- 1Faculty of Medicine, University of Geneva (UNIGE), Geneva, Switzerland
- 2Department of Radiation Oncology, Geneva University Hospitals (HUG), Geneva, Switzerland
In cancer treatment, mild hyperthermia (HT) represents an old, but recently revived opportunity to increase the efficacy of radiotherapy (RT) without increasing side effects, thereby widening the therapeutic window. HT disrupts cellular homeostasis by acting on multiple targets, and its combination with RT produces synergistic antitumoral effects on specific pathophysiological mechanisms, associated to DNA damage and repair, hypoxia, stemness and immunostimulation. HT is furthermore associated to direct tumor cell kill, particularly in higher temperature levels. A phenomenon of temporary resistance to heat, known as thermotolerance, follows each HT session. Cancer treatment requires innovative concepts and combinations to be tested but, for a meaningful development of clinical trials, the understanding of the underlying mechanisms of the tested modalities is essential. In this mini-review, we aimed to describe the synergistic effects of the combination of HT with RT as well as the phenomena of thermal shock and thermotolerance, in order to stimulate clinicians in new, clinically relevant concepts and combinations, which become particularly relevant in the era of technological advents in both modalities but also cancer immunotherapy.
Highlights
● The combination of radiotherapy and mild hyperthermia has attracted increasing interest in recent years and remains a promising yet relatively poorly studied approach for cancer treatment. To incite future, innovative concepts of the association of both modalities, their underlying mechanisms, interactions and potential need to be understood. This review summarizes current knowledge of the above and aims to be a helpful resource for guiding new preclinical and clinical studies advancing this opportunity.
1 Introduction
Treating cancer with heat is known since the 19th century: W.B. Coley was able to cause their tumor regression, in patients to whom he administered preparations of killed bacterial cultures (“Coley’s toxin”), and thus induced fever (1, 2). Mild hyperthermia (HT) is still today a therapeutic anticancer modality, where a tissue is exogenously heated to temperatures between 39–45°C, using electromagnetic waves, ultrasound, thermal conduction, or hyperthermic infusion (3). Using ionizing irradiation to shrink tumors is known since the early 20th century: since the discovery of radium by Marie Curie, radiotherapy (RT) has made a tremendous progress, both in the fields of biology and technology and became one of the pillars of cancer treatment. The synergistic combination of both HT and RT is known since the 1970s, where thermoradiotherapy (TRT), at first at a preclinical level (2, 4), then confirmed at meta-analysis clinical level, has been shown to improve outcomes, as compared to RT alone, without increasing serious adverse effects, at least for several advanced cancers, such as breast (5), cervical (6), esophageal (7), and head and neck (8). Today, a revived interest in TRT is observed, possibly because the underlying mechanisms of the synergy between RT and HT might create new opportunities to further improve therapeutic outcomes in modern settings, such as, for instance, the context of immunotherapy (9). Therefore, understanding the biological mechanisms of the synergistic effect of TRT might help conceptualize innovative associations to be tested in future clinical studies and becomes clinically relevant. We thus aimed to summarize and put into perspective the established knowledge on the interactions between HT and RT but also their limitations, to probe modern research in the field.
2 The synergy of thermoradiotherapy: two pieces in a puzzle
2.1 DNA damage
Radiation-induced DNA damage is the pivotal, classical radiobiologic event regarded as the major effect of RT in both tumors and healthy tissues (10). This occurs either by direct ionization or, predominantly, by inducing radiolysis of water molecules, resulting in the production of free-oxygen radicals (ROS), which then induce DNA oxidation (11, 12). Such damage includes DNA-base alterations, DNA-DNA/DNA-protein cross-links, single-strand breaks and double-strand breaks, the latter being the most lethal (13, 14). The accumulation of unrepaired DNA lesions activates the cell-cycle checkpoint machinery (ATM/p53/p21 or ATM/CHK2/CDC25A-C pathways), leading to the temporary arrest of the cell cycle. If DNA damage is not quickly repaired, the cell-cycle checkpoint machinery remains active, thereby promoting cell death (via mitotic catastrophe, apoptosis, senescence or autophagy) (13, 15, 16). Therefore, the greater the efficiency of DNA repair mechanisms, the lower the cytotoxicity of ionizing radiation. This is the key to the therapeutic effect of RT: tumor cells, due to their reduced capacity to repair DNA, are more sensitive to RT than healthy cells, thus creating a therapeutic window that is explored in the clinical setting and makes RT a very efficient anti-cancer treatment (13, 15, 17).
Heat, especially at temperatures exceeding ≈41°C, damages several proteins involved in DNA repair, called DNA-damage responses (DDRs). DDRs repairing double-strand breaks are particularly affected, i.e., non-homologous end joining (NHEJ), homologous recombination (HR) and back-up non-homologous end joining (back-up NHEJ), thus making HT a great tool to enhance the cytotoxicity of ionizing radiation (14, 15, 18, 19). Moreover, heat also slows DNA replication in tumor cells, thus reducing their reproductive capacity (14, 18, 19). It is highly controversial whether HT, by producing ROS, can directly cause single-or double-strand breaks (18, 19).
Radioresistance is dominated by the moment in the cell cycle where exposure to irradiation occurs: cells in the synthesis (S) phase, as compared to cells in G1, G2 or mitotic (M) phase, are particularly radioresistant, because their DDRs are physiologically upregulated. Therefore, HT, which damages DDRs, can radiosensitize previously radioresistant cells (13, 19). In terms of synergy, both RT and HT effects on DNA damage and repair display a very interesting complementarity, which can widen the therapeutic window of RT.
2.2 Hypoxia
Hypoxia is another challenge for therapeutic RT, as it is directly associated to radioresistance. Indeed, as a tumor grows, the local vasculature becomes insufficient to support its growing nutrient needs. Cancer cells and other cells situated in the tumor microenvironment (TME) thus secrete many pro-angiogenic factors, especially vascular endothelial growth factor (VEGF), leading to the development of an abnormal local vascular network. Tumor blood vessels are dilated, tortuous, immature, highly permeable and heterogeneously distributed. This abnormal vascular network is unable to properly vascularize the tumor, tumoral oxygen requirements remain higher than its availability, and the microenvironment becomes hypoxic (20–22).
The generation of ROS by ionizing radiation, the primary mediator of RT cytotoxicity, is chemically dependent on the local partial pressure of oxygen (pO2). Therefore, a chronically hypoxic TME favors radioresistance (11, 12, 23). Indeed, hypoxia is associated with poor clinical prognosis, because it not only increases resistance to treatment, but also intrinsically enhances the tumor malignancy, through the activation of hypoxia-inducible factors (HIFs), which stimulate angiogenesis, reduce p53 expression, inhibit apoptosis, and reduce the immune system response (24–27).
HT, up to ≈ 42°C, induces a transient vasodilation of abnormal tumor blood vessels and an increase of vascular permeability, leading to an increase in tumor perfusion. Therefore, the TME regains a normal oxygen partial pressure (pO2), a normal concentration of nutrients and a normal pH, which reverse the established radioresistance (14, 25, 28–30). The duration of the reoxygenation is still controversial but may last up to 24 hours (14, 25). Nonetheless, this mechanism becomes counterproductive at temperatures above ≈ 42°C: heat causes vascular damage, leading to vascular occlusion and thus to a prolonged worsening of local hypoxia, with hypoxic cells dying from ischemia (14, 15, 25, 28, 30) Therefore, the radiosensitizing vascular effects of HT may only be achievable at temperatures below ≈ 42°C. Of note, HT-induced reoxygenation of tumor tissue could be further enhanced by the addition of drugs that increase local oxygen availability, such as oxygen mimetics (e.g., misonidazole), mitochondrial respiration inhibitors (e.g., atovaquone), carbogen gas breathing, or oxygen-carrying nanoparticles (11, 28, 31).
2.3 Cancer stem cells
Cancer stem cells (CSCs) represent an important challenge of cancer treatment. Indeed, this small subpopulation of cancer cells promotes cancer initiation, progression, metastasis, treatment resistance and recurrence (32–34). They possess some characteristics of normal stem cells, such as self-renewal and unlimited proliferation capacities (14, 31, 33, 35). CSCs are harbored particularly in hypoxic areas of the tumor, because a hypoxic TME promotes stem-like properties, inhibits cellular differentiation and apoptosis (32, 35, 36). These specific microenvironments are called “niches”: they are surrounded and symbiotically collaborate with cancer-associated cells to increase proliferation, resist to treatment, evade the immune system, metastasize and differentiate into multiple tumor cell types (35, 37). Due to oxygen and nutrient local deprivation, a fraction of CSCs, as well as chronically hypoxic tumor cells, is maintained in a state of quiescence (i.e., cell cycle reversibly arrested in G0 phase). Quiescent cells no longer undergo mitosis and upregulate their DNA repair pathways, rendering them constitutively resistant to cytotoxic treatments (32, 38) and DNA-damaging agents, such as RT (31–33, 37–39). Some evidences suggests that HT may be able to radiosensitize CSCs, as well as quiescent tumor cells, although the underlying pathophysiological mechanisms are not fully understood, but may include HT-induced TME reoxygenation and damage to DDRs (14, 32, 33, 40, 41).
2.4 Immunostimulation
2.4.1 Hyperthermia-induced immunostimulation
HT possesses immunostimulatory effects only. During an infection, the hypothalamus induces fever in response to elevated blood levels of cytokines, especially IL-1, IL-6 and TNF-α. In addition to inhibiting bacterial replication, fever creates a pro-inflammatory state in tissues that allows for increased immunogenicity of immune cells. The immune effects of fever may be reproducible with local HT, potentiating immune targeting of neoplastic cells escaping immunosurveillance (42). Heat-induced activation of the immune system is mediated by heat shock proteins (HSPs); they increase antigen presentation and maturation of dendritic cells, stimulate the phagocytic function of macrophages, induce the release of tumor neoantigens from tumor cells, favor the proliferation and cytotoxicity of CD8+ T and NK cells, promote the activation and proliferation of B lymphocytes and inhibit T-regulatory (Treg) cells. Heat-stimulated macrophages produce cytokines that increase the expression of cell-adhesion molecules on endothelial cells to facilitate leukocyte infiltration into the tissue (42–46). Mild HT also appears to induce immunogenic cell death (ICD), a specific type of apoptosis that promotes an adaptive immune response (44, 45, 47). Finally, the vasodilatory effect and the increase in vascular permeability produced by HT facilitate the arrival and penetration of immune cells into the heated tissue (28, 43).
2.4.2 Radiotherapy-induced immunostimulation/immunosuppression
RT, however, has both immunostimulatory and immunosuppressive effects. Indeed, RT induces local secretion of certain immunostimulatory pro-inflammatory cytokines (IFNs, IL-1β) that facilitate T-cell entry into the tumor (by upregulating adhesion molecules on the endothelium) and induces activation and enhanced antigen-presenting capacity of dendritic cells and T cells (22, 46–48). RT also appears to increase the expression of MHC-I and NKG2D receptors on the tumor surface, which, respectively, facilitates tumor cell recognition by T cells and enhances NK cells activity and cytotoxicity (22, 48–50). RT is thus a known trigger of ICD (22, 48, 50, 51). However, RT induces the secretion of various chemokines, some of which attract immunostimulatory cells (dendritic, T and NK cells), but others immunosuppressing cells (Treg, tumor associated macrophages (TAMs), myeloid-derived suppressor cells (MDSCs)), the latter facilitating tumor progression. Therefore, RT is considered a double-edged sword, in terms of tumor immunogenicity (22, 48). Ionizing radiation also damages leukocytes situated in the tumor and stimulates the secretion of anti-inflammatory cytokines (TGF-β, IL-6, IL-10, CSF-1), which reduce the antigen-presenting abilities of dendritic cells, reduces T CD8+ lymphocytes cytotoxicity and CD4+ cell differentiation, attracts cancer-associated fibroblasts (CAFs), creates a radioresistant state in tumors and contributes to tumor cells’ proliferation and invasion (22, 48). Upregulation of PD-L1 has also been observed after RT (22, 48, 52). Furthermore, doses >10Gy/fraction destroy abnormal fragile tumor vessels: this not only impedes the infiltration of immune cells into the tumor but also exacerbates local hypoxia, thereby inducing tumor radioresistance (22).
Some evidence suggests that the addition of HT to RT leads to an increase in tumor immunogenicity (53–56). However, to optimize the combination of RT-HT from an immunostimulatory point of view, a better understanding of the ideal parameters of both modalities is needed: optimal fractionation, total dose and sequence of RT with immune checkpoint inhibitors (ICIs) are still under investigation, with data suggesting that moderate hypofractionated regimens are the most promising (22, 48–50, 57), while for HT, the ideal temperature frame is unknown, with fever-range temperatures up to 41°C being potential optimal HT parameters for immunostimulation, as some immunostimulatory effects only occur at specific temperatures (42, 43). In the context of RT-induced immunostimulation, a rare, still increasingly sought, phenomenon, called the abscopal effect, has been observed: it consists of the regression of non-irradiated lesions, suggesting a systemic and immunogenic effect of RT, a classically considered “local” treatment. Although incompletely understood, it seems that the abscopal effect represents the distant effect of in-situ, tumor-specific immunostimulation, through activation of antigen-presenting cells following the local release of tumor-associated antigens (TAAs) after RT, leading to the activation of T CD8+ lymphocytes and consequent tumor destruction by cell-mediated immunity (22, 48, 49, 57). The abscopal effect seems indeed to occur more frequently when ICIs are administered (48, 49, 57, 58). Interestingly, putative abscopal responses have been reported in patients treated with RT and HT, although these remain at an exploratory level (59), with ferroptosis having been suggested as an implicated mechanism (53–56). Importantly, ICIs can be added to the combination of RT and HT, promising to further enhance the immunostimulatory combination of both (42–45, 48–50).
3 Hyperthermia-intrinsic effects
3.1 Direct cytotoxicity related to thermal shock
HT also exerts a direct cytotoxicity, which occurs particularly at temperatures above ≈ 42°C and increases as the temperature rises (14, 60). When a cell undergoes a thermal shock, intracellular proteins are denatured and unfolded, and end up aggregating (14, 28, 61, 62). All cellular proteins are affected: nuclear proteins malfunction, the cytoskeleton collapses and the organelles responsible for protein production (ER and Golgi) are damaged. Since nuclear proteins are fragile, a temperature of 40°C is sufficient to damage them (28). The centrosome is also affected, leading cells undergoing mitosis, particularly tumor cells which are unable to slow replication to repair damage, to die by mitotic catastrophe (62). Moreover, membrane lipids are altered, leading to increased cellular membrane permeability and mitochondrial uncoupling (28). Mitochondrial dysfunctions result in a significant release of ROS, closing the circle by indirectly bringing linking HT to DNA damage (18, 28). Through these various cellular pathways, HT appears to have the capacity to kill a portion of heated tumor cells by apoptosis (<43°C) or necrosis (>43°C), particularly in the most acidic and hypoxic areas of tumors (14, 24, 28, 63). However, the direct killing by HT seems to be significant only at temperatures above those optimizing radiosensitization (14, 24). Furthermore, protumorigenic endothelial cells and fibroblasts in the TME are particularly sensitive to thermal shock (32). Interestingly, some drugs can have a heat-sensitizing effect by modulating cell apoptosis pathways (e.g., verapamil, lidocaine), as shown in vitro (61), which suggests the theoretical opportunity to use thermos-sensitizers, a nice parallel to the use of radiosensitizers to enhance RT efficacy.
3.2 Thermotolerance
Cells actively control the integrity of their proteins: after a heat shock, unfolded and aggregated proteins result in the activation of several HSPs, through the transcription of heat-shock factor 1 (HSF1) (28, 62, 64, 65). The activation of these specific chaperones, dedicated to maintaining cellular homeostasis, result in several repair-related procedures, such as the reversal of heat-induced protein misfolding, the elimination of irreparable proteins or organelles in the lysosome (autophagy), the repair of the centrosome and cytoskeleton, the upregulation of DNA-repair proteins, the inhibition of apoptosis and the production of paracrine signals inducing heat shock response in nearby cells (28, 62, 64, 66). HSPs thus provide damaged cells with an opportunity to repair themselves before dying, if the damage is reparable (28, 62, 64, 66). Furthermore, HSP pathways are involved in the promotion of local angiogenesis, cell proliferation, adhesion, invasion and metastasis (62, 64, 66). Tumor cells exhibit numerous cellular dysfunctions and thereby possess a high basal level of HSPs activation, due to their hypoxic, acidic and nutrient-deprived microenvironment as well as their rapid division and genomic instability (64, 65, 67). Therefore, tumor cells under chronic overexpression of HSPs are not only subject to resistance to HT but also have an increased malignancy (28, 46, 62, 64–67).Following HT, a transient activation of HSPs pathways results in a temporary resistance to heat, a phenomenon known as “thermotolerance” (28, 62). Thermotolerance appears a few hours after a HT session, peaks at 24 hours, and may take up to 5 days to resolve. The exact timing and intensity of the effect depends on the cell type and the heating parameters (temperature, duration, interval between sessions, etc.): for example, the higher the temperature reached in the tumor, the longer the thermotolerance seems to last (62, 68). This has important clinical implications, as thermotolerance renders the radiosensitizing effect of HT transient, thus requiring the therapeutic interventions to be performed within an overall short time (short therapeutic window). It also requires the HT sessions to be separated by at least 48–72 hours, limiting the number of sessions that can be performed per week to one or two. Indeed, performing daily HT sessions considerably reduces its effect (28, 60, 68) and should be avoided. Alternatively, HSPs- inhibitors can be applied to reduce thermotolerance and possibly decrease tumor aggressiveness, but with the risk of a reduced heat-induced immune response (28, 46, 62, 64–67, 69).
4 Key mechanisms of the interaction between HT-RT
This work aimed to concisely review the underlying mechanisms of interaction between RT and HT, with the goal of bringing this synergistic effect closer to current clinical research focus. Indeed, most of the hallmarks of cancer are addressed in a beautifully complementary manner by the combination of the two modalities (Figure 1):
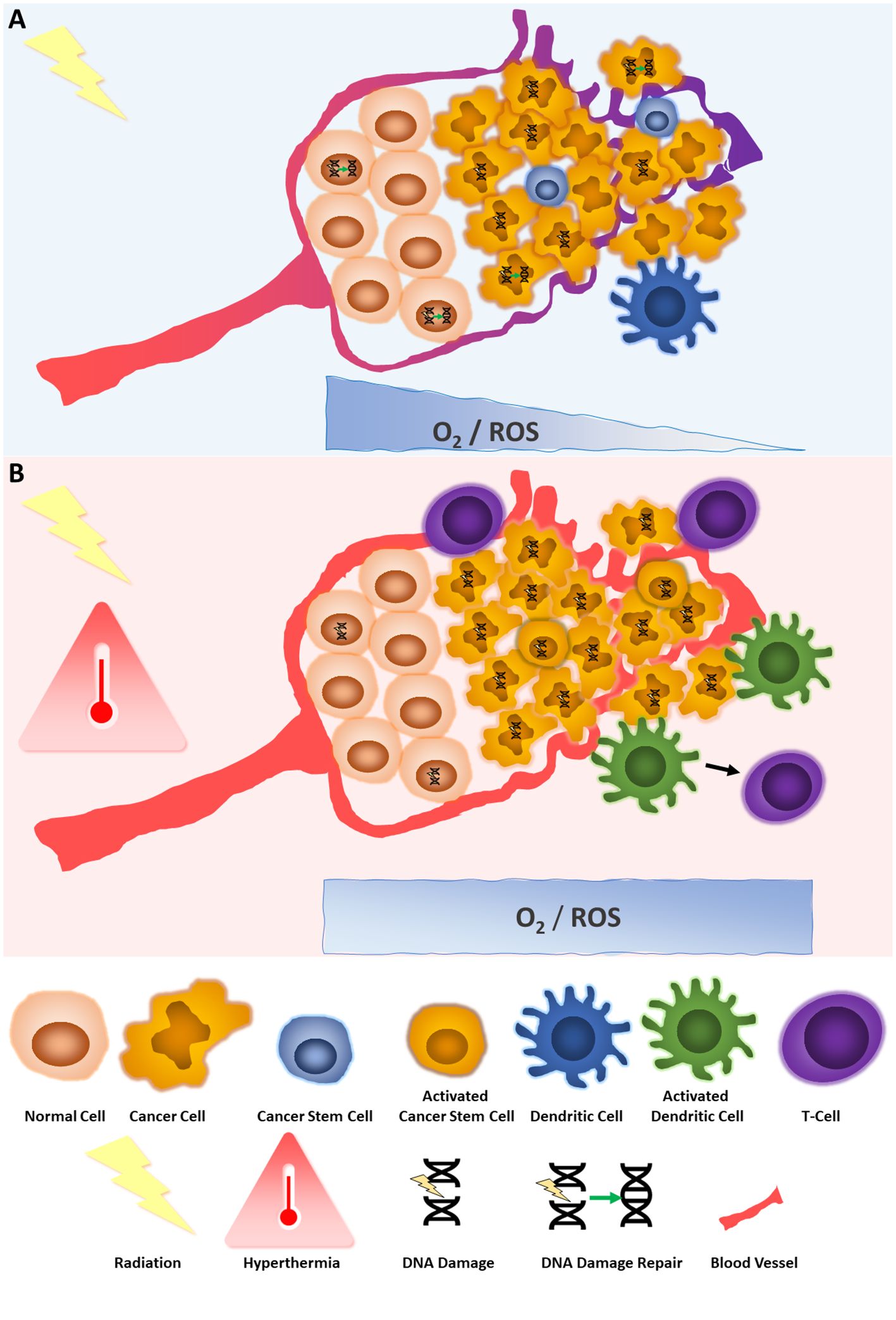
Figure 1 Key mechanisms of the interaction between hyperthermia and radiotherapy. (A) The effect of radiotherapy on tumor and normal cells. Radiation provokes DNA damage, in dividing cells, dependent on oxygen levels and therefore production of ROS. DNA breaks are repaired more readily in normal cells than cancer cells. Vessels around tumor cells are abnormal and do not supply sufficient oxygen, leading to hypoxia and less radiation-induced DNA damage. Cancer stem cells are in quiescent state and poorly affected by irradiation. Irradiation can also affect immune cells, such as dendritic cells but its immunostimulatory role is context dependent. (B) The effect of radiotherapy combined with hyperthermia on tumor and normal cells. Hyperthermia potentializes the effects of irradiation by reducing DNA damage repair, increasing oxygen levels by vessel dilation, increasing ROS, bringing cancer stem cells out of their quiescent state, and stimulating the immune system such as dendritic and T-cells against the tumor.
DNA damage: As a major radiobiologic event, it depends on ROS, leading to the temporary arrest of the cell cycle. If not quickly repaired, it will lead to cell death. Tumor cells have a reduced capacity of DNA-repair, thus are more sensitive to RT than healthy cells. HT damages several DDRs, thus enhancing RT cytotoxicity. Radioresistance is dominated by the cell cycle and the presence of DDRs: thus, TRT reverses radioresistance.
Hypoxia: Directly associated to radioresistance, hypoxia becomes present as tumor grows and local vasculature becomes insufficient to support its nutrient needs, leading to the development of an anarchic vascular network, reducing local oxygen partial pressure (pO2). HT vasodilates transitorily abnormal tumor blood vessels, inducing an increase in tumor perfusion. Therefore, the TME is reoxygenated, reversing radioresistance. However, this mechanism is only valid for temperatures <42°C.
Stemness: CSCs promote cancer initiation, progression, metastasis, treatment resistance and recurrence and are harbored particularly in hypoxic areas of the tumor, leading to radioresistance. HT appears to radiosensibilize CSCs, although the underlying pathophysiological processes are not understood.
Immunostimulation: HT possesses only immunostimulatory effects, through inflammation, mediated by HSPs, leading to increased immunogenicity, leukocyte infiltration, vasodilation and ultimately ICD. RT produces both immunostimulatory and immunosuppressive effects: by releasing TAAs, stimulating inflammatory cytokines, promoting dendritic cells maturation and preparing antigen presentation to T and NK cells by the immune host system, it makes the tumor an in situ vaccine, while, by damaging leukocytes situated in the tumor, stimulating other anti-inflammatory cytokines and attracting CAFs/TAMs/MDSCs/Treg, it can create a radioresistant state in tumors and upregulate PD-L1. The RT-induced abscopal effect is an immunogenic effect that could potentially be enhanced with TRT.
Two HT-intrinsic mechanisms of action, which represent compensatory responses of cell to heat, are also described: direct tumor kill related to thermal shock, present only at higher temperatures, can further contribute to HT cytotoxicity; thermotolerance, on the other hand, represents a limitation of HT for anticancer treatments.
5 Challenges, perspectives, and conclusion
Several physiological mechanisms explaining the synergy of TRT are now understood. Nevertheless, other gray zones remain, such as the optimal temperature for achieving the most effective radiosensitizing effect, the duration of heat-induced vasodilation (14, 24), the pathophysiology underlying CSCs and quiescent cells radiosensitization by HT (32), the exact molecular mechanisms underlying the DNA repair inhibition (18, 19) and the optimal parameters to obtain clinically meaningful benefits from the TRT-induced immunostimulation (42, 43) (22, 48–50).
Several strategies to enhance both the local and systemic effects of TRT in tumor kill are attempted or provide interesting opportunities to be explored: the addition of systemic therapies, such as ICIs (43–45), drugs increasing local oxygen availability (11, 27, 30), vascular-disrupting agents (VDAs) (14, 70), DNA-repair inhibitors (16, 18, 19), HSP inhibitors (28, 62, 69), antioxidant enzyme inhibitors (12, 71) or heat-sensitizing molecules (61) could theoretically increase the synergistic effect. This, however, remains to be seen: the paradigm of radiation sensitizers and radioprotectors and the challenges of its clinical implementation has shown that these combinations can be challenging in terms of selectivity and practical implementation (72).
Both the technological evolution as well as the improved understanding at a mechanistic level, of both RT and HT, offers exciting opportunities: high-precision radiotherapy, resulting in oncological efficacy and remarkably improved treatment tolerance, in the field of RT, improved heat delivery planning and thermometry (e.g., MRI thermometry), accumulation of improved quality clinical data, in the field of HT, promise new therapeutic opportunities for modern TRT.
An increasing number of preclinical and clinical studies are focusing on heating tumors with nanoparticles: magnetic nanoparticles (MNPs) generate heat when exposed to an alternating magnetic field, while gold nanoparticles are excited by light (73). Injected intravenously, nanoparticles have the advantage to preferentially accumulate in tumor tissues due to the abnormal permeability of their immature neo-vessels (Enhanced Permeability and Retention effect). Absorbed by tumor cells through endocytosis, nanoparticles can also directly cause various cellular dysfunctions, such as oxidative stress, cytoskeleton disruption or DNA damage (73, 74). Since nanoparticles hold great promise for heating tumors more precisely and selectively, they are now being clinically tested in association with RT for the treatment of glioblastoma and prostate cancer, and are in preclinical stages for other indications (75, 76). Although a lot needs to be undertaken before these advancements become ready for prime-time, they promise high-precision TRT and could permit to refine the already established synergy between RT and HT. These perspectives hold promise for cancer cure and merit study with established modern tools, incorporating translational aspects that will then be brought to the patient.
Author contributions
MR: Conceptualization, Investigation, Methodology, Visualization, Writing – original draft, Writing – review & editing. AD: Conceptualization, Methodology, Project administration, Supervision, Visualization, Writing – review & editing. PT: Conceptualization, Methodology, Project administration, Supervision, Visualization, Writing – review & editing.
Funding
The author(s) declare financial support was received for the research, authorship, and/or publication of this article. Open access funding by University of Geneva.
Acknowledgments
This review was written as part of MR’s medicine master thesis.
Conflict of interest
The authors declare that the research was conducted in the absence of any commercial or financial relationships that could be construed as a potential conflict of interest.
Publisher’s note
All claims expressed in this article are solely those of the authors and do not necessarily represent those of their affiliated organizations, or those of the publisher, the editors and the reviewers. Any product that may be evaluated in this article, or claim that may be made by its manufacturer, is not guaranteed or endorsed by the publisher.
References
1. Coley WB. The treatment of Malignant tumors by repeated inoculations of erysipelas. With a report of ten original cases. 1893. Clin Orthop Relat Res. (1991) 262):3–11.
2. Overgaard J. The heat is (still) on–the past and future of hyperthermic radiation oncology. Radiother Oncol. (2013) 109:185–7. doi: 10.1016/j.radonc.2013.11.004
3. Kok HP, Cressman ENK, Ceelen W, Brace CL, Ivkov R, Grull H, et al. Heating technology for Malignant tumors: a review. Int J Hyperthermia. (2020) 37:711–41. doi: 10.1080/02656736.2020.1779357
4. Emami B, Song CW. Physiological mechanisms in hyperthermia: a review. Int J Radiat Oncol Biol Phys. (1984) 10:289–95. doi: 10.1016/0360-3016(84)90015-4
5. Datta NR, Puric E, Klingbiel D, Gomez S, Bodis S. Hyperthermia and radiation therapy in locoregional recurrent breast cancers: A systematic review and meta-analysis. Int J Radiat Oncol Biol Phys. (2016) 94:1073–87. doi: 10.1016/j.ijrobp.2015.12.361
6. Datta NR, Stutz E, Gomez S, Bodis S. Efficacy and safety evaluation of the various therapeutic options in locally advanced cervix cancer: A systematic review and network meta-analysis of randomized clinical trials. Int J Radiat Oncol Biol Phys. (2019) 103:411–37. doi: 10.1016/j.ijrobp.2018.09.037
7. Hu Y, Li Z, Mi DH, Cao N, Zu SW, Wen ZZ, et al. Chemoradiation combined with regional hyperthermia for advanced oesophageal cancer: a systematic review and meta-analysis. J Clin Pharm Ther. (2017) 42:155–64. doi: 10.1111/jcpt.2017.42.issue-2
8. Datta NR, Rogers S, Ordonez SG, Puric E, Bodis S. Hyperthermia and radiotherapy in the management of head and neck cancers: A systematic review and meta-analysis. Int J Hyperthermia. (2016) 32:31–40. doi: 10.3109/02656736.2015.1099746
9. Zhang Z, Liu X, Chen D, Yu J. Radiotherapy combined with immunotherapy: the dawn of cancer treatment. Signal Transduction Targeted Ther. (2022) 7:258. doi: 10.1038/s41392-022-01102-y
10. Chalmers AJ, Carruthers RD. Radiobiology summaries: DNA damage and repair. Clin Oncol. (2021) 33:275–8. doi: 10.1016/j.clon.2020.12.006
11. Li C, Wiseman L, Okoh E, Lind M, Roy R, Beavis AW, et al. Exploring hypoxic biology to improve radiotherapy outcomes. Expert Rev Mol Med. (2022) 24:e21. doi: 10.1017/erm.2022.14
12. Wang H, Jiang H, Van De Gucht M, De Ridder M. Hypoxic radioresistance: can ROS be the key to overcome it? Cancers (Basel). (2019) 11(1):112. doi: 10.3390/cancers11010112
13. Thoms J, Bristow RG. DNA repair targeting and radiotherapy: a focus on the therapeutic ratio. Semin Radiat Oncol. (2010) 20:217–22. doi: 10.1016/j.semradonc.2010.06.003
14. Elming PB, Sorensen BS, Oei AL, Franken NAP, Crezee J, Overgaard J, et al. Hyperthermia: the optimal treatment to overcome radiation resistant hypoxia. Cancers (Basel). (2019) 11(1):60. doi: 10.3390/cancers11010060
15. Oei AL, Kok HP, Oei SB, Horsman MR, Stalpers LJA, Franken NAP, et al. Molecular and biological rationale of hyperthermia as radio- and chemosensitizer. Adv Drug Delivery Rev. (2020) 163-164:84–97. doi: 10.1016/j.addr.2020.01.003
16. Huang RX, Zhou PK. DNA damage response signaling pathways and targets for radiotherapy sensitization in cancer. Signal Transduct Target Ther. (2020) 5:60. doi: 10.1038/s41392-020-0150-x
17. Carusillo A, Mussolino C. DNA damage: from threat to treatment. Cells. (2020) 9(7):1665. doi: 10.3390/cells9071665
18. Mantso T, Goussetis G, Franco R, Botaitis S, Pappa A, Panayiotidis M. Effects of hyperthermia as a mitigation strategy in DNA damage-based cancer therapies. Semin Cancer Biol. (2016) 37-38:96–105. doi: 10.1016/j.semcancer.2016.03.004
19. Oei AL, Vriend LE, Crezee J, Franken NA, Krawczyk PM. Effects of hyperthermia on DNA repair pathways: one treatment to inhibit them all. Radiat Oncol. (2015) 10:165. doi: 10.1186/s13014-015-0462-0
20. Viallard C, Larrivee B. Tumor angiogenesis and vascular normalization: alternative therapeutic targets. Angiogenesis. (2017) 20:409–26. doi: 10.1007/s10456-017-9562-9
21. Horsman MR, Vaupel P. Pathophysiological basis for the formation of the tumor microenvironment. Front Oncol. (2016) 6:66. doi: 10.3389/fonc.2016.00066
22. Jarosz-Biej M, Smolarczyk R, Cichon T, Kulach N. Tumor microenvironment as A "Game changer" in cancer radiotherapy. Int J Mol Sci. (2019) 20(13):3212. doi: 10.3390/ijms20133212
23. Moloney JN, Cotter TG. ROS signalling in the biology of cancer. Semin Cell Dev Biol. (2018) 80:50–64. doi: 10.3390/ijms20133212
24. Jing X, Yang F, Shao C, Wei K, Xie M, Shen H, et al. Role of hypoxia in cancer therapy by regulating the tumor microenvironment. Mol Cancer. (2019) 18:157. doi: 10.1186/s12943-019-1089-9
25. Dunne M, Regenold M, Allen C. Hyperthermia can alter tumor physiology and improve chemo- and radio-therapy efficacy. Adv Drug Delivery Rev. (2020) 163-164:98–124. doi: 10.1016/j.addr.2020.07.007
26. Fu Z, Mowday AM, Smaill JB, Hermans IF, Patterson AV. Tumour hypoxia-mediated immunosuppression: mechanisms and therapeutic approaches to improve cancer immunotherapy. Cells. (2021) 10:1006. doi: 10.3390/cells10051006
27. Korbecki J, Siminska D, Gassowska-Dobrowolska M, Listos J, Gutowska I, Chlubek D, et al. Chronic and cycling hypoxia: drivers of cancer chronic inflammation through HIF-1 and NF-kappaB activation: A review of the molecular mechanisms. Int J Mol Sci. (2021) 22(19):10701. doi: 10.3390/ijms221910701
28. Vilaplana-Lopera N, Besh M, Moon EJ. Targeting hypoxia: revival of old remedies. Biomolecules. (2021) 11(11):1604. doi: 10.3390/biom11111604
29. Scutigliani EM, Liang Y, Crezee H, Kanaar R, Krawczyk PM. Modulating the heat stress response to improve hyperthermia-based anticancer treatments. Cancers (Basel). (2021) 13(6):1243. doi: 10.3390/cancers13061243
30. Vaupel P, Piazena H, Notter M, Thomsen AR, Grosu AL, Scholkmann F, et al. From localized mild hyperthermia to improved tumor oxygenation: physiological mechanisms critically involved in oncologic thermo-radio-immunotherapy. Cancers (Basel). (2023) 15(5):1394. doi: 10.3390/cancers15051394
31. Wang H, Li J, Wang Y, Gong X, Xu X, Wang J, et al. Nanoparticles-mediated reoxygenation strategy relieves tumor hypoxia for enhanced cancer therapy. J Control Release. (2020) 319:25–45. doi: 10.1016/j.jconrel.2019.12.028
32. Paul R, Dorsey JF, Fan Y. Cell plasticity, senescence, and quiescence in cancer stem cells: Biological and therapeutic implications. Pharmacol Ther. (2022) 231:107985. doi: 10.1016/j.pharmthera.2021.107985
33. Oei AL, Vriend LEM, Krawczyk PM, Horsman MR, Franken NAP, Crezee J. Targeting therapy-resistant cancer stem cells by hyperthermia. Int J Hyperthermia. (2017) 33:419–27. doi: 10.1080/02656736.2017.1279757
34. Huang H, Yu K, Mohammadi A, Karanthanasis E, Godley A, Yu JS. It's getting hot in here: targeting cancer stem-like cells with hyperthermia. J Stem Cell Transplant Biol. (2017) 2(2):113.
35. Yang L, Shi P, Zhao G, Xu J, Peng W, Zhang J, et al. Targeting cancer stem cell pathways for cancer therapy. Signal Transduct Target Ther. (2020) 5:8. doi: 10.1038/s41392-020-0110-5
36. Emami Nejad A, Najafgholian S, Rostami A, Sistani A, Shojaeifar S, Esparvarinha M, et al. The role of hypoxia in the tumor microenvironment and development of cancer stem cell: a novel approach to developing treatment. Cancer Cell Int. (2021) 21:62. doi: 10.1186/s12935-020-01719-5
37. Plaks V, Kong N, Werb Z. The cancer stem cell niche: how essential is the niche in regulating stemness of tumor cells? Cell Stem Cell. (2015) 16:225–38. doi: 10.1016/j.stem.2015.02.015
38. Skvortsov S, Debbage P, Lukas P, Skvortsova I. Crosstalk between DNA repair and cancer stem cell (CSC) associated intracellular pathways. Semin Cancer Biol. (2015) 31:36–42. doi: 10.1016/j.semcancer.2014.06.002
39. Zhang J, Si J, Gan L, Di C, Xie Y, Sun C, et al. Research progress on therapeutic targeting of quiescent cancer cells. Artif Cells Nanomed Biotechnol. (2019) 47:2810–20. doi: 10.1080/21691401.2019.1638793
40. Man J, Shoemake JD, Ma T, Rizzo AE, Godley AR, Wu Q, et al. Hyperthermia sensitizes glioma stem-like cells to radiation by inhibiting AKT signaling. Cancer Res. (2015) 75:1760–9. doi: 10.1158/0008-5472.CAN-14-3621
41. Masunaga S, Ono K, Akaboshi M, Nishimura Y, Suzuki M, Kinashi Y, et al. Reduction of hypoxic cells in solid tumours induced by mild hyperthermia: special reference to differences in changes in the hypoxic fraction between total and quiescent cell populations. Br J Cancer. (1997) 76:588–93. doi: 10.1038/bjc.1997.430
42. Lee S, Son B, Park G, Kim H, Kang H, Jeon J, et al. Immunogenic effect of hyperthermia on enhancing radiotherapeutic efficacy. Int J Mol Sci. (2018) 19(9):2795. doi: 10.3390/ijms19092795
43. Toraya-Brown S, Fiering S. Local tumour hyperthermia as immunotherapy for metastatic cancer. Int J Hyperthermia. (2014) 30:531–9. doi: 10.3109/02656736.2014.968640
44. Yang X, Gao M, Xu R, Tao Y, Luo W, Wang B, et al. Hyperthermia combined with immune checkpoint inhibitor therapy in the treatment of primary and metastatic tumors. Front Immunol. (2022) 13:969447. doi: 10.3389/fimmu.2022.969447
45. Liu P, Ye M, Wu Y, Wu L, Lan K, Wu Z. Hyperthermia combined with immune checkpoint inhibitor therapy: Synergistic sensitization and clinical outcomes. Cancer Med. (2023) 12:3201–21. doi: 10.1002/cam4.5085
46. Albakova Z, Mangasarova Y. The HSP immune network in cancer. Front Immunol. (2021) 12:796493. doi: 10.3389/fimmu.2021.796493
47. Kroemer G, Galluzzi L, Kepp O, Zitvogel L. Immunogenic cell death in cancer therapy. Annu Rev Immunol. (2013) 31:51–72. doi: 10.1146/annurev-immunol-032712-100008
48. Liu Y, Dong Y, Kong L, Shi F, Zhu H, Yu J. Abscopal effect of radiotherapy combined with immune checkpoint inhibitors. J Hematol Oncol. (2018) 11:104. doi: 10.1186/s13045-018-0647-8
49. Brix N, Tiefenthaller A, Anders H, Belka C, Lauber K. Abscopal, immunological effects of radiotherapy: Narrowing the gap between clinical and preclinical experiences. Immunol Rev. (2017) 280:249–79. doi: 10.1111/imr.12573
50. McLaughlin M, Patin EC, Pedersen M, Wilkins A, Dillon MT, Melcher AA, et al. Inflammatory microenvironment remodelling by tumour cells after radiotherapy. Nat Rev Cancer. (2020) 20:203–17. doi: 10.1038/s41568-020-0246-1
51. Zhu M, Yang M, Zhang J, Yin Y, Fan X, Zhang Y, et al. Immunogenic cell death induction by ionizing radiation. Front Immunol. (2021) 12:705361. doi: 10.3389/fimmu.2021.705361
52. Hsieh RC, Krishnan S, Wu RC, Boda AR, Liu A, Winkler M, et al. ATR-mediated CD47 and PD-L1 up-regulation restricts radiotherapy-induced immune priming and abscopal responses in colorectal cancer. Sci Immunol. (2022) 7:eabl9330. doi: 10.1126/sciimmunol.abl9330
53. Oei AL, Korangath P, Mulka K, Helenius M, Coulter JB, Stewart J, et al. Enhancing the abscopal effect of radiation and immune checkpoint inhibitor therapies with magnetic nanoparticle hyperthermia in a model of metastatic breast cancer. Int J Hyperthermia. (2019) 36:47–63. doi: 10.1080/02656736.2019.1685686
54. Wang H, Li X, Xi X, Hu B, Zhao L, Liao Y, et al. Effects of magnetic induction hyperthermia and radiotherapy alone or combined on a murine 4T1 metastatic breast cancer model. Int J Hyperthermia. (2011) 27:563–72. doi: 10.3109/02656736.2011.583618
55. Chi MS, Tien DC, Chi KH. Inhomogeneously distributed ferroptosis with a high peak-to-valley ratio may improve the antitumor immune response. Front Oncol. (2023) 13:1178681. doi: 10.3389/fonc.2023.1178681
56. Podolska MJ, Shan X, Janko C, Boukherroub R, Gaipl US, Szunerits S, et al. Graphene-induced hyperthermia (GIHT) combined with radiotherapy fosters immunogenic cell death. Front Oncol. (2021) 11:664615. doi: 10.3389/fonc.2021.664615
57. Ngwa W, Irabor OC, Schoenfeld JD, Hesser J, Demaria S, Formenti SC. Using immunotherapy to boost the abscopal effect. Nat Rev Cancer. (2018) 18:313–22. doi: 10.1038/nrc.2018.6
58. Rodriguez-Ruiz ME, Vanpouille-Box C, Melero I, Formenti SC, Demaria S. Immunological mechanisms responsible for radiation-induced abscopal effect. Trends Immunol. (2018) 39:644–55. doi: 10.1016/j.it.2018.06.001
59. Chi MS, Mehta MP, Yang KL, Lai HC, Lin YC, Ko HL, et al. Putative abscopal effect in three patients treated by combined radiotherapy and modulated electrohyperthermia. Front Oncol. (2020) 10:254. doi: 10.3389/fonc.2020.00254
60. Ademaj A, Veltsista DP, Ghadjar P, Marder D, Oberacker E, Ott OJ, et al. Clinical evidence for thermometric parameters to guide hyperthermia treatment. Cancers (Basel). (2022) 14(3):625. doi: 10.3390/cancers14030625
61. Ahmed K, Tabuchi Y, Kondo T. Hyperthermia: an effective strategy to induce apoptosis in cancer cells. Apoptosis. (2015) 20:1411–9. doi: 10.1007/s10495-015-1168-3
62. Ahmed K, Zaidi SF, Mati Ur R, Rehman R, Kondo T. Hyperthermia and protein homeostasis: Cytoprotection and cell death. J Therm Biol. (2020) 91:102615. doi: 10.1016/j.jtherbio.2020.102615
63. Roti Roti JL. Cellular responses to hyperthermia (40-46 degrees C): cell killing and molecular events. Int J Hyperthermia. (2008) 24:3–15. doi: 10.1080/02656730701769841
64. Hu C, Yang J, Qi Z, Wu H, Wang B, Zou F, et al. Heat shock proteins: Biological functions, pathological roles, and therapeutic opportunities. MedComm (2020). (2022) 3:e161. doi: 10.1002/mco2.161
65. Tabuchi Y, Kondo T. Targeting heat shock transcription factor 1 for novel hyperthermia therapy (review). Int J Mol Med. (2013) 32:3–8. doi: 10.3892/ijmm.2013.1367
66. Ren X, Li T, Zhang W, Yang X. Targeting heat-shock protein 90 in cancer: an update on combination therapy. Cells. (2022) 11(16):2556. doi: 10.3390/cells11162556
67. Chatterjee S, Burns TF. Targeting heat shock proteins in cancer: A promising therapeutic approach. Int J Mol Sci. (2017) 18(9):1978. doi: 10.3390/ijms18091978
68. Horsman MR, Overgaard J. Hyperthermia: a potent enhancer of radiotherapy. Clin Oncol (R Coll Radiol). (2007) 19:418–26. doi: 10.1016/j.clon.2007.03.015
69. Scutigliani EM, Liang Y M, Rodermond H, Mei X, Korver MP, et al. Evaluation of the heat shock protein 90 inhibitor ganetespib as a sensitizer to hyperthermia-based cancer treatments. Cancers (Basel). (2022) 14(21):5250. doi: 10.3390/cancers14215250
70. Horsman MR. Angiogenesis and vascular targeting: relevance for hyperthermia. Int J Hyperthermia. (2008) 24:57–65. doi: 10.1080/02656730701829710
71. Cui Q, Wang JQ, Assaraf YG, Ren L, Gupta P, Wei L, et al. Modulating ROS to overcome multidrug resistance in cancer. Drug Resist Updat. (2018) 41:1–25. doi: 10.1016/j.drup.2018.11.001
72. Montoro A, Obrador E, Mistry D, Forte GI, Bravatà V, Minafra L, et al. Radioprotectors, radiomitigators, and radiosensitizers. In: Baatout S, editor. Radiobiology textbook. Springer International Publishing, Cham (2023). p. 571–628.
73. Kaur P, Aliru ML, Chadha AS, Asea A, Krishnan S. Hyperthermia using nanoparticles–Promises and pitfalls. Int J Hyperthermia. (2016) 32:76–88. doi: 10.3109/02656736.2015.1120889
74. Datta NR, Krishnan S, Speiser DE, Neufeld E, Kuster N, Bodis S, et al. Magnetic nanoparticle-induced hyperthermia with appropriate payloads: Paul Ehrlich's "magic (nano)bullet" for cancer theranostics? Cancer Treat Rev. (2016) 50:217–27. doi: 10.1016/j.ctrv.2016.09.016
75. Mahmoudi K, Bouras A, Bozec D, Ivkov R, Hadjipanayis C. Magnetic hyperthermia therapy for the treatment of glioblastoma: a review of the therapy's history, efficacy and application in humans. Int J Hyperthermia. (2018) 34:1316–28. doi: 10.1080/02656736.2018.1430867
Keywords: hyperthermia, radiotherapy, cancer biology, hypoxia, DNA-repair, tumor microenvironment, immunotherapy, thermotolerance
Citation: Righini MF, Durham A and Tsoutsou PG (2024) Hyperthermia and radiotherapy: physiological basis for a synergistic effect. Front. Oncol. 14:1428065. doi: 10.3389/fonc.2024.1428065
Received: 05 May 2024; Accepted: 17 July 2024;
Published: 06 August 2024.
Edited by:
Ira Ida Skvortsova, Innsbruck Medical University, AustriaReviewed by:
Mau-Shin Chi, Shin Kong Wu Ho-Su Memorial Hospital, TaiwanWenzhi Tu, Shanghai Jiao Tong University, China
Copyright © 2024 Righini, Durham and Tsoutsou. This is an open-access article distributed under the terms of the Creative Commons Attribution License (CC BY). The use, distribution or reproduction in other forums is permitted, provided the original author(s) and the copyright owner(s) are credited and that the original publication in this journal is cited, in accordance with accepted academic practice. No use, distribution or reproduction is permitted which does not comply with these terms.
*Correspondence: Pelagia G. Tsoutsou, Pelagia.Tsoutsou@hug.ch
†These authors share senior authorship