- 1Department of Oncology, Changhai Hospital, Naval Military Medical University, Shanghai, China
- 2Clinical Cancer Institute, Center for Translational Medicine, Naval Military Medical University, Shanghai, China
- 3Department of Gastroenterology, Changzheng Hospital, Naval Military Medical University, Shanghai, China
In recent years, numerous studies have demonstrated that the tumor immune microenvironment (TIME) is capable of regulating the growth of tumors, and tumor-infiltrating immune cells in the TIME can affect the prognosis and treatment responses of patients. Consequently, therapies targeting these immune cells have emerged as important antitumor treatments. As a crucial componet of the perioperative treatment of malignant tumors, neoadjuvant chemotherapy (NACT) can improve the surgical resection rate and prognosis of patients and is a suitable clinical model to evaluate the effect of chemotherapy on the TIME. To provide a rationale for developing valid combinational therapies, this review summarizes the impact of NACT on the TIME, the relationship between tumor-infiltrating immune cells and treatment responses of patients, and the prognostic value of these infiltrating immune cells.
Introduction
Neoadjuvant chemotherapy (NACT) is a central part of the comprehensive treatments for locally advanced malignant tumors and was first proposed by Frei in 1982 (1), referring to systemic chemotherapy applied before surgery or radiotherapy. NACT is superior in reducing the clinical tumor stage, increasing the surgical resection rate, reducing postoperative complications, preventing postoperative metastasis, and improving the postoperative survival rate of patients. Because of its sensitivity and efficiency, NACT is beneficial for establishing an effective chemotherapy regimen for postoperative adjuvant chemotherapy and for improving the long-term efficacy of treatment after surgery (2–9).
The tumor microenvironment (TME), which is closely associated with tumor progression and the response to treatments, includes blood vessels, immune cells, fibroblasts, and extracellular matrix (10–13). All immune components within the TME, including innate and adaptive immune cells, extracellular immune factors, and cell surface molecules, are defined as the tumor immune microenvironment (TIME), which is very relevant to the occurrence, development, recurrence, and metastasis of tumors (14–16). The immune structure, composed of the location, type, density, and functional status of immune cells in tumors, is different among patients and is crucial to the response rates and prognosis of patients (17–19).
Chemotherapy, a conventional treatment for most malignancies, can inhibit tumor cell mitosis and nucleic acid anabolism as well as directly interfere with tumor cell DNA replication (20). Although considered to weaken the immune system and induce various adverse effects in prior reports, recent studies have proven that classical cytotoxic drugs not only kill tumor cells but also induce immunogenicity. Meanwhile, chemotherapy activates the immune system by promoting lymphocyte activation and decreasing suppressive immune cells (21, 22).
NACT is a good clinical model to evaluate the effect of chemotherapy on the TIME because it is convenient to obtain paired tumor tissues before and after treatment. To provide strategies and theoretical bases for combining immunotherapy and enhancing the efficacy of NACT, this review summarizes the remodeling effect of NACT on the TIME in gastrointestinal tumors by outlining the changes in tumor-infiltrating immune cells before and after NACT, the efficacy prediction, and the associated prognostic value (Table 1).
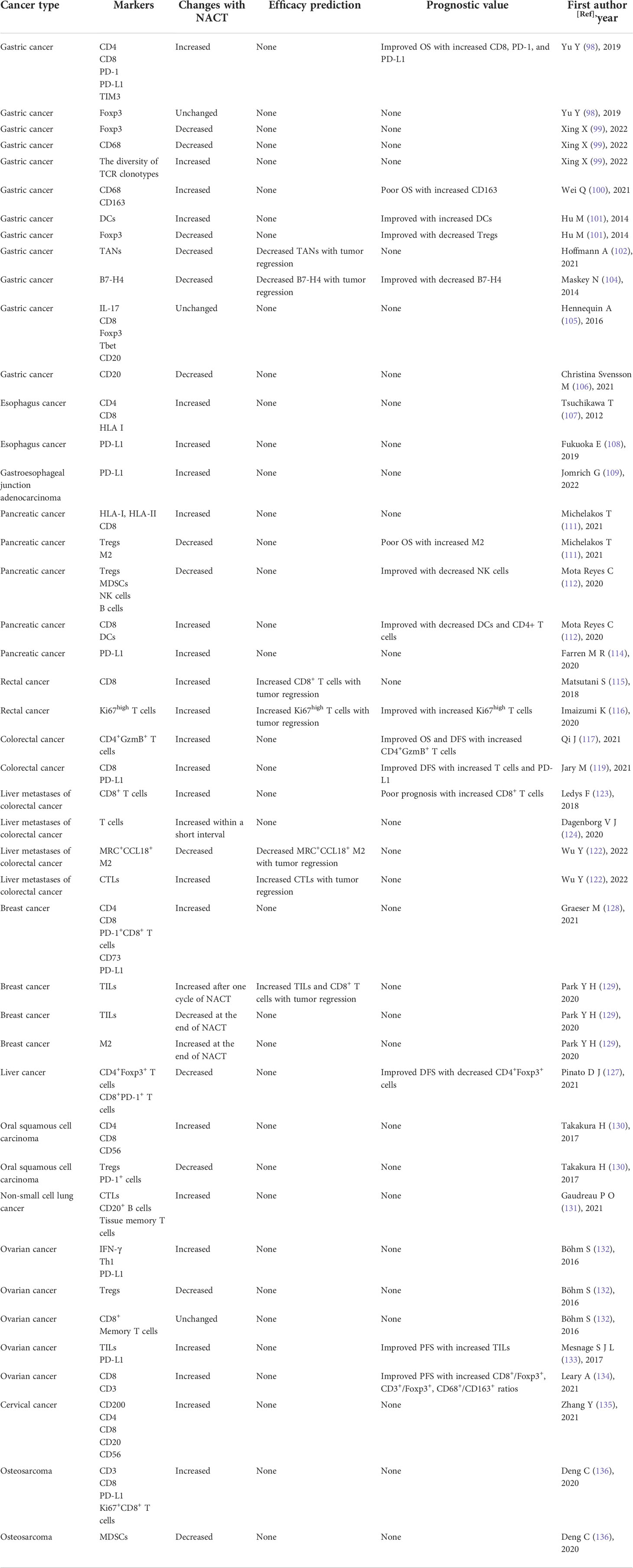
Table 1 Summary of the remodeling effect of NACT on the TIME and the associated prognostic significance.
The composition of TIME with clinical significance
The TIME, where tumor cells can effectively modify their surroundings by secreting a variety of cytokines and chemokines, is an integral and indispensable part of tumor tissues (Figure 1). Moreover, immune cells in the TIME have been proven to be related to tumor development, metastasis, and recurrence. Innate immune cells (macrophages, neutrophils, dendritic cells, myeloid-derived suppressor cells, and natural killer cells) and adaptive immune cells (T and B cells) in the TIME can act as tumor antagonists or tumor agonists. Although these immune cells tend to kill tumor cells in the early stage, tumor cells are still able to evade immune surveillance through a variety of mechanisms, even creating a variety of ways to inhibit the function of antitumor immune cells and reduce the clinical effectiveness of antitumor therapies.
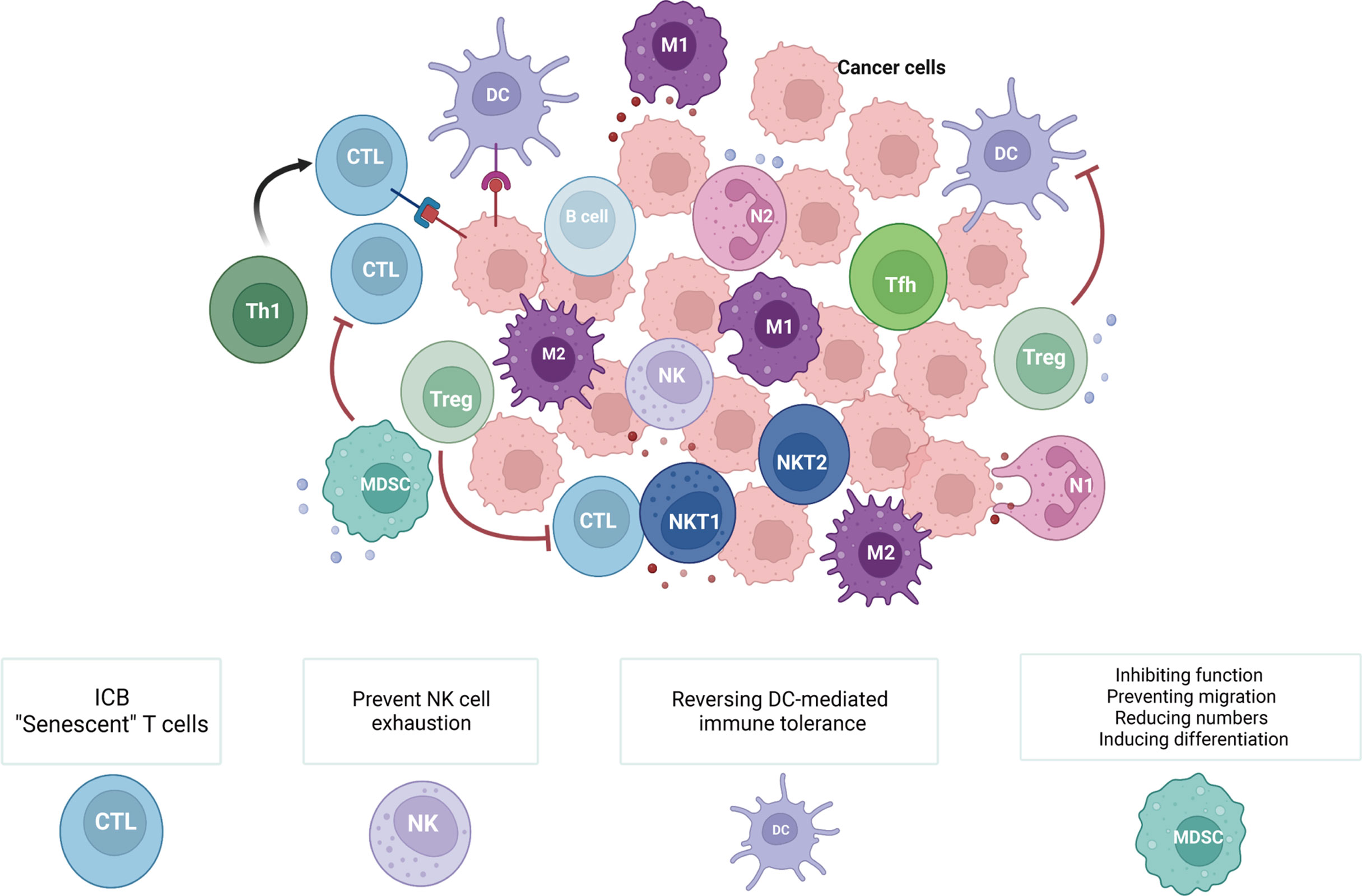
Figure 1 Immune cells within the TIME and related cancer immunotherapies. The TIME consists of various innate immune cells (macrophages, neutrophils, dendritic cells, myeloid-derived suppressor cells, and natural killer cells) and adaptive immune cells (T and B cells). During different stages of tumors and due to the diversity of the TIME, these immune cells have distinct roles in antitumor immunity. Therefore, methods for cancer immunotherapy that target tumor-suppressing immune cells include ‘exhausted’ T cells (ICB), ‘senescent’ T cells, exhausted NK cells, DC-mediated immunological tolerance, and MDSCs (inhibiting the function of MDSCs, preventing the migration of MDSCs, reducing the number of MDSCs, and inducing the differentiation of MDSCs). (Created with Biorender.com).
CD8+ T cells
CD8+ T cells, a key component of the adaptive immune system (23), play an important role in antitumor immune responses by recognizing and eliminating tumor cells. It has been reported that the density of CD8+ T cells is closely related to the clinical prognosis of patients (24, 25). To elicit efficient anticancer immune responses, CD8+ T cells need to go through a series of events in the ‘cancer immune cycle’, including neoantigen production by tumor cells, antigen recognition and antigen presentation by dendritic cells (DCs), and activation of cytotoxic lymphocytes (cytotoxic T lymphocytes, CTLs). Finally, activated CTLs infiltrate into tumor tissues and destroy tumor cells (26–28).
However, the immunosuppressive network in the TME can remodel CD8+ T cells, leading to CD8+ T-cell dysfunction, failure to remove tumor cells, and weakened antitumor immunity (26, 29). Two of the main dysfunctional states of T cells in the TME are ‘exhaustion’ and ‘senescence’ (29, 30). ‘Exhausted’ T cells are characterized by upregulated expression of inhibitory receptors, such as programmed cell death protein 1 (PD-1), cytotoxic T-lymphocyte antigen-4 (CTLA-4), T-cell immunoglobulin and mucin domain containing-3 (Tim-3), lymphocyte activation gene 3 (LAG-3), T-cell immunoreceptor with Ig and ITIM domains (TIGIT), and so on (29, 31). Elevated levels of ‘exhausted’ T cells in tumors are associated with a poor prognosis, and targeting inhibitory receptors to restore CD8+ T-cell function has important implications in clinical treatments (29, 32). Moreover, the efficacy of immune checkpoint blockade (ICB) therapy has been demonstrated in various antitumor immunotherapies (29, 33). Unlike ‘exhausted’ T cells, ‘senescent’ T cells exhibit a senescence-associated secretory phenotype (SASP), producing a mass of inflammatory cytokines (IL-2, TNF-α, IFN-γ) and suppressive cytokines (IL-10 and TGF-β). Due to their vital roles in immunosuppression and tumor progression, senescent T cells are new targets for antitumor immunotherapy (29, 34).
CD4+ T cells
CD4+ T cells, helper T lymphocytes (Th), are involved in adaptive immune responses (35) and can regulate the state and function of other immune cells, playing a significant role in autoimmunity, allergic reactions, and antitumor immune responses (36). CD4+ Th cells are divided into different subpopulations, including Th1, Th2, Th17, Tfh, and Tregs, and each subpopulation displays a specific role in tumor immune responses, inhibiting or promoting tumor cell growth (24). After antigen-induced activation, Th1 cells generate inflammation by producing inflammatory factors such as TNF-α and IFN-γ, as well as promoting DC maturation and improving CTL function (37). Th1 cells are frequently associated with favorable clinical outcomes in patients (38). Studies have shown that cytokines such as IFN-γ secreted by Th1 cells not only enhance CD8+ T-cell differentiation but also directly inhibit tumor cell growth by causing their senescence (39, 40). Tfh (follicular helper T cells, Tfh) cells exert antitumor effects in tumors by mediating B-cell differentiation into plasma cells, antibody class switching, and the production of antibodies. Although the presence of Tfh cells predicts a favorable outcome, their antitumor functions may be blocked by the PD-L1/PD-1 signaling pathway in the TME (41).
Regulatory T cells (Tregs), expressing forkhead protein P3 (Foxp3), are pivotal in maintaining immune homeostasis and peripheral tolerance (42), as well as suppressing overactive immune responses such as autoimmune diseases (43). Clonal expansions of Tregs are highly heterogeneous, observed in many types of tumors, and are closely associated with a poor prognosis and reduced survival rates (44, 45). In the TIME, Tregs mainly inhibit antitumor immune responses through two mechanisms and participate in the process of immune escape (43, 46). Tregs release inhibitory cytokines (such as IL-10 and TGF-β) to prevent the infiltration and activity of tumor-specific T cells (37). However, Tregs also impede the development and maturation of DCs (47). Tumor-infiltrating Tregs express numerous negative costimulatory molecules (such as PD-L1 and PD-L2) that inhibit CD8+ T-cell activation and interact with the receptor PD-1 on CD8+ T cells to block TCR signaling, thereby repressing the activity of CD8+ T cells (48).
NK cells and NKT cells
NK (natural killer, NK) cells are innate lymphocytes that kill tumor cells nonspecifically in the early stages, performing antitumor immune surveillance (49). According to their expression of CD16 and CD56, NK cells are divided into two subsets: CD56hiCD16± and CD56loCD16hi (50). The CD56hiCD16± subset secretes inflammatory cytokines, whereas CD56loCD16hi mainly exerts cytotoxic and killing functions (50). To promote antitumor immune responses, NK cells recruit DCs to tumor sites and secrete cytokines, promoting DC maturation (51). In addition, the quantity of infiltrating NK cells is remarkably linked to improved patient outcomes (52, 53). Tumor cells and other cells in the TME may prevent NK-cell activation and inhibit their function by secreting a variety of cytokines (including IL-6, IL-10, TGF-β, PGE2, and IDO) (49, 54). Moreover, the activation of inhibitory immune checkpoints (such as CTLA-4, PD-1, and Tim-3) hampers NK-cell function (49). Blocking the checkpoint receptor TIGIT can prevent NK-cell exhaustion and restore NK-cell activity (55).
NKT (natural killer T, NKT) cells, a subset of natural lymphocytes, simultaneously express certain surface markers of T cells and NK cells (56) and play a critical role in innate and adaptive immunity. In light of the various types of TCRs, NKT cells exhibit two main subtypes: type I NKT and type II NKT cells. Type I NKT cells secrete Th1-type cytokines (IFN-γ, IL-12) to boost antitumor immune responses (57), while type II NKT cells suppress antitumor immunity by producing IL-13 to restrain the function of CD8+ T cells (58). In addition, NKT cells transform into their various subpopulations and exhibit different functions in different environments (59, 60).
Dendritic cells
DCs are recognized as the most powerful and specialized antigen-presenting cells known so far (61). DCs recognize and present antigens to CTLs and simultaneously provide costimulatory signals and cytokines to T cells for further activation (49, 62). As reported, infiltrating DCs in the TME are positively associated with the prognosis of patients (63, 64). DCs are functionally differentiated into distinct subpopulations: classical DCs (cDCs), plasmacytoid DCs (pDCs), and monocyte-derived inflammatory DCs (moDCs) (59). cDCs and pDCs are present and active under steady-state conditions, whereas moDCs appear only during inflammation (59). In the TME, multiple immunosuppressive factors, such as VEGF and IL-10, hinder DC maturation and impair their capability for antigen presentation and T-cell activation (65, 66). Tumor cells can also polarize DCs into tolerogenic DCs (67), and reversing DC-mediated immune tolerance is the main therapeutic strategy against DCs in the TME.
Macrophages
Macrophages derived from circulating monocytes are significant immune cells in the TME, accounting for approximately 50% of tumor tissues (68–70). Macrophages play a principal role in immune defense, immune homeostasis, immune surveillance, antigen presentation, and immune regulation (71). Macrophages exhibit two distinguished types, inflammatory M1 (classically activated) and immunosuppressive M2 (alternately activated) (72). M1 macrophages secrete inflammatory cytokines and reactive oxygen/nitrogen that are essential for host defense and tumor killing (73, 74). Monocytes in the bone marrow are recruited into the TME under the action of various chemokines and further differentiate and develop into mature macrophages (75–77), tumor-associated macrophages (TAMs). TAMs are phenotypically and functionally similar to M2 macrophages, which express low levels of MHC class II molecules, manifest decreased antigen presentation activity, and secrete high levels of immunosuppressive cytokines. During the course of tumor evolution, TAMs accelerate tumorigenesis, development, invasion, and metastasis, especially in angiogenesis and lymphangiogenesis (72, 78).
Neutrophils
Neutrophils represent the front line of the body’s defense system, accounting for 55% to 70% of circulating leukocytes (79). By creating neutrophil extracellular traps and secreting different cytokines and chemokines, neutrophils not only fight infection by phagocytosis but also trigger inflammatory responses (80, 81). Tumor-associated neutrophils (TANs) manifest N1 (tumor-suppressing) and N2 (tumor-promoting) phenotypes, and the phenotype of TANs depends on the type and stage of tumors (59). In the early stage of tumorigenesis, neutrophils secrete cytokines (such as IFN-γ) to recruit and stimulate immune cells to restrain tumor growth. Neutrophils isolated from early-stage lung cancer prompted CD4+ T cells to release IFN-γ, which in turn enhanced the differentiation of CD8+ T cells (82) and supported antitumor immunity. However, with the development of tumors, neutrophils gradually shift into an immunosuppressive phenotype (59, 83) and promote tumor progression (82). TANs have been reported to be associated with a poor prognosis in gastric cancer by enhancing the migration, invasion, and epithelial-mesenchymal transition of gastric cancer cells (84). Additionally, TANs recruit macrophages and Tregs to the TME, resulting in liver cancer progression and resistance to sorafenib (14).
Myeloid-derived suppressor cells
MDSCs, tumor-promoting cells in the TME, are bone marrow-derived immunosuppressive heterogeneous cell populations consisting of myeloid progenitor cells, immature macrophages, immature granulocytes, and immature dendritic cells (85). Granulocytic or polymorphonuclear MDSCs (PMN-MDSCs) and mononuclear MDSCs (monocytic MDSCs, M-MDSCs) are the two main subsets of MDSCs (86, 87). In addition to promoting tumor angiogenesis, stemness, and metastasis, activated MDSCs suppress antitumor immunity mediated by T cells, NK cells, and macrophages (88, 89). In addition, MDSCs are linked to a shorter overall survival (50) and they are vital therapeutic targets due to their important role in the establishment of a premetastatic niche (59). Currently, therapies against MDSCs basically focus on inhibiting their immunosuppressive function, preventing their migration to the TME, reducing their numbers, and driving their differentiation into an inflammatory phenotype (90).
B cells
Although the research to date primarily concentrates on T cells, increasing evidence suggests that tumor-infiltrating B lymphocytes (TIL-Bs), comprising tumor-infiltrating B cells and plasma cells, have an indispensable synergistic role in tumor control (91). The most prominent phenotypes of TIL-Bs are the effector and regulatory B-cell (Breg) subsets (91). Depending on the composition of the TME and the phenotype and antibodies produced by B cells, TIL-Bs exert antitumor or protumor effects (92). By presenting antigens to CD4+ and CD8+ T cells, B cells trigger antigen-specific immune responses in the TME (92, 93). In addition, B cells promote tumor-specific B-cell maturation and isotype switching and tumor-specific T-cell effects by promoting the formation of tumor-associated tertiary lymphoid structures (TLSs) (92, 94). In vitro, B cells isolated from melanoma patients triggered antibody-dependent cell cytotoxicity (ADCC) after producing IgG (immune globulin G, IgG), thus eliminating tumor cells (92). Studies have shown that the presence of TIL-Bs is associated with a favorable prognosis in patients (91, 95). However, Bregs can suppress antitumor immunity by releasing inflammatory mediators (such as IL-10) and expressing inhibitory costimulatory molecules (such as PD-L1), and the infiltration of Bregs is positively correlated with Tregs (96, 97).
The remodeling effect of NACT on the TIME and its prognostic value in gastrointestinal tumors
Gastric cancer
An analysis of paired pre- and post-NACT tumor samples from 60 patients with gastric cancer revealed that, whereas the expression of Foxp3 remained unchanged, the expression of CD4, CD8, PD-1, PD-L1, and TIM3 increased dramatically after NACT. These increased CD4+ T cells and CD8+ T cells following NACT demonstrated that chemotherapy sparked hosts’ antitumor immune responses. However, following initial immune stimulation, IFN-γ produced by activated T cells generated negative feedback to induce the expression of PD-L1, which then reprogrammed the TIME from an active state to a generally balanced environment. This indicated the dynamic bidirectional transfer of the gastric cancer TIME during chemotherapy (98). Additionally, variations in TIM3, PD-1, and PD-L1 between baseline and post-NACT demonstrated a substantially positive relationship with one another, suggesting that dual targeting against PD-L1 and TIM3 may be a potentially beneficial choice for gastric cancer patients (98). In multivariate analysis, upregulated expression of CD8+ T cells, PD-1, and PD-L1 post-NACT were favorable prognostic factors of overall survival (OS) (98), possibly because they reflected powerful immunological flexibility.
However, another study uncovered slightly dissimilar outcomes. Foxp3+ Tregs decreased significantly in a cohort of 30 matched patients (before and after NACT), according to research by Xing X et al. Furthermore, patients who responded to chemotherapy had higher levels of Tregs prior to NACT, and following NACT, these patients had lower Treg and higher CTL levels (99). In addition, the TCR numbers decreased significantly, while the number of specific TCR clones increased, indicating an increase in T-cell diversity following NACT (99). This suggests that chemotherapy can boost antitumor immunity and alleviate immunosuppression in gastric cancer patients by increasing the expansion of T cells. Moreover, Xing X et al. also analyzed another 1416 patients (341 of whom underwent NACT) and discovered that the recruitment of CD68+ macrophages was reduced in the NACT group and that CD8+ T cells post NACT were an independent predictor of a better outcome (99).
In contrast, the study by Wei Q et al. showed that in 50 paired patients, the expression of CD68 and CD163 increased significantly after NACT, implying that NACT promoted the recruitment of CD68+ macrophages and CD163+ macrophages (100). Higher CD163 expression after NACT was found to be an independent predictor of a poor OS in multivariate analysis. Moreover, the CD163/CD68 ratio and the CD8/CD3 ratio after chemotherapy were correlated, and a higher CD8/CD3 ratio neutralized the immunosuppressive effect of M2 macrophages (100).
Another examination of postoperative samples from 102 patients (56 patients receiving NACT: NACT group) revealed that DCs increased considerably in the NACT group and were significantly associated with histopathological type, depth of invasion, TNM stage, and lymph node metastasis. Consistent with the above study (99), this study also noted a reduced number of Tregs following NACT. Increased DCs and decreased Tregs post-NACT served as biomarkers for a favorable prognosis in gastric cancer (101).To explore the alterations of TANs in the TME, Hoffmann A et al. analyzed postoperative gastric cancer tissues from 622 patients (173 patients receiving NACT) and assessed TANs in the mucosa, tumor surface, tumor center, invasion front, and tumor scar. According to their findings, TANs at the invasion front decreased remarkably in the NACT group, and the proportions of TANs at the tumor center and invasive front were correlated with tumor regression. TANs were also linked to the ratio of CD8+ T cells in the tumor center and invasive front, and a higher density of CD8+ T cells in the tumor center was associated with an improved OS (102). The decreased proportion of TANs post-NACT was further confirmed by transcriptome sequencing data from another study involving 35 paired patients (103). These studies demonstrate that the systemic influences of NCAT (leukopenia and neutropenia) also lead to local effects of markedly reduced TANs in the TME (102).
In addition to concentrating on tumor-infiltrating immune cells, studies have also investigated the expression of costimulatory molecules after NACT. The expression of the negative costimulatory molecule B7-H4 decreased after NACT, and its lower expression was a biomarker for treatment effectiveness and a good prognosis. Thus, NACT enhanced antitumor immunity by downregulating the expression of the negative costimulatory molecule B7-H4, leading to reduced survival rates in gastric cancer patients (104).
Despite the fact that the bulk of research has shown variations in the TIME, Hennequin A et al. found no significant variances. In their research, the proportions of IL-17+ T cells, CD8+ T cells, Foxp3+ Tregs, Tbet+ T cells, and CD20+ B cells in the central and invasive margins of postoperative tumor tissue from 82 patients (42 of whom received NACT) were analyzed. There was no discernible impact of NACT on immune cell density, kind, or prognostic value (105). In contrast, another study found a reduction in the fraction of CD20+ B cells after NACT in 39 esophageal and 109 gastric cancer paired patients (pre-NACT and post-NACT) (106).
In view of the differences in the antibodies used, the number of samples, and the heterogeneity between patients and within the tumor, there are some discrepancies among the aforementioned studies. In summary, NACT modulates the tumor immunity of gastric cancer in a bidirectional manner, not only stimulating antitumor immune responses but also inducing tumor immunosuppression. The effect of chemotherapy on the TIME should be taken into account when devising combined regimens.
Esophageal cancer
In an analysis of 18 patients with esophageal squamous cell carcinoma (8 patients receiving NACT: NACT group), Tsuchikawa T et al. illustrated that CD4+ T cells, CD8+ T cells, and the expression of HLA I increased in the NACT group and that in esophageal squamous cell carcinoma, NACT improved patients’ survival by inducing T lymphocyte infiltration and upregulating HLA class I expression (107).
Similarly, another study showed that in 69 paired patients (pre- and post-NACT), the infiltration of CD8+ T cells and the expression of PD-L1 on immune cells increased following NACT, which suggested that PD-1/PD-L1 blockade synergizes with NACT in the treatment of patients with esophageal squamous cell carcinoma (108). Increased levels of PD-1 were also observed in 40 patients with gastroesophageal junction adenocarcinoma after NACT (109).
Overall, NACT influences the TIME of esophageal cancer and gastroesophageal junction tumors, and combined therapy targeting the PD-1/PD-L1 pathway may improve the prognosis of these patients.
Pancreatic cancer
An investigation of the gene expression of pancreatic ductal adenocarcinoma (PDAC) in the GEO database (gene chip: GSE129492) made it obvious that 83 genes were differentially expressed between the NACT and non-NACT groups. Furthermore, these genes were mainly engaged in the following biological pathways: immune system, cytokine signal transduction in the immune system, innate immune system, TCR signaling in CD8+ T cells, CD40/CD40 L signaling, and TCR signaling in CD4+ T cells. These findings suggest that NACT affects the TIME of PDAC (110).
Michelakos T et al. analyzed the expression of HLA-I, HLA-II, and immune cells from 248 patients with PDAC. They found that after NACT, HLA-A deficiency was reduced, immune escape of tumor cells was weakened, the density of CD8+ T cells increased, and Tregs and M2 macrophages decreased. Additionally, abundant infiltration of M2 macrophages was an independent predictor of a poor OS. Therefore, NACT ameliorated the immunosuppressive TIME in PDAC (111).
Similar to the study mentioned above (111), another examination involving 27 pancreatic cancer patients treated with NACT substantiated that NACT reactivated a potent and prolonged local immune response in PDAC. After NACT, the infiltration of Tregs, MDSCs, NK cells, and B cells was reduced significantly, while the proportion of CD8+ T cells to all leukocytes and the percentage of DCs increased dramatically. Furthermore, CD4+ T cells and NK cells were independent prognostic factors (112). Identical outcomes were noticed in another investigation where the function of Tregs and B cells was suppressed following NACT in PDAC (113).
Research has also been performed on the changes in Tfh cells in PDAC following NACT. As previously reported, the PD-L1/PD-1 signaling pathway may suppress the antitumor activity of Tfh cells (41). In patients who received NACT, the function of Tfh cells was reversed, and their capacity to express CXCL13 and IL-21 was considerably enhanced. As a result, Tfh cells shaped an immune-active TIME by recruiting CD8+ T cells and promoting the maturation of B cells into antibody-producing plasma cells. Meanwhile, a higher Tfh cell density was associated with a better patient prognosis. These results suggested that NACT unleashed antitumor immunity locally in PDAC by reversing Tfh cell function (41).
In addition, NACT increased the expression of the costimulatory molecule PD-L1 in 24 PDAC patients (6 of whom received NACT) (114), suggesting that NACT patients will benefit from PD-1/PD-L1 targeted therapy.
On the whole, NACT can reverse the suppressive microenvironment of pancreatic cancer and reinforce patients’ antitumor immune responses. Incorporating immunotherapy (such as PD-1/PD-L1 targeted therapy) may be beneficial for patients with pancreatic cancer.
Colorectal cancer
According to an analysis by Matsutani S et al. of tumors from 64 rectal cancer patients (33 of whom received NACT), CD8+ T cells increased following NACT, and a higher density of CD8+ T cells was associated with better clinicopathological responses, which demonstrated that T-cell-mediated immune responses play an essential role in the clinicopathological reaction to NACT (115). Additionally, detection of T-cell activation status revealed increased T-cell activation after NACT. T-cell subset characterization of 188 rectal cancer patients (46 patients receiving NACT) showed that the density of total and activated T cells (Ki67high) increased remarkably after NACT, and the infiltration of T cells was much greater in patients with a better therapeutic outcome. In the multivariate analysis, the number of stromal Ki67highCD8+ T cells following NACT was a better prognostic factor (116).
NACT can also activate local immune responses in colorectal cancer. After analyzing immune cells in 77 patients with stage II/III colorectal cancer (38 patients receiving NACT), researchers discovered that in the NACT group, the infiltration of CD4+GzmB+ T cells in the central region of the tumors increased, and CD4+GzmB+ T cells were a better prognostic factor for OS and disease-free survival (DFS) (117). Furthermore, several studies noted increased expression of CD8+ T cells and PD-L1 in tumors (118) and metastatic sites (119, 120) of colorectal cancer after NACT, with upregulated T cells and PD-L1 on immune cells predicting a better DFS (119). Therefore, NACT enhanced the antitumor immune response by promoting the recruitment of CD4+GzmB+ T cells and CD8+ T cells in colorectal cancer. Recruited CD8+ T cells then released IFN-γ, which in turn led to upregulated PD-L1 levels.
Liver metastases, the leading cause of colorectal cancer death, occur in 50% of patients and manifest a highly heterogeneous microenvironment (121, 122). Understanding how NACT affects the microenvironment of liver metastasis is essential for developing therapeutic approaches as well as for determining the key mechanisms of NACT. One study, comprising 114 patients with colorectal cancer liver metastasis (47 patients receiving NACT), highlighted that T-cell infiltration and PD-L1 expression increased significantly in patients who accepted NACT (123). These results suggested that NACT recruited immune cells in patients with colorectal cancer liver metastases, but the recruited CD8+ T cells might induce immune tolerance, which is responsible for the poor outcome of certain patients. Another study found time-dependent alternations in T cells, with T cells increasing in the group that underwent surgical resection only within a short interval (<9.5 weeks) after the completion of NACT (124). Moreover, the Treg/CTL ratio was lower in the short-interval group (124). This study illustrated that the immunosuppressive milieu gradually becomes prominent after NACT, while the time-dependent changes in immune cells indicated that there might be a time window of opportunity for application of immunotherapy.
Wu Y et al. depicted the spatiotemporal immune landscape of colorectal cancer liver metastases at the single-cell level (a total of 20 patients, 11 of whom received NACT) and learned that there were highly metabolically activated MRC+CCL18+ M2 macrophages in the metastatic milieu. In patients with partial remission, MRC+CCL18+ M2 macrophages decreased and CTLs increased in the TIME of liver metastasis, indicating that antitumor immunity had recovered, whereas the inhibitory TIME was more obvious in nonresponding patients (122). This study revealed that NACT potently restored the tumor immunological homeostasis in patients who responded to chemotherapy, and targeting metabolic pathways can be employed as a combined therapy option.
To conclude, in patients with rectal cancer and colorectal cancer, NACT stimulates antitumor immune responses, and combining immunotherapy may be an effective choice. Meanwhile, NACT reshapes the TIME of colorectal cancer liver metastases in a time-dependent manner, and it is of benefit for these patients to combine immunotherapy or metabolic therapy at an appropriate time point.
Liver cancer
Transarterial chemoembolization (TACE) is a preferred local therapy for patients with Barcelona stage B liver cancer (125) and is able to hinder tumor progression. As has been reported, the efficacy of TACE is correlated with treatment-induced immune modulation (126). After analyzing 119 patients with liver cancer (58 of whom received TACE before surgery), Pinato D J et al. observed that CD4+Foxp3+ cells and CD8+PD-1+ cells were lower in the TACE group, and lower CD4+Foxp3+ cells were associated with a better DFS. In addition, signaling pathways associated with chemokine secretion, regulation of immune cell function, complement cascade activation, and production of interleukins and cytokines were upregulated. This study found that TACE has pleiotropic effects in regulating the TIME, reducing the proportions of Tregs and exhausted T cells, and upregulating proinflammatory signaling pathways. Adding immunotherapies such as depleting Tregs and inhibiting Treg function to enhance the antitumor effect of TACE can be viable therapeutic strategies (127).
Other types of tumors
There are studies regarding early changes in immune infiltration post NACT in breast cancer. A study involving 66 paired triple-negative breast cancer patients showed that after one cycle of NACT, the infiltration of total T cells, CD4+ T cells, CD8+ T cells, and PD-1+CD8+ T cells increased, and the expression of CD73 and PD-L1 also increased significantly (128). Another study also displayed dynamic changes in the TME following NACT. According to the study by Park YH et al., NACT induced dynamic changes in the TME, and the role varied with breast cancer subtypes and pathological remission. Only one cycle of NACT increased the infiltration of TILs and induced an activated immune microenvironment. Compared with the baseline, the residual tumors exhibited an immunosuppressive state at the end of treatments, in which the abundance of TILs and immune-stimulated cell types was reduced, and immunosuppressive M2 macrophages were increased. Higher levels of post-NACT TILs and CD8+ T cells were associated with complete pathological remission. Additionally, the on-treatment immune response was more predictive of treatment outcome than immune signatures in paired baseline samples, although these were strongly correlated (129). This study showed that NACT initially induced antitumor immunity, but eventually, it became immunosuppressive, and the antitumor efficacy was impaired. Including immunomodulatory therapy might be more beneficial early on rather than later (129).
An analysis of 18 patients with oral squamous cell carcinoma (8 of whom received NACT) showed that the infiltration of CD4+ T cells, CD8+ T cells, and CD56+ NK cells into tumors were much greater, whereas the proportions of Tregs and PD-1+ cells decreased. These results support the immunomodulatory role of NACT in oral squamous cell carcinoma (130).
Gaudreau P O et al. studied the TIME of 511 patients with non-small cell lung cancer (146 of whom had NACT) and discovered that following NACT, the infiltration of CTLs, CD20+ B cells, CD8+CD103+ and CD4+CD103+PD-1+TIM3- tissue memory T cells increased noticeably. NACT, however, had no impact on the clonality and abundance of TCRs or tumor mutational load. This study showed that NACT promoted antitumor immunity in non-small cell lung cancer by recruiting T and B cells and by phenotypic polarization toward cytotoxic and memory CD8+ T cells or CD4+ memory T cells, suggesting that combining T-cell agonists (such as TLR9, STING, and IL-10 agonists) can be a promising treatment for non-small cell lung cancer (131).
NACT reshaped the TIME of ovarian cancer as well. An analysis of 54 patients with high-grade serous ovarian cancer who received NACT showed that IFN-γ produced by CD4+ T cells and antitumor Th1-related genes increased after NACT, whereas CD8+ T cells and CD45RO+ memory cells remained unaltered. Moreover, the expression of PD-L1 was significantly elevated, and in patients responding well to chemotherapy, the proportion of Tregs was reduced (132). Similarly, Mesnage S J L et al. also observed a significant increase in TILs and PD-L1 following NACT, and multivariate analysis showed that a higher TIL level post NACT was an independent predictor of a better PFS (133). More recently, the study by Leary A et al. focused on the impact of NACT on the balance between immune-active and immune-tolerant subpopulations (134). Their results indicated that NACT significantly increased CD3+ T cells and CD8+ T cells, whereas higher CD8+/Foxp3+, CD3+/Foxp3+, and CD68+/CD163+ ratios after NACT were associated with a better PFS (134). Altogether, NACT can enhance the immune response by regulating the balance between immune-active and immune-tolerant subsets, but this effect is attenuated by elevated PD-L1. Chemotherapy combined with immunotherapy can help to improve disease control in advanced high-grade serous ovarian cancer (132–134).
In a retrospective analysis of 109 patients with cervical cancer, increased signaling in CD4+ T cells, CD8+ T cells, CD20+ B cells, and CD56+ NK cells was noticed after NACT, particularly in those who had a good response. By RNA sequencing, upregulation of the immunosuppressive molecule CD200 was also detected. This study suggested that NACT improved local antitumor immunity in cervical cancer, and immune checkpoint inhibitors are worthy of further exploration as an addition to NACT therapy (135).
Deng C et al. showed that after NACT, CD3+ T cells, CD8+ T cells, Ki67+CD8+ T cells, and PD-L1+ immune cells increased, while HLA-DR-CD33+ MDSCs decreased. The conclusion was drawn that NACT activated the local immune state of osteosarcoma and relieved the immunosuppressive state, and research into the immunomodulatory effect of NACT on patients with osteosarcoma may offer a stronger theoretical foundation for selecting immunotherapies to combine with NACT (136).
Conclusion and perspectives
The TIME plays a significant role in the survival and prognosis of patients. Researchers have demonstrated that chemotherapy can alter the TIME by regulating the amount and activity of various immune cells. In light of its convenience in obtaining clinical samples before and after chemotherapy, extensive research has focused on the effect of NACT on the TIME in gastrointestinal cancer, which assists in offering a reference for formulating more effective combined therapeutic methods. In this review, we recapitulated the remodeling effect of NACT on the TIME, the efficacy prediction role of infiltrating immune cells, and their associated prognostic value in multiple tumors (Table 1). In general, NACT can impact the TIME and improve antitumor immunity by changing the number and function of infiltrating immune cells. After NACT, antitumor immune cells, such as CD4+ T cells, CD8+ T cells, M1 macrophages, and DCs, increase, while immunosuppressive cells tend to decrease. Additionally, NACT can alter the expression of inhibitory receptors such as PD-1 and CTLA-4, and therapies combining ICB with NACT may be a promising approach to improving the treatment response and survival of patients.
There are, however, some defects in the current research that need to be resolved immediately. First, the fundamental status of the included populations, the severity of their disease, and the number of cycles of chemotherapy, are quite different across studies. In addition, standards and norms for the score of tumor-infiltrating immune cells vary from research to research. Finally, inadequate preoperative biopsy representativeness and inconsistent evaluation time points also lead to discrepancies. To minimize these biases and better elucidate the immune alterations, we need to broaden the included cohorts and set precise criteria for assessing the infiltrating immune cells. Nevertheless, the effort is worth it due to the expectation that appropriate individualized immunotherapies will be administered based on the post-NACT tumor-infiltrating immune cell profile, which is expected to become a new approach to antitumor immunochemotherapy in the future.
Author contributions
YW, PG, ZH, and LC conceived the present study. XL, YJ, JYL, JL and YZ conducted the literature search. The first draft of the manuscript was written by YW and PG. XP, BN, and XZ critically revised the work. All authors contributed to the article and approved the submitted version.
Funding
This study was supported by grants from the National Natural Science Foundation of China (82072707), the Scientific Research Program of the Shanghai Municipal Commission of Science and Technology (19411970700 and 20Y11909400) and the Changhai Hospital 234 Project (2019YXK019 and 2020YXK029).
Conflict of interest
The authors declare that the research was conducted in the absence of any commercial or financial relationships that could be construed as a potential conflict of interest.
Publisher’s note
All claims expressed in this article are solely those of the authors and do not necessarily represent those of their affiliated organizations, or those of the publisher, the editors and the reviewers. Any product that may be evaluated in this article, or claim that may be made by its manufacturer, is not guaranteed or endorsed by the publisher.
References
1. Frei E, 3RD. Clinical cancer research: an embattled species. Cancer (1982) 50(10):1979–92. doi: 10.1002/1097-0142(19821115)50:10<1979::AID-CNCR2820501002>3.0.CO;2-D
2. Schuhmacher C, Gretschel S, Lordick F, Reichardt P, Hohenberger W, Eisenberger CF, et al. Neoadjuvant chemotherapy compared with surgery alone for locally advanced cancer of the stomach and cardia: European organisation for research and treatment of cancer randomized trial 40954. J Clin Oncol (2010) 28(35):5210–8. doi: 10.1200/JCO.2009.26.6114
3. Read RL, Flitcroft K, Snook KL, Boyle FM, Spillane AJ. Utility of neoadjuvant chemotherapy in the treatment of operable breast cancer. ANZ J Surg (2015) 85(5):315–20. doi: 10.1111/ans.12975
4. Saigusa S, Inoue Y, Tanaka K, Toiyama Y, Kawamura M, Okugawa Y, et al. Significant correlation between LKB1 and LGR5 gene expression and the association with poor recurrence-free survival in rectal cancer after preoperative chemoradiotherapy. J Cancer Res Clin Oncol (2013) 139(1):131–8. doi: 10.1007/s00432-012-1308-x
5. Salmenkylä S, Kouri M, Österlund P, Pukkala E, Luukkonen P, Hyöty M, et al. Does preoperative radiotherapy with postoperative chemotherapy increase acute side-effects and postoperative complications of total mesorectal excision? report of the randomized Finnish rectal cancer trial. Scand J Surg SJS Off Organ Finnish Surg Soc Scand Surg Soc (2012) 101(4):275–82. doi: 10.1177/145749691210100410
6. Hayes DF, Schott AF. Neoadjuvant chemotherapy: What are the benefits for the patient and for the investigator? JNCI Monographs (2015) 2015(51):36–9. doi: 10.1093/jncimonographs/lgv004
7. Kallergi G, Aggouraki D, Zacharopoulou N, Stournaras C, Georgoulias V, Martin SS. Evaluation of α-tubulin, detyrosinated α-tubulin, and vimentin in CTCs: identification of the interaction between CTCs and blood cells through cytoskeletal elements. Breast Cancer Res BCR (2018) 20(1):67. doi: 10.1186/s13058-018-0993-z
8. Hu Y, Hu D, Li W, Yu X. Neoadjuvant chemotherapy brings more survival benefits than postoperative chemotherapy for resectable gastric cancer: a meta-analysis of randomized controlled trials. J BUON: Off J Balkan Union Oncol (2019) 24(1):201–14.
9. Zhao Q, Li Y, Tian Y, Chen YN, Tan BB, Zhao XF, et al. Histological complete response after neoadjuvant XELOX in advanced gastric carcinoma. Hepato-gastroenterology (2013) 60(123):638–40. doi: 10.5754/hge121131
10. Oya Y, Hayakawa Y, Koike K. Tumor microenvironment in gastric cancers. Cancer Sci (2020) 111(8):2696–707. doi: 10.1111/cas.14521
11. Zeng D, Li M, Zhou R, Zhang J, Sun H, Shi M, et al. Tumor microenvironment characterization in gastric cancer identifies prognostic and immunotherapeutically relevant gene signatures. Cancer Immunol Res (2019) 7(5):737–50. doi: 10.1158/2326-6066.CIR-18-0436
12. Lo CS, Sanii S, Kroeger DR, Milne K, Talhouk A, Chiu DS, et al. Neoadjuvant chemotherapy of ovarian cancer results in three patterns of tumor-infiltrating lymphocyte response with distinct implications for immunotherapy. Clin Cancer Res (2017) 23(4):925–34. doi: 10.1158/1078-0432.CCR-16-1433
13. Dieci MV, Radosevic-Robin N, Fineberg S, van den Eynden G, Ternes N, Penault-Llorca F, et al. Update on tumor-infiltrating lymphocytes (TILs) in breast cancer, including recommendations to assess TILs in residual disease after neoadjuvant therapy and in carcinoma in situ: A report of the international immuno-oncology biomarker working group on breast cancer. Semin Cancer Biol (2018) 52(Pt 2):16–25. doi: 10.1016/j.semcancer.2017.10.003
14. Zhou SL, Zhou ZJ, Hu ZQ, Huang XW, Wang Z, Chen EB, et al. Tumor-associated neutrophils recruit macrophages and T-regulatory cells to promote progression of hepatocellular carcinoma and resistance to sorafenib. Gastroenterology (2016) 150(7):1646–58.e17. doi: 10.1053/j.gastro.2016.02.040
15. Song HJ, Srivastava A, Lee J, Kim YS, Kim KM, Ki Kang W, et al. Host inflammatory response predicts survival of patients with Epstein-Barr virus-associated gastric carcinoma. Gastroenterology (2010) 139(1):84–92.e2. doi: 10.1053/j.gastro.2010.04.002
16. Loeser H, Kraemer M, Gebauer F, Bruns C, Schröder W, Zander T, et al. Indoleamine 2,3-dioxygenase (IDO) expression is an independent prognostic marker in esophageal adenocarcinoma. J Immunol Res (2020) 2020:2862647. doi: 10.1155/2020/2862647
17. Peng H, Wu X, Zhong R, Yu T, Cai X, Liu J, et al. Profiling tumor immune microenvironment of non-small cell lung cancer using multiplex immunofluorescence. Front Immunol (2021) 12:750046. doi: 10.3389/fimmu.2021.750046
18. McCoy MJ, Hemmings C, Miller TJ, Austin SJ, Bulsara MK, Zeps N, et al. Low stromal Foxp3+ regulatory T-cell density is associated with complete response to neoadjuvant chemoradiotherapy in rectal cancer. Br J Cancer (2015) 113(12):1677–86. doi: 10.1038/bjc.2015.427
19. Nejati R, Goldstein JB, Halperin DM, Wang H, Hejazi N, Rashid A, et al. Prognostic significance of tumor-infiltrating lymphocytes in patients with pancreatic ductal adenocarcinoma treated with neoadjuvant chemotherapy. Pancreas (2017) 46(9):1180–7. doi: 10.1097/MPA.0000000000000914
20. Krysko DV, Garg AD, Kaczmarek A, Krysko O, Agostinis P, Vandenabeele P. Immunogenic cell death and DAMPs in cancer therapy. Nat Rev Cancer (2012) 12(12):860–75. doi: 10.1038/nrc3380
21. Galluzzi L, Humeau J, Buqué A, Zitvogel L, Kroemer G. Immunostimulation with chemotherapy in the era of immune checkpoint inhibitors. Nat Rev Clin Oncol (2020) 17(12):725–41. doi: 10.1038/s41571-020-0413-z
22. Kroemer G, Galluzzi L, Kepp O, Zitvogel L. Immunogenic cell death in cancer therapy. Annu Rev Immunol (2013) 31:51–72. doi: 10.1146/annurev-immunol-032712-100008
23. Hashimoto M, Kamphorst AO, Im SJ, Kissick HT, Pillai RN, Ramalingam SS, et al. CD8 T cell exhaustion in chronic infection and cancer: Opportunities for interventions. Annu Rev Med (2018) 69:301–18. doi: 10.1146/annurev-med-012017-043208
24. Van Der Leun AM, Thommen DS, Schumacher TN. CD8(+) T cell states in human cancer: insights from single-cell analysis. Nat Rev Cancer (2020) 20(4):218–32. doi: 10.1038/s41568-019-0235-4
25. Fridman WH, Pagès F, Sautès-Fridman C, Galon J. The immune contexture in human tumours: impact on clinical outcome. Nat Rev Cancer (2012) 12(4):298–306. doi: 10.1038/nrc3245
26. Hossain MA, Liu G, Dai B, Si Y, Yang Q, Wazir J, et al. Reinvigorating exhausted CD8(+) cytotoxic T lymphocytes in the tumor microenvironment and current strategies in cancer immunotherapy. Medicinal Res Rev (2021) 41(1):156–201. doi: 10.1002/med.21727
27. Chen DS, Mellman I. Oncology meets immunology: the cancer-immunity cycle. Immunity (2013) 39(1):1–10. doi: 10.1016/j.immuni.2013.07.012
28. Farhood B, Najafi M, Mortezaee K. CD8(+) cytotoxic T lymphocytes in cancer immunotherapy: A review. J Cell Physiol (2019) 234(6):8509–21. doi: 10.1002/jcp.27782
29. Zhao Y, Shao Q, Peng G. Exhaustion and senescence: two crucial dysfunctional states of T cells in the tumor microenvironment. Cell Mol Immunol (2020) 17(1):27–35. doi: 10.1038/s41423-019-0344-8
30. Schietinger A, Greenberg PD. Tolerance and exhaustion: defining mechanisms of T cell dysfunction. Trends Immunol (2014) 35(2):51–60. doi: 10.1016/j.it.2013.10.001
31. Lichtenegger FS, Rothe M, Schnorfeil FM, Deiser K, Krupka C, Augsberger C, et al. Targeting LAG-3 and PD-1 to enhance T cell activation by antigen-presenting cells. Front Immunol (2018) 9:385. doi: 10.3389/fimmu.2018.00385
32. Sharma P, Allison JP. Immune checkpoint targeting in cancer therapy: toward combination strategies with curative potential. Cell (2015) 161(2):205–14. doi: 10.1016/j.cell.2015.03.030
33. Thommen DS, Schreiner J, Müller P, Herzig P, Roller A, Belousov A, et al. Progression of lung cancer is associated with increased dysfunction of T cells defined by coexpression of multiple inhibitory receptors. Cancer Immunol Res (2015) 3(12):1344–55. doi: 10.1158/2326-6066.CIR-15-0097
34. Ye J, Peng G. Controlling T cell senescence in the tumor microenvironment for tumor immunotherapy. Oncoimmunology (2015) 4(3):e994398. doi: 10.4161/2162402X.2014.994398
35. Tay RE, Richardson EK, Toh HC. Revisiting the role of CD4(+)T cells in cancer immunotherapy-new insights into old paradigms. Cancer Gene Ther (2021) 28(1-2):5–17. doi: 10.1038/s41417-020-0183-x
36. Borst J, Ahrends T, Babala N, Melief CJM, Kastenmueller W. CD4(+) T cell help in cancer immunology and immunotherapy. Nat Rev Immunol (2018) 18(10):635–47. doi: 10.1038/s41577-018-0044-0
37. Ahrends T, Borst J. The opposing roles of CD4(+) T cells in anti-tumour immunity. Immunology (2018) 154(4):582–92. doi: 10.1111/imm.12941
38. Becht E, Giraldo NA, Germain C, de Reyniès A, Laurent-Puig P, Zucman-Rossi J, et al. Immune contexture, immunoscore, and malignant cell molecular subgroups for prognostic and theranostic classifications of cancers. Adv Immunol (2016) 130:95–190. doi: 10.1016/bs.ai.2015.12.002
39. Hernandez-Segura A, Nehme J, Demaria M. Hallmarks of cellular senescence. Trends Cell Biol (2018) 28(6):436–53. doi: 10.1016/j.tcb.2018.02.001
40. Braumuller H, Wieder T, Brenner E, Assmann S, Hahn M, Alkhaled M, et al. T-helper-1-cell cytokines drive cancer into senescence. Nature (2013) 494(7437):361–5. doi: 10.1038/nature11824
41. Lin X, Ye L, Wang X, Liao Z, Dong J, Yang Y, et al. Follicular helper T cells remodel the immune microenvironment of pancreatic cancer via secreting CXCL13 and IL-21. Cancers (2021) 13(15):3678. doi: 10.3390/cancers13153678
42. Lahl K, Loddenkemper C, Drouin C, Freyer J, Arnason J, Eberl G, et al. Selective depletion of Foxp3(+) regulatory T cells induces a scurfy-like disease. J Exp Med (2007) 204(1):57–63. doi: 10.1084/jem.20061852
43. Cortez JT, Montauti E, Shifrut E, Gatchalian J, Zhang YS, Shaked O, et al. CRISPR screen in regulatory T cells reveals modulators of Foxp3. Transplantation (2020) 104(7):1309–. doi: 10.1038/s41586-020-2246-4
44. Guo X, Zhang Y, Zheng L, Zheng C, Song J, Zhang Q, et al. Global characterization of T cells in non-small-cell lung cancer by single-cell sequencing. Nat Med (2018) 24(10):1628–. doi: 10.1038/s41591-018-0045-3
45. Zhang L, Yu X, Zheng L, Zhang Y, Li Y, Fang Q, et al. Lineage tracking reveals dynamic relationships of T cells in colorectal cancer. Nature (2018) 564(7735):268. doi: 10.1038/s41586-018-0694-x
46. Wolf D, Sopper S, Pircher A, Gastl G, Wolf AM. Treg(s) in cancer: Friends or foe? J Cell Physiol (2015) 230(11):2598–605. doi: 10.1002/jcp.25016
47. Vignali DA, Collison LW, Workman CJ. How regulatory T cells work. Nat Rev Immunol (2008) 8(7):523–32. doi: 10.1038/nri2343
48. De Simone M, Arrigoni A, Rossetti G, Gruarin P, Ranzani V, Politano C, et al. Transcriptional landscape of human tissue lymphocytes unveils uniqueness of tumor-infiltrating T regulatory cells. Immunity (2016) 45(5):1135–47. doi: 10.1016/j.immuni.2016.10.021
49. Guillerey C, Huntington ND, Smyth MJ. Targeting natural killer cells in cancer immunotherapy. Nat Immunol (2016) 17(9):1025–36. doi: 10.1038/ni.3518
50. Hossain F, Al-Khami AA, Wyczechowska D, Hernandez C, Zheng L, Reiss K, et al. Inhibition of fatty acid oxidation modulates immunosuppressive functions of myeloid-derived suppressor cells and enhances cancer therapies. Cancer Immunol Res (2015) 3(11):1236–47. doi: 10.1158/2326-6066.CIR-15-0036
51. Böttcher JP, Bonavita E, Chakravarty P, Blees H, Cabeza-Cabrerizo M, Sammicheli S, et al. NK cells stimulate recruitment of cDC1 into the tumor microenvironment promoting cancer immune control. Cell (2018) 172(5):1022–37.e14. doi: 10.1016/j.cell.2018.01.004
52. Sivori S, Pende D, Quatrini L, Pietra G, Della Chiesa M, Vacca P, et al. NK cells and ILCs in tumor immunotherapy. Mol aspects Med (2021) 80:100870. doi: 10.1016/j.mam.2020.100870
53. Habif G, Crinier A, André P, Vivier E, Narni-Mancinelli E. Targeting natural killer cells in solid tumors. Cell Mol Immunol (2019) 16(5):415–22. doi: 10.1038/s41423-019-0224-2
54. Terrén I, Orrantia A, Vitallé J, Zenarruzabeitia O, Borrego F. NK cell metabolism and tumor microenvironment. Front Immunol (2019) 10:2278. doi: 10.3389/fimmu.2019.02278
55. Zhang Q, Bi J, Zheng X, Chen Y, Wang H, Wu W, et al. Blockade of the checkpoint receptor TIGIT prevents NK cell exhaustion and elicits potent anti-tumor immunity. Nat Immunol (2018) 19(7):723. doi: 10.1038/s41590-018-0132-0
56. Nair S, Dhodapkar MV. Natural killer T cells in cancer immunotherapy. Front Immunol (2017) 8:1178. doi: 10.3389/fimmu.2017.01178
57. Terabe M, Berzofsky JA. Tissue-specific roles of NKT cells in tumor immunity. Front Immunol (2018) 9:1838. doi: 10.3389/fimmu.2018.01838
58. Izhak L, Ambrosino E, Kato S, Parish ST, O'Konek JJ, Weber H, et al. Delicate balance among three types of T cells in concurrent regulation of tumor immunity [J]. Cancer Res (2013) 73(5):1514–23. doi: 10.1158/0008-5472.CAN-12-2567
59. Hinshaw DC, Shevde LA. The tumor microenvironment innately modulates cancer progression [J]. Cancer Res (2019) 79(18):4557–66. doi: 10.1158/0008-5472.CAN-18-3962
60. Krijgsman D, Hokland M, Kuppen PJK. The role of natural killer T cells in cancer-a phenotypical and functional approach. Front Immunol (2018) 9:367. doi: 10.3389/fimmu.2018.00367
61. Wculek SK, Cueto FJ, Mujal AM, Melero I, Krummel MF, Sancho D. Dendritic cells in cancer immunology and immunotherapy. Nat Rev Immunol (2020) 20(1):7–24. doi: 10.1038/s41577-019-0210-z
62. Batista FD, Harwood NE. The who, how and where of antigen presentation to b cells. Nat Rev Immunol (2009) 9(1):15–27. doi: 10.1038/nri2454
63. Melaiu O, Chierici M, Lucarini V, Jurman G, Conti LA, De Vito R, et al. Cellular and gene signatures of tumor-infiltrating dendritic cells and natural-killer cells predict prognosis of neuroblastoma. Nat Commun (2020) 11(1):5992. doi: 10.1038/s41467-020-19781-y
64. Kießler M, Plesca I, Sommer U, Wehner R, Wilczkowski F, Müller L, et al. Tumor-infiltrating plasmacytoid dendritic cells are associated with survival in human colon cancer. J immunother Cancer (2021) 9(3):e001813. doi: 10.1136/jitc-2020-001813
65. Fridlender ZG, Sun J, Kim S, Kapoor V, Cheng G, Ling L, et al. Polarization of tumor-associated neutrophil phenotype by TGF-beta: "N1" versus "N2" TAN. Cancer Cell (2009) 16(3):183–94. doi: 10.1016/j.ccr.2009.06.017
66. Motta JM, Rumjanek VM. Sensitivity of dendritic cells to microenvironment signals. J Immunol Res (2016) 2016:4753607. doi: 10.1155/2016/4753607
67. Tran Janco JM, Lamichhane P, Karyampudi L, Knutson KL. Tumor-infiltrating dendritic cells in cancer pathogenesis. J Immunol (Baltimore Md 1950) (2015) 194(7):2985–91. doi: 10.4049/jimmunol.1403134
68. Kim J, Bae JS. Tumor-associated macrophages and neutrophils in tumor microenvironment. Mediators Inflammation (2016) 2016:6058147. doi: 10.1155/2016/6058147
69. Wang N, Liu W, Zheng Y, Wang S, Yang B, Li M, et al. CXCL1 derived from tumor-associated macrophages promotes breast cancer metastasis via activating NF-κB/SOX4 signaling. Cell Death Dis (2018) 9(9):880. doi: 10.1038/s41419-018-0876-3
70. Cardoso AP, Pinto ML, Pinto AT, Oliveira MI, Pinto MT, Gonçalves R, et al. Macrophages stimulate gastric and colorectal cancer invasion through EGFR Y(1086), c-src, Erk1/2 and akt phosphorylation and smallGTPase activity. Oncogene (2014) 33(16):2123–33. doi: 10.1038/onc.2013.154
71. Chen H, Shi R, Luo B, Yang X, Qiu L, Xiong J, et al. Macrophage peroxisome proliferator-activated receptor γ deficiency delays skin wound healing through impairing apoptotic cell clearance in mice. Cell Death Dis (2015) 6(1):e1597. doi: 10.1038/cddis.2014.544
72. Yang L, Zhang Y. Tumor-associated macrophages: from basic research to clinical application. J Hematol Oncol (2017) 10:58. doi: 10.1186/s13045-017-0430-2
73. Aras S, Zaidi MR. TAMeless traitors: macrophages in cancer progression and metastasis. Br J Cancer (2017) 117(11):1583–91. doi: 10.1038/bjc.2017.356
74. Narayanan S, Kawaguchi T, Yan L, Peng X, Qi Q, Takabe K. Tumor infiltrating lymphocytes and macrophages improve survival in microsatellite unstable colorectal cancer. Ann Surg Oncol (2018) 25:S63–S. doi: 10.1038/s41598-019-49878-4
75. Peiseler M, Kubes P. Macrophages play an essential role in trauma-induced sterile inflammation and tissue repair. Eur J Trauma Emergency Surg Off Publ Eur Trauma Soc (2018) 44(3):335–49. doi: 10.1007/s00068-018-0956-1
76. Varol C, Mildner A, Jung S. Macrophages: development and tissue specialization. Annu Rev Immunol (2015) 33:643–75. doi: 10.1146/annurev-immunol-032414-112220
77. Qin L, Wu YL, Toneff MJ, Li D, Liao L, Gao X, et al. NCOA1 directly targets m-CSF1 expression to promote breast cancer metastasis. Cancer Res (2014) 74(13):3477–88. doi: 10.1158/0008-5472.CAN-13-2639
78. Pathria P, Louis TL, Varner JA. Targeting tumor-associated macrophages in cancer. Trends Immunol (2019) 40(4):310–27. doi: 10.1016/j.it.2019.02.003
79. Wang J, Jia Y, Wang N, Zhang X, Tan B, Zhang G, et al. The clinical significance of tumor-infiltrating neutrophils and neutrophil-to-CD8+ lymphocyte ratio in patients with resectable esophageal squamous cell carcinoma. J Trans Med (2014) 12:7. doi: 10.1186/1479-5876-12-7
80. Kolaczkowska E, Kubes P. Neutrophil recruitment and function in health and inflammation. Nat Rev Immunol (2013) 13(3):159–75. doi: 10.1038/nri3399
81. Tecchio C, Scapini P, Pizzolo G, Cassatella MA. On the cytokines produced by human neutrophils in tumors. Semin Cancer Biol (2013) 23(3):159–70. doi: 10.1016/j.semcancer.2013.02.004
82. Tecchio C, Cassatella MA. Neutrophil-derived chemokines on the road to immunity. Semin Immunol (2016) 28(2):119–28. doi: 10.1016/j.smim.2016.04.003
83. Fridlender ZG, Albelda SM. Tumor-associated neutrophils: friend or foe? Carcinogenesis (2012) 33(5):949–55. doi: 10.1093/carcin/bgs123
84. Li S, Cong X, Gao H, Lan X, Li Z, Wang W, et al. Tumor-associated neutrophils induce EMT by IL-17a to promote migration and invasion in gastric cancer cells. J Exp Clin Cancer Res CR (2019) 38(1):6. doi: 10.1186/s13046-018-1003-0
85. Gabrilovich DI, Nagaraj S. Myeloid-derived suppressor cells as regulators of the immune system. Nat Rev Immunol (2009) 9(3):162–74. doi: 10.1038/nri2506
86. Gabrilovich DI, Ostrand-Rosenberg S, Bronte V. Coordinated regulation of myeloid cells by tumours. Nat Rev Immunol (2012) 12(4):253–68. doi: 10.1038/nri3175
87. Bronte V, Brandau S, Chen SH, Colombo MP, Frey AB, Greten TF, et al. Recommendations for myeloid-derived suppressor cell nomenclature and characterization standards [J]. Nat Commun (2016) 7:12150. doi: 10.1038/ncomms12150
88. Condamine T, Dominguez GA, Youn JI, Kossenkov AV, Mony S, Alicea-Torres K, et al. Lectin-type oxidized LDL receptor-1 distinguishes population of human polymorphonuclear myeloid-derived suppressor cells in cancer patients. Sci Immunol (2016) 1(2):aaf8943. doi: 10.1126/sciimmunol.aaf8943
89. Gabrilovich DI. Myeloid-derived suppressor cells. Cancer Immunol Res (2017) 5(1):3–8. doi: 10.1158/2326-6066.CIR-16-0297
90. Veglia F, Sanseviero E, Gabrilovich DI. Myeloid-derived suppressor cells in the era of increasing myeloid cell diversity. Nat Rev Immunol (2021) 21(8):485–98. doi: 10.1038/s41577-020-00490-y
91. Laumont CM, Banville AC, Gilardi M, Hollern DP, Nelson BH. Tumour-infiltrating b cells: immunological mechanisms, clinical impact and therapeutic opportunities. Nat Rev Cancer (2022) 22(7):414–30. doi: 10.1038/s41568-022-00466-1
92. Sharonov GV, Serebrovskaya EO, Yuzhakova DV, Britanova OV, Chudakov DM. B cells, plasma cells and antibody repertoires in the tumour microenvironment. Nat Rev Immunol (2020) 20(5):294–307. doi: 10.1038/s41577-019-0257-x
93. Bruno TC, Ebner PJ, Moore BL, Squalls OG, Waugh KA, Eruslanov EB, et al. Antigen-presenting intratumoral b cells affect CD4(+) TIL phenotypes in non-small cell lung cancer patients. Cancer Immunol Res (2017) 5(10):898–907. doi: 10.1158/2326-6066.CIR-17-0075
94. Sautès-Fridman C, Petitprez F, Calderaro J, Fridman WH. Tertiary lymphoid structures in the era of cancer immunotherapy. Nat Rev Cancer (2019) 19(6):307–25. doi: 10.1038/s41568-019-0144-6
95. Garaud S, Buisseret L, Solinas C, Gu-Trantien C, de Wind A, Van den Eynden G, et al. Tumor-infiltrating b cells signal functional humoral immune responses in breast cancer. JCI Insight (2019) 4(18):e129641. doi: 10.1172/jci.insight.129641
96. Rosser EC, Mauri C. Regulatory b cells: origin, phenotype, and function. Immunity (2015) 42(4):607–12. doi: 10.1016/j.immuni.2015.04.005
97. Sarvaria A, Madrigal JA, Saudemont A. B cell regulation in cancer and anti-tumor immunity. Cell Mol Immunol (2017) 14(8):662–74. doi: 10.1038/cmi.2017.35
98. Yu Y, Ma X, Zhang Y, Zhang Y, Ying J, Zhang W, et al. Changes in expression of multiple checkpoint molecules and infiltration of tumor immune cells after neoadjuvant chemotherapy in gastric cancer. J Cancer (2019) 10(12):2754–63. doi: 10.7150/jca.31755
99. Xing X, Shi J, Jia Y, Dou Y, Li Z, Dong B, et al. Effect of neoadjuvant chemotherapy on the immune microenvironment in gastric cancer as determined by multiplex immunofluorescence and T cell receptor repertoire analysis. J immunother Cancer (2022) 10(3):e003984. doi: 10.1136/jitc-2021-003984
100. Wei Q, Xu Q, Yuan X, Li JJ, Chen L, Luo C, et al. Immunological impact of chemotherapy on the tumor microenvironment in gastric cancer. J Surg Oncol (2021) 123(8):1708–15. doi: 10.1002/jso.26449
101. Hu M, Li K, Maskey N, Xu Z, Peng C, Wang B, et al. Decreased intratumoral Foxp3 tregs and increased dendritic cell density by neoadjuvant chemotherapy associated with favorable prognosis in advanced gastric cancer. Int J Clin Exp Pathol (2014) 7(8):4685–94.
102. Hoffmann A, Behrens HM, Heckl S, Krüger S, Becker T, Röcken C. Neoadjuvant/Perioperative treatment affects spatial distribution and densities of tumor associated neutrophils and CD8+ lymphocytes in gastric cancer. J personalized Med (2021) 11(11):1184. doi: 10.3390/jpm11111184
103. Li Z, Gao X, Peng X, May Chen MJ, Li Z, Wei B, et al. Multi-omics characterization of molecular features of gastric cancer correlated with response to neoadjuvant chemotherapy. Sci Adv (2020) 6(9):eaay4211. doi: 10.1126/sciadv.aay4211
104. Maskey N, Li K, Hu M, Xu Z, Peng C, Yu F, et al. Impact of neoadjuvant chemotherapy on lymphocytes and co-inhibitory B7-H4 molecule in gastric cancer: low B7-H4 expression associates with favorable prognosis. Tumour Biol J Int Soc Oncodevelopmental Biol Med (2014) 35(12):11837–43. doi: 10.1007/s13277-014-2410-2
105. Hennequin A, Derangère V, Boidot R, Apetoh L, Vincent J, Orry D, et al. Tumor infiltration by tbet+ effector T cells and CD20+ b cells is associated with survival in gastric cancer patients. Oncoimmunology (2016) 5(2):e1054598. doi: 10.1080/2162402X.2015.1054598
106. Christina Svensson M, Lindén A, Nygaard J, Borg D, Hedner C, Nodin B, et al. T Cells, b cells, and PD-L1 expression in esophageal and gastric adenocarcinoma before and after neoadjuvant chemotherapy: relationship with histopathological response and survival. Oncoimmunology (2021) 10(1):1921443. doi: 10.1080/2162402X.2021.1921443
107. Tsuchikawa T, Miyamoto M, Yamamura Y, Shichinohe T, Hirano S, Kondo S. The immunological impact of neoadjuvant chemotherapy on the tumor microenvironment of esophageal squamous cell carcinoma. Ann Surg Oncol (2012) 19(5):1713–9. doi: 10.1245/s10434-011-1906-x
108. Fukuoka E, Yamashita K, Tanaka T, Sawada R, Sugita Y, Arimoto A, et al. Neoadjuvant chemotherapy increases PD-L1 expression and CD8(+) tumor-infiltrating lymphocytes in esophageal squamous cell carcinoma. Anticancer Res (2019) 39(8):4539–48. doi: 10.21873/anticanres.13631
109. Jomrich G, Kollmann D, Ramazanova D, Ristl R, Grose RP, Ilhan-Mutlu A, et al. Expression of programmed cell death protein 1 (PD-1) and programmed cell death 1 ligand (PD-L1) in adenocarcinomas of the gastroesophageal junction change significantly after neoadjuvant treatment. Eur J Surg Oncol J Eur Soc Surg Oncol Br Assoc Surg Oncol (2022) 48(2):383–90. doi: 10.1016/j.ejso.2021.08.016
110. Wu MY, Shen M, Xu MD, Yu ZY, Tao M. FOLFIRINOX regulated tumor immune microenvironment to extend the survival of patients with resectable pancreatic ductal adenocarcinoma. Gland Surg (2020) 9(6):2125–35. doi: 10.21037/gs-20-828
111. Michelakos T, Cai L, Villani V, Sabbatino F, Kontos F, Fernández-Del Castillo C, et al. Tumor microenvironment immune response in pancreatic ductal adenocarcinoma patients treated with neoadjuvant therapy. J Natl Cancer Institute (2021) 113(2):182–91. doi: 10.1093/jnci/djaa073
112. Mota Reyes C, Teller S, Muckenhuber A, Konukiewitz B, Safak O, Weichert W, et al. Neoadjuvant therapy remodels the pancreatic cancer microenvironment via depletion of protumorigenic immune cells. Clin Cancer Res (2020) 26(1):220–31. doi: 10.1158/1078-0432.CCR-19-1864
113. Hane Y, Tsuchikawa T, Nakamura T, Hatanaka KC, Saito T, Tanaka K, et al. Immunological gene signature associated with the tumor microenvironment of pancreatic cancer after neoadjuvant chemotherapy. Pancreas (2020) 49(9):1240–5. doi: 10.1097/MPA.0000000000001665
114. Farren MR, Sayegh L, Ware MB, Chen HR, Gong J, Liang Y, et al. Immunologic alterations in the pancreatic cancer microenvironment of patients treated with neoadjuvant chemotherapy and radiotherapy. JCI Insight (2020) 5(1):e130362. doi: 10.1172/jci.insight.130362
115. Matsutani S, Shibutani M, Maeda K, Nagahara H, Fukuoka T, Nakao S, et al. Significance of tumor-infiltrating lymphocytes before and after neoadjuvant therapy for rectal cancer. Cancer Sci (2018) 109(4):966–79. doi: 10.1111/cas.13542
116. Imaizumi K, Suzuki T, Kojima M, Shimomura M, Sakuyama N, Tsukada Y, et al. Ki67 expression and localization of T cells after neoadjuvant therapies as reliable predictive markers in rectal cancer. Cancer Sci (2020) 111(1):23–35. doi: 10.1111/cas.14223
117. Qi J, Liu X, Yan P, He S, Lin Y, Huang Z, et al. Analysis of immune landscape reveals prognostic significance of cytotoxic CD4(+) T cells in the central region of pMMR CRC. Front Oncol (2021) 11:724232. doi: 10.3389/fonc.2021.724232
118. Huang KC, Chiang SF, Chen WT, Chen TW, Hu CH, Yang PC, et al. Decitabine augments chemotherapy-induced PD-L1 upregulation for PD-L1 blockade in colorectal cancer. Cancers (2020) 12(2):462. doi: 10.3390/cancers12020462
119. Jary M, Liu WW, Yan D, Bai I, Muranyi A, Colle E, et al. Immune microenvironment in patients with mismatch-repair-proficient oligometastatic colorectal cancer exposed to chemotherapy: the randomized MIROX GERCOR cohort study. Mol Oncol (2021) 16(11):2260–73. doi: 10.1002/1878-0261.13173
120. Dosset M, Vargas TR, Lagrange A, Boidot R, Végran F, Roussey A, et al. PD-1/PD-L1 pathway: an adaptive immune resistance mechanism to immunogenic chemotherapy in colorectal cancer. Oncoimmunology (2018) 7(6):e1433981. doi: 10.1080/2162402X.2018.1433981
121. Dekker E, Tanis PJ, Vleugels JLA, Kasi PM, Wallace MB. Colorectal cancer. Lancet (London England) (2019) 394(10207):1467–80. doi: 10.1016/S0140-6736(19)32319-0
122. Wu Y, Yang S, Ma J, Chen Z, Song G, Rao D, et al. Spatiotemporal immune landscape of colorectal cancer liver metastasis at single-cell level. Cancer Discovery (2022) 12(1):134–53. doi: 10.1158/2159-8290.CD-21-0316
123. Ledys F, Klopfenstein Q, Truntzer C, Arnould L, Vincent J, Bengrine L, et al. RAS status and neoadjuvant chemotherapy impact CD8+ cells and tumor HLA class I expression in liver metastatic colorectal cancer. J immunother Cancer (2018) 6(1):123. doi: 10.1186/s40425-018-0438-3
124. Dagenborg VJ, Marshall SE, Yaqub S, Grzyb K, Boye K, Lund-Iversen M, et al. Neoadjuvant chemotherapy is associated with a transient increase of intratumoral T-cell density in microsatellite stable colorectal liver metastases. Cancer Biol Ther (2020) 21(5):432–40. doi: 10.1080/15384047.2020.1721252
125. Galle PR, Forner A, Llovet JM, Mazzaferro V, Piscaglia F, Raoul JL, et al. EASL clinical practice guidelines: Management of hepatocellular carcinoma. J Hepatol (2018) 69(1):182–236. doi: 10.1016/j.jhep.2018.03.019
126. Tampaki M, Ionas E, Hadziyannis E, Deutsch M, Malagari K, Koskinas J. Association of TIM-3 with BCLC stage, serum PD-L1 detection, and response to transarterial chemoembolization in patients with hepatocellular carcinoma. Cancers (2020) 12(1):212. doi: 10.3390/cancers12010212
127. Pinato DJ, Murray SM, Forner A, Kaneko T, Fessas P, Toniutto P, et al. Trans-arterial chemoembolization as a loco-regional inducer of immunogenic cell death in hepatocellular carcinoma: implications for immunotherapy. J immunother Cancer (2021) 9(9):e003311. doi: 10.1136/jitc-2021-003311
128. Graeser M, Feuerhake F, Gluz O, Volk V, Hauptmann M, Jozwiak K, et al. Immune cell composition and functional marker dynamics from multiplexed immunohistochemistry to predict response to neoadjuvant chemotherapy in the WSG-ADAPT-TN trial. J immunother Cancer (2021) 9(5):e002198. doi: 10.1136/jitc-2020-002198
129. Park YH, Lal S, Lee JE, Choi YL, Wen J, Ram S, et al. Chemotherapy induces dynamic immune responses in breast cancers that impact treatment outcome. Nat Commun (2020) 11(1):6175. doi: 10.1038/s41467-020-19933-0
130. Takakura H, Domae S, Ono T, Sasaki A. The immunological impact of chemotherapy on the tumor microenvironment of oral squamous cell carcinoma. Acta Med Okayama (2017) 71(3):219–26. doi: 10.18926/AMO/55204
131. Gaudreau PO, Negrao MV, Mitchell KG, Reuben A, Corsini EM, Li J, et al. Neoadjuvant chemotherapy increases cytotoxic T cell, tissue resident memory T cell, and b cell infiltration in resectable NSCLC. J Thorac Oncol Off Publ Int Assoc Study Lung Cancer (2021) 16(1):127–39. doi: 10.1016/j.jtho.2020.09.027
132. Böhm S, Montfort A, Pearce OM, Topping J, Chakravarty P, Everitt GL, et al. Neoadjuvant chemotherapy modulates the immune microenvironment in metastases of tubo-ovarian high-grade serous carcinoma. Clin Cancer Res (2016) 22(12):3025–36. doi: 10.1158/1078-0432.CCR-15-2657
133. Mesnage SJL, Auguste A, Genestie C, Dunant A, Pain E, Drusch F, et al. Neoadjuvant chemotherapy (NACT) increases immune infiltration and programmed death-ligand 1 (PD-L1) expression in epithelial ovarian cancer (EOC). Ann Oncol Off J Eur Soc Med Oncol (2017) 28(3):651–7. doi: 10.1093/annonc/mdw625
134. Leary A, Genestie C, Blanc-Durand F, Gouy S, Dunant A, Maulard A, et al. Neoadjuvant chemotherapy alters the balance of effector to suppressor immune cells in advanced ovarian cancer. Cancer immunol immunother CII (2021) 70(2):519–31. doi: 10.1007/s00262-020-02670-0
135. Zhang Y, Yu M, Jing Y, Cheng J, Zhang C, Cheng L, et al. Baseline immunity and impact of chemotherapy on immune microenvironment in cervical cancer [J]. Br J Cancer (2021) 124(2):414–24. doi: 10.1038/s41416-020-01123-w
Keywords: tumor immune microenvironment, tumor-infiltrating immune cells, neoadjuvant chemotherapy, immunotherapy, prognostic value
Citation: Wang Y, Gao P, Hao Z, Chen L, Li X, Jiao Y, Liu J, Li J, Zhang Y, Peng X, Ning B and Zhan X (2022) The effect of neoadjuvant chemotherapy on the tumor immune microenvironment in gastrointestinal tumors. Front. Oncol. 12:1054598. doi: 10.3389/fonc.2022.1054598
Received: 27 September 2022; Accepted: 21 October 2022;
Published: 08 November 2022.
Edited by:
Jian Zhang, Fourth Military Medical University, ChinaReviewed by:
Xiuliang Cui, Beijing Institute of Pharmacology and Toxicology, ChinaMaria Alejandra Garcia Marquez, University Hospital of Cologne, Germany
Copyright © 2022 Wang, Gao, Hao, Chen, Li, Jiao, Liu, Li, Zhang, Peng, Ning and Zhan. This is an open-access article distributed under the terms of the Creative Commons Attribution License (CC BY). The use, distribution or reproduction in other forums is permitted, provided the original author(s) and the copyright owner(s) are credited and that the original publication in this journal is cited, in accordance with accepted academic practice. No use, distribution or reproduction is permitted which does not comply with these terms.
*Correspondence: Xiaobo Peng, cHhiMDIzQDEyNi5jb20=; Beifang Ning, bmluZ2JlaWZhbmdAMTYzLmNvbQ==; Xianbao Zhan, emhhbnhpYW5iYW9AY3Njby5vcmcuY24=
†These authors have contributed equally to this work