanotransduction. J Cell Biol (2016) 215:445–56. doi: 10.1083/jcb.201609037
34. Burridge K, Turner CE, Romer LH. Tyrosine phosphorylation of paxillin and pp125FAK accompanies cell adhesion to extracellular matrix: a role in cytoskeletal assembly. J Cell Biol (1992) 119:893–903. doi: 10.1083/jcb.119.4.893
35. Kaplan KB, Bibbins KB, Swedlow JR, Arnaud M, Morgan DO, Varmus HE, et al. Association of the amino-terminal half of c-Src with focal adhesions alters their properties and is regulated by phosphorylation of tyrosine 527. EMBO J (1994) 13:4745–56. doi: 10.1002/j.1460-2075.1994.tb06800.x
36. Petch LA, Bockholt SM, Bouton A, Parsons JT, Burridge K. Adhesion-induced tyrosine phosphorylation of the p130 src substrate. J Cell Sci (1995) 108:1371–9. doi: 10.1242/jcs.108.4.1371
37. Schaller MD, Parsons JP. Focal adhesion kinase and associated proteins. Curr Opin Cell Biol (1994) 6:705–10. doi: 10.1016/0955-0674(94)90097-3
38. Chen HC, Guan JL. Association of focal adhesion kinase with its potential substrate phosphatidylinositol 3- kinase. Proc Natl Acad Sci USA (1994) 91:10148–52. doi: 10.1073/pnas.91.21.10148
39. Schlaepfer MD, Hanks SK, Hunter T, Van Der Geer P. Integrin-mediated signal transduction linked to Ras pathway by GRB2 binding to focal adhesion kinase. Nat (1994) 372:786–91. doi: 10.1038/372786a0
40. Zhong C, Chrzanowska Wodnicka M, Brown J, Shaub A, Belkin AM, Burridge K. Rho-mediated contractility exposes a cryptic site in fibronectin and induces fibronectin matrix assembly. J Cell Biol (1998) 141:539–51. doi: 10.1083/jcb.141.2.539
41. Singh P, Carraher C, Schwarzbauer JE. Assembly of fibronectin extracellular matrix. Annu Rev Cell Dev Biol (2010) 26:397–419. doi: 10.1146/annurev-cellbio-100109-104020
42. Schwartz MA, Shattil SJ. Signaling networks linking integrins and Rho family GTPases. TIBS (2000) 25:388–91. doi: 10.1016/S0968-0004(00)01605-4
43. Epstein DL, Rowlette L, Roberts BC. Acto-myosin drug effects and aqueous outflow function. Invest Ophthamol Vis Sci (1999) 40:74–81.
44. Tian B, Geiger B, Epstein DL, Kaufman PL. Cytoskeletal involvement in the regulation of aqueous humor outflow. Invest Ophthamol Vis Sci (2000) 41:619–23.
45. Lawson CD, Burridge K. The on-off relationship of Rho and Rac during integrin-mediated adhesion and cell migration. Small GTPases. (2014) 5:e27958. doi: 10.4161/sgtp.27958
46. Knipe RS, Tagr AM, Liao JK. The rho kinases: critical mediators of multiple profibrotic processes and rational targets for new therapies for pulmonary fibrosis. Pharmacol Rev (2015) 67:103–17. doi: 10.1124/pr.114.009381
47. Del Pozo MA, Alderson NB, Kiosses WB, Chiang HH, Anderson RG, Schwartz MA. Integrins regulate Rac targeting by internalization of membrane domains. Sci (2004) 303:839–42. doi: 10.1126/science.1092571
48. Guilluy C, Swaminathan V, Garcia-Mata R, O'Brien ET, Superfine R, Burridge K. The Rho GEFs LARG and GEF-H1 regulate the mechanical response to force on integrins. Nat Cell Biol (2011) 13:722–7. doi: 10.1038/ncb2254
49. Filla M, Woods A, Kaufman PL, Peters DM. β1 and β3 integrins cooperate to induce syndecan-4-containing cross-linked actin networks in human trabecular meshwork cells. Invest Ophthalmol Vis Sci (2006) 47:1956–67. doi: 10.1167/iovs.05-0626
50. Filla M, Schwinn MK, Sheibani N, Kaufman PL, Peters DM. Regulation of cross-linked actin network (CLAN) formation in human trabecular meshwork (HTM) cells by convergence of distinct β1 and β3 integrin pathways. Invest Ophthamol Vis Sci (2009) 50:5723–31. doi: 10.1167/iovs.08-3215
51. Clark AF, Wilson K, De Kater AW, Allingham RR, McCartney MD. Dexamethasone-induced ocular hypertension in perfusion-cultured human eyes. Invest. Ophthalmol Vis Sci (1995) 36:478–89.
52. Clark AF, Brotchie D, Read AT, Hellberg P, English-Wright S, Pang IH, et al. Dexamethasone alters F-actin architecture and promotes cross-linked actin network formation in human trabecular meshwork tissue. Cell Motil Cytoskeleton (2005) 60:83–95. doi: 10.1002/cm.20049
53. Peotter JL, Phillips J, Tong T, Dimeo K, Gonzalez JMJr, Peters DM. Involvement of Tiam1, RhoG and ELMO2/ILK in Rac1-mediated phagocytosis in human trabecular meshwork cells. Exp Eye Res (2016) 347:301–11. doi: 10.1016/j.yexcr.2016.08.009
54. Bustos RI, Forget M-A, Settleman JE, Hansen SH. Coordination of Rho and Rac GTPase function via p190B RhoGAP. Curr Biol (2008) 18:1606–11. doi: 10.1016/j.cub.2008.09.019
55. Pattabiraman PP, Rao PV. Mechanistic basis of Rho GTPase-induced extracellular matrix synthesis in trabecular meshwork cells. Am J Physiol Cell Physiol (2010) 298:C749–63. doi: 10.1152/ajpcell.00317.2009
56. Pattabiraman PP, Maddala R, Rao PV. Regulation of plasticity and fibrogenic activity of trabecular meshwork cells by Rho GTPase signaling. J Cell Physiol (2014) 229:927–42. doi: 10.1002/jcp.24524
57. Pattabiraman P, Rao P. Hic-5 regulates actin cytoskeletal reorganization and expression of fibrogenic markers and myocilin in trabecular meshwork cells. Invest Ophthamol Vis Sci (2015) 56:5656–69. doi: 10.1167/iovs.15-17204
58. Rao PV, Pattabiraman PP, Kopczynski CC. Role of the Rho GTPase/Rho kinase signaling pathway in pathogenesis and treatment of glaucoma: Bench to bedside research. Exp Eye Res (2017) 158:23–32. doi: 10.1016/j.exer.2016.08.023
59. Rao VP, Epstein DL. Rho GTPase/Rho kinase inhibition as a novel target for the treatment of glaucoma. Biodrugs (2007) 21:167–71. doi: 10.2165/00063030-200721030-00004
60. Yamada KM, Even-Ram S. Integrin regulation of growth factor receptors. Nat Cell Biol (2002) 4:E75–6. doi: 10.1038/ncb0402-e75
61. Schaffidi AK, Petrovic N, Moodley YP, Fogel-Petrovic M, Kroeger KM, Seeber RM, et al. αvβ3 integrin interacts with the transforming growth factor β (TGFβ) type II receptor to potentiate the proliferative effects of TGFβ1 in living human lung fibroblasts. J Biol Chem (2004) 279:37726–33. doi: 10.1074/jbc.M403010200
62. Chen X, Wang H, Liao H-J, Hu W, Gewin L, Mernaugh G, et al. Integrin-mediated type II TGF-β receptor tyrosine dephosphorylation controls SMAD-dependent profibrotic signaling. J Clin Invest (2014) 124:3295–310. doi: 10.1172/JCI71668
63. Sarker FA, Prior VG, Bax S, O'Neill GM. Forcing a growth factor response – tissue-stiffness modulation of integrin signaling and crosstalk with growth factor receptors. J Cell Sci (2020) 133:jcs242461. doi: 10.1242/jcs.242461
64. Sottile J, Hocking DC. Fibronectin polymerization regulates the composition and stability of extracellular matrix fibrils and cell-matrix adhesions. Mol Biol Cell (2002) 13:3546–59. doi: 10.1091/mbc.e02-01-0048
65. Velling T, Risteli J, Wennerberg K, Mosher DF, Johansson S. Polymerization of type I and III collagens Is dependent on fibronectin and enhanced by integrins α11β1and α2β1. Glycobiology Extracellular Matrices (2002) 277:37377–81. doi: 10.1074/jbc.M206286200
66. Li S, Van Den Diepstraten C, D'Souza SJ, Chan BMC, Pickering JG. Vascular smooth muscle cells orchestrate the assembly of type I collagen via α2β1 integrin, RhoA and fibronectin polymerization. Am J Pathol (2003) 163:1045–56. doi: 10.1016/S0002-9440(10)63464-5
67. Dallas SL, Sivakumar P, Jones CJ, Chen Q, Peters DM, Mosher DF, et al. Fibronectin regulates latent transforming growth factor-beta (TGF beta) by controlling matrix assembly of latent TGF beta-binding protein-1. J Biol Chem (2005) 280:18871–80. doi: 10.1074/jbc.M410762200
68. Sabatier L, Chen D, Fagotto-Kaufman C, Hubmacher D, McKee MD, Annis DS, et al. Fibrillin assembly requires fibronectin. Mol Biol Cell (2009) 20:846–58. doi: 10.1091/mbc.e08-08-0830
69. Filla MS, Faralli JA, Peotter JL, Peters DM. The role of integrins in glaucoma. Exp Eye Res (2017) 158:124–36. doi: 10.1016/j.exer.2016.05.011
70. Tomasini-Johansson BR, Zbyszynski PW, Toraason I, Peters DM, Kwon GS. PEGylated pUR4/FUD peptide inhibitor of fibronectin fibrillogenesis decreases fibrosis in murine unilateral ureteral obstruction model of kidney disease. PloS One (2018) 13. doi: 10.1371/journal.pone.0205360
71. Bowers SLK, Davis-Rodriguez S, Thomas ZM, Rudomanova V, Bacon WC, Beiersdorfer A, et al. Inhibition of fibronectin polymerization alleviates kidney injury due to ischemia-reperfusion. Amer J Physiol-Renal Physiol (2019) 316:F1293–8. doi: 10.1152/ajprenal.00117.2019
72. Altrock E, Sens C, Wuerfel C, Vasel M, Kawelke N, Dooley S, et al. Inhibition of fibronectin deposition improves experimental liver fibrosis. J Hepatol (2015) 62:625–33. doi: 10.1016/j.jhep.2014.06.010
73. Valiente-Alandi I, Potter SJ, Salvador AM, Schafer AE, Schips T, Carrillo-Salinas F, et al. Inhibiting fibronectin attenuates fibrosis and improves cardiac function in a model of heart failure. Circulation (2018) 138:1236–52. doi: 10.1161/CIRCULATIONAHA.118.034609
74. Filla M, Dimeo K, Tong T, Peters DM. Disruption of fibronectin matrix affects type IV collagen, fibrillin, and laminin deposition into extracellular matrix of human trabecular meshwork (HTM) cells. Exp Eye Res (2017) 165:7–19. doi: 10.1016/j.exer.2017.08.017
75. Faralli JA, Filla MS, McDowell CM, Peters DM. Disruption of fibronectin fibrillogenesis affects intraocular pressure (IOP) in BALB/cJ mice. PloS One (2020) 15:e0237932. doi: 10.1371/journal.pone.0237932
76. Mckeown-Longo PJ, Mosher DF. Interaction of the 70,000-mol-wt amino-terminal fragment of fibronectin with the matrix-assembly receptor of fibroblasts. J Cell Biol (1985) 100:364–74. doi: 10.1083/jcb.100.2.364
77. Bultmann H, Santas AJ, Peters DM. Fibronectin fibrillogenesis involves the heparin II binding domain of fibronectin. J Biol Chem (1998) 273:2601–9. doi: 10.1074/jbc.273.5.2601
78. Schiller HB, Hermann M, Polleux J, Vignaud T, Zanivan S, Friedel CC, et al. β1- and αv-class integrins cooperate to regulate myosin II during rigidity sensing of fibronectin-based microenvironments. Nat Cell Biol (2013) 15:625–36. doi: 10.1038/ncb2747
79. Fernandez-Sauze S, Grall D, Cseh B, Obberghen-Schilling EV. Regulation of fibronectin matrix assembly and capillary morphogenesis in endothelial cells by Rho family GTPases. Exp Cell Res (2009) 315:2092–104. doi: 10.1016/j.yexcr.2009.03.017
80. Efthymiou G, Radwanska A, Grapa A-I, Beghelli-De La Forest Divonne S, Grall D, Schaub S, et al. Fibronectin extra domains tune cellular responses and confer topographically distinct features to fibril networks. J Cell Sci (2021) 134(4):jcs252957134. doi: 10.1242/jcs.252957
81. Maurer LM, Tomasini-Johansson BR, Ma W, Annis DS, Eickstaedt NL, Ensenberger MG, et al. Extended binding site on fibronectin for the functional upstream domain of protein F1 of Streptococcus pyogenes. J Biol Chem (2010) 285:41087–99. doi: 10.1074/jbc.M110.153692
82. Faralli JA, Gagen D, Filla MS, Crotti TN, Peters DM. Dexamethasone increases αvβ3 integrin expression and affinity through a calcineurin/NFAT pathway. BBA- Mol Cell Res (2013) 1833:3306–13. doi: 10.1016/j.bbamcr.2013.09.020
83. Lutjen-Drecoll E. Functional morphology of the trabecular meshwork in primate eyes. Prog Retin Eye Res (1998) 18:91–119. doi: 10.1016/S1350-9462(98)00011-1
84. Hinz B. The myofibroblast: paradigm for a mechanically active cell. J Biomech (2010) 43:146–55. doi: 10.1016/j.jbiomech.2009.09.020
85. Roberts AL, Mavlyutov TA, Perlmutter TE, Curry SM, Harris SL, Chauhan AK, et al. Fibronectin extra domain A (FN-EDA) elevates intraocular pressure through Toll-like receptor 4 signaling. Sci Rep (2020) 10:9815. doi: 10.10.1038/s41598-020-66756-6
86. Mavlyutov TA, Myrah JJ, Chauhan AK, Liu Y, McDowell CM. Fibronectin extra domain A (FN-EDA) causes glaucomatous trabecular meshwork, retina, and optic nerve damage in mice. Cell Biosci (2022) 12:72. doi: 10.1186/s13578-022-00800-y
87. Liao Y-F, Gotwals PJ, Koteliansky VE, Sheppard D, Van De Water L. The EIIIA segment of fibronectin is a ligand for integrins α9β1 and α4β1 providing a novel mechanism for regulating cell adhesion by alternative splicing. J Biol Chem (2002) 277:14467–74. doi: 10.1074/jbc.M201100200
88. Kohan M, Muro AF, White ES, Berkman N. EDA-containing cellular fibronectin induces fibroblast differentiation through binding to α4β7 integrin receptor and MAPK/Erk 1/2-dependent signaling. FASEB J (2010) 24:4503–12. doi: 10.1096/fj.10-154435
89. Mamuya F, Duncan MK. αV integrins and TGF-β-induced EMT: a circle of regulation. J Cell Mol Med (2012) 16:445–55. doi: 10.1111/j.1582-4934.2011.01419.x
90. Junglass B, Yu AHL, Welge-Lussen U, Tamm E, Fuchshofer R. Connective tissue growth factor induces extracellular matrix deposition in human trabecular meshwork cells. Exp Eye Res (2009) 88:1065–75. doi: 10.1016/j.exer.2009.01.008
91. Junglass B, Kuespert S, Seleem AA, Struller T, Ullmann S, Bosl M, et al. Connective tissue growth factor causes glaucoma by modifying the actin cytoskeleton of the trabecular meshwork. Am J Pathol (2012) 180:2386–403. doi: 10.1016/j.ajpath.2012.02.030
92. Hennig R, Kuespert S, Haunberger A, Goepferich A, Fuchshofer R. Cyclic RGD peptides target human trabecular meshwork cells while ameliorating connective tissue growth factor-induced fibrosis. J Drug Target (2016) 24:952–9. doi: 10.3109/1061186X.2016.1163709
93. Grotendorst GR, Rahmanie H, Duncan MR. Combinatorial signaling pathways determine fibroblast proliferation and myofibroblast differentiation. FASEB J (2004) 18:469–79. doi: 10.1096/fj.03-0699com
94. Braunger BM, Fuchshofer R, Tamm ER. The aqueous humor outflow pathways in glaucoma: A unifying concept of disease mechanisms and causative treatment. Eur J Pharm Biopharm (2015) 95:173–81. doi: 10.1016/j.ejpb.2015.04.029
95. Puklin-Faucher E, Sheetz MP. The mechanical integrin cycle. J Cell Sci (2009) 122:179–86. doi: 10.1242/jcs.042127
96. Zent J, Guo LW. Signaling mechanisms of myofibroblastic activation: outside-in and inside-out. Cell Physiol Biochem (2018) 49:848–68. doi: 10.1159/000493217
97. Asano Y, Ihn A, Yamane K, Jinnin M, Tamaki K. Increased expression of the integrin αvβ5 induces the myofibroblastic differentiation of dermal fibroblasts. Am J Pathol (2006) 168:499–510. doi: 10.2353/ajpath.2006.041306
98. Tripathi RC, Li J, Chan WF, Tripathi BJ. Aqueous humor in glaucomatous eyes contains an increased level of TGF-β2. Exp Eye Res (1994) 59:723–7. doi: 10.1006/exer.1994.1158
99. Fleenor DL, Shepard AR, Hellberg PE, Jacobson N, Pang I-H, Clark AF. TGFbeta2-induced changes in trabecular meshwork: implications for intraocular pressure. Invest Ophthamol Vis Sci (2006) 47:226–34. doi: 10.1167/iovs.05-1060
100. Shepard AR, Millar JC, Pang I-H, Jacobson N, Wang WH, Clark AF. Adenoviral gene transfer of active human transforming growth factor-β2 elevates intraocular pressure and reduces outflow facility in rodent eyes. Invest Ophthamol Vis Sci (2010) 51:2067–76. doi: 10.1167/iovs.09-4567
101. Pattabiraman PP, Rinokoski T, Poeschla E, Proia A, Challa P, Rao PV. RhoA GTPase-induced ocular hypertension in a rodent model is associated with increased fibrogenic activity in the trabecular meshwork. Am J Path (2015) 185:496–512. doi: 10.1016/j.ajpath.2014.10.023
102. Lin CW, Sherman B, Moore LA, Laethem CL, Lu DW, Pattabiraman PP, et al. Discovery and preclinical development of netarsudil, a novel ocular hypotensive agent for the treatment of glaucoma. J Ocul Pharmacol Ther (2018) 34:40–51. doi: 10.1089/jop.2017.0023
103. Wipff PJ, Hinz B. Integrins and the activation of latent transforming growth factor β1- an intimate relationship. Eur J Cell Biol (2008) 87:601–15. doi: 10.1016/j.ejcb.2008.01.012
104. Munger JS, Sheppard D. Cross talk among TGF-β signaling pathways, integrins, and the extracellular matrix. Cold Harbor Perspect Biol (2012) 3:005017. doi: 10.1101/cshperspect.a0005017
105. Murphy-Ullrich JE, Suto MJ. Thrombospondin-1 regulation of latent TGF-β activation: A therapeutic target for fibrotic disease. Matrix Biol (2018) 68-69:28–53. doi: 10.1016/j.matbio.2017.12.009
106. Flugel-Koch C, Ohlmann A, Fuchshofer R, Welge-Lussen U, Tamm ER. Thrombospondin-1 in the trabecular meshwork: localization in normal and glaucomatous eyes, and induction by TGF-beta1 and dexamethasone. Exp Eye Res (2004) 79:649–63. doi: 10.1016/j.exer.2004.07.005
107. Wipff PJ, Rifkin DB, Meister J-J, Hinz B. Myofibroblast contraction activates latent TGF-β1 from the extracellular matrix. J Cell Biol (2007) 179:1311–23. doi: 10.1083/jcb.200704042
108. Marinkovic A, Mih JD, Park J-A, Liu F, Tschumperlin DJ. Improved throughput traction microscopy reveals pivotal role for matrix stiffness in fibroblast contractility and TGF-β responsiveness. Amer J Physiol-Lung Cell Mol Physiol (2012) 303:L169–80. doi: 10.1152/ajplung.00108.2012
109. Klingberg F, Chow ML, Koehler A, Boo S, Buscemi L, Quinn TM, et al. Prestress in the extracellular matrix sensitizes latent TGF-β1 for activation. J Cell Biol (2014) 207:283–97. doi: 10.1083/jcb.201402006
110. Filla MS, Meyers KA, Faralli JA, Peters DM. Overexpression and activation of αvβ3 integrin differentially affects TGFβ2 signaling in human trabecular meshwork cells. Cells (2021) 10:1923. doi: 10.10.3390/cells10081923
111. Filla MS, Faralli JA, Dunn CR, Khan H, Peters DM. NFATc1 regulation of dexamethasone-induced TGFB2 expression is cell cycle dependent in trabecular meshwork cells. Cells (2023) 12:503. doi: 10.3390/cells12030504
112. Heldin C-H, Moustakas A. Signaling receptors for TGF-β family members. Cold Spring Harb Perspect Biol (2016) 8:a022053. doi: 10.1101/cshperspect.a022053
113. Galliher AJ, Schiemann WP. β3 Integrin and Src facilitate transforming growth factor-β mediated induction of epithelial-mesenchymal transition in mammary epithelial cells. Breast Cancer Res (2006) 8:R42. doi: 10.1186/bcr1524
114. Tsukamoto T, Kajiwara K, Nada S, Okada M. Src mediates TGF-β-induced intraocular pressure elevation in glaucoma. J Cell Physiol (2018) 234:1730–44. doi: 10.1002/jcp.27044
115. Sharma A, Tiwari A, Bhoria P, Gupta V, Gupta A, Luthra-Guptasarma M. Fibrotic remodeling of the extacellular matrix through a novel (engineered, dual-function) antibody reactive to a cryptic epitope on the N-terminal 30 kDa fragment of fibronectin. PloS One (2013) 6:e69343. doi: 10.1371/journal.prone.0069343
Keywords: integrins, trabecular meshwork, fibronectin, extracellular matrix, fibrosis, TGFβ2, myofibroblast
Citation: Faralli JA, Filla MS and Peters DM (2023) Role of integrins in the development of fibrosis in the trabecular meshwork. Front. Ophthalmol. 3:1274797. doi: 10.3389/fopht.2023.1274797
Received: 08 August 2023; Accepted: 09 October 2023;
Published: 24 October 2023.
Edited by:
Teri L. Belecky-Adams, Purdue University Indianapolis, United StatesReviewed by:
Padmanabhan Paranji Pattabiraman, Purdue University Indianapolis, United StatesCopyright © 2023 Faralli, Filla and Peters. This is an open-access article distributed under the terms of the Creative Commons Attribution License (CC BY). The use, distribution or reproduction in other forums is permitted, provided the original author(s) and the copyright owner(s) are credited and that the original publication in this journal is cited, in accordance with accepted academic practice. No use, distribution or reproduction is permitted which does not comply with these terms.
*Correspondence: Donna M. Peters, ZG1wZXRlcjJAd2lzYy5lZHU=
Disclaimer: All claims expressed in this article are solely those of the authors and do not necessarily represent those of their affiliated organizations, or those of the publisher, the editors and the reviewers. Any product that may be evaluated in this article or claim that may be made by its manufacturer is not guaranteed or endorsed by the publisher.
Research integrity at Frontiers
Learn more about the work of our research integrity team to safeguard the quality of each article we publish.