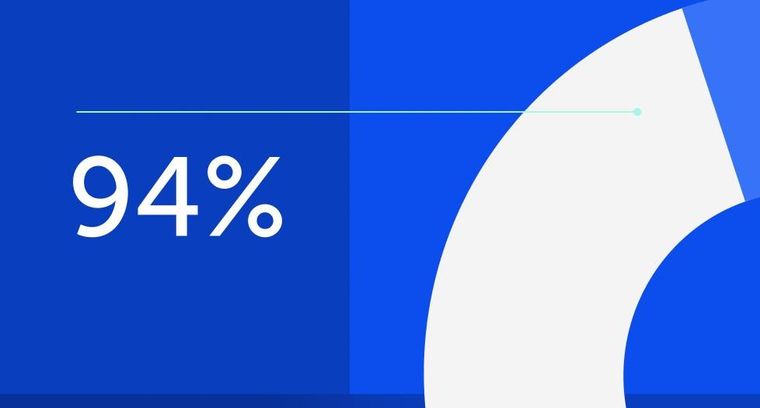
94% of researchers rate our articles as excellent or good
Learn more about the work of our research integrity team to safeguard the quality of each article we publish.
Find out more
REVIEW article
Front. Oncol., 03 August 2021
Sec. Radiation Oncology
Volume 11 - 2021 | https://doi.org/10.3389/fonc.2021.704664
This article is part of the Research TopicWomen in Radiation Oncology: 2021View all 22 articles
In the last years, extensive investigation on miRNomics have shown to have great advantages in cancer personalized medicine regarding diagnosis, treatment and even clinical outcomes. Prostate cancer (PCa) is the second most common male cancer and about 50% of all PCa patients received radiotherapy (RT), despite some of them develop radioresistance. Here, we aim to provide an overview on the mechanisms of miRNA biogenesis and to discuss the functional impact of miRNAs on PCa under radiation response. As main findings, 23 miRNAs were already identified as being involved in genetic regulation of PCa cell response to RT. The mechanisms of radioresistance are still poorly understood, despite it has been suggested that miRNAs play an important role in cell signaling pathways. Identification of miRNAs panel can be thus considered an upcoming and potentially useful strategy in PCa diagnosis, given that radioresistance biomarkers, in both prognosis and therapy still remains a challenge.
Small non-protein-coding RNA molecules, composed of around 22 nucleotides, are commonly named as miRNAs (1–3). Briefly, miRNAs are expected to account for 1-5% of the human genome and to interfere with at least 30% of the protein-coding genes (4, 5). The first miRNA was discovered in 1993 by Lee, Freinbaum and Ambros (6, 7), and since then an increasing load of literature data have pointed that they can act as both tumor suppressors and oncogenes (1–3). Indeed, it has been shown that miRNAs play an important role in gene expression, mainly when associated with the monitoring of several cell and metabolic pathways, being also an essential component of the gene silencing machinery in most eukaryotic organisms (4, 8).
In recent years, many studies have confirmed the involvement of miRNAs in biological processes of several types of cancer (4, 9, 10). The relationship between miRNAs and cancer was demonstrated for the first time in 2002, with miRNAs being stated as a potential mechanism that may contribute to improve some cancer therapeutic approaches through restoring or blocking the miRNAs function (11). Among the various types of cancer with increasing prevalence nowadays, prostate cancer (PCa) is the second most common in male and the fifth leading cause of death in men. Based on Global Cancer Observatory (GLOBOCAN) 2020, more than 1.4 million new cases of PCa and 375,304 associated deaths were recorded (12). One of the treatments applied in cancer is radiotherapy (RT), a therapeutic modality that uses ionizing radiation to induce damage in unwanted cells. The main goal of RT consists in delivering a precise dose of radiation in a target volume, such as tumor, promoting the tumor cells eradication with as minimal damage as possible in surrounding normal tissues (13). Currently, RT is one of the most often used therapeutic approaches in PCa patients, featured by several levels of complexity (13, 14), with around 50% of all PCa patients receiving RT at some stage of treatment, while 10–45% of PCa cases are resistant to irradiation (15, 16). Besides the RT dose is standardized among patients, local recurrences are common and can occur even when modern techniques are used (17). Patient’s local recurrences appear mostly as a result of uncontrolled cell reproduction and unregulated cancer cells growth that invades and interferes with the normal function of surrounding tissues and organs (1). Various regulatory factors and genes have shown to be able to directly modulate cell cycle, differentiation and even death. For instance, tumor-suppressor genes or oncogenes or both are regulatory factors able to modulate the environmental conditions contributing to cancer development (2, 4, 18). In this way, miRNAs may be viewed as promising biomarkers capable of predicting radiation response and to develop a customized treatment for each patient, ultimately opening a new therapeutic window for personalized intervention in PCa patients.
Therefore, in the present work, we aimed to explore the mechanisms of miRNA biogenesis, the role of miRNAs in cancer, and the functional impact of miRNAs on PCa radiation response, towards to provide a detailed review of the miRNA expression signatures in PCa tumor and cell lines with therapeutic impact in RT.
For data selection ‘PICOS Worksheet and Search Strategy’ was followed. A detailed and careful literature search was done using PubMED and Web of Science databases. For the Boolean search combinations, the terms used were “miRNA AND prostate cancer OR prostate neoplasia OR prostate carcinoma AND radiotherapy OR radiation therapy”, resulting in 71 papers from PubMED and 46 from “Web of Science”. Inclusion criteria include articles written in English, published between January 2000 and June 2021, and works referred to clinical studies and pre-clinical studies with cell lines and animal models. Exclusion criteria include not repeat articles on different databases, articles not available and papers that employ non-conventional RT, that with focus on radiotoxicity, and papers on radio sensitization-related biomarkers applied for either diagnostic or prognostic purposes. Then, these 117 articles were analyzed by independent researchers, and 54 articles were selected for further analysis, with 63 articles being eliminated because did not fulfil with the inclusion criteria. Papers related to other malignancies in addition to PCa and those in whom was unable to obtain the whole data were also excluded (Figure 1).
miRNA biogenesis is controlled at multiple steps, and transcriptional regulation has been proposed to be the major mechanism controlling tissue and cell type-specific expression of miRNAs (4, 19). Briefly, the miRNAs biogenesis includes their transcription at cell nucleus, export to the cytoplasm and subsequent processing and maturation (20), with two processes being involved in achieving the mature miRNA: canonical or non-canonical biogenesis of miRNA (Figure 2).
Figure 2 Simplified overview of the canonical and non-canonical miRNA biogenesis pathways. MiRNA generated by canonical pathway is transcribed by RNA polymerase II to a primary transcript called pre-miRNA. Subsequently, this structure is processed into the nucleus by DGCR8/Drosha complex; producing the pre-miRNA, which is exported to the cytoplasm by exportin-5. In the cytoplasm, pre-miRNA is cleaved by RNase III endonuclease (Dicer protein), resulting in a double stranded miRNA, which contains the mature miRNA. After, double strand is separated by helicases and create the mature miRNA; on the contrary, in non-canonical pathways, miRNA located within of mirtrons and are associated with spliceosome-dependent mechanisms. After, the mature miRNA produced by different pathways enters into the RISC complex and regulated genes through messenger RNA (mRNA) degradation and mRNA translation repression.
In the canonical pathway, the miRNA gene is typically transcribed by RNA polymerase II to generate long primary transcripts (pre-miRNA) in the nucleus. Subsequently, the pre-miRNA is processed by RNA polymerase III (Drosha protein) and DiGeorge syndrome critical region gene 8 (DGCR8) protein, thus producing the pre-miRNA, a double-stranded miRNA of variable length with approximately 18-25 nucleotides (4, 5, 19, 21, 22). The resulting structure is exported to the cytoplasm via Exportin-5 and RanGTP (5, 19, 21, 23). In the cytoplasm, pre-miRNA is cleaved by the Dicer protein to create a duplex miRNA, which contains the mature miRNA. When the duplex unwinds, both RNA strands are separated by helicases and the resulting mature miRNA is incorporated into a functional ribonucleoprotein complex, called RNA-Induced Silencing Complex (RISC), while the other strand is degraded (4, 9, 19). RISC is a ribonucleoprotein complex composed by a set of proteins linked to a small molecule of RNA and it is responsible to perform cell surveillance, inhibiting the translation of the gene into a protein through enzymatic destruction, which effectively silences the gene (5). Both miRNA and RISC complex (miRISC) regulate gene expression through two mechanisms: messenger RNA (mRNA) degradation and mRNA translation repression (22, 23).
Non-canonical pathway is an alternative biogenesis pathway, where miRNA is associated with spliceosome-dependent mechanisms (19). In this pathway, the miRNAs located within the introns of coding or non-coding genes of proteins (“mirtrons”) enter in the miRNA processing pathway without Drosha-mediated cleavage (23).
miRNAs can be used in cancer diagnosis to improve the treatment planning and therapeutic sensitivity, to prevent the occurrence of several medications-associated side effects and toxicity, and even to monitor treatment (Figure 3). Accumulating evidence underline that miRNAs have an extensive impact due to their involvement in cancer hallmarks, and thus has been considered an important therapeutic target in cancer management (24).
Figure 3 Scheme of the potential of miRNAs in personalized prostate cancer. MiRNAs are present in biological samples, which could be a useful tool for diagnosis and staging in the first consult, allowing an accurate risk stratification. Based on information collected, the treatment can be planned. Treatments can be personalized according to radioresistance or radiosensitive of cancer. If cancer is radioresistance, radical prostatectomy is the therapeutic approach more indicated. Otherwise, RT is ideal treatment to apply to radiosensitive tumors. Also, miRNA signature can give information about the risk of develop side effects or if the patient is responding or not to treatment, leading to a better tumor control with reduced side effects, which contribute to a better patient quality life.
In PCa, there is a dysregulation in miRNAs expression, which can modulate the expression of oncogenes and tumor suppressor genes (20, 22, 23, 25). Moreover, treatment resistance is still a huge problem, so that miRNAs modulation therapy could be a new therapeutic target in cancer patients and used to monitor the therapeutic responses, besides could be useful to predict response to therapies, such chemotherapy and RT (20, 22, 23, 26). The miRNAs therapeutic board approaches include oligonucleotides, small artificial molecules and miRNA-mediated virus or non-virus transfection. In the case of oligonucleotides and small artificial molecules they could be used to inhibit miRNAs or to interfere indirectly with other transcription factors or target genes associated with miRNA-specific modulation. Consequently, several methods have been examined using anti-sense oligonucleotides and it is expected that someday they will be safely implemented (27, 28). Other promissory strategy includes the downregulation of miRNAs using a miRNA-mediated virus or non-virus transfection methods that increases the targeted miRNA. Several studies are being carried out on such matter, taking into account the introduction of artificial double-stranded miRNA – mimic of targeted downregulated miRNA (18).
At that time, biological samples, such as blood, serum and urine, allow to classify the cancer risk at same time that provide prognostic data, allowing to address the cancer aggressiveness, predisposition to metastization or development of radio/chemoresistance. Moreover, depending on the cancer risk, an active surveillance or some specific treatments should be recommended. In the last case, miRNAs can help to predict the response to radiation and the likelihood of side effects’ occurrence. So, miRNA expression provides new insights if treatment is being the most appropriate, and if not, treatment must be changed or adjusted (Figure 4). Regarding side effects, changes in miRNAs expression can be used to overcome these toxicities or to understand their signs before the need to interrupt the therapy with a possible impairment in therapeutics results (29–31).
Figure 4 Scheme illustrates different microRNA therapy approaches – anti-microRNA therapy (A) and mimicking microRNA therapy (B) to change miRNA-regulated gene expression. In therapy (A) the microRNA inhibitors are transfected to cells and suppressing onco-microRNA functions and consequently increased protein production. In contrast, in therapy (B) are introduced microRNA mimetics to target cells where interact with tumor suppressor microRNA target, suppressing of protein production.
Currently, the adoption and promotion of personalized therapy has been increasingly notorious, and it is even considered essential in multiple clinical conditions. Specifically, there is increasing evidence underlining those miRNAs can influence the way that cells respond to ionizing radiation, making them more radiosensitive or radioresistant through several specific pathways. These include modifying DNA repair pathways which interfere with cell cycle checkpoints activation, tumor microenvironment and apoptosis. Some miRNAs are involved in controlling cell cycle progression, tumor microenvironment, apoptosis, and radio-related signals pathway (32–34).
In the context of tumor microenvironment, miRNAs have aroused a high interest. MiRNAs play an important role in regulating tumor radiation response, which involve DNA repair, epithelial-to-mesenchymal transition (EMT), and stemness (35–39). However, radio resistance is a complex phenomenon, and thus more studies are needed to better understand such processes. The response rates to radiation differ according to the modality used, namely the way through which radiation is delivered, the dose of radiation used, tumor stage/grade, confounding medical co-morbidities, and intrinsic tumor microenvironment (40, 41).
As mentioned above, miRNAs can be employed in therapeutic approaches to mimic or inhibit gene expression at translation level - Figure 4 (42, 43). In the first approach, if the miRNA is under expressed, it can be restored by adding miRNA. In the second approach, if the miRNA is overexpressed, artificial anti-miRNAs can be added to block miRNA (29, 33, 42). Preclinical studies have shown that the use of miRNAs is well-tolerated without triggering significant adverse effects. However, it is necessary to improve both the efficiency and targeted delivery to the tumor before treating patients (15).
To the best of authors’ knowledge, only few clinical trials have investigated the miRNA expression profile induced by RT in PCa patients (Figure 5). For example, Zedan et al. measured miRNA-21, miRNA-93, miRNA‐125b, and miRNA‐221 levels in plasma from PCa patients. Among other aspects the authors verified that miRNA-221 and miRNA-93 transcription decreased in patients’ plasma following RT, being thus radiosensitive (44, 45). Also, Linuma et al., in a study where low-dose rate prostate brachytherapy (BT) was applied to PCa patients, they stated that miRNA-93 was significantly downregulated in extracellular vesicles from patients’ serum after BT (34). In addition, miRNA-145 expression was analyzed in tumor tissue of 30 PCa patients and it was suggested that miRNA-145 can improve response to RT reducing the efficiency of the repair of radiation-induced DNA double-strand breaks (DSB). Thus, when miRNA-145 is overexpressed, PCa cells are sensitized to ionizing radiation (46).
Figure 5 Scheme illustration on the use of miRNAs as a therapeutic strategy in prostate cancer (PCa) cell lines. MiRNAs can be divided into mimic or antagomiR and some have also revealed to be useful in clinics.
Other study verified an upregulation of miRNA-95 in 9 tissue specimens of PCa patients, related to radiation resistance by targeting sphingosine-1-phosphatase 1 (SGPP1) (47). Ambs and colleagues analyzed the miRNA-106b-25 cluster expression in 60 primary PCa and 16 non-tumor PCa tissues and concluded that this miRNA has high levels of expression in primary PCa tissues compared to non-tumor prostate tissues (48). In contrast, miRNA-1272 was found downregulated in PCa tissues (49).
Two other studies investigated miRNAs in extracellular vesicles as markers of therapeutic efficacy. Li et al. found a panel of 9 serum-derived extracellular vesicles-miRNAs (miRNA-200c-3p, miRNA-323-3p, miRNA-379-5p, miRNA-409-3p, miRNA-411-5p, miRNA-493-5p, miRNA-494-3p, miRNA-543, and miRNA-654-3p) with potential to predict the therapeutic benefit of carbon ion RT. Additionally, miRNA-654-3p in serum exosomes was considered a potential non-invasive biomarker to predict the efficacy of carbon ion RT in PCa (50). Likewise, Malla et al. collected 25 serum-derived extracellular vesicles-miRNAs from patients treated with RT. Five miRNAs were identified (let-7a-5p, miRNA-141-3p, miRNA-145-5p, miRNA-21-5p, and miRNA-99b-5p), but only let-7a-5p and miRNA-21-5p were overexpressed in high-risk PCa patients after RT.
More recently, miRNA-541-3p was studied in 33 PCa tissues and normal adjacent tissues before and after RT treatments. Interestingly, He et al. found that miRNA-541-3p enhances the radiosensitivity of PCa by inhibiting HSP27 expression and downregulating β-catenin (51).
However, further studies need to be carried out to confirm the miRNAs potential as new outcome biomarkers for PCa patients, as well as to validate the results already obtained, namely through larger patients’cohorts, due to the small sample size of studies-derived data available to date.
The Radiation Therapy Oncology Group (RTOG) has performed several potential trials in the field of RT, exploring tissue-based molecular biomarkers with predictive or prognostic value.
More recently, Croce et al. revealed data on the miRNAs potential in cancer, and then other studies demonstrated that the ectopic modulation of specific miRNAs can influence the cancer hallmarks by deregulating its mechanisms (52, 53). In comparison with invasive methods, miRNAs, whose origin seems to be specific from tissue, are very stable and directly detectable in circulating biofluids (54). Also, miRNAs can be isolated and purified from serum, plasma, urine, saliva, peripheral blood cells, among other biological samples (55). Also, miRNAs can circulate in the interstitial fluids and bloodstream through membrane-bound vesicles, such as exosomes (50–90 nm) and microvesicles (1 μm), and even in non-vesicles, such as the ribonucleoprotein complex, which corresponds to the main mechanism. Indeed, accumulating evidence identified circulating miRNAs in apoptotic bodies, exosomes, high-density lipoprotein, and RNA binding proteins as a form of a cell-to-cell communication channel (56).
Circulating miRNA are evolutionarily conserved across species and can be measured easily and efficiently using real time quantitative protein chain reaction (RT-qPCR), microarray platforms, nanostring techniques, next-generation sequencing (NGS) and biosensors (56). Furthermore, evidence reveals that tumor-associated signature of miRNAs allows to discriminate different cancer subtypes and pathologies by using high-quality measurement techniques. Such finding can significantly contribute to the selection of a more efficient therapeutic approach (57, 58). Indeed, since miRNAs are involved in different cancer mechanisms, they can also be used in targeted therapy, however it continuous to be a challenge regarding stability of miRNAs and its tissue specificity and permeability (55). With the technological advance, such as in the field of nanotechnology, and with the raise in miRNA research, it is expected that, in the future, one of the therapeutic approaches for cancer may be the administration of synthetic anti-sense or mimics oligonucleotides (59, 60).
Several studies have shown the clinical usefulness of some miRNAs and their potential in therapeutic efficacy of RT (54, 61–63). Some miRNAs exhibit predictive value regarding the treatment response through sample analysis extracted from non-invasive liquid biopsies. Thus, miRNAs can give relevant data to achieve a proper patient therapeutic monitoring, as they promote an early detection of PCa relapse/progression, ultimately providing a better control of cancer (44). To underlined that this ability to predict whether a patient is responsive or nonresponsive to a particular treatment modality will allow the expansion of personalized medicine, with individual and personalized treatments being selected for a particular patient, avoiding the risks of toxicity, side effects and relapses. Moreover, “real-time” monitoring of miRNAs may provide an early identification of patients who are failing to radiation therapy response, offering the opportunity to try a more efficient alternative treatment (64). In 2008, it was published the first evidence of a miRNA signature that changed the response to RT (65). Subsequently, increasing evidence has been generated with the intent of discovering an “universal” miRNA molecular profile.
The interaction of ionizing radiation with cells induces some biological responses, including direct DNA damage from ionization or indirectly by ROS generation. Then, different pathways are activated in an intent to repair the damaged DNA, induce cell cycle arrest or even cell death (66). As stated above, RT induce damages, including single-strand breaks (SSB) and DSB. These breaks can be restored by DNA repair pathways, such as base excision repair (BER), nucleotide excision repair (NER), mismatch Repair (MMR), nonhomologous end-joining (NHEJ) or homologous recombination (HR) (67). But radiation can also change the miRNA expression and consequently alters the levels of associated proteins. Most of these studies have been done in vitro using PCa cell lines, such as, PC3, DU145, LNCaP and 22Rv1.
MiRNA are involved in the management of such different cell processes (Figure 6). For example, MiRNA-99a, a member of miRNA-99 family, and miRNA-100 have a role in DNA repair. The inhibition of this miRNAs will prevent p53 dependent apoptosis, increasing the recruitment of DNA repair proteins (BRCA1, RAD51), consequently influencing SWI/SNF-related matrix-associated actin-dependent regulator of chromatin subfamily A member 5 (SMARCA5) and spinal muscular atrophy with respiratory distress type 1 (SMARD1) in LNCaP, PC3 and DU145 cells after irradiation exposure (68, 69).
Figure 6 Overview of miRNAs involved on DNA damage repair, cell cycle arrest, and cell death induced by ionizing radiation. Thus, photon beams cause DNA damage directly or indirectly by reactive oxygen species (ROS). In order to repair DNA damage, the cell activate DNA damage response pathways including nucleotide excision repair (NER), non-homologous end joining (NHEJ) and homologous recombination (HR). Additionally, miRNAs regulated cell cycle progression to allow DNA damage repair and depends on cyclin dependent kinases (CDKs), cyclins and transcription factors family EF2. Also, miRNAs are influenced by several factors in the tumor microenvironment such as hypoxia and epithelial mesenchymal transition (EMT) and play an important role in biological processes as apoptosis and autophagy. Consequently, hypoxia promotes DNA repair by transcription of the androgen receptor expression. AKT, Protein kinase B; CDC25A, Cell division cycle 25 A; G1 and G2, transition phases of the cell cycle; HSP27, Heat shock protein 27; M, Mitosis; PTEN, Phosphatase and TENsin homolog; Rb, Retinoblastoma protein; RXRA, Retinoid X receptor alpha; S, phase S; SGGP1, Sphingosine-1-phosphate phosphatase 1. Black inhibition line displays direct targeting; black dashed-inhibition line displays indirect targeting; arrow display an induction of tumor microenvironment.
Josson et al. reported that miRNA-521 modulates the radio sensitivity of LNCaP cells by specifically restoring DNA repair protein, Cockayne syndrome protein A (CSA) and manganese superoxide dismutase (MnSOD), an anti-apoptotic enzyme. If miRNA-521 is overexpressed it will further sensitize cells to RT contributing to a raise in RT efficacy (65).
Furthermore, miRNA-890 is downregulated in LNCaP, PC3 and DU145 cells and targets mitotic pathways composed of several regulators, including mitotic arrested deficient 2 like 2 (MAD2L2), WEE1 kinase, xeroderma pigmentosum complementation group C (XPC), and KU80 proteins. Also, Hatano et al. revealed that miRNA-744-3p can directly influence RAD23 Homolog B, Nucleotide Excision Repair Protein (RAD23B) in LNCaP, PC3 and DU145 cells. Both miRNAs are involved in DDR systems induced by irradiation, such as DNA DSB repair and NER pathways (70, 71). El Bezawy et al. mentioned that miRNA-875-3p inhibits HR pathway in PC3 and DU145 cells by controlling checkpoint kinase 1 (CHK1) expression and zinc finger E-box-binding homeobox (ZEB), which have impact on EMT (72).
Similarly, there are some miRNAs involved in cell cycle arrest. MicroRNA–16–5p is located at chromosome 13q14 and it is downregulated in LNCaP cells. Wang et al. showed that this miRNA is a tumor suppressor and is involved in PCa onset. The overexpression of miRNA-16-5p is linked to cell proliferation suppression and modulates the Cyclin D1/E1-pRb-E2F1 pathway, inducing G0/G1 phase arrest after irradiation, which consequently increase the radio sensitivity in LNCaP cells (34).
In G2/M phase, elevated levels of miRNA-95 promote radio resistance in PC3 cells. The target of this miRNA is associated with SGPP1, an antagonist of sphingosine-1-phosphate signaling (S1P) that is responsible to protect against ionizing radiation-induced cell death. Briefly, SGPP1 suppresses the G2/M checkpoint, while increases proliferation, invasiveness, and the migratory capabilities of cancer cells (47, 73).
Also, miRNA-106 has been implicated in several pathways involved in raising the PCa cells radioresistance. Hoey et al. analyzed miRNA-106a and concluded that it is overexpressed in PC3 and DU145 cells and is significantly increased in high-grade than low-to-intermediate-grade cancer. The miRNA-106a targets lipopolysaccharide-induced TNF-α factor (LITAF), which is responsible to confer a radioresistant phenotype that increases cell survival and proliferation after irradiation (74). Li et al. showed that miRNA-106b have a novel role in RT due to its involvement in p21-activated cell cycle arrest regulation. Therefore, an inhibitory approach with addition of anti-miRNA-106b may reduce the miRNA-106b levels and will then change the p21 levels. After irradiation, a marked decreased in miRNA-106b expression was also stated in LNCaP cells (48, 73, 75), along with a correlation between miRNA-106b and Caspase-7 (76, 77).
Mao et al. showed that miRNA-449a targets c-Myc in LNCaP, PC3 and DU145 cells, which controls cdc2/Cyclin B1 cell cycle signal. This miRNA also enhances radiation-induced growth inhibition, radiation-induced G2/M arrest, and apoptosis by modulating the Cdc25A/Rb/E2F1 pathway. Likewise, c-Myc, which controls Cdc25A expression, is a miRNA449a target and is involved in PCa progression and its expression decreases after cells are submitted to radiation. So, when miRNA-449a is overexpressed, it promotes radio sensitivity in vitro by triggering destabilization and decreasing the expression of c-Myc and increasing both G2/M arrest and apoptosis (78, 79).
Moreover, miRNA-191 was correlated with radiation response in vitro and in vivo. Normally, this miRNA is overexpressed in PCa and it was related with radiation resistance through interaction with a novel target, retinoid X receptor alpha (RXRA) in PCa cell lines (PC3 and DU45). Low levels of RXRA expression was linked with a higher risk of distant relapse following RT. Mechanistically, miR-191 also effects cell cycle distribution and proliferation, reducing G2-M phase arrest post-radiation (80).
More recently, miRNA-107 has been related with radiation response of PCa. Lo et al. found that miRNA-107 regulated granulin and is downregulated in response to ionizing radiation in PC3 cells. MiRNA-107 was downregulated in PCa cells and tissues in comparison with normal prostate cells, but when is overexpressed, blocked granulin and promoted the radiosensitivity in PC3 cells. Mechanistically, miR-107 induced G1/S phase arrest and G2/M phase transit. Besides, also enhancing delayed apoptosis through suppression of p21 and CHK2-phosphorylation (81).
According to Duan et al., miR-498 is linked to PCa cells proliferation, radio sensitivity, invasion, and migration. After exposure to ionizing radiation, this miRNA is under expressed and induces radiation resistance in LNCaP and DU145 cells by reducing radiation-induced apoptosis through BAX and Bcl-2 expression regulation (82). Additionally, miR-498 is related to an important cell cycle regulator, phosphatase and TENsin homolog (PTEN), that suppresses the protein kinase B (AKT) signaling pathway, inhibits cell cycle progression, and affects ionizing radiation-induced apoptosis triggered by caspase 3/7 activity. In addition, with PTEN and AKT inhibition, EMT changes through influence of a raised expression of vimentin and a decreased of E-cadherin (82). Also, delays in response to DNA damage trigger cell death through several mechanisms, such as apoptosis, senescence and autophagy (83). Hsu et al. suggested that miR-18a acts as an oncomiRNA in cancer progression and it is upregulated in 22Rv1, PC3, LNCaP and DU145 cells. MiR-18a is related to STK4, a pro-apoptotic kinase that mediated AKT apoptosis cascade by phosphorylate Caspase 9 and Bad (84). In addition, Yang et al. showed that miR-18a were modulated by growth arrest-specific 5 (GAS5), which protects from radiation and promotes apoptosis when low expressed (85).
Recently, miR-541-3p has been investigated in radiation response in PCa tissue samples and cell lines. MiR-541-3p has low expression in PCa tissues, however, when submitted to RT is overexpressed in PCa cells (LNCaP, DU-145, PC3, and PrEC). Thus, using the mimic approach, miRNA-541-3p interacted directly with HSP27 and increased the radiosensitivity by enhanced apoptosis (51).
In a loss-of-function setting, miR-541-3p knockdown increased the proliferative potential and decreased the apoptotic rate of irradiated cells, ultimately reducing cell radiosensitivity. Conversely, miR-541-3p overexpression by miRNA mimic increased cell sensitivity as a result of a reduction in cell viability and colony formation, paralleled by increased apoptosis. Mechanistically, HSP27, validated as a direct target of the miRNA, was proposed as the potential mediator of miR-541-3p-induced radiosensitization, as suggested by rescue experiments showing a partial reversion of miRNA biological effects upon HSP27 ectopic overexpression.
Also, miR-29b expression or deletion was observed in tumor tissues and cell lines. Mao et al. demonstrated that miR-29b-3p improves radiation-induced cell apoptosis and sensitizes LNCaP cells to radiation by targeting Wnt1-inducible-signaling protein 1 (WISP1). Also, this miRNA was found to be a regulator of EMT and inhibits the PCa cells proliferation and invasion by controlling different targets, among them MCL-1, MMP-2, DNMT3B, and AKT3 (86).
MiRNA-19a was also analyzed in LNCaP, PC3 and DU145 cell lines and it was found downregulated in p53 positive radiosensitive LNCaP cells. Thus, it was suggested that miRNA-19a inhibition can provide a new therapeutic strategy for radioresistant PCa with mutated p53. This miRNA is related with prostate transmembrane protein, androgen-induced l(PMEPA1), and tumor protein p53 inducible nuclear protein 1 (TP53INP1) (87). Another miRNA, miRNA-17-3p was found at reduced amounts in PC3 cells and there have been some suggestions that this miRNA promotes carcinogenesis, by inhibition of mitochondrial antioxidant enzymes, such as manganese superoxide dismutase (MnSOD), glutathione, peroxidase 2 (Gpx2), and thioredoxin reductase 2 (Trx2) (88). In this context, Xu et al. provided a proof-of-concept evidence that miR-17-3p upregulation influences the radiotherapeutic efficiency through suppressing ionizing irradiation-mediated antioxidant responses, and in turn contributing to a raise in ROS level (89).
MiRNA-32 regulates DAB2 interacting protein (DAB2IP) and may contribute to the radioresistant PCa cells due to reduced ionizing radiation-induced cell apoptosis. Moreover, when this miRNA is overexpressed in LNCaP, PC3 and DU145 cells, it inhibits the expression of Bim protein, a pro-apoptotic member of the BCL-2 family and induces autophagy by targeting DAB2IP (48, 90). MiRNA-32 is also regulated by androgen and it has been implicated to another target gene, B-cell translocation gene 2 (BTG2), which is associated with PCa aggressiveness (91). Another functional study in DU145 and PC3 cell lines showed that miRNA‐124 or miRNA‐144 overexpression inhibit hypoxia‐induced autophagy and enhance radiosensitivity by regulating PIM1 (92).
A recent study on miR-1272 has revealed a relation with radio sensitivity of DU145 cells due to a consistent reduction of clonogenic cell survival mediated by miR-1272 upon irradiation. Authors transformed cells in a manner of gain-of-function using miR-1272 mimics. They found that besides reduced tumor growth and enhanced response to RT, miR-1272 affected the GFR/AKT/ERK1 pathways, ultimately affecting migration, invasiveness, and preventing EMT, all essential steps of the metastatic cascade (49).
Several studies have also shown that miRNA-145 overexpression sensitizes LNCaP and PC3 cells to ionizing radiation. This miRNA suppresses DNA (cytosine-5-)-methyltransferase 3 beta (DNMT3b), which have a crucial role in carcinogenesis, influencing PCa cells cycle, apoptosis, growth, and migration. Some data suggest that the overexpression of miRNA-145 can improve radio sensitivity through DNA DSB downregulation and directly targeting oncogenes (46, 65, 93). More recently, El Bezawy et al. described that miRNA-145 mimics can silence and deregulate the Speckle-type pox virus and zinc finger protein (POZ) protein (SPOP), causing an increase in PCa cells radio sensitivity by decreasing RAD51 and CHK1 expression and targeting ZEB1, that increases E-cadherin expression (94).
Another important feature linked to cancer cells is hypoxia, responsible for promoting tumor progression and the aggressive phenotype (95). In this sense, miRNA-301a and 301b, members of miRNA-301 family, also present clinical interest. It is known that miRNA-301a is an oncomir and it has been proposed that miRNA-301b can act as a tumor suppressor in LNCaP, PC3 and DU145 cells. However, in study of Wang et al. both these miRNAs were related to hypoxia and led to a decrease in autophagy in LNCaP, PC3 and DU145 cells by targeting N-myc downstream-regulated gene 2 (NDRG2). If these miRNAs are overexpressed, they may induce radio resistance in PCa cells by decreasing NDRG2 that suppresses EMT (96–98). Also, cancer change EMT. Several pathways have been clarified as involved in EMT deregulation, namely those linked to a control in transcription factors and epithelial specific markers, such as a decrease in cytokeratins and E-cadherin, and an increase in mesenchymal markers, such as fibronectin, N-cadherin, and vimentin (99). Indeed, El Bezawy et al. demonstrated that miRNA-875-5p is under expressed in PC3 and DU145 cells. MiRNA-875-5p is directly related to E-cadherin, with neutralization of EMT and improvement of radiation response through targeting epidermal growth factor receptor (EGFR), being these some of the major roles of E-cadherin. Also, it is involved in HR to repair DNA by regulating checkpoint kinase 1 (CHK1) expression and ZEB 1 (72, 100).
Also, MiR-34a and let-7 family (let-7a, let-7b, let-7c, let-7d, let-7e, let-7f, let-7g and let-7i) appeared upregulated following fractionated irradiation in LNCaP and PC3 cells, but not in DU145 cells. All these miRNAs are related to p53 gene, but only miRNA-34a has been proposed to be used as a radio sensitivity predictor, as it targets cyclin E2, besides to also interact with EMT (87, 101). Other studies suggest that miRNA-34 can be used to potentiate the therapeutic effect, as it is overexpressed in LNCaP and underexpressed in PC3 cell line (65, 102, 103). Also, other study should that let-7 family expression was downregulated in LNCaP, PC3 and DU145 cells and revealed to be able to regulate the expression of RAS oncogene, such KRAS and c-Myc (104). Furthermore, Dong et al. demonstrated that let-7a induced cell cycle arrest at the G1/S phase modulating the expression of E2F Transcription Factor 2 (E2F2) and G1/S-specific cyclin-D2 (CCND2) (105).
MiRNA-205 is under expressed and mediates autophagy, which is an important mechanism that can influence the LNCaP, PC3, DU145 cells radio sensitivity (106). Autophagy acts like a protective mechanism of PCa cells to stressful conditions, including radiation-induced cell apoptosis (107). Also, a potential direct functional target of miRNA-205 is tumor protein p53-inducible nuclear protein 1 (TP53INP1), which can interact with other protein families, such as Light chain 3 (LC3) and autophagy-related protein 8 (ATG8), thereby promoting autophagy and apoptosis targeting several cells signaling components, namely mitogen-activated protein kinase (MAPK) and androgen receptor (106, 107). Furthermore, miRNA-205 is also important to support the basal membrane in prostate epithelium, protein kinase C epsilon (PKCϵ) and ZEB1 expression, proteins involved in EMT (40). In the same context, miRNA-30a has been able to suppress autophagy and enhance radiosensitivity of PCa cells by targeting TP53INP1 (107).
Still related to autophagy, miRNA-195 is linked to PC3 and DU145 progression by targeting ribosomal protein S6 kinase B1(RPS6KB1), with its overexpression being responsible to enhance the RT efficacy through T cell by blocking the PD-L1 immune checkpoint, which is related to regulation of cytokines secretions in the tumor (108).
McDermott et al. showed that miR-4284 negatively regulates ring finger protein, LIM domain interacting (RLIM) and RasGEF domain family member 1A (RASGEF1A) genes. These genes are associated with RT resistance and oncogenesis. Authors also underlined that miR-4284 is down-regulated in RR-22Rv1 and AMC-22Rv1 cells, and stated a non-significant trend towards the acquisition of age-related radio resistance. Besides, another five miRNAs (miR-210, miR-23a, miR23b, miR-24, and miR-29) were identified in both hypoxic and isogenic radioresistant 22Rv1 models, when compared to the more radiosensitive WT-22Rv1 cell line (109).
Thus, a set of evidence in PCa treatment show that RT can significantly change the miRNA expression levels, but only a few studies investigate the impact of miRNA expression on radiation response in PCa (Table 1).
Anyway, and despite the accumulating evidence on this subject, it is important to mention that miRNA expression levels can be modified following PCa irradiation (29, 87, 111, 112). But, despite such alterations in miRNA expression patterns are inconsistent, even within the same cell line, because it largely depends on radiation dose and recovery time post-irradiation of cells (15), it is also a matter of high focus nowadays.
Despite the relative few numbers of studies exploiting the miRNAs relation with radiation response of PCa cells, this subject has been progressively explored and it continuous to be a challenge regarding the role of miRNAs as predictive markers for therapeutic targets.
One limitation of the miRNAs signature is linked to the inconsistency found among studies, mostly attributed to the methodologies applied: clinical trials/experimental studies, therapeutic conditions, pathology type, cell type, among others. Thus, to overcome this drawback, further studies must be designed to get high-quality, reproducible, and valid representative miRNAs to achieve results capable of promoting a patient-tailored treatment. Also worth of note is that most studies analyzed the potential role of miRNAs in vitro, so that new experiments should be done in vivo or in human tissue samples to support such findings. Thus, the selection of the most appropriate miRNAs remains a challenge.
In short, it has been shown that several miRNAs that modulated the cell response to ionizing radiation. Thus, miRNAs can be applied in therapy to reduce the radio resistance of cells through modulation of cell pathways and biological processes. However, larger and prospective studies are essential to define the value of miRNAs as therapeutic adjuvant to RT. Also, in this context, and since the number of studies is increasing, it is important to ensure a proper organization of data by creating databases of miRNA expressions for cancer research. In the future, we hope to find miRNA target relevant in daily clinical practice, with those capable of predicting the RT efficacy response being highly valuable in RT treatment management.
All authors have contributed equally to this work. All authors contributed to the article and approved the submitted version.
The authors declare that the research was conducted in the absence of any commercial or financial relationships that could be construed as a potential conflict of interest.
All claims expressed in this article are solely those of the authors and do not necessarily represent those of their affiliated organizations, or those of the publisher, the editors and the reviewers. Any product that may be evaluated in this article, or claim that may be made by its manufacturer, is not guaranteed or endorsed by the publisher.
The authors would like to acknowledge Fundação para a Ciência e Tecnologia by the PhD fellowship (SFRH/BD/138271/2018) for SS.
1. Esquela-Kerscher A, Slack FJ. Oncomirs - microRNAs With a Role in Cancer. Nat Rev Cancer (2006) 6(4):259–69. doi: 10.1038/nrc1840
2. Wang D, Qiu C, Zhang H, Wang J, Cui Q, Yin Y. Human microRNA Oncogenes and Tumor Suppressors Show Significantly Different Biological Patterns: From Functions to Targets. PLoS One (2010) 5(9):e13067. doi: 10.1371/journal.pone.0013067
3. Zhou K, Liu M, Cao Y. New Insight Into microRNA Functions in Cancer: Oncogene–microRNA–Tumor Suppressor Gene Network. Front Mol Biosci (2017) 4:46. doi: 10.3389/fmolb.2017.00046
4. MacFarlane LA, Murphy PR. MicroRNA: Biogenesis, Function and Role in Cancer. Curr Genomics (2010) 11(7):537–61. doi: 10.2174/138920210793175895
5. Wilson RC, Doudna JA. Molecular Mechanisms of RNA Interference. Annu Rev Biophys (2013) 42:217–39. doi: 10.1146/annurev-biophys-083012-130404
6. Sedwick C. Victor Ambros: The Broad Scope of microRNAs. J Cell Biol (2013) 201:492–3. doi: 10.1083/jcb.2014pi
7. Lee RC, Feinbaum RL, Ambros V. The C. Elegans Heterochronic Gene Lin-4 Encodes Small RNAs With Antisense Complementarity to Lin-14. Cell (1993) 75(5):843–54. doi: 10.1016/0092-8674(93)90529-Y
8. Achkar NP, Cambiagno DA, Manavella PA. miRNA Biogenesis: A Dynamic Pathway. Trends Plant Sci (2016) 21(12):1034–44. doi: 10.1016/j.tplants.2016.09.003
9. Lu M, Zhang Q, Deng M, Miao J, Guo Y, Gao W, et al. An Analysis of Human microRNA and Disease Associations. PLoS One (2008) 3(10):e3420. doi: 10.1371/journal.pone.0003420
10. Ling H, Fabbri M, Calin GA. MicroRNAs and Other Non-Coding RNAs as Targets for Anticancer Drug Development. Nat Rev Drug Discov (2013) 12(11):847–65. doi: 10.1038/nrd4140
11. Slaby O, Laga R, Sedlacek O. Therapeutic Targeting of Non-Coding RNAs in Cancer. Biochem J (2017) 474(24):4219–51. doi: 10.1042/BCJ20170079
12. Ferlay J, Ervik M, Lam F, Colombet M, Mery L, Piñeros M, et al. Global Cancer Observatory: Cancer Today. Lyon, France: International Agency for Research on Cancer (2020). Available at: http://gco.iarc.fr/today/home.
13. Perez C, Halperin E, Brady L. Principles and Practice of Radiation Oncology 5a edition. Philadelphia: Wolters Kluwer Health/Lippincott Williams & Wilkins (2008).
14. Brausi M, Hoskin P, Andritsch E, Banks I, Beishon M, Boyle H, et al. ECCO Essential Requirements for Quality Cancer Care: Prostate Cancer. Crit Rev Oncology/Hematol (2020) 148:102861. doi: 10.1016/j.critrevonc.2019.102861
15. Gandellini P, Rancati T, Valdagni R, Zaffaroni N. miRNAs in Tumor Radiation Response: Bystanders or Participants? Trends Mol Med (2014) 20(9):529–39. doi: 10.1016/j.molmed.2014.07.004
16. Xu M, Gong S, Li Y, Zhou J, Du J, Yang C, et al. Identifying Long Non-Coding RNA of Prostate Cancer Associated With Radioresponse by Comprehensive Bioinformatics Analysis. Front Oncol (2020) 10:498. doi: 10.3389/fonc.2020.00498
17. Fabris L, Ceder Y, Chinnaiyan AM, Jenster GW, Sorensen KD, Tomlins S, et al. The Potential of MicroRNAs as Prostate Cancer Biomarkers. Eur Urol (2016) 70(2):312–22. doi: 10.1016/j.eururo.2015.12.054
18. Christopher AF, Kaur RP, Kaur G, Kaur A, Gupta V, Bansal P. MicroRNA Therapeutics: Discovering Novel Targets and Developing Specific Therapy. Perspect Clin Res (2016) 7(2):68–74. doi: 10.4103/2229-3485.179431
19. Li Z, Rana TM. Therapeutic Targeting of microRNAs: Current Status and Future Challenges. Nat Rev Drug Discov (2014) 13(8):622–38. doi: 10.1038/nrd4359
20. Staedel C, Tran TPA, Giraud J, Darfeuille F, Di Giorgio A, Tourasse NJ, et al. Modulation of Oncogenic miRNA Biogenesis Using Functionalized Polyamines. Sci Rep (2018) 8(1):1667. doi: 10.1038/s41598-018-20053-5
21. Velagapudi SP, Vummidi BR, Disney MD. Small Molecule Chemical Probes of microRNA Function. Curr Opin Chem Biol (2015) 24:97–103. doi: 10.1016/j.cbpa.2014.10.024
22. Di Giorgio A, Tran TP, Duca M. Small-Molecule Approaches Toward the Targeting of Oncogenic miRNAs: Roadmap for the Discovery of RNA Modulators. Future Med Chem (2016) 8(7):803–16. doi: 10.4155/fmc-2016-0018
23. Iorio MV, Croce CM. microRNA Involvement in Human Cancer. Carcinogenesis (2012) 33(6):1126–33. doi: 10.1093/carcin/bgs140
24. Van Roosbroeck K, Calin GA. Cancer Hallmarks and MicroRNAs: The Therapeutic Connection. Adv Cancer Res (2017) 135:119–49. doi: 10.1016/bs.acr.2017.06.002
25. Gu LQ, Wanunu M, Wang MX, McReynolds L, Wang Y. Detection of miRNAs With a Nanopore Single-Molecule Counter. Expert Rev Mol Diagn (2012) 12(6):573–84. doi: 10.1586/erm.12.58
26. Rothschild SI. microRNA Therapies in Cancer. Mol Cell Ther (2014) 2:7. doi: 10.1186/2052-8426-2-7
27. Wen D, Danquah M, Chaudhary AK, Mahato RI. Small Molecules Targeting microRNA for Cancer Therapy: Promises and Obstacles. J Control Release (2015) 219:237–47. doi: 10.1016/j.jconrel.2015.08.011
28. Ishida M, Selaru FM. miRNA-Based Therapeutic Strategies. Curr Anesthesiol Rep (2013) 1(1):63–70. doi: 10.1007/s40139-012-0004-5
29. Cellini F, Morganti AG, Genovesi D, Silvestris N, Valentini V. Role of microRNA in Response to Ionizing Radiations: Evidences and Potential Impact on Clinical Practice for Radiotherapy. Molecules (2014) 19(4):5379–401. doi: 10.3390/molecules19045379
30. Kopcalic K, Petrovic N, Stanojkovic TP, Stankovic V, Bukumiric Z, Roganovic J, et al. Association Between miR-21/146a/155 Level Changes and Acute Genitourinary Radiotoxicity in Prostate Cancer Patients: A Pilot Study. Pathol Res Pract (2019) 215(4):626–31. doi: 10.1016/j.prp.2018.12.007
31. Balázs K, Antal L, Sáfrány G, Lumniczky K. Blood-Derived Biomarkers of Diagnosis, Prognosis and Therapy Response in Prostate Cancer Patients. J Pers Med (2021) 11(4):296. doi: 10.3390/jpm11040296
32. Zhao L, Lu X, Cao Y. MicroRNA and Signal Transduction Pathways in Tumor Radiation Response. Cell Signal (2013) 25(7):1625–34. doi: 10.1016/j.cellsig.2013.04.004
33. Korpela E, Vesprini D, Liu SK. MicroRNA in Radiotherapy: Mirage or Mirador? Br J Cancer (2015) 112(5):777–82. doi: 10.1038/bjc.2015.6
34. Wang F, Mao A, Tang J, Zhang Q, Yan J, Wang Y, et al. microRNA-16-5p Enhances Radiosensitivity Through Modulating Cyclin D1/E1-pRb-E2F1 Pathway in Prostate Cancer Cells. J Cell Physiol (2019) 234(8):13182–90. doi: 10.1002/jcp.27989
35. Xing F, Wu K, Watabe K. MicroRNAs in Cancer Stem Cells: New Regulators of Stemness. Curr Pharm Des (2014) 20(33):5319–27. doi: 10.2174/1381612820666140128210912
36. Muhammad N, Bhattacharya S, Steele R, Ray RB. Anti-miR-203 Suppresses ER-Positive Breast Cancer Growth and Stemness by Targeting SOCS3. Oncotarget (2016) 7(36):58595–605. doi: 10.18632/oncotarget.11193
37. Zhang P, Wang L, Rodriguez-Aguayo C, Yuan Y, Debeb BG, Chen D, et al. miR-205 Acts as a Tumour Radiosensitizer by Targeting ZEB1 and Ubc13. Nat Commun (2014) 5:5671. doi: 10.1038/ncomms6671
38. Yadav S, Kowolik CM, Lin M, Zuro D, Hui SK, Riggs AD, et al. SMC1A is Associated With Radioresistance in Prostate Cancer and Acts by Regulating Epithelial-Mesenchymal Transition and Cancer Stem-Like Properties. Mol Carcinog (2019) 58(1):113–25. doi: 10.1002/mc.22913
39. Mao A, Liu Y, Zhang H, Di C, Sun C. microRNA Expression and Biogenesis in Cellular Response to Ionizing Radiation. DNA Cell Biol (2014) 33(10):667–79. doi: 10.1089/dna.2014.2401
40. El Bezawy R, Tinelli S, Tortoreto M, Doldi V, Zuco V, Folini M, et al. miR-205 Enhances Radiation Sensitivity of Prostate Cancer Cells by Impairing DNA Damage Repair Through PKCepsilon and ZEB1 Inhibition. J Exp Clin Cancer Res (2019) 38(1):51. doi: 10.1186/s13046-019-1060-z
41. Palacios DA, Miyake M, Rosser CJ. Radiosensitization in Prostate Cancer: Mechanisms and Targets. BMC Urol (2013) 13:4. doi: 10.1186/1471-2490-13-4
42. Bai Z, Wei J, Yu C, Han X, Qin X, Zhang C, et al. Non-Viral Nanocarriers for Intracellular Delivery of microRNA Therapeutics. J Mater Chem B (2019) 7:1209–25. doi: 10.1039/C8TB02946F
43. Matin F, Jeet V, Clements JA, Yousef GM, Batra J. MicroRNA Theranostics in Prostate Cancer Precision Medicine. Clin Chem (2016) 62(10):1318–33. doi: 10.1373/clinchem.2015.242800
44. Zedan AH, Hansen TF, Assenholt J, Madsen JS, Osther PJS. Circulating miRNAs in Localized/Locally Advanced Prostate Cancer Patients After Radical Prostatectomy and Radiotherapy. Prostate (2019) 79(4):425–32. doi: 10.1002/pros.23748
45. Labbé M, Hoey C, Ray J, Potiron V, Supiot S, Liu SK, et al. microRNAs Identified in Prostate Cancer: Correlative Studies on Response to Ionizing Radiation. Mol Cancer (2020) 19(1):63. doi: 10.1186/s12943-020-01186-6
46. Gong P, Zhang T, He D, Hsieh JT. MicroRNA-145 Modulates Tumor Sensitivity to Radiation in Prostate Cancer. Radiat Res (2015) 184(6):630–8. doi: 10.1667/RR14185.1
47. Huang X, Taeb S, Jahangiri S, Emmenegger U, Tran E, Bruce J, et al. miRNA-95 Mediates Radioresistance in Tumors by Targeting the Sphingolipid Phosphatase SGPP1. Cancer Res (2013) 73(23):6972–86. doi: 10.1158/0008-5472.CAN-13-1657
48. Ambs S, Prueitt RL, Yi M, Hudson RS, Howe TM, Petrocca F, et al. Genomic Profiling of microRNA and Messenger RNA Reveals Deregulated microRNA Expression in Prostate Cancer. Cancer Res (2008) 68(15):6162–70. doi: 10.1158/0008-5472.CAN-08-0144
49. Rotundo F, Cominetti D, El Bezawy R, Percio S, Doldi V, Tortoreto M, et al. miR-1272 Exerts Tumor-Suppressive Functions in Prostate Cancer via HIP1 Suppression. Cells (2020) 9(2):435. doi: 10.3390/cells9020435
50. Yu Q, Li P, Weng M, Wu S, Zhang Y, Chen X, et al. Nano-Vesicles Are a Potential Tool to Monitor Therapeutic Efficacy of Carbon Ion Radiotherapy in Prostate Cancer. J BioMed Nanotechnol (2018) 14(1):168–78. doi: 10.1166/jbn.2018.2503
51. He Z, Shen F, Qi P, Zhai Z, Wang Z. miR-541-3p Enhances the Radiosensitivity of Prostate Cancer Cells by Inhibiting HSP27 Expression and Downregulating β-Catenin. Cell Death Discov (2021) 7(1):18. doi: 10.1038/s41420-020-00387-8
52. Peng Y, Croce CM. The Role of MicroRNAs in Human Cancer. Signal Transduct Targeted Ther (2016) 1(1):15004. doi: 10.1038/sigtrans.2015.4
53. Zaheer U, Faheem M, Qadri I, Begum N, Yassine HM, Al Thani AA, et al. Expression Profile of MicroRNA: An Emerging Hallmark of Cancer. Curr Pharm Des (2019) 25(6):642–53. doi: 10.2174/1386207322666190325122821
54. Małachowska B, Tomasik B, Stawiski K, Kulkarni S, Guha C, Chowdhury D, et al. Circulating microRNAs as Biomarkers of Radiation Exposure: A Systematic Review and Meta-Analysis. Int J Radiat OncologyBiologyPhys (2020) 106(2):390–402. doi: 10.1016/j.ijrobp.2019.10.028
55. Cui M, Wang H, Yao X, Zhang D, Xie Y, Cui R, et al. Circulating MicroRNAs in Cancer: Potential and Challenge. Front Genet (2019) 10:626–. doi: 10.3389/fgene.2019.00626
56. Singh VK, Pollard HB. Ionizing Radiation-Induced Altered microRNA Expression as Biomarkers for Assessing Acute Radiation Injury. Expert Rev Mol Diag (2017) 17(10):871–4. doi: 10.1080/14737159.2017.1366316
57. He Y, Lin J, Kong D, Huang M, Xu C, Kim T-K, et al. Current State of Circulating MicroRNAs as Cancer Biomarkers. Clin Chem (2015) 61(9):1138–55. doi: 10.1373/clinchem.2015.241190
58. Wang H, Peng R, Wang J, Qin Z, Xue L. Circulating microRNAs as Potential Cancer Biomarkers: The Advantage and Disadvantage. Clin Epigenet (2018) 10(1):1–10. doi: 10.1186/s13148-018-0492-1
59. Ganju A, Khan S, Hafeez BB, Behrman SW, Yallapu MM, Chauhan SC, et al. miRNA Nanotherapeutics for Cancer. Drug Discov Today (2017) 22(2):424–32. doi: 10.1016/j.drudis.2016.10.014
60. Goyal R, Kapadia CH, Melamed JR, Riley RS, Day ES. Layer-By-Layer Assembled Gold Nanoshells for the Intracellular Delivery of miR-34a. Cell Mol Bioeng (2018) 11(5):383–96. doi: 10.1007/s12195-018-0535-x
61. Filella X, Foj L. miRNAs as Novel Biomarkers in the Management of Prostate Cancer. Clin Chem Lab Med (2017) 55(5):715–36. doi: 10.1515/cclm-2015-1073
62. Doldi V, El Bezawy R, Zaffaroni N. MicroRNAs as Epigenetic Determinants of Treatment Response and Potential Therapeutic Targets in Prostate Cancer. Cancers (2021) 13(10):2380. doi: 10.3390/cancers13102380
63. Leung CM, Li SC, Chen TW, Ho MR, Hu LY, Liu WS, et al. Comprehensive microRNA Profiling of Prostate Cancer Cells After Ionizing Radiation Treatment. Oncol Rep (2014) 31(3):1067–78. doi: 10.3892/or.2014.2988
64. Mueller A-K, Lindner K, Hummel R, Haier J, Watson DI, Hussey DJ. MicroRNAs and Their Impact on Radiotherapy for Cancer. Radiat Res (2016) 185(6):668–77. doi: 10.1667/RR14370.1
65. Josson S, Sung SY, Lao K, Chung LW, Johnstone PA. Radiation Modulation of microRNA in Prostate Cancer Cell Lines. Prostate (2008) 68(15):1599–606. doi: 10.1002/pros.20827
66. Srinivas US, Tan BWQ, Vellayappan BA, Jeyasekharan AD. ROS and the DNA Damage Response in Cancer. Redox Biol (2019) 25:101084. doi: 10.1016/j.redox.2018.101084
67. Ford JM, Kastan MB. 11 - DNA Damage Response Pathways and Cancer. In: Niederhuber JE, Armitage JO, Kastan MB, Doroshow JH, Tepper JE, editors. Abeloff’s Clinical Oncology, Sixth Edition. Philadelphia: Elsevier (2020). p. 154–64.e4.
68. Rane JK, Erb HH, Nappo G, Mann VM, Simms MS, Collins AT, et al. Inhibition of the Glucocorticoid Receptor Results in an Enhanced miR-99a/100-Mediated Radiation Response in Stem-Like Cells From Human Prostate Cancers. Oncotarget (2016) 7(32):51965–80. doi: 10.18632/oncotarget.10207
69. Sun D, Lee YS, Malhotra A, Kim HK, Matecic M, Evans C, et al. miR-99 Family of microRNAs Suppresses the Expression of Prostate Specific Antigen and Prostate Cancer Cell Proliferation. Cancer Res (2011) 71(4):1313–24. doi: 10.1158/0008-5472.CAN-10-1031
70. Hatano K, Kumar B, Zhang Y, Coulter JB, Hedayati M, Mears B, et al. A Functional Screen Identifies miRNAs That Inhibit DNA Repair and Sensitize Prostate Cancer Cells to Ionizing Radiation. Nucleic Acids Res (2015) 43(8):4075–86. doi: 10.1093/nar/gkv273
71. Zhang M, Li H, Zhang Y. Oncogenic miR-744 Promotes Prostate Cancer Growth Through Direct Targeting of LKB1. Oncol Lett (2019) 17(2):2257–65. doi: 10.3892/ol.2018.9822
72. El Bezawy R, Cominetti D, Fenderico N, Zuco V, Beretta GL, Dugo M, et al. miR-875-5p Counteracts Epithelial-to-Mesenchymal Transition and Enhances Radiation Response in Prostate Cancer Through Repression of the EGFR-ZEB1 Axis. Cancer Lett (2017) 395:53–62. doi: 10.1016/j.canlet.2017.02.033
73. Razdan A, de Souza P, Roberts TL. Role of MicroRNAs in Treatment Response in Prostate Cancer. Curr Cancer Drug Targets (2018) 18(10):929–44. doi: 10.2174/1568009618666180315160125
74. Hoey C, Ray J, Jeon J, Huang X, Taeb S, Ylanko J, et al. miRNA-106a and Prostate Cancer Radioresistance: A Novel Role for LITAF in ATM Regulation. Mol Oncol (2018) 12(8):1324–41. doi: 10.1002/1878-0261.12328
75. Li B, Shi XB, Nori D, Chao CK, Chen AM, Valicenti R, et al. Down-Regulation of microRNA 106b is Involved in P21-Mediated Cell Cycle Arrest in Response to Radiation in Prostate Cancer Cells. Prostate (2011) 71(6):567–74. doi: 10.1002/pros.21272
76. Hudson RS, Yi M, Esposito D, Glynn SA, Starks AM, Yang Y, et al. MicroRNA-106b-25 Cluster Expression is Associated With Early Disease Recurrence and Targets Caspase-7 and Focal Adhesion in Human Prostate Cancer. Oncogene (2013) 32(35):4139–47. doi: 10.1038/onc.2012.424
77. Eron SJ, Raghupathi K, Hardy JA. Dual Site Phosphorylation of Caspase-7 by PAK2 Blocks Apoptotic Activity by Two Distinct Mechanisms. Structure (2017) 25(1):27–39. doi: 10.1016/j.str.2016.11.001
78. Mao A, Zhao Q, Zhou X, Sun C, Si J, Zhou R, et al. MicroRNA-449a Enhances Radiosensitivity by Downregulation of C-Myc in Prostate Cancer Cells. Sci Rep (2016) 6:27346. doi: 10.1038/srep27346
79. Mao A, Liu Y, Wang Y, Zhao Q, Zhou X, Sun C, et al. miR-449a Enhances Radiosensitivity Through Modulating pRb/E2F1 in Prostate Cancer Cells. Tumour Biol (2016) 37(4):4831–40. doi: 10.1007/s13277-015-4336-8
80. Ray J, Haughey C, Hoey C, Jeon J, Murphy R, Dura-Perez L, et al. miR-191 Promotes Radiation Resistance of Prostate Cancer Through Interaction With RXRA. Cancer Lett (2020) 473:107–17. doi: 10.1016/j.canlet.2019.12.025
81. Lo H-C, Hsu J-H, Lai L-C, Tsai M-H, Chuang EY. MicroRNA-107 Enhances Radiosensitivity by Suppressing Granulin in PC-3 Prostate Cancer Cells. Sci Rep (2020) 10(1):14584–. doi: 10.1038/s41598-020-71128-1
82. Duan XM, Liu XN, Li YX, Cao YQ, Silayiding A, Zhang RK, et al. MicroRNA-498 Promotes Proliferation, Migration, and Invasion of Prostate Cancer Cells and Decreases Radiation Sensitivity by Targeting PTEN. Kaohsiung J Med Sci (2019) 35(11):659–71. doi: 10.1002/kjm2.12108
83. Borges HL, Linden R, Wang JYJ. DNA Damage-Induced Cell Death: Lessons From the Central Nervous System. Cell Res (2008) 18(1):17–26. doi: 10.1038/cr.2007.110
84. Hsu TI, Hsu CH, Lee KH, Lin JT, Chen CS, Chang KC, et al. MicroRNA-18a is Elevated in Prostate Cancer and Promotes Tumorigenesis Through Suppressing STK4 In Vitro and In Vivo. Oncogenesis (2014) 3:e99. doi: 10.1038/oncsis.2014.12
85. Yang J, Hao T, Sun J, Wei P, Zhang H. Long Noncoding RNA GAS5 Modulates α-Solanine-Induced Radiosensitivity by Negatively Regulating miR-18a in Human Prostate Cancer Cells. Biomed Pharmacother (2019) 112:108656. doi: 10.1016/j.biopha.2019.108656
86. Mao A, Tang J, Tang D, Wang F, Liao S, Yuan H, et al. MicroRNA-29b-3p Enhances Radiosensitivity Through Modulating WISP1-Mediated Mitochondrial Apoptosis in Prostate Cancer Cells. J Cancer (2020) 11(21):6356–64. doi: 10.7150/jca.48216
87. John-Aryankalayil M, Palayoor ST, Makinde AY, Cerna D, Simone CB 2nd, Falduto MT, et al. Fractionated Radiation Alters Oncomir and Tumor Suppressor miRNAs in Human Prostate Cancer Cells. Radiat Res (2012) 178(3):105–17. doi: 10.1667/RR2703.1
88. Dai H, Wang C, Yu Z, He D, Yu K, Liu Y, et al. MiR-17 Regulates Prostate Cancer Cell Proliferation and Apoptosis Through Inhibiting JAK-STAT3 Signaling Pathway. Cancer Biother Radiopharm (2018) 33(3):103–9. doi: 10.1089/cbr.2017.2386
89. Xu Z, Zhang Y, Ding J, Hu W, Tan C, Wang M, et al. miR-17-3p Downregulates Mitochondrial Antioxidant Enzymes and Enhances the Radiosensitivity of Prostate Cancer Cells. Mol Ther Nucleic Acids (2018) 13:64–77. doi: 10.1016/j.omtn.2018.08.009
90. Liao H, Xiao Y, Hu Y, Yin Z, Liu L. microRNA-32 Induces Radioresistance by Targeting DAB2IP and Regulating Autophagy in Prostate Cancer Cells. Oncol Lett (2015) 10(4):2055–62. doi: 10.3892/ol.2015.3551
91. Jalava SE, Urbanucci A, Latonen L, Waltering KK, Sahu B, Janne OA, et al. Androgen-Regulated miR-32 Targets BTG2 and Is Overexpressed in Castration-Resistant Prostate Cancer. Oncogene (2012) 31(41):4460–71. doi: 10.1038/onc.2011.624
92. Gu H, Liu M, Ding C, Wang X, Wang R, Wu X, et al. Hypoxia-Responsive miR-124 and miR-144 Reduce Hypoxia-Induced Autophagy and Enhance Radiosensitivity Of Prostate Cancer Cells via Suppressing PIM1. Cancer Med (2016) 5(6):1174–82. doi: 10.1002/cam4.664
93. Xue G, Ren Z, Chen Y, Zhu J, Du Y, Pan D, et al. A Feedback Regulation Between miR-145 and DNA Methyltransferase 3b in Prostate Cancer Cell and Their Responses to Irradiation. Cancer Lett (2015) 361(1):121–7. doi: 10.1016/j.canlet.2015.02.046
94. El Bezawy R, Tripari M, Percio S, Cicchetti A, Tortoreto M, Stucchi C, et al. SPOP Deregulation Improves the Radiation Response of Prostate Cancer Models by Impairing DNA Damage Repair. Cancers (Basel) (2020) 12(6):1462. doi: 10.3390/cancers12061462
95. Petrova V, Annicchiarico-Petruzzelli M, Melino G, Amelio I. The Hypoxic Tumour Microenvironment. Oncogenesis (2018) 7(1):10. doi: 10.1038/s41389-017-0011-9
96. Fort RS, Matho C, Oliveira-Rizzo C, Garat B, Sotelo-Silveira JR, Duhagon MA. An Integrated View of the Role of miR-130b/301b miRNA Cluster in Prostate Cancer. Exp Hematol Oncol (2018) 7:10. doi: 10.1186/s40164-018-0102-0
97. Wang W, Liu M, Guan Y, Wu Q. Hypoxia-Responsive Mir-301a and Mir-301b Promote Radioresistance of Prostate Cancer Cells via Downregulating Ndrg2. Med Sci Monit (2016) 22:2126–32. doi: 10.12659/MSM.896832
98. Kolluru V, Chandrasekaran B, Tyagi A, Dervishi A, Ankem M, Yan X, et al. miR-301a Expression: Diagnostic and Prognostic Marker for Prostate Cancer. Urol Oncol (2018) 36(11):503.e9–.e15. doi: 10.1016/j.urolonc.2018.07.014
99. Ribatti D, Tamma R, Annese T. Epithelial-Mesenchymal Transition in Cancer: A Historical Overview. Trans Oncol (2020) 13(6):100773. doi: 10.1016/j.tranon.2020.100773
100. Fenderico N, Cominetti D, El Bezawy R, Tortoreto M, Dugo M, Valdagni R, et al. MiR-875-5p Impairs Prostate Cancer Metastasis by Remodeling Tumor Secretome and Enhances Tumor Radiation Response via EGFR Suppression (Abstract). Mol Cancer Ther (2015) 14(12):A134–A134. doi: 10.1158/1535-7163.TARG-15-A134
101. Zhang L, Liao Y, Tang L. MicroRNA-34 Family: A Potential Tumor Suppressor and Therapeutic Candidate in Cancer. J Exp Clin Cancer Res (2019) 38(1):53. doi: 10.1186/s13046-019-1059-5
102. Kojima K, Fujita Y, Nozawa Y, Deguchi T, Ito M. MiR-34a Attenuates Paclitaxel-Resistance of Hormone-Refractory Prostate Cancer PC3 Cells Through Direct and Indirect Mechanisms. Prostate (2010) 70(14):1501–12. doi: 10.1002/pros.21185
103. Bertoli G, Cava C, Castiglioni I. MicroRNAs as Biomarkers for Diagnosis, Prognosis and Theranostics in Prostate Cancer. Int J Mol Sci (2016) 17(3):421. doi: 10.3390/ijms17030421
104. Weidhaas JB, Babar I, Nallur SM, Trang P, Roush S, Boehm M, et al. MicroRNAs as Potential Agents to Alter Resistance to Cytotoxic Anticancer Therapy. Cancer Res (2007) 67(23):11111–6. doi: 10.1158/0008-5472.CAN-07-2858
105. Dong Q, Meng P, Wang T, Qin W, Qin W, Wang F, et al. MicroRNA Let-7a Inhibits Proliferation of Human Prostate Cancer Cells In Vitro and In Vivo by Targeting E2F2 and CCND2. PLoS One (2010) 5(4):e10147. doi: 10.1371/journal.pone.0010147
106. Wang W, Liu J, Wu Q. MiR-205 Suppresses Autophagy and Enhances Radiosensitivity of Prostate Cancer Cells by Targeting TP53INP1. Eur Rev Med Pharmacol Sci (2016) 20(1):92–100.
107. Xu CG, Yang MF, Fan JX, Wang W. MiR-30a and miR-205 are Downregulated in Hypoxia and Modulate Radiosensitivity of Prostate Cancer Cells by Inhibiting Autophagy via TP53INP1. Eur Rev Med Pharmacol Sci (2016) 20(8):1501–8.
108. Tao Z, Xu S, Ruan H, Wang T, Song W, Qian L, et al. MiR-195/-16 Family Enhances Radiotherapy via T Cell Activation in the Tumor Microenvironment by Blocking the PD-L1 Immune Checkpoint. Cell Physiol Biochem (2018) 48(2):801–14. doi: 10.1159/000491909
109. McDermott N, Meunier A, Wong S, Buchete V, Marignol L. Profiling of a Panel of Radioresistant Prostate Cancer Cells Identifies Deregulation of Key miRNAs. Clin Transl Radiat Oncol (2017) 2:63–8. doi: 10.1016/j.ctro.2017.01.005
110. Kanwal R, Plaga AR, Liu X, Shukla GC, Gupta S. MicroRNAs in Prostate Cancer: Functional Role as Biomarkers. Cancer Lett (2017) 407:9–20. doi: 10.1016/j.canlet.2017.08.011
111. Templin T, Paul S, Amundson SA, Young EF, Barker CA, Wolden SL, et al. Radiation-Induced Micro-RNA Expression Changes in Peripheral Blood Cells of Radiotherapy Patients. Int J Radiat Oncol Biol Phys (2011) 80(2):549–57. doi: 10.1016/j.ijrobp.2010.12.061
Keywords: prostate cancer, radiotherapy, RNA therapy, microRNA, oncomiR, oncosupressor miR
Citation: Soares S, Guerreiro SG, Cruz-Martins N, Faria I, Baylina P, Sales MG, Correa-Duarte MA and Fernandes R (2021) The Influence of miRNAs on Radiotherapy Treatment in Prostate Cancer – A Systematic Review. Front. Oncol. 11:704664. doi: 10.3389/fonc.2021.704664
Received: 03 May 2021; Accepted: 06 July 2021;
Published: 03 August 2021.
Edited by:
Alan Jay Katz, St. Francis Hospital, United StatesReviewed by:
Laure Marignol, Trinity College Dublin, IrelandCopyright © 2021 Soares, Guerreiro, Cruz-Martins, Faria, Baylina, Sales, Correa-Duarte and Fernandes. This is an open-access article distributed under the terms of the Creative Commons Attribution License (CC BY). The use, distribution or reproduction in other forums is permitted, provided the original author(s) and the copyright owner(s) are credited and that the original publication in this journal is cited, in accordance with accepted academic practice. No use, distribution or reproduction is permitted which does not comply with these terms.
*Correspondence: Rúben Fernandes, cmZlcm5hbmRlc0Blc3MuaXBwLnB0; Susana G. Guerreiro, Z3VlcnJlaXJvLnN1QGdtYWlsLmNvbQ==
†ORCID: Maria Goreti Sales, orcid.org/0000-0001-9936-7336
Disclaimer: All claims expressed in this article are solely those of the authors and do not necessarily represent those of their affiliated organizations, or those of the publisher, the editors and the reviewers. Any product that may be evaluated in this article or claim that may be made by its manufacturer is not guaranteed or endorsed by the publisher.
Research integrity at Frontiers
Learn more about the work of our research integrity team to safeguard the quality of each article we publish.