- 1Department of Pharmacy, The Affiliated Cancer Hospital of Nanjing Medical University, Jiangsu Cancer Hospital, Jiangsu Institute of Cancer Research, Nanjing, China
- 2Jiangsu Breast Disease Center, The First Affiliated Hospital with Nanjing Medical University, Nanjing, China
The significance of matrix stiffness in cancer development has been investigated in recent years. The gradual elastic force the extracellular matrix imparts to cells, known as matrix stiffness, is one of the most important types of mechanical stimulation. Increased matrix stiffness alters the biological activity of cells, which promotes the growth of numerous malignancies, including breast cancer. Comprehensive studies have demonstrated that increasing matrix stiffness activates molecular signaling pathways that are closely linked to breast cancer progression. There are many articles exploring the relationship between mechanism hardness and breast cancer, so we wanted to provide a systematic summary of recent research advances. In this review, we briefly introduce the mechanism of matrix stiffness in breast cancer, elaborate on the effect of extracellular matrix stiffness on breast cancer biological behavior and signaling pathways, and finally, we will talk about breast cancer treatment that focuses on matrix stiffness.
1 Introduction
Breast cancer is one of the most common malignancies among women. One of the most obvious signs of breast cancer is tissue hardening, which can be detected by palpating malignant nodules (1, 2). The stiffness of normal healthy breast tissue is approximately 0.2 kPa, while that of breast cancer tissue is over 4 kPa (3). In addition to the increased stiffness of breast cancer tissues, adjacent matrix tissues are also affected. A study in an animal model demonstrated that when breast tissue become invasive, the adjacent matrix tissue is also much stiffer than the distant normal tissue (1). These phenomena arise from abnormal changes in the structure and composition of the tumor extracellular matrix (ECM) in breast cancer (2).
The ECM is an intricate network of three-dimensional macromolecules consisting mainly of collagen, non-collagen, elastin, proteoglycans and glycosaminoglycans (4); it offers appropriate chemical signals and mechanical stimulation to regulate cell shape, metabolism, function, migration, proliferation, and differentiation (4, 5). Mechanical stimulation involves compression, matrix stiffness, and hydrodynamics (6). Moreover, matrix stiffness, also known as rigidity or modulus of elasticity, is defined as the resistance of a material to deformation by a force applied at a very slow rate (quasi-static) (7). Stiffness is an intrinsic material property of tissues, and increased tissue hardness is the most obvious and recognized mechanical abnormality in tumors, including breast cancer (8).
With the application of atomic force microscopy (AFM) and the continuous refinement of 3D culture techniques, the study of matrix stiffness has become more feasible (9, 10). Hydrogels are good candidates in the study of ECM physical properties using 3D modeling (11). Various types of hydrogels have been used in the study of matrix stiffness, including polyacrylamide hydrogels, hyaluronic acid hydrogels, collagen hydrogels, gelatin hydrogels, etc. (12). Based on the fact that the matrix stiffness is a constant state of transformation with the dynamics of the ECM, more advanced stimuli-responsive hydrogels were synthesized (13). Stimuli-responsive hydrogels can adjust their stiffness in response to external physical or chemical stimuli to better mimic the in vivo environment in matrix stiffness studies (11, 13). In addition, to better approach the treatment of breast cancer from the aspect of stromal stiffness, various 3D experimental models have been developed, mainly including cancer cell lines, 3D spheroids, in vivo patient-derived xenografts (PDX), and in vitro patient-derived organoids (PDO) (14–17). For example, PDOs have been established from breast cancer, and this model can be used to predict drug response in cancer patients, which in turn informs the patient’s treatment regimen (17). 3D spheroids also have extensive use in exploring the role of matrix stiffness in breast cancer invasion (18).
The formation of tumors mainly depends on the balance between increased matrix stiffness and matrix degradation (19). Collagen accumulation and pathological collagen cross-linking are the major causes of increased ECM stiffness in breast cancer (7). Analysis of human breast tissue samples has revealed that the transition from non-malignant tissue to invasive ductal carcinoma (IDC) corresponds to significant collagen deposition, resulting in stromal stiffening (20). In addition, computational analysis of mammographic images has shown that dense breast tissue has a stiffer matrix, contains more linearized and bound collagen, and is associated with a higher risk of breast cancer (21, 22). Degradation of breast cancer matrix is mainly dependent on the regulation of lysyl oxidase (LOX), lysyl oxidase like-1-4 (LOXL 1-4), and matrix metalloproteinases (MMPs), which are extracellular matrix remodeling enzymes (23, 24). LOX promotes the cross-linking of elastin and collagen in the ECM and prevents collagen degradation, which promotes breast cancer progression (25). Furthermore, MMPs remodel the ECM by degrading ECM proteins, which in turn promote breast cancer metastasis (26). Thus, an excessively stiff matrix or excessive matrix degradation can promote the progression of breast cancer.
Matrix stiffness is closely related to malignant breast cancer phenotypes, including proliferation, metastasis, invasion, and drug resistance. There are many articles exploring the relationship between matrix stiffness and breast cancer, so we want to provide a systematic summary of recent research advances. In this review, we systematically introduce the major causes of breast stiffening and summarize the role of matrix stiffness in breast cancer initiation and progression and its potential applications. This may provide clues for studying matrix stiffness in breast cancer and exploring its clinical applications in breast cancer treatment.
2 Formation of matrix stiffness in breast cancer
The stiffness of cancer tissue is mainly determined by cancer and stromal cells (27). Matrix deposition and cross-linking are the two major causes of breast cancer stiffening (28, 29) (Figure 1). Cancer cells and stromal cells are jointly involved in matrix deposition and cross-linking and determine matrix stiffness (27).
2.1 Matrix deposition
Among all stromal cells, cancer-associated fibroblasts (CAFs) are the most efficient in depositing and remodeling the ECM in the tumor microenvironment (30, 31). Stromal cells with high alpha-smooth muscle actin (aSMA) expression are known as cancer-associated fibroblasts (CAFs) (32). Through Notch signaling, interaction between cancer cells and fibroblasts can advance the CAF phenotype in breast cancer (30). Cancer cells can also promote the transformation of fibroblasts into CAFs by secreting TGFβ, which in turn further promotes tumor progression through ECM remodeling (33, 34). During breast cancer progression, up to 80% of stromal cells acquire the CAF phenotype (35). CAFs synthesize and secrete collagen procollagen molecules, which are processed and arranged to form collagen fibers. As fibrillar collagen (both type I and type III) is progressively deposited in the ECM, the normal ECM gradually transforms into dense fibrous tumor stroma (36, 37). Except for CAFs, other stromal cells play an important role in causing increased matrix stiffness, including macrophages. Macrophages secrete a variety of soluble factors that induce ECM deposition, thereby stiffening the extracellular matrix (20). In addition, during breast cancer progression, breast cancer epithelial cells gradually lose epithelial markers to acquire mesenchymal markers and mesenchymal cell-like properties through epithelial-mesenchymal transition (EMT), which in turn exerts a function like that of CAFs, synthesizing and secreting collagen, leading to stromal deposition promoting an increase in stromal stiffness (38). In summary, both stromal cells and breast cancer cells undergoing EMT can promote increased matrix stiffness through matrix deposition.
2.2 Matrix cross-linking
CAFs and cancer cells highly express LOX/LOXs (39), which are amine oxidases that mainly regulate covalent cross-linking between ECM collagen and elastin (40, 41). In breast cancer, LOX and collagen influence the architecture of the ECM and create a favorable microenvironment for tumor development and progression (42).
LOX was reported to promote fibrosis of breast tissue through collagen cross-linking, leading to increased matrix stiffness in breast tumors (43, 44). Increased matrix stiffness can induce the assembly of focal adhesions and up-regulate GFR-dependent PI3K signaling, ultimately leading to tumor progression (1). Furthermore, breast cancer cells and CAFs can synthesize and secrete proteolytically active MMPs (45), which can degrade almost all proteins in the ECM when metal ions are used as cofactors (46). However, when collagen is cross-linked, MMPs are unable to break down the collagen, thus increasing matrix stiffness (47). The phenomenon of collagen cross-linking leading to increased matrix stiffness in cancer cells is widespread. For instance, when highly expressed in pancreatic cancer cells, tissue transglutaminase (TG2) crosslinks proteins to stiffen the pancreatic tumor tissue (48). However, TG2 promotion of breast tumor matrix cross-linking has not been elucidated. In conclusion, matrix cross-linking is essential for enhancing the stiffness of cancer tissues (29).
3 Initiation and progression of breast cancer regulated by matrix stiffness
Increased matrix stiffness leads to breast malignancy and contributes to the malignant phenotypes of breast cancer by promoting breast cancer proliferation, metastasis, invasion, immune evasion, stemness, and drug resistance through the regulation of breast cancer and stromal cells (Figure 2). With the development of 3d culture technology, it has become possible to simulate different matrix stiffnesses using hydrogels, making it possible to study in vitro how matrix stiffness affects cell signaling pathways. Matrix stiffness affects tumor and non-tumor cells through multiple molecular signaling pathways in breast tumors that promote tumor progression (Table 1).
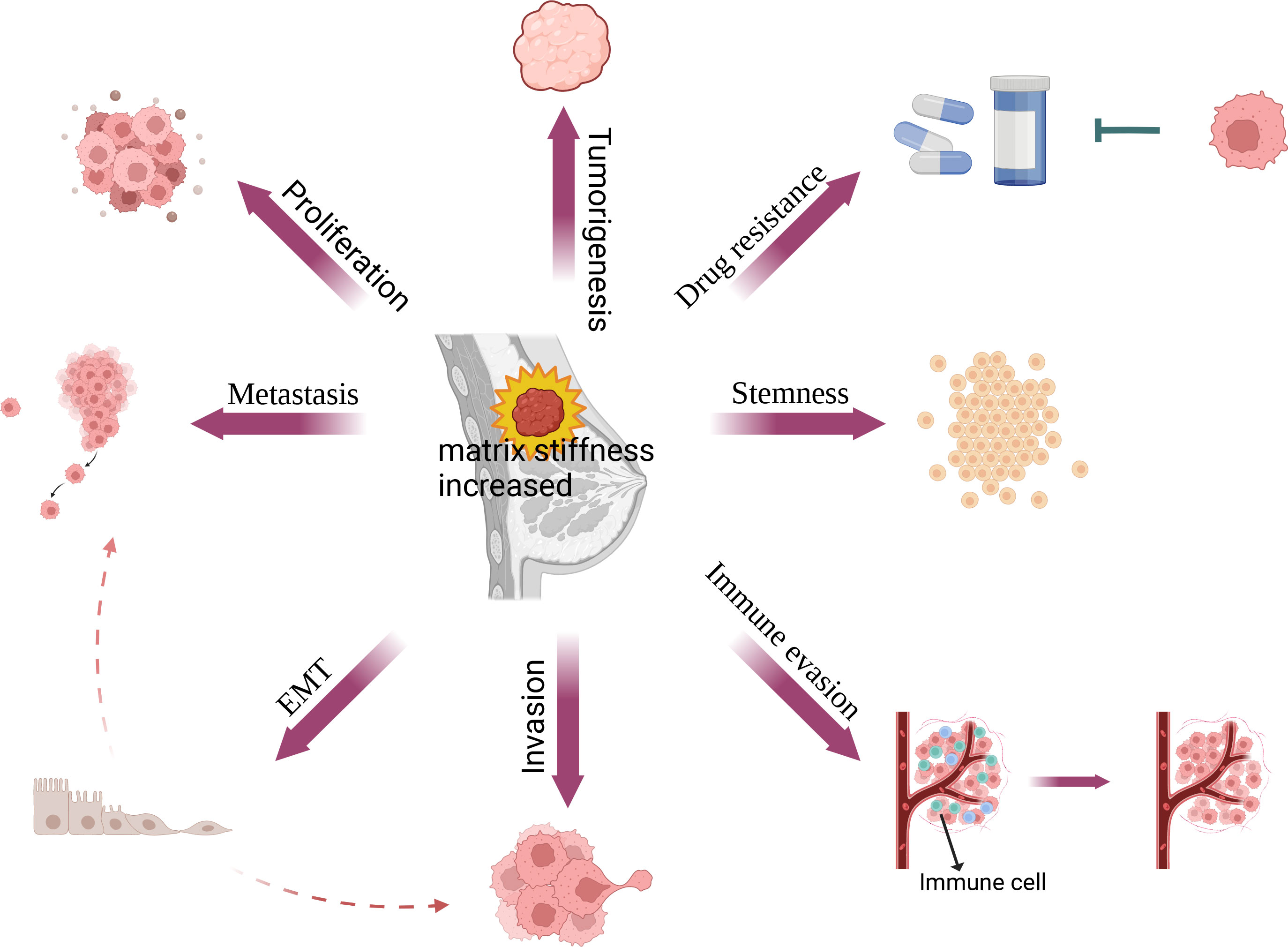
Figure 2 Relationship between matrix stiffness and breast cancer. In breast cancer, matrix stiffness can affect its proliferation, EMT, metastasis, invasion, immune evasion, stemness and drug resistance, thus further promoting fibrosis and the progression of breast cancer.
3.1 Tumorigenesis of breast cancer promoted by matrix stiffness
Mammary density (MD) is associated with an overall increased lifetime risk of malignancy. Increased mammary density is primarily caused by the deposition of fibrillar collagen (68). It has been shown to result in an increase in stromal stiffness which disrupts the physiologic breast morphogenesis (69, 70). Even a small increase in matrix stiffness results in activation of Rho GTPase and induces collagen matrix contraction to disrupt tissue structure. Rho GTPase also activates the ROCK pathway, which can lead to malignant changes in the breast (49). Collagen cross-linking leads to matrix stiffening promotes integrin aggregation, enhances PI3K activity, and induces oncogene-initiated invasion of epithelial cells (1).
Mammary epithelial cells in cultured soft matrix can grow into normal epithelial tubules; however, in hard matrix, they exhibit an abnormal tumor-like morphology (71). Study of epigenomic changes show that increased stromal stiffness leads to increased nuclear ruffling and lamellipodia-associated chromatin, ultimately inducing a tumor phenotype (72). In a mouse experiment, it was also found that increased matrix stiffness increased mammary tumorigenesis by about three times (73). So, increased matrix stiffness is inextricably linked to breast cancer initiation.
3.2 Proliferation of breast cancer cells regulated by matrix stiffness
The uncontrolled proliferation of cancer cells is one of the dominant features of cancer (74). Mesenchymal stem cells (MSC) in a stiff matrix can differentiate into cancer-associated fibroblasts (CAF) with increased expression of the yes-associated protein (YAP) (50). YAP, an important regulatory molecule in the Hippo pathway, is phosphorylated to enter the nucleus to transcribe anti-apoptotic and pro-proliferative genes, thereby regulating cell proliferation and apoptosis and controlling organ size (75–77). In the Wnt pathway, nuclear YAP can promote cell proliferation by up-regulating β-catenin expression (51, 52). In addition, an increase in mammary gland density is often accompanied by an increase in matrix stiffness. Regions with high breast density have increased stromal collagen and epithelial cell contents (78). When NMuMG mammary epithelial cells are cultured on a hard substrate, Wnt3a increases the integrin-linked kinases (ILK)-mediated Frizzled-1 expression and thus promotes epithelial cell proliferation through the integrin signaling pathway (53). Provenzano et al. simulated increased matrix stiffness by increasing the matrix collagen density. They found that matrix stiffness promoted the proliferation of breast cancer cells through FAK-Rho and FAK-Ras-ERK signaling networks (54). Similar conclusions have been reached in animal experiments. Injecting breast cancer cells cultured in a stiffer matrix into mice can form larger tumors (79). In conclusion, matrix stiffness drives breast cancer cell proliferation.
3.3 Invasion and metastasis of breast cancer cells regulated by matrix stiffness
Changes in matrix stiffness significantly affect the cytoskeletal structure and ability of breast cancer cells to invade and metastasize. By analyzing PAM50 tumor subtypes, Adam et al. found that compared to the less aggressive luminal A and normal-like subtypes, the more aggressive subtypes such as basal, human epidermal growth factor receptor 2 (HER2), and luminal B, had stiffer matrix and poorer overall survival. They suggested that increased matrix stiffness enhances breast cancer invasion (79). Mechanistically, integrins play important roles in this process (Figure 3). Integrin receptors activate insulin receptors (IR) by forming β1 and β3 integrins and IR complexes. IR activates the PI3K/AKT/mTORC1 signaling axis to promote breast cancer cell metastasis (55). Moreover, matrix stiffness can directly activate integrin β1 and focal adhesion kinase (FAK), which accelerates focal adhesion (FA) maturation and induces downstream cascades of intracellular signals in the RhoA/ROCK pathway. ROCK isoforms differentially regulate the RhoA/ROCK1/p-MLC and RhoA/ROCK2/p-cofilin pathways in a coordinated fashion to modulate breast cancer cell motility in a substrate stiffness-dependent manner through integrin β1-activated FAK signaling (58). Moreover, with the activation of EGFR and PLCγ1, the expression of Mena, a protein associated with metastasis in breast cancer, is up-regulated in a stiff matrix. High Mena expression further increases matrix stiffness by depositing fibronectin via α5 integrin (56, 57). In conclusion, integrins are important in promoting the invasive metastasis of breast cancer cells.
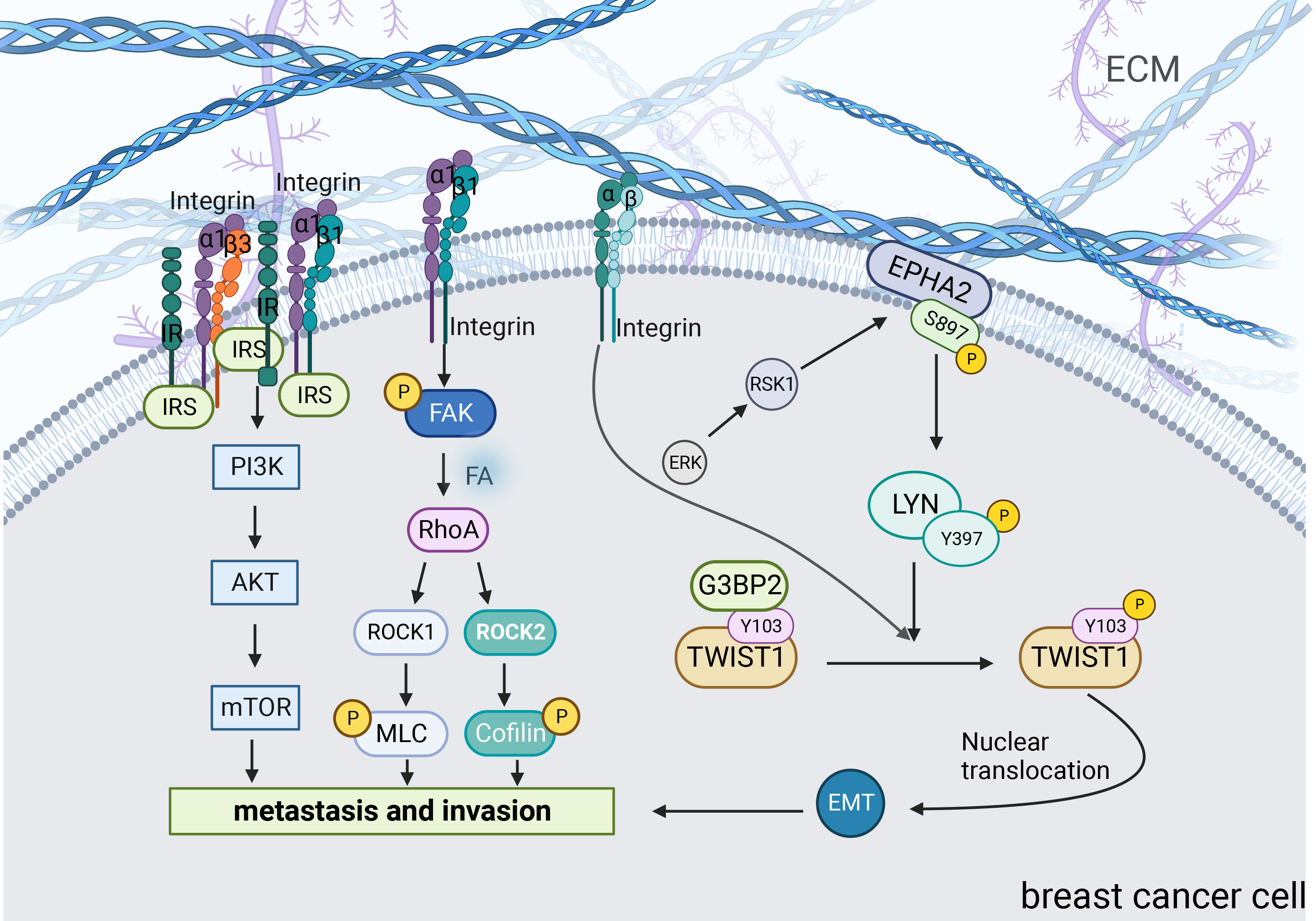
Figure 3 Signaling pathways associated with increased matrix stiffness leading to breast cancer invasion and metastasis. Matrix stiffness activates many mechanoreactive signaling pathways in cells through transmembrane proteins including integrins. Pathways such as PI3K, ROCK and TWIST1 play a major role in this transduction. Central players in these signaling pathways can connect with other molecules and ultimately translate changes in the ECM into relevant biological changes.
One of the key processes that promotes the progression of metastasis in cancer cells is the epithelial-mesenchymal transition (EMT). EMT, the process by which epithelial cells lose polarity, intercellular adhesion, acquire migratory, and invasive properties to become mesenchymal cells, is thought to play a key role in initiating the metastatic cascade response. Thus, EMT allows cancer cells to leave the primary tumor, invade the surrounding ECM, enter the blood and lymphatic vessels, and spread to all body parts (80). When matrix stiffness increasing, cells in the matrix gradually develop an EMT phenotype, indicating that they are more likely to undergo invasive and metastatic spreading (81, 82).
TWIST1 is a basic helix-loop-helix (bHLH) transcription factor that promotes tumor metastasis by initiating EMT and degrading ECT (59, 60). Furthermore, the increase in matrix stiffness causes TWIST1 to move toward the nucleus, directly affecting the EMT program. G3BP2 is a TWIST1 binding protein and tyrosine residue Y103 is present in its binding sequence. In a soft matrix, there is a strong tendency for the two to interact; however, in a stiffened matrix, TWIST1 dissociates from G3BP2 and is transferred to the nucleus (81). Fattet et al. have shown that increased matrix stiffness activates extracellular signal-regulated kinases (ERK) and ribosomal S6 kinase1 (RSK1). Activated ERK/RSK1 phosphorylates the ephrin Receptor EPHA2 at serine 897 (S897). Moreover, phosphorylated EPHA2 activates LYN Kinase to form an EPHA2/LYN complex. This complex phosphorylates Y103 in the TWIST1-G3BP2 binding sequence, leading to TWIST1-G3BP2 dissociation (83). Additionally, it has been demonstrated that during matrix stiffness, activated integrins phosphorylate Y103 through tyrosine kinases, which eventually prevents TWIST1 from binding to G3BP2 (81). Additionally, a study found a positive correlation between TWIST1 expression and tumor stiffness in patients with breast cancer (84). Barriga et al. found that high tissue stiffness promoted EMT triggering neural crest migration, and this study in turn confirmed in vivo that higher matrix stiffness increased the propensity of cells to undergo EMT, leading to distant metastasis (85). Generally, increased matrix stiffness promotes breast cancer metastasis by activating the EMT through a mechanical conduction pathway.
In addition, there is a phenomenon in the process of cancer recurrence and metastasis, which is that breast cancer recurrence and metastasis are usually detected in tissues that are softer than normal breast or primary breast tumors (such as bone marrow, liver, brain, and lung) (86). Therefore, the soft microenvironment can promote the survival of disseminated breast cancer cells at the secondary site. When breast cancer cells were cultured on the soft matrix mimicking the site of metastasis, they were found to remain dormant for a long time to escape the killing effects of chemotherapy drugs. Soft matrix can also induce chemical resistance in breast cancer by increasing autophagy, making metastatic breast cancer more difficult to treat (87).
3.4 Stemness of breast cancer cells regulated by matrix stiffness
Cancer stem cells (CSC) are a small subpopulation of cancer cells that maintain their self-renewal and undifferentiated abilities. Breast cancer stem cells (BCSC) can self-renew, differentiate, drive tumor progression, and mediate drug resistance and metastasis (88). Pang et al. investigated the relationship between matrix stiffness and BCSC by detecting CSC markers CD44, Nanog, and CD49f. They found that the expression of all these markers increased when the matrix stiffness increased. By comparing the expression of CD44 at different matrix stiffness values, they found that BCSCs were preferentially located in a stiff microenvironment (61).
BCSCs were mainly regulated by ILK. In the presence of increased matrix stiffness, ILK regulates BCSC development via the PI3K/Akt pathway and promotes angiogenesis in tumor cells, ultimately contributing to tumor metastatic spread (61). A recent study found that cells cultured on hard polyacrylamide hydrogels (9 kPa) had a significantly higher proportion of BCSCs compared to cells cultured on soft polyacrylamide hydrogels (0.5 kPa; matching the compliance of normal mammary glands). Exploration of the mechanism revealed that when matrix stiffness was increased, TAZ dissociated from NANOG, promoting the transcription of SOX2 and OCT4, which in turn increased the proportion of BCSCs in the breast cancer and promoted the stemness phenotype of breast cancer (62). In another study, by using three different hydrogels, Matrigel, collagen I, and fibrinogen gels, to simulate three different matrix compositions, collagen, laminin, and fibronectin, respectively, it was found that the increased matrix stiffness due to different matrix compositions had different effects on the stemness of breast cancer (89). Yan Li et al. cultured breast cancer cells using different stiffness of polyacrylamide hydrogels and found that increased matrix stiffness promotes YAP nuclear translocation, which in turn promotes BCSCs maintenance (63). Matrix stiffness may be important for the induction and maintenance of CSC; however, this requires further investigation.
3.5 Drug resistance of breast cancer cells regulated by matrix stiffness
Drug resistance is one of the most important factors affecting breast cancer treatment outcomes (90). Improving the sensitivity of breast cancer cells to chemotherapeutic agents is essential to improve the survival rate of patients with breast cancer. In addition, the ability to achieve effective drug concentrations at the tumor site is also essential for the treatment of cancer. Most chemotherapeutic agents are dose-dependent, and chemotherapeutic agents need to pass through the tumor vasculature system, cross the vessel wall to enter, and pass through the interstitial space of the tumor to reach the cancer cells to exert their therapeutic effects. However, when stromal stiffness increases, the extravascular hydrostatic pressure, or interstitial pressure (IFP), increases within the tumor, resulting in inhibited drug extravasation. On the other hand, increased stromal stiffness leads to vascular compression, resulting in inadequate perfusion within the tumor, further reducing drug concentration. More unfortunately, when stromal stiffness is increased, the dense ECM further impedes the effective diffusion of chemotherapeutic agents, ultimately making it difficult to achieve effective concentrations and reducing the efficacy of chemotherapeutic agents (91–93).
The responsiveness of primary breast cancer cells to chemotherapeutic agents is altered after they are removed from the host microenvironment and transferred to hard-surface cultures in vitro. The activities of PTX and DOX were strongly correlated with matrix hardness. Substrates that are too hard can reduce the activities of PTX and DOX, leading to drug resistance (94). In addition, the activity of targeted drugs for breast cancer treatment can be influenced by stromal stiffness. Lapatinib is an orally administered small-molecule epidermal growth factor tyrosine kinase inhibitor. It is primarily used to treat HER2(human epidermal growth factor receptor-2)-amplified breast cancer. Furthermore, the ratio of HER2 phosphorylation decrease with increasing matrix stiffness and was negatively correlated with lapatinib insensitivity (95).
Sorafenib is a small-molecule tyrosine kinase inhibitor with anti-angiogenic activity that has been used to treat hepatocellular and renal cancers (96). Hepatocellular cancer cells on stiff substrates show resistance to sorafenib compared to those on soft substrates (97). The same phenomenon has been observed in breast cancer cells (98). Breast cancer cells cultured on harder substrates were more resistant to sorafenib (99).
Moreover, the EMT affects the sensitivity of breast cancer cells to chemotherapy. Notably, increased matrix stiffness promotes the nuclear translocation of YAP, triggering EMT and increasing drug resistance. However, only the MDA-MB-231 cell line showed drug resistance with increased simulated matrix stiffness during the experiment (64). This suggests that the effect of matrix stiffness on drug resistance is related to the cell line. Additionally, matrix stiffness can regulate YAP’s translocation, dephosphorylation, and transcriptional activity by increasing ILK expression, ultimately leading to increased drug resistance in breast cancer cells (8). In summary, targeting matrix stiffness is a prospective strategy for improving the efficacy of chemotherapy.
In addition to chemotherapy, radiotherapy is also an important treatment for breast cancer. One study showed that low doses of radiation had no significant effect on tumor cell migration when matrix stiffness was increased, but when high doses of radiation were changed, tumor cell adhesion increased and migration rate decreased significantly. On soft substrates, low doses of radiation can reduce the migration rate of tumor cells. These results indicate that the radiosensitivity of tumors on hard substrates is dose dependent (100). But the results are not widely accepted. Rieken et al. suggested that radiation promotes tumor migration by inducing integrin overexpression (101). In conclusion, the mechanism of the influence of matrix stiffness on radiosensitivity is still unclear, and some conclusions are still controversial, which may be closely related to radiation dose, radiation time and cell types (93).
In addition, the targeted therapies of breast cancer could also be affected by matrix stiffness. Lapatinib is a targeted drug for the treatment of HER2-amplified breast cancer (102). Increased matrix stiffness leads to YAP overexpression, which in turn modulates the Hippo pathway and reduces the efficacy of lapatinib (95, 103). In conclusion, matrix stiffness has an impact on multiple treatments for breast cancer, including chemotherapy, radiotherapy and targeted therapy.
3.6 Immune evasion of breast cancer cells regulated by matrix stiffness
Immunotherapy is a novel modality for the treatment of breast cancer. However, breast cancer is considered a low-immune reactive cancer. The key to immunotherapy is the interaction between the programmed death-1 receptor (PD-1) and programmed death ligand 1 (PD-L1). Previous studies revealed a positive association between high PD-L1 expression and matrix stiffness. High PD-L1 expression in breast cancer is associated with poor prognosis (65, 66). On a physical level, when collagen crosslinks and matrix stiffness increases, T cells have difficulty penetrating the matrix and their ability to migrate in the matrix is greatly diminished, thus limiting the further role of T cells in the tumor (67). Therefore, reversing immune evasion in breast cancer remains a challenge.
4 Therapy for breast cancer by targeting matrix stiffness
As the study of matrix stiffness has intensified, new directions for breast cancer treatment have been provided. Matrix targeting in breast cancer can be broadly divided into two types:1) Reducing the source of matrix stiffness. 2) Blocking the effect of matrix stiffness on the downstream pathways (Table 2).
To reduce the source of matrix stiffness and collagen cross-linking, ECM enzymes, such as LOX/LOXLs, MMPs, and CAFs, can be used to directly block the excessive synthesis of certain ECM components. For example, 4-methylumbelliferone (MU) can significantly inhibit the synthesis and accumulation of hyaluronic acid (HA, a matrix component), which promotes tumor cell metastasis (110). β-Aminopropionitrile (BAPN) acts as a LOX inhibitor and suppresses breast cancer proliferation and metastasis by inhibiting collagen cross-linking (25, 104, 105). Tetrathiomolybdate (TM), a LOX inhibitor, belongs to a group of copper chelators that inhibit LOX activity by binding to and depleting copper. A phase IIa TM study is underway in breast cancer patients at an intermediate to high risk of recurrence (25).
Prinomastat is a selective oral matrix MMP -2, -9, -13 and -14 inhibitor. The drug has been shown to prevent angiogenesis and tumor development in a range of preclinical models, including those of colon, breast, lung, melanoma, and glioma (106). Growth factors, including TGF-β, PDGF, and VEGF, can also be used as targets to block the increase in matrix stiffness. Pirfenidone (PFD) is a potent TGF-β inhibitor approved for treating pulmonary and renal fibrosis (111). For example, Hamidreza et al. showed that PFD reduced breast cancer epithelial-mesenchymal transition and globule formation by targeting CAFs (107).
Downstream receptors of matrix stiffness, such as integrins, FAK, Rho GTPase, and AKT, can be used as therapeutic targets. Seon-Ok Lee et al. found that fomes fomentarius ethanol (FFE) could inhibit MDA-MB-231cells motility and growth, by reducing the expression of MMP-9 and phosphorylated Akt (108). Furthermore, the complex formation of HER2-Src-α6β4 integrin influences the targeted therapy with lapatinib. Cuiying Liu et al. explored how stiffness regulated the response of breast cancer cells to lapatinib. They found that, on the stiff substrate, the HER2 is difficult to combine with β4 integrin molecules, constructing fewer complexes of HER2-Src-α6β4 integrin. Consequently, free HER2 molecules were inhibited by lapatinib. In addition, as early as 2002, the concept of “biomechanopharmacology” was first proposed (109). The development of this field will provide new ideas for future treatments.
5 Conclusions and perspectives
The role of the ECM in tumorigenesis has been increasingly studied, and changes in matrix stiffness have also been considered as factors contributing to disease development. This review begins with an introduction to the mechanical microenvironment in breast cancer. We then elaborated on the effect of extracellular matrix stiffness on breast cancer’s biological behavior and signaling pathway. Finally, we discuss the transformation treatments for matrix stiffness in breast cancer.
In addition, several questions remain unanswered. Can matrix hardness be integrated into clinical research? Is there an interaction between the various mechanical stimuli? Can mechanical stimuli such as matrix stiffness be measured quantitatively? Are there signaling pathways other than those mentioned above? Research into the effects of matrix hardness on signaling pathways is only beginning, and the effects of matrix stiffness on biological pathways, such as transcription, post-transcriptional modification, translation, and post-translational modification, need to be further investigated. In addition, we also noted that antibody-drug conjugates (ADCs) are gradually becoming a novel treatment for breast cancer. However, studies on the aspect of ADCs related to matrix stiffness are still relatively scarce, and further studies are needed to explore the relationship between the two subsequently. Although studies on the effects of matrix stiffness on breast cancer are already underway, our understanding of the mechanisms involved is limited to the tip of the iceberg. We will be able to develop new therapeutic options through a better understanding of matrix stiffness. We believe that concerted efforts by researchers are required to address these questions.
Author contributions
RX: Writing – original draft. JW: Writing – review & editing. QD: Writing – review & editing. PY: Writing – original draft.
Funding
The author(s) declare that no financial support was received for the research, authorship, and/or publication of this article.
Acknowledgments
All authors would like to thank Biorender (www.biorender.com) for Figures editing.
Conflict of interest
The authors declare that the research was conducted in the absence of any commercial or financial relationships that could be construed as a potential conflict of interest.
Publisher’s note
All claims expressed in this article are solely those of the authors and do not necessarily represent those of their affiliated organizations, or those of the publisher, the editors and the reviewers. Any product that may be evaluated in this article, or claim that may be made by its manufacturer, is not guaranteed or endorsed by the publisher.
References
1. Levental KR, Yu H, Kass L, Lakins JN, Egeblad M, Erler JT, et al. Matrix crosslinking forces tumor progression by enhancing integrin signaling. Cell (2009) 139(5):891–906. doi: 10.1016/j.cell.2009.10.027
2. Lu P, Weaver VM, Werb Z. The extracellular matrix: a dynamic niche in cancer progression. J Cell Biol (2012) 196(4):395–406. doi: 10.1083/jcb.201102147
3. Paszek MJ, Zahir N, Johnson KR, Lakins JN, Rozenberg GI, Gefen A, et al. Tensional homeostasis and the malignant phenotype. Cancer Cell (2005) 8(3):241–54. doi: 10.1016/j.ccr.2005.08.010
4. Theocharis AD, Skandalis SS, Gialeli C, Karamanos NK. Extracellular matrix structure. Adv Drug Deliv Rev (2016) 97:4–27. doi: 10.1016/j.addr.2015.11.001
5. Piperigkou Z, Götte M, Theocharis AD, Karamanos NK. Insights into the key roles of epigenetics in matrix macromolecules-associated wound healing. Adv Drug Deliv Rev (2018) 129:16–36. doi: 10.1016/j.addr.2017.10.008
6. Avvisato CL, Yang X, Shah S, Hoxter B, Li W, Gaynor R, et al. Mechanical force modulates global gene expression and beta-catenin signaling in colon cancer cells. J Cell Sci (2007) 120(Pt 15):2672–82. doi: 10.1242/jcs.03476
7. Piersma B, Hayward MK, Weaver VM. Fibrosis and cancer: A strained relationship. Biochim Biophys Acta Rev Canc (2020) 1873(2):188356. doi: 10.1016/j.bbcan.2020.188356
8. Qin X, Lv X, Li P, Yang R, Xia Q, Chen Y, et al. Matrix stiffness modulates ILK-mediated YAP activation to control the drug resistance of breast cancer cells. Biochim Biophys Acta Mol Bas Dis (2020) 1866(3):165625. doi: 10.1016/j.bbadis.2019.165625
9. Xia F, Youcef-Toumi K. Review: Advanced Atomic Force Microscopy Modes for Biomedical Research. Biosens (Basel) (2022) 12(12):1116. doi: 10.3390/bios12121116
10. Shamir ER, Ewald AJ. Three-dimensional organotypic culture: experimental models of mammalian biology and disease. Nat Rev Mol Cell Biol (2014) 15(10):647–64. doi: 10.1038/nrm3873
11. Ting MS, Travas-Sejdic J, Malmström J. Modulation of hydrogel stiffness by external stimuli: soft materials for mechanotransduction studies. J Mater Chem B (2021) 9(37):7578–96. doi: 10.1039/d1tb01415c
12. Merivaara A, Koivunotko E, Manninen K, Kaseva T, Monola J, Salli E, et al. Stiffness-Controlled Hydrogels for 3D Cell Culture Models. Polymers (Basel) (2022) 14(24):5530. doi: 10.3390/polym14245530
13. Sievers J, Mahajan V, Welzel PB, Werner C, Taubenberger A. Precision Hydrogels for the Study of Cancer Cell Mechanobiology. Adv Healthc Mater (2023) 12(14):e2202514. doi: 10.1002/adhm.202202514
14. Hidalgo M, Amant F, Biankin AV, Budinská E, Byrne AT, Caldas C, et al. Patient-derived xenograft models: an emerging platform for translational cancer research. Cancer Discov (2014) 4(9):998–1013. doi: 10.1158/2159-8290.CD-14-0001
15. Sachs N, Clevers H. Organoid cultures for the analysis of cancer phenotypes. Curr Opin Genet Dev (2014) 24:68–73. doi: 10.1016/j.gde.2013.11.012
16. Ben-David U, Beroukhim R, Golub TR. Genomic evolution of cancer models: perils and opportunities. Nat Rev Canc (2019) 19(2):97–109. doi: 10.1038/s41568-018-0095-3
17. Yalcin GD, Danisik N, Baygin RC, Acar A. Systems Biology and Experimental Model Systems of Cancer. J Pers Med (2020) 10(4):180. doi: 10.3390/jpm10040180
18. Micalet A, Moeendarbary E, Cheema U. 3D In Vitro Models for Investigating the Role of Stiffness in Cancer Invasion. ACS Biomater Sci Eng (2023) 9(7):3729–41. doi: 10.1021/acsbiomaterials.0c01530
19. van der Slot-Verhoeven AJ, van Dura EA, Attema J, Blauw B, Degroot J, Huizinga TW, et al. The type of collagen cross-link determines the reversibility of experimental skin fibrosis. Biochim Biophys Acta (2005) 1740(1):60–7. doi: 10.1016/j.bbadis.2005.02.007
20. Acerbi I, Cassereau L, Dean I, Shi Q, Au A, Park C, et al. Human breast cancer invasion and aggression correlates with ECM stiffening and immune cell infiltration. Integr Biol (Camb) (2015) 7(10):1120–34. doi: 10.1039/c5ib00040h
21. Northey JJ, Barrett AS, Acerbi I, Hayward MK, Talamantes S, Dean IS, et al. Stiff stroma increases breast cancer risk by inducing the oncogene ZNF217. J Clin Invest (2020) 130(11):5721–37. doi: 10.1172/JCI129249
22. Boyd NF, Li Q, Melnichouk O, Huszti E, Martin LJ, Gunasekara A, et al. Evidence that breast tissue stiffness is associated with risk of breast cancer. PloS One (2014) 9(7):e100937. doi: 10.1371/journal.pone.0100937
23. Yip C, Foidart P, Noël A, Sounni NE. MT4-MMP: The GPI-Anchored Membrane-Type Matrix Metalloprotease with Multiple Functions in Diseases. Int J Mol Sci (2019) 20(2):354. doi: 10.3390/ijms20020354
24. Kirschmann DA, Seftor EA, Fong SF, Nieva DR, Sullivan CM, Edwards EM, et al. A molecular role for lysyl oxidase in breast cancer invasion. Cancer Res (2002) 62(15):4478–83.
25. Ferreira S, Saraiva N, Rijo P, Fernandes AS. LOXL2 Inhibitors and Breast Cancer Progression. Antioxidants (Basel) (2021) 10(2):312. doi: 10.3390/antiox10020312
26. Wang J, Boddupalli A, Koelbl J, Nam DH, Ge X, Bratlie KM, et al. Degradation and Remodeling of Epitaxially Grown Collagen Fibrils. Cell Mol Bioeng (2019) 12(1):69–84. doi: 10.1007/s12195-018-0547-6
27. Ishihara S, Haga H. Matrix Stiffness Contributes to Cancer Progression by Regulating Transcription Factors. Cancers (Basel) (2022) 14(4):1049. doi: 10.3390/cancers14041049
28. Zhang K, Grither WR, Van Hove S, Biswas H, Ponik SM, Eliceiri KW, et al. Mechanical signals regulate and activate SNAIL1 protein to control the fibrogenic response of cancer-associated fibroblasts. J Cell Sci (2016) 129(10):1989–2002. doi: 10.1242/jcs.180539
29. Patwardhan S, Mahadik P, Shetty O, Sen S. ECM stiffness-tuned exosomes drive breast cancer motility through thrombospondin-1. Biomaterials (2021) 279:121185. doi: 10.1016/j.biomaterials.2021.121185
30. Sahai E, Astsaturov I, Cukierman E, DeNardo DG, Egeblad M, Evans RM, et al. A framework for advancing our understanding of cancer-associated fibroblasts. Nat Rev Cancer (2020) 20(3):174–86. doi: 10.1038/s41568-019-0238-1
31. Luo H, Xia X, Huang LB, An H, Cao M, Kim GD, et al. Pan-cancer single-cell analysis reveals the heterogeneity and plasticity of cancer-associated fibroblasts in the tumor microenvironment. Nat Commun (2022) 13(1):6619. doi: 10.1038/s41467-022-34395-2
32. Rudnick JA, Kuperwasser C. Stromal biomarkers in breast cancer development and progression. Clin Exp Metastasis (2012) 29(7):663–72. doi: 10.1007/s10585-012-9499-8
33. Polanska UM, Orimo A. Carcinoma-associated fibroblasts: non-neoplastic tumour-promoting mesenchymal cells. J Cell Physiol (2013) 228(8):1651–7. doi: 10.1002/jcp.24347
34. Polanska UM, Acar A, Orimo A. Experimental generation of carcinoma-associated fibroblasts (CAFs) from human mammary fibroblasts. J Vis Exp (2011) 56):e3201. doi: 10.3791/3201
35. Kalluri R, Zeisberg M. Fibroblasts in cancer. Nat Rev Canc (2006) 6(5):392–401. doi: 10.1038/nrc1877
36. Chen Y, Terajima M, Yang Y, Sun L, Ahn YH, Pankova D, et al. Lysyl hydroxylase 2 induces a collagen cross-link switch in tumor stroma. J Clin Invest (2015) 125(3):1147–62. doi: 10.1172/JCI74725
37. Provenzano PP, Eliceiri KW, Campbell JM, Inman DR, White JG, Keely PJ. Collagen reorganization at the tumor-stromal interface facilitates local invasion. BMC Med (2006) 4(1):38. doi: 10.1186/1741-7015-4-38
38. Mina SG, Huang P, Murray BT, Mahler GJ. The role of shear stress and altered tissue properties on endothelial to mesenchymal transformation and tumor-endothelial cell interaction. Biomicrofluidics (2017) 11(4):044104. doi: 10.1063/1.4991738
39. Peyrol S, Raccurt M, Gerard F, Gleyzal C, Grimaud JA, Sommer P. Lysyl oxidase gene expression in the stromal reaction to in situ and invasive ductal breast carcinoma. Am J Pathol (1997) 150(2):497–507.
40. Afratis NA, Klepfish M, Karamanos NK, Sagi I. The apparent competitive action of ECM proteases and cross-linking enzymes during fibrosis: Applications to drug discovery. Adv Drug Deliv Rev (2018) 129:4–15. doi: 10.1016/j.addr.2018.03.004
41. Vallet SD, Ricard-Blum S. Lysyl oxidases: from enzyme activity to extracellular matrix cross-links. Essays Biochem (2019) 63(3):349–64. doi: 10.1042/EBC20180050
42. Yu M, Shen W, Shi X, Wang Q, Zhu L, Xu X, et al. Upregulated LOX and increased collagen content associated with aggressive clinicopathological features and unfavorable outcome in oral squamous cell carcinoma. J Cell Biochem (2019) 120(9):14348–59. doi: 10.1002/jcb.28669
43. van der Slot AJ, van Dura EA, de Wit EC, De Groot J, Huizinga TW, Bank RA, et al. Elevated formation of pyridinoline cross-links by profibrotic cytokines is associated with enhanced lysyl hydroxylase 2b levels. Biochim Biophys Acta (2005) 1741(1-2):95–102. doi: 10.1016/j.bbadis.2004.09.009
44. Colpaert CG, Vermeulen PB, Fox SB, Harris AL, Dirix LY, Van Marck EA. The presence of a fibrotic focus in invasive breast carcinoma correlates with the expression of carbonic anhydrase IX and is a marker of hypoxia and poor prognosis. Breast Cancer Res Treat (2003) 81(2):137–47. doi: 10.1023/A:1025702330207
45. Das K, Prasad R, Ansari SA, Roy A, Mukherjee A, Sen P. Matrix metalloproteinase-2: A key regulator in coagulation proteases mediated human breast cancer progression through autocrine signaling. BioMed Pharmacother (2018) 105:395–406. doi: 10.1016/j.biopha.2018.05.155
46. Cabral-Pacheco GA, Garza-Veloz I, Castruita-De la Rosa C, Ramirez-Acuña JM, Perez-Romero BA, Guerrero-Rodriguez JF, et al. The Roles of Matrix Metalloproteinases and Their Inhibitors in Human Diseases. Int J Mol Sci (2020) 21(24):9739. doi: 10.3390/ijms21249739
47. Ferrari R, Martin G, Tagit O, Guichard A, Cambi A, Voituriez R, et al. MT1-MMP directs force-producing proteolytic contacts that drive tumor cell invasion. Nat Commun (2019) 10(1):4886. doi: 10.1038/s41467-019-12930-y
48. Lee J, Condello S, Yakubov B, Emerson R, Caperell-Grant A, Hitomi K, et al. Tissue Transglutaminase Mediated Tumor-Stroma Interaction Promotes Pancreatic Cancer Progression. Clin Cancer Res (2015) 21(19):4482–93. doi: 10.1158/1078-0432.CCR-15-0226
49. Tarchi SM, Pernia Marin M, Hossain MM, Salvatore M. Breast stiffness, a risk factor for cancer and the role of radiology for diagnosis. J Transl Med (2023) 21(1)582. doi: 10.1186/s12967-023-04457-0
50. Ishihara S, Inman DR, Li WJ, Ponik SM, Keely PJ. Mechano-Signal Transduction in Mesenchymal Stem Cells Induces Prosaposin Secretion to Drive the Proliferation of Breast Cancer Cells. Cancer Res (2017) 77(22):6179–89. doi: 10.1158/0008-5472.CAN-17-0569
51. Jiang L, Li J, Zhang C, Shang Y, Lin J. YAP−mediated crosstalk between the Wnt and Hippo signaling pathways (Review). Mol Med Rep (2020) 22(5):4101–6. doi: 10.3892/mmr.2020.11529
52. Liu C, Pei H, Tan F. Matrix Stiffness and Colorectal Cancer. Onco Targets Ther (2020) 13:2747–55. doi: 10.2147/OTT.S231010
53. Astudillo P. Extracellular matrix stiffness and Wnt/β-catenin signaling in physiology and disease. Biochem Soc Trans (2020) 48(3):1187–98. doi: 10.1042/BST20200026
54. Provenzano PP, Inman DR, Eliceiri KW, Keely PJ. Matrix density-induced mechanoregulation of breast cell phenotype, signaling and gene expression through a FAK-ERK linkage. Oncogene (2009) 28(49):4326–43. doi: 10.1038/onc.2009.299
55. Bui T, Rennhack J, Mok S, Ling C, Perez M, Roccamo J, et al. Functional Redundancy between β1 and β3 Integrin in Activating the IR/Akt/mTORC1 Signaling Axis to Promote ErbB2-Driven Breast Cancer. Cell Rep (2019) 29(3):589–602.e6. doi: 10.1016/j.celrep.2019.09.004
56. Berger AJ, Renner CM, Hale I, Yang X, Ponik SM, Weisman PS, et al. Scaffold stiffness influences breast cancer cell invasion via EGFR-linked Mena upregulation and matrix remodeling. Matrix Biol (2020) 85-86:80–93. doi: 10.1016/j.matbio.2019.07.006
57. Gupton SL, Riquelme D, Hughes-Alford SK, Tadros J, Rudina SS, Hynes RO, et al. Mena binds α5 integrin directly and modulates α5β1 function. J Cell Biol (2012) 198(4):657–76. doi: 10.1083/jcb.201202079
58. Peng Y, Chen Z, Chen Y, Li S, Jiang Y, Yang H, et al. ROCK isoforms differentially modulate cancer cell motility by mechanosensing the substrate stiffness. Acta Biomater (2019) 88:86–101. doi: 10.1016/j.actbio.2019.02.015
59. Eckert MA, Lwin TM, Chang AT, Kim J, Danis E, Ohno-Machado L, et al. Twist1-induced invadopodia formation promotes tumor metastasis. Cancer Cell (2011) 19(3):372–86. doi: 10.1016/j.ccr.2011.01.036
60. Yang J, Mani SA, Donaher JL, Ramaswamy S, Itzykson RA, Come C, et al. Twist, a master regulator of morphogenesis, plays an essential role in tumor metastasis. Cell (2004) 117(7):927–39. doi: 10.1016/j.cell.2004.06.006
61. Pang MF, Siedlik MJ, Han S, Stallings-Mann M, Radisky DC, Nelson CM. Tissue Stiffness and Hypoxia Modulate the Integrin-Linked Kinase ILK to Control Breast Cancer Stem-like Cells. Cancer Res (2016) 76(18):5277–87. doi: 10.1158/0008-5472.CAN-16-0579
62. Liu X, Ye Y, Zhu L, Xiao X, Zhou B, Gu Y, et al. Niche stiffness sustains cancer stemness via TAZ and NANOG phase separation. Nat Commun (2023) 14(1):238. doi: 10.1038/s41467-023-35856-y
63. Li Y, Randriantsilefisoa R, Chen J, Cuellar-Camacho JL, Liang W, Li W. Matrix Stiffness Regulates Chemosensitivity, Stemness Characteristics, and Autophagy in Breast Cancer Cells. ACS Appl Bio Mater (2020) 3(7):4474–85. doi: 10.1021/acsabm.0c00448
64. Joyce MH, Lu C, James ER, Hegab R, Allen SC, Suggs LJ, et al. Phenotypic Basis for Matrix Stiffness-Dependent Chemoresistance of Breast Cancer Cells to Doxorubicin. Front Oncol (2018) 8:337. doi: 10.3389/fonc.2018.00337
65. Majidpoor J, Mortezaee K. The efficacy of PD-1/PD-L1 blockade in cold cancers and future perspectives. Clin Immunol (2021) 226:108707. doi: 10.1016/j.clim.2021.108707
66. Azadi S, Aboulkheyr Es H, Razavi Bazaz S, Thiery JP, Asadnia M, Ebrahimi Warkiani M. Upregulation of PD-L1 expression in breast cancer cells through the formation of 3D multicellular cancer aggregates under different chemical and mechanical conditions. Biochim Biophys Acta Mol Cell Res (2019) 1866(12):118526. doi: 10.1016/j.bbamcr.2019.118526
67. Salmon H, Franciszkiewicz K, Damotte D, Dieu-Nosjean MC, Validire P, Trautmann A, et al. Matrix architecture defines the preferential localization and migration of T cells into the stroma of human lung tumors. J Clin Invest (2012) 122(3):899–910. doi: 10.1172/JCI45817
68. Paszek MJ, Weaver VM. The tension mounts: mechanics meets morphogenesis and malignancy. J Mammary Gland Biol Neoplasia (2004) 9(4):325–42. doi: 10.1007/s10911-004-1404-x
69. Ng MR, Brugge JS. A stiff blow from the stroma: collagen crosslinking drives tumor progression. Cancer Cell (2009) 16(6):455–7. doi: 10.1016/j.ccr.2009.11.013
70. Mieulet V, Garnier C, Kieffer Y, Guilbert T, Nemati F, Marangoni E, et al. Stiffness increases with myofibroblast content and collagen density in mesenchymal high grade serous ovarian cancer. Sci Rep (2021) 11(1):4219. doi: 10.1038/s41598-021-83685-0
71. Wozniak MA, Desai R, Solski PA, Der CJ, Keely PJ. ROCK-generated contractility regulates breast epithelial cell differentiation in response to the physical properties of a three-dimensional collagen matrix. J Cell Biol (2003) 163(3):583–95. doi: 10.1083/jcb.200305010
72. Stowers RS, Shcherbina A, Israeli J, Gruber JJ, Chang J, Nam S, et al. Matrix stiffness induces a tumorigenic phenotype in mammary epithelium through changes in chromatin accessibility. Nat BioMed Eng (2019) 3(12):1009–19. doi: 10.1038/s41551-019-0420-5
73. Provenzano PP, Inman DR, Eliceiri KW, Knittel JG, Yan L, Rueden CT, et al. Collagen density promotes mammary tumor initiation and progression. BMC Med (2008) 6:11. doi: 10.1186/1741-7015-6-11
74. Sledge GW Jr., Miller KD. Exploiting the hallmarks of cancer: the future conquest of breast cancer. Eur J Canc (2003) 39(12):1668–75. doi: 10.1016/s0959-8049(03)00273-9
75. Zhao B, Li L, Guan KL. Hippo signaling at a glance. J Cell Sci (2010) 123(Pt 23):4001–6. doi: 10.1242/jcs.069070
76. Dupont S, Morsut L, Aragona M, Enzo E, Giulitti S, Cordenonsi M, et al. Role of YAP/TAZ in mechanotransduction. Nature (2011) 474(7350):179–83. doi: 10.1038/nature10137
77. Wang C, Gu C, Jeong KJ, Zhang D, Guo W, Lu Y, et al. YAP/TAZ-Mediated Upregulation of GAB2 Leads to Increased Sensitivity to Growth Factor-Induced Activation of the PI3K Pathway. Cancer Res (2017) 77(7):1637–48. doi: 10.1158/0008-5472.CAN-15-3084
78. Guo YP, Martin LJ, Hanna W, Banerjee D, Miller N, Fishell E, et al. Growth factors and stromal matrix proteins associated with mammographic densities. Cancer Epidemiol Biomarkers Prev (2001) 10(3):243–8.
79. Watson AW, Grant AD, Parker SS, Hill S, Whalen MB, Chakrabarti J, et al. Breast tumor stiffness instructs bone metastasis via maintenance of mechanical conditioning. Cell Rep (2021) 35(13):109293. doi: 10.1016/j.celrep.2021.109293
80. Rozova VS, Anwer AG, Guller AE, Es HA, Khabir Z, Sokolova AI, et al. Machine learning reveals mesenchymal breast carcinoma cell adaptation in response to matrix stiffness. PloS Comput Biol (2021) 17(7):e1009193. doi: 10.1371/journal.pcbi.1009193
81. Wei SC, Fattet L, Tsai JH, Guo Y, Pai VH, Majeski HE, et al. Matrix stiffness drives epithelial-mesenchymal transition and tumour metastasis through a TWIST1-G3BP2 mechanotransduction pathway. Nat Cell Biol (2015) 17(5):678–88. doi: 10.1038/ncb3157
82. Lee GY, Kenny PA, Lee EH, Bissell MJ. Three-dimensional culture models of normal and malignant breast epithelial cells. Nat Methods (2007) 4(4):359–65. doi: 10.1038/nmeth1015
83. Fattet L, Jung HY, Matsumoto MW, Aubol BE, Kumar A, Adams JA, et al. Matrix Rigidity Controls Epithelial-Mesenchymal Plasticity and Tumor Metastasis via a Mechanoresponsive EPHA2/LYN Complex. Dev Cell (2020) 54(3):302–16.e7. doi: 10.1016/j.devcel.2020.05.031
84. Rabie EM, Zhang SX, Dunn CE, Nelson CM. Substratum stiffness signals through integrin-linked kinase and β1-integrin to regulate midbody proteins and abscission during EMT. Mol Biol Cell (2021) 32(18):1664–76. doi: 10.1091/mbc.E21-02-0072
85. Barriga EH, Franze K, Charras G, Mayor R. Tissue stiffening coordinates morphogenesis by triggering collective cell migration in vivo. Nature (2018) 554(7693):523–7. doi: 10.1038/nature25742
86. Chambers AF, Groom AC, MacDonald IC. Dissemination and growth of cancer cells in metastatic sites. Nat Rev Canc (2002) 2(8):563–72. doi: 10.1038/nrc865
87. Anlaş AA, Nelson CM. Soft Microenvironments Induce Chemoresistance by Increasing Autophagy Downstream of Integrin-Linked Kinase. Cancer Res (2020) 80(19):4103–13. doi: 10.1158/0008-5472.CAN-19-4021
88. Alvarez-Elizondo MB, Weihs D. Breast cancer stem cells: mechanobiology reveals highly invasive cancer cell subpopulations. Cell Mol Life Sci (2022) 79(3):134. doi: 10.1007/s00018-022-04181-w
89. Li C, Qiu S, Liu X, Guo F, Zhai J, Li Z, et al. Extracellular matrix-derived mechanical force governs breast cancer cell stemness and quiescence transition through integrin-DDR signaling. Signal Transduct Target Ther (2023) 8(1):247. doi: 10.1038/s41392-023-01453-0
90. Dave B, Mittal V, Tan NM, Chang JC. Epithelial-mesenchymal transition, cancer stem cells and treatment resistance. Breast Cancer Res (2012) 14(1):202. doi: 10.1186/bcr2938
91. Netti PA, Berk DA, Swartz MA, Grodzinsky AJ, Jain RK. Role of extracellular matrix assembly in interstitial transport in solid tumors. Cancer Res (2000) 60(9):2497–503.
92. Stylianopoulos T, Munn LL, Jain RK. Reengineering the Physical Microenvironment of Tumors to Improve Drug Delivery and Efficacy: From Mathematical Modeling to Bench to Bedside. Trends Canc (2018) 4(4):292–319. doi: 10.1016/j.trecan.2018.02.005
93. Deng B, Zhao Z, Kong W, Han C, Shen X, Zhou C. Biological role of matrix stiffness in tumor growth and treatment. J Transl Med (2022) 20(1):540. doi: 10.1186/s12967-022-03768-y
94. Medina SH, Bush B, Cam M, Sevcik E, DelRio FW, Nandy K, et al. Identification of a mechanogenetic link between substrate stiffness and chemotherapeutic response in breast cancer. Biomaterials (2019) 202:1–11. doi: 10.1016/j.biomaterials.2019.02.018
95. Lin CH, Jokela T, Gray J, LaBarge MA. Combinatorial Microenvironments Impose a Continuum of Cellular Responses to a Single Pathway-Targeted Anti-cancer Compound. Cell Rep (2017) 21(2):533–45. doi: 10.1016/j.celrep.2017.09.058
96. Mao Y, Zhu L, Huang Z, Luo C, Zhou T, Li L, et al. Stem-like tumor cells involved in heterogeneous vasculogenesis in breast cancer. Endocr Relat Cancer (2020) 27(1):23–39. doi: 10.1530/ERC-19-0054
97. Gao J, Rong Y, Huang Y, Shi P, Wang X, Meng X, et al. Cirrhotic stiffness affects the migration of hepatocellular carcinoma cells and induces sorafenib resistance through YAP. J Cell Physiol (2019) 234(3):2639–48. doi: 10.1002/jcp.27078
98. Schwartz AD, Barney LE, Jansen LE, Nguyen TV, Hall CL, Meyer AS, et al. A biomaterial screening approach reveals microenvironmental mechanisms of drug resistance. Integr Biol (Camb) (2017) 9(12):912–24. doi: 10.1039/c7ib00128b
99. Nguyen TV, Sleiman M, Moriarty T, Herrick WG, Peyton SR. Sorafenib resistance and JNK signaling in carcinoma during extracellular matrix stiffening. Biomaterials (2014) 35(22):5749–59. doi: 10.1016/j.biomaterials.2014.03.058
100. Panzetta V, Verde G, Pugliese M, Artiola V, Arrichiello C, Muto P, et al. Adhesion and Migration Response to Radiation Therapy of Mammary Epithelial and Adenocarcinoma Cells Interacting with Different Stiffness Substrates. Cancers (Basel) (2020) 12(5):1170. doi: 10.3390/cancers12051170
101. Rieken S, Habermehl D, Wuerth L, Brons S, Mohr A, Lindel K, et al. Carbon ion irradiation inhibits glioma cell migration through downregulation of integrin expression. Int J Radiat Oncol Biol Phys (2012) 83(1):394–9. doi: 10.1016/j.ijrobp.2011.06.2004
102. Johnston SR, Leary A. Lapatinib: a novel EGFR/HER2 tyrosine kinase inhibitor for cancer. Drugs Today (Barc) (2006) 42(7):441–53. doi: 10.1358/dot.2006.42.7.985637
103. Lin CH, Pelissier FA, Zhang H, Lakins J, Weaver VM, Park C, et al. Microenvironment rigidity modulates responses to the HER2 receptor tyrosine kinase inhibitor lapatinib via YAP and TAZ transcription factors. Mol Biol Cell (2015) 26(22):3946–53. doi: 10.1091/mbc.E15-07-0456
104. Hajdú I, Kardos J, Major B, Fabó G, Lőrincz Z, Cseh S, et al. Inhibition of the LOX enzyme family members with old and new ligands. Selectivity analysis revisited. Bioorg Med Chem Lett (2018) 28(18):3113–8. doi: 10.1016/j.bmcl.2018.07.001
105. Moon HJ, Finney J, Ronnebaum T, Mure M. Human lysyl oxidase-like 2. Bioorg Chem (2014) 57:231–41. doi: 10.1016/j.bioorg.2014.07.003
106. Scatena R. Prinomastat, a hydroxamate-based matrix metalloproteinase inhibitor. A novel pharmacological approach for tissue remodelling-related diseases. Expert Opin Investig Drugs (2000) 9(9):2159–65. doi: 10.1517/13543784.9.9.2159
107. Es HA, Cox TR, Sarafraz-Yazdi E, Thiery JP, Warkiani ME. Pirfenidone Reduces Epithelial-Mesenchymal Transition and Spheroid Formation in Breast Carcinoma through Targeting Cancer-Associated Fibroblasts (CAFs). Cancers (Basel) (2021) 13(20):5118. doi: 10.3390/cancers13205118
108. Lee SO, Lee MH, Lee KR, Lee EO, Lee HJ. Fomes fomentarius Ethanol Extract Exerts Inhibition of Cell Growth and Motility Induction of Apoptosis via Targeting AKT in Human Breast Cancer MDA-MB-231 Cells. Int J Mol Sci (2019) 20(5):1147. doi: 10.3390/ijms20051147
109. Liu C, Li X, Hua W, Li J, Han X, Ha Q, et al. Porous Matrix Stiffness Modulates Response to Targeted Therapy in Breast Carcinoma. Small (2016) 12(34):4675–81. doi: 10.1002/smll.201601365
110. Nagy N, Kuipers HF, Frymoyer AR, Ishak HD, Bollyky JB, Wight TN, et al. 4-methylumbelliferone treatment and hyaluronan inhibition as a therapeutic strategy in inflammation, autoimmunity, and cancer. Front Immunol (2015) 6:123. doi: 10.3389/fimmu.2015.00123
111. Wang G, Zhou X, Guo Z, Huang N, Li J, Lv Y, et al. The Anti-fibrosis drug Pirfenidone modifies the immunosuppressive tumor microenvironment and prevents the progression of renal cell carcinoma by inhibiting tumor autocrine TGF-β. Cancer Biol Ther (2022) 23(1):150–62. doi: 10.1080/15384047.2022.2035629
Keywords: matrix stiffness, mechanical stimulation, extracellular matrix, breast cancer, signaling pathways
Citation: Xu R, Yin P, Wei J and Ding Q (2023) The role of matrix stiffness in breast cancer progression: a review. Front. Oncol. 13:1284926. doi: 10.3389/fonc.2023.1284926
Received: 29 August 2023; Accepted: 04 October 2023;
Published: 17 October 2023.
Edited by:
Daniel P. Bezerra, Oswaldo Cruz Foudantion (FIOCRUZ), BrazilReviewed by:
Ahmet Acar, Middle East Technical University, TürkiyeDeanna Edwards, Vanderbilt University Medical Center, United States
Sarah Boyle, Centre for Cancer Biology (CCB), Australia
Michele Zanoni, Scientific Institute of Romagna for the Study and Treatment of Tumors (IRCCS), Italy
Emmanuelle Liaudet-coopman, Institut National de la Santé et de la Recherche Médicale (INSERM), France
Copyright © 2023 Xu, Yin, Wei and Ding. This is an open-access article distributed under the terms of the Creative Commons Attribution License (CC BY). The use, distribution or reproduction in other forums is permitted, provided the original author(s) and the copyright owner(s) are credited and that the original publication in this journal is cited, in accordance with accepted academic practice. No use, distribution or reproduction is permitted which does not comply with these terms.
*Correspondence: Jifu Wei, d2VpamlmdUBob3RtYWlsLmNvbQ==; Qiang Ding, ZGluZ3FpYW5nQG5qbXUuZWR1LmNu
† These authors have contributed equally to this work and share first authorship