- 1Department of Marine Science, Institute of Marine Science, Federal University of São Paulo – UNIFESP, São Paulo, Brazil
- 2Department of Ecotoxicology, Santa Cecilia University (Unisanta), São Paulo, Brazil
- 3Department of Biological and Environmental Sciences, Biosciences Institute, São Paulo State University – UNESP, São Vicente, SP, Brazil
- 4Merieux Nutriscience, Environment Analysis, São Paulo, Brazil
- 5Marine Inorganic Chemistry Laboratory, Oceanographic Institute, University of São Paulo – USP, São Paulo, SP, Brazil
- 6National Institute of Science and Technology of Energy & Environment, Federal University of Bahia, Salvador, Bahia, Brazil
- 7Interdisciplinary Center for Energy and Environment, Federal University of Bahia, Salvador, Bahia, Brazil
Environmental and climate changes have placed increasing pressure on the resilience of marine ecosystems. In addition to these transformations, coastal environments are also affected by anthropogenic stressors, such as metal contamination. Bivalves play a crucial ecological role in marine and estuarine ecosystems. This study aimed to evaluate the effects of CO2-induced acidification, warming, and mixed metals contamination on the mangrove mussel Mytella charruana. We evaluated DNA damage (strand breaks), lipid peroxidation (LPO) levels, and reduced glutathione (GSH) content, as well as the enzymatic activities of glutathione S-transferase (GST) and glutathione peroxidase (GPx) in the gills and digestive glands. Additionally, neurotoxicity was assessed in muscle tissues through acetylcholinesterase (AChE) activity. Laboratory experiments were conducted using sediments spiked with metals (Cu, Pb, Zn, and Hg), alongside a control group (non-spiked sediments), combining with three pH levels (7.5, 7.1, and 6.7) and two temperatures (25 and 27°C). Five mussels per treatment (four replicates) were exposed for 96 h. Two pools of two organisms each were separated per replicate (n = 8) and their gills, digestive glands, and muscles were dissected for biochemical biomarkers analyses. Temperature increase and metal contamination were the primary factors modulating antioxidant responses in the gills and digestive glands, as well as AChE activity in the muscle. However, when combined with CO2-induced acidification, these stressors also affected DNA integrity and LPO. Acidification alone showed no effect for any biomarker analyzed. Higher IBR values indicated effects for combined metal exposure, even at concentrations below individual safety levels. Here, we provide insights from a short-term experiment on the complex interactions between predicted scenarios, in which climate change stressors influenced estuarine mussel responses when associated with a mixture of metals in sediments. These findings contribute to understanding of organismal responses in complex scenarios of contamination and climate change, particularly in estuarine environments.
1 Introduction
Since the pre-industrial period, atmospheric CO2 concentrations have increased from 277 to 414 ppm in 2021, and they have continued to increase by approximately 2.4 ppm per year over the last decade, reaching 1.070 ppm until the end of the century (Friedlingstein et al., 2020; IPCC Intergovernmental Panel on Climate Change, 2022). In addition, current surface seawater temperatures have been approximately 1.0 °C warmer since the beginning of the industrial period (Lough et al., 2018; Garcia-Soto et al., 2021; IPCC Intergovernmental Panel on Climate Change, 2022). The global ocean absorbs approximately one third of atmospheric CO2 and heat (Caldeira and Wickett, 2003; Levitus et al., 2005) leading to an expected reduction of surface ocean water pHs of an average of 0.3–0.4 units and temperature elevation by 2.6–4.8°C by 2100 (IPCC Intergovernmental Panel on Climate Change, 2014).
Ocean acidification and warming are critical threats to coastal ecosystems (Boyd et al., 2018; Lu et al., 2018; Bahamon et al., 2020; Cotovicz Júnior et al., 2022). Furthermore, sediments from densely urbanized and industrialized coastal areas are frequently contaminated by metals and metalloids such as mercury, arsenic, cadmium, lead, zinc, and copper (Boyd, 2010; Kim et al., 2016; Alves et al., 2022). Under such conditions, estuarine and coastal organisms are commonly exposed to the metals present in sediments (Trevizani et al., 2023; Pinho et al., 2024). Previous studies have reported that both low pH/high pCO2 and warming can affect the solubility and speciation of metals in the environment (Tsai et al., 2003; Sokolova and Lannig, 2008; Millero et al., 2009; Banaee et al., 2024) as well as their availability, increasing their potential toxic effects on biota (Roberts et al., 2013; Halsband et al., 2024).
Bivalves play a crucial ecological role in marine and estuarine ecosystems (Byrne and O'halloran, 2001). The benthic mangrove mussel Mytella charruana (Mollusca: Mytilidae) is commonly found in tropical estuarine regions (Yuan et al., 2016), adhering to hard substrates or forming dense aggregates in estuarine mud. Owing to its suspension-feeding mode, this species is exposed to sediment contamination through water and food ingestion. Bivalve molluscs are considered effective bioindicators because of their tolerance to fluctuations in abiotic factors (Belivermiş et al., 2016, 2023), including elevated pCO2 (Range et al., 2012), and their capacity to accumulate contaminants throughout their life cycle, making them widely used in environmental risk assessments of metal pollution in marine systems (Zhang et al., 2010; Basallote et al., 2015; Campana et al., 2015; Chandurvelan et al., 2015; Rocha et al., 2016). Therefore, these organisms can be considered excellent test organisms for evaluating scenarios of marine acidification and warming in sediments contaminated by metals.
Although many organisms, such as bivalves, have evolved to resist daily or seasonal abiotic variations in coastal areas (Ross et al., 2016; Zhao et al., 2020; Trevisan and Mello, 2024), complex interactions in multiple potential stressors vary and can drive changes in physiological, biochemical, and ecological fitness tolerance thresholds (Pörtner et al., 2004; Noyes et al., 2009; Maulvault et al., 2017; Alves et al., 2024) as well as their metal accumulation potential (Lacoue-Labarthe et al., 2011; Belivermiş et al., 2016; Nardi et al., 2018), increasing their vulnerability.
Specifically, acidification directly affects mollusks by (i) alterations in shell structure and growth (Michaelidis et al., 2005; Beniash et al., 2010; Dickinson et al., 2013; Harvey et al., 2013; Thomsen et al., 2013; Sokolova et al., 2016), (ii) changes in the opening and closing of valves (Clements et al., 2018), (iii) changes in metabolic processes (heat tolerance and growth rates) (Duarte et al., 2014; Nikinmaa and Anttila, 2015; Wang et al., 2015; Freitas et al., 2017; Cao et al., 2022), and (iv) induction of oxidative stress (Matozzo et al., 2013; Regoli and Giuliani, 2014; Cao et al., 2018). In addition, temperature increases in coastal surface waters affect the physiological processes in bivalves, increasing metal uptake (Belivermiş et al., 2016; Maulvault et al., 2016; Sampaio et al., 2018), changing their energy balance (Sokolova and Lannig, 2008; Cherkasov et al., 2010), causing oxidative stress (Verlecar et al., 2007; Mangan et al., 2017), modifying the lysosomal system (Izagirre et al., 2014; Múgica et al., 2015), and mortality (Lannig et al., 2006).
The effects of exposure to toxic substances or other stressors can be assessed by using various biomarkers. These serve as monitoring tools that provide mechanistic insights into contaminant concentrations in tissues and their associated toxic effects and, by detecting sublethal changes, biomarkers offer an early warning of environmental quality degradation (Depledge et al., 1995; Monserrat et al., 2007; Hwang et al., 2008). This study aimed to evaluate the biomarker responses of M. charruana exposed to multiple stressors simultaneously, such as CO2-induced acidification, warming, and sediment metal contamination. Considering the potential effects in multiple stressor scenarios, we hypothesized that the acidification and warming of coastal waters caused by the increase in atmospheric CO2 can modify the toxicity of metals due to changes in the physiology of the mussel M. charruana. These results will allow for a better understanding of the mechanisms underlying organismal resilience, prediction of ecological impacts, and improvement of environmental risk assessments in a changing ocean.
2 Materials and methods
2.1 Experimental design
Biomarker responses of the gills, digestive glands, and muscles of M. charruana were assessed using a full factorial experimental design with three fixed factors (and their interactions): (i) CO2-induced acidification, (ii) warming, and (iii) sediment contamination by metals (Cu, Pb, Zn, and Hg). The effects of changes in pH/pCO2 were tested at three levels: pH 7.5 (control pH/pCO2 – without CO2 injection), pH 7.1 (medium pH/medium pCO2), and pH 6.7 (low pH/low pCO2). This range of tested pH/pCO2 levels was established based on predictions of oceanic pH made using Representative Concentration Pathways (RCP) 8.5 (Bindoff et al., 2022) and the Coupled Model Intercomparison Project (CMIP6) of the World Climate Research Program (IPCC Intergovernmental Panel on Climate Change, 2021). Two temperatures were tested: 25°C (control temperature) and 27°C (increased temperature), based on the projections range for the end of the century by Intergovernmental Panel on Climate Change on the Representative Concentration Pathways (RCP) 4.5 model (IPCC Intergovernmental Panel on Climate Change, 2014). The metal contamination in sediment was assessed using sediment spiked with Cu, Pb, Zn, and Hg, metals commonly reported in estuarine sediments contaminated, and harmful to organisms (Alves et al., 2022; Zhang et al., 2024). According to a pilot assay, the concentrations of metals were established in two levels: control (cntrl) (no metal-spiked sediment) and a mild metal contamination, corresponding to 75% of the Interim Sediment Quality Guidelines (ISQG) and Probable Effects Level (PEL) [Canadian Council of Ministers of the Environment (CCME), 2002], simulating a reality commonly found in contaminated coastal regions.
Specimens of M. charruana were obtained from fishermen's farms in mangrove areas along the banks of the Bertioga Channel (Bertioga, northern coast of São Paulo State, Brazil, within a marine protected area), where this species is abundant. They were transported to the laboratory in thermal boxes for acclimatization. Before the experiments, the organisms underwent a 7-day acclimation period under controlled laboratory conditions. They were divided into three distinct groups of 60 individuals of homogeneous size and kept in 45 L aquariums containing natural seawater diluted with freshwater (reaching salinity 24), without sediments, to simulate estuarine condition (hereafter referred as experimental water), under constant aeration. Water pH and temperature were gradually adjusted over the 7-day period (±0.4 unit/day) to reach the target experimental conditions, minimizing osmotic and thermal stress on the organisms. During the acclimatization period, the organisms were fed every 48 h with a commercial suspension designed for filter-feeding organisms (Phyto-Plus B®), containing a mixture of microalgae species (2–23 μm), at a dosage of 50 μL per individual. Prior to the experiment, eight organisms were randomly collected from each acclimatization aquarium, and their gills, digestive gland, and muscle tissues were removed for analysis to determine the baseline physiological conditions (T0).
The bioassays were conducted in a hermetic box system adapted from Altafim et al. (2023). Each box contained glass bottles (3 L) with 500 mL of sediment and 2 L of filtered experimental water (salinity 24), with four replicates per treatment, each containing 5 individuals (n = 5). The organisms were fed once after 2 days of exposure, and the experiment lasted for 4 days. Physicochemical parameters (i.e., pH, dissolved oxygen (DO), and salinity) were measured at the beginning and at the end of the bioassay. At the end of exposure, the organisms were kept on ice, and the gills, digestive glands, and muscle tissues were collected for biomarker analyses, in pools of two organisms per replicate (n = 8 per experimental treatment). After the dissection of the organisms, the soft tissues were immediately stored in an ultra-freezer (−80°C).
2.2 Sediment collection, characterization and spiking
Sediment samples were collected from a reference area, in a mangrove portion located at the mouth of the Itaguaré River (23.763°S, 45.773°W), also in the municipality of Bertioga. This location is legally protected as the Restinga of Bertioga State Park (Decree N°. 53.526/2008). In the laboratory, sediment was stored at a constant temperature of 4°C and protected from light until the start of the experiment. The sediment was characterized for grain size fractions through dry sieving and classified based on the Wentworth (1922) scale. The CaCO3 content was quantified by digestion using hydrochloric acid (HCL 5 N) (Hirota and Szyper, 1975), and the organic matter (OM) content was quantified using the loss by ignition method (Luczak et al., 1997).
For the sediment spiking process, the moisture content of the sediment was determined by oven-drying (for 4 d at 60 °C). The moisture was restored by adding distilled water and the spiked sediment was carried out using stock solutions of each metal of interest, prepared by the dissolution of inorganic salts of copper sulfate (CuSO4), lead chloride (PbCl2), zinc sulfate (ZnSO4), and mercuric chloride (HgCl2) (Merck®, reagent grade) in distilled water, following the protocols established by the United States Environmental Protection Agency (United States Environmental Protection Agency (USEPA), 2001) and the American Society for Testing and Materials (American Society for Testing Materials (ASTM), 2014). Quantities of each metal stock solution were spiked to an amount of the sediment, in order to reach nominal concentrations, in dry weight, of 14.03 mg kg−1 of Cu, 22.65 mg kg−1 of Pb, 93.0 mg kg−1 of Zn, and 0.1 mg kg−1 of Hg. This method has been described in detail by Alves et al. (2024). An amount of non-spiked sediment was subjected to the same spiking process as a negative contamination control, in which only distilled water was added to the same volume of the metal stock solution used.
2.3 CO2 Injection system, pH, and temperature control
The CO2-induced acidification was controlled by an automated series of solenoid valves connected to an Apex Fusion® software that manipulated the CO2 injection. The method employed was CO2 indirect introduction, which manipulated the pCO2 in the atmosphere of semi-closed boxes (Altafim et al., 2023; Alves et al., 2024). To achieve this, there is a direct introduction of CO2 into a beaker containing distilled water, allowing CO2 to disperse into the atmosphere inside the box, controlling the decrease and stabilization of pH in each experimental unit of each treatment. The pH of each experimental treatment box was continuously monitored throughout the exposure period using pH sensors connected to the Apex system, as when the pH increased or decreased the established value by 0.05, the system automatically released or opened CO2 injection to restore the set pH.
The temperature at 25 ± 1°C was maintained by air conditioning the room, while the temperature of 27 ± 1°C was kept stable by aquarium thermostats within the respective treatment boxes during the entire exposure period. Additionally, before and during the experiment period, the temperature in each box was calibrated and constantly monitored using analog glass thermometers.
2.4 Carbonate system speciation analysis
Total alkalinity (TA) was measured in each replicate in all treatments, after the exposure time, through titration with HCl (0.1 M) (Sigma-Aldrich analytical grade) using an automatic titrator (Hanna HI901C) coupled to a meter (Hanna Instruments, ref. HI1131) for pH measurement. Then, data from pH (total pH scale/mol kg−1 H2O), TA, measured salinity and temperature were used to estimate the parameters of the carbonate system speciation (partial pressure of CO2 (pCO2), inorganic carbon (TIC), HCO−3, , Ω aragonite and Ω calcite saturation states), using the CO2SYS program (Pierrot et al., 2006), employing the dissociation constants of carbonic acid in seawater (Mehrbach et al., 1973; Dickson and Millero, 1987; Dickson, 1990).
2.5 Sediment metal analysis
The concentrations of metals (Cu, Pb, Zn, and Hg) in the sediments from each contamination treatment (control and metal contamination) were measured before the beginning of the experiments (T0) and at the end of exposure (T1) from each treatment. All laboratory instruments and non-volumetric glassware were decontaminated with detergent, ultrapure water, and 5% HNO3.
The sediment samples were lyophilized and subjected to partial acid digestion following a modification of the USEPA method 3050B (United States Environmental Protection Agency (USEPA), 1996). The digestion methods for Cu, Pb, and Zn were detailed by Altafim et al. (2023) and the acid extracts were quantified using Inductively Coupled Plasma Optical Emission spectroscopy (ICP-OES) (Varian equipment, model 710ES), following USEPA 6010C method (United States Environmental Protection Agency (USEPA), 2007). To control method accuracy and precision, certified reference material (CRM) was analyzed using the same analytical procedure for the respective analyses. The certified reference material SS-2 (EnviroMAT Contaminated Soil, SCP Science) (n = 3) was used for Cu, Pb, and Zn quantification, with recoveries of 87–97% (Supplementary Table 1). The analytical limits of quantification (LOQ) were calculated for each element of interest for an uncertainty of 20% (m = 1.0 g; v = 50 mL) (Supplementary Table 2).
For the determination of mercury (Hg) in sediments, the Direct Mercury Analyzer DMA-80 Tri Cell (Milestone, Italy) was used. This method eliminates the need of sample preparation, enabling direct analysis through a simplified process involving drying, decomposition, amalgamation, and detection by atomic absorption spectrometry (AAS). The main advantage lies in its efficiency, bypassing time-consuming steps. The samples were weighed (50 mg) directly into nickel boats and subjected to a heating cycle: drying at 200 °C for 60 seconds, decomposition at 650°C for 120 s and amalgamation for 12 s. Mercury vapor was transported by 99.9% pure oxygen (White Martins, Brazil) at 4 kg cm1, with detection based on absorbance at 253.7 nm. Each analysis was completed in approximately 5 min. Method accuracy was validated using CRM MESS-3 and DORM-4 (National Research Council of Canada, NRC – CNRC) (n = 3), yielding results of 0.088 ± 0.004 μ g−1 (MESS-3) and 0.457 ± 0.04 μg g−1 (DORM-4), consistent with certified values of 0.091 ± 0.009 μg g−1 and 0.412 ± 0.036 μg g−1, with recovery result of 97 and 111%, respectively (Supplementary Table 1). Calibration curves (0.01–100 ng Hg) showed determination coefficients > 0.999, with detection and quantification limits of 4.0 pg and 12.0 pg, calculated as (3σ/S) and (10σ/S), where σ is the standard deviation of 10 blank measurements and S is the calibration curve slope.
2.6 Biomarkers responses
Aliquots of the samples of gills and digestive glands of mussels were separated for quantification of levels of non-protein thiols (reduced glutathione, GSH), and DNA damage (strand-breaks). In addition, glutathione-S-transferase (GST) and glutathione peroxidase (GPx) enzymatic activities were analyzed. The levels of LPO in gills were also quantified. Adductor muscle tissues were separated for acetylcholinesterase (AChE) enzymatic activity analysis. The tissues were homogenized (in a pool of 2 organisms) to 5% w/v in a Tris-HCl buffer in pH 7.6 in Tris-HCl buffer (50 mM Tris; 1 mM EDTA; 1 mM DTT; 50 M Sucrose; KCl a 150 mM, 1 mM PMSF, pH 7.6) in a cell disruptor (Loccus L-beader 24). Aliquots of gill and digestive gland homogenates were separated for total protein quantification, DNA damage, and LPO (150 μl). Then, the remained samples were centrifuged for 20 min at 12,000 G in 4°C and the supernatant was separated for analysis of GST and GPx enzymatic activities, and GSH levels. In the case of muscle tissue, the supernatant fraction was used to determine AChE. Total protein contents were quantified in the homogenized and centrifuged fractions using the dye-binding method by Bradford (1976) using bovine serum albumin protein (BSA) as standard. All analyzes were performed on a microplate reader (Biotek-Synergy ™ HT).
For DNA damage analysis, the alkaline precipitation method described by Olive (1988) was used, with DNA quantification performed through fluorescence (Gagné and Blaise, 1995). DNA damage was expressed as μg of DNA mg−1 of protein. Oxidative stress-induced changes in LPO levels were assessed indirectly using the thiobarbituric acid reactive substances (TBARs) method, following Wills (1987), with results expressed as μM TBARs mg−1 of total protein. GSH levels were determined using the method of Sedlak and Lindsay (1968), measured spectrophotometrically at 415 nm, and expressed as nmol min−1 mg−1 of total protein. GST enzymatic activity was determined by incubating supernatants with 42 mM 1-chloro-2,4-dinitrobenzene (CDNB), 1 mM glutathione, and 80 mM K2HPO4 (pH 6.5). The reaction was monitored spectrophotometrically at 340 nm for 5 min, with measurements taken at 30-s intervals (Keen et al., 1976). GST enzymatic activity was expressed as nmol GSH-CDNB min−1 mg−1 of protein. GPx enzymatic activity was assessed following the method of Sies et al. (1979), using a reaction mixture containing 1 mM hydroperoxide in sodium phosphate buffer (pH 7.0), with NADPH added as a cofactor. Absorbance was measured spectrophotometrically at 340 nm, and GPx enzymatic activity was expressed as nmol min−1 mg−1 of total protein. Neurotoxicity effects were assessed by measuring AChE activity following the method of Ellman et al. (1961). A reaction mixture containing 10 mmol L−1 DTNB and 0.075 mol L−1 acetylcholine iodide in potassium phosphate buffer (pH 7.6) was added to the samples. Absorbance was immediately recorded at 405 nm for 2 min, with measurements taken at 30-s intervals. AChE activity was expressed as nmol NTB min1 mg1 of protein.
2.7 Data treatment
Mean sediment quality guideline quotients (mSQGQs) were calculated for each experimental treatment based on the Probable Effects Level (PEL) [Canadian Council of Ministers of the Environment (CCME), 2002] to estimate the relative impact of sediment metal contamination in sediments on biomarker responses in Mytella charruana. The mSQGQ was determined by normalizing the concentration of each metal in the sediments to its respective sediment quality guideline (SQG) and then averaging the individual metal quotients into a single value (Long, 2006). The means Probable Effects Level quotient (mPELq) was determined according to Equation 1:
where “Ci” represents the investigated content of metal “i”, “PELi” represents the PEL of metal “i” and n represents the number of metal species. Categories of mPELq was classified according to ecotoxicological risk with 10, 25, 50, and 75% probability of toxicity, respectively: low risk level (mPELq ≤ 0.1); low to medium risk level (0.11 < mPELq < 1.5); medium to high risk level (1.51 < mPELq < 2.3), and high ecotoxicological risk level (mPELq > 2.3) (Long and MacDonald, 1998).
Biological data were analyzed by first assessing potential laboratory effects of acclimation to pH/pCO2 and warming on the general health condition of the tested organisms. This was done by comparing biomarker data before (T0) and after the bioassays (control treatment for each pH and temperature). Then the effects of CO2-induced acidification, temperature, and metal contamination on biomarker responses were assessed. For both comparisons, a General Linear Model (GLM) analysis with an identity link function were built using the statistical software (Jamovi Project, 2022) (version 2.3) Gallucci (2019) for each biomarker analyzed. A confidence level of p ≤ 0.05 and the distribution was determined according to the best fit of the model to the data distribution, which are the lowest Akaike Information Criterion (AIC) (Quinn and Keough, 2002) and best normality of residuals were adopted for all statistical analyses. Tukey's post hoc test was performed, when necessary, to detect significant differences between levels.
2.7.1 Integrative approach
Mean values of each biomarker as variables was transformed by Z-score. After, PCA was performed using the software PAST (version 5.0.2) (Hammer et al., 2001), and values greater than ≥ 0.4 were considered relevant associations.
A more general view of the biomarker responses was obtained by means of the calculation of the “Integrated Biomarker Response” – version 2 individual (IBRv2i) for each treatment, following the methodology proposed by Sanchez et al. (2013) and adapted by Mattos et al. (2024). This IBR approach compares the average of biomarker responses of mussels exposed to stressors (and their interactions) with the average of those of animals under control conditions (at normal or basal levels of each biomarker), maintaining biomarker data for each individual in order to enabling the use of statistical analysis. For this study, the respective control groups of each pH at each temperature were used as reference. The index was calculated as: (i) log transformation of the data; (ii) Z-score; (iii) subtraction of the mean Z-score of the control or reference group from the Z-score of all groups; (iv) modulus of all Z-scores to obtain absolute values; (v) sum of the absolute Z-scores of the biomarkers of each experimental group with the weight attributed to each biomarker according to its systemic importance (GSH, GST, and GPx = 1; DNA and LPO = 2) to obtain the IBRv2i. In general, lower values of the IBR index are indicative of a better health status (or greater animal fitness), and higher scores may indicate a worsening of the physiological condition of the organisms. IBRv2i of the gills and digestive gland were compared to the control using the same GLM approach described to detect significant differences between levels.
3 Results
3.1 Physicochemical analysis of sediment
The grain size analysis demonstrated a predominance of very fine sands (54.74%), followed by fine sands (41.38%), with 0.87% silt and clay. The sediment had CaCO3 levels of 2.23% and OM of 1.90%.
Sediment metal spiking was effective, with sediment concentrations at the beginning of exposure approaching the pre-established nominal concentrations set for this study. The concentrations of Cu, Pb, Zn, and Hg in sediment are in Table 1. The final exposure period (T1) concentrations of trace metals added to the sediment did not show substantial differences among pH and temperature levels comparing with the beginning (T0). In addition, the mPELq values of metals spiked sediment in the control and metal contamination treatments at 25 and 27°C exhibited low to medium ecotoxicological risk classification at the beginning and end of the experiment (Table 1).
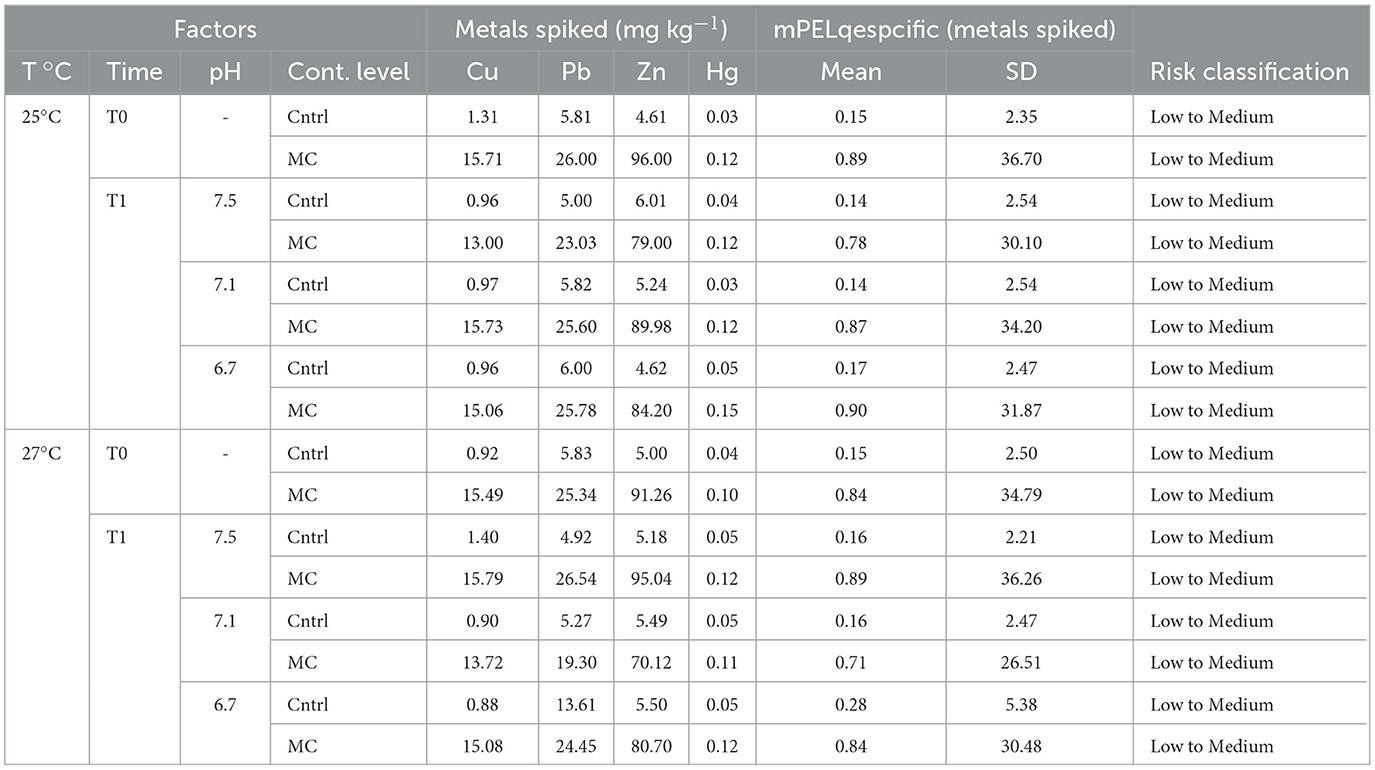
Table 1. Analytical concentrations (mg kg−1) and calculated mPELq for the spiked trace metals, other trace elements, and all trace elements in the tested sediments, at the beginning and end of the tests, in all experimental treatments.
3.2 Carbonate chemistry speciation
In general, the physical-chemical parameters of the seawater measured at the beginning and the end of the bioassay were maintained within acceptable limits for the experiment and low variations regarding at T0. Salinity levels were maintained around 25 ± 1, while dissolved oxygen concentrations remained 5.95 ± 0.68 mg L−1, and non-ionized ammonia were 0.02 ± 0.01 mg L−1. The pH and temperature exhibited minimal variation within the same treatment but were well different between the tested levels, with 7.46 ± 0.03 for the control, 7.14 ± 0.04 for the medium pH and 6.8 ± 0.03 to low pH, and 24.42 ± 0.63 °C / 27.09 ± 0.42 °C, respectively (Supplementary Table 3).
The carbonate system parameters analyzed did not vary regarding the increase in temperature, validating the manipulations by CO2 injection (Table 2). As expected, the CO2-induced acidification treatments (pH 7.1 and 6.7) had increasing in total alkalinity, total inorganic carbon, pCO2, and CO2 concentrations. An inverse relationship with TIC, ΩCa and ΩAr saturations and carbonate concentrations were reduced. These analyses confirmed the reduction of pH by manipulations with CO2 injection and are in line with other studies (Ferraz et al., 2022; Altafim et al., 2023; Alves et al., 2024; Portugal et al., 2025).
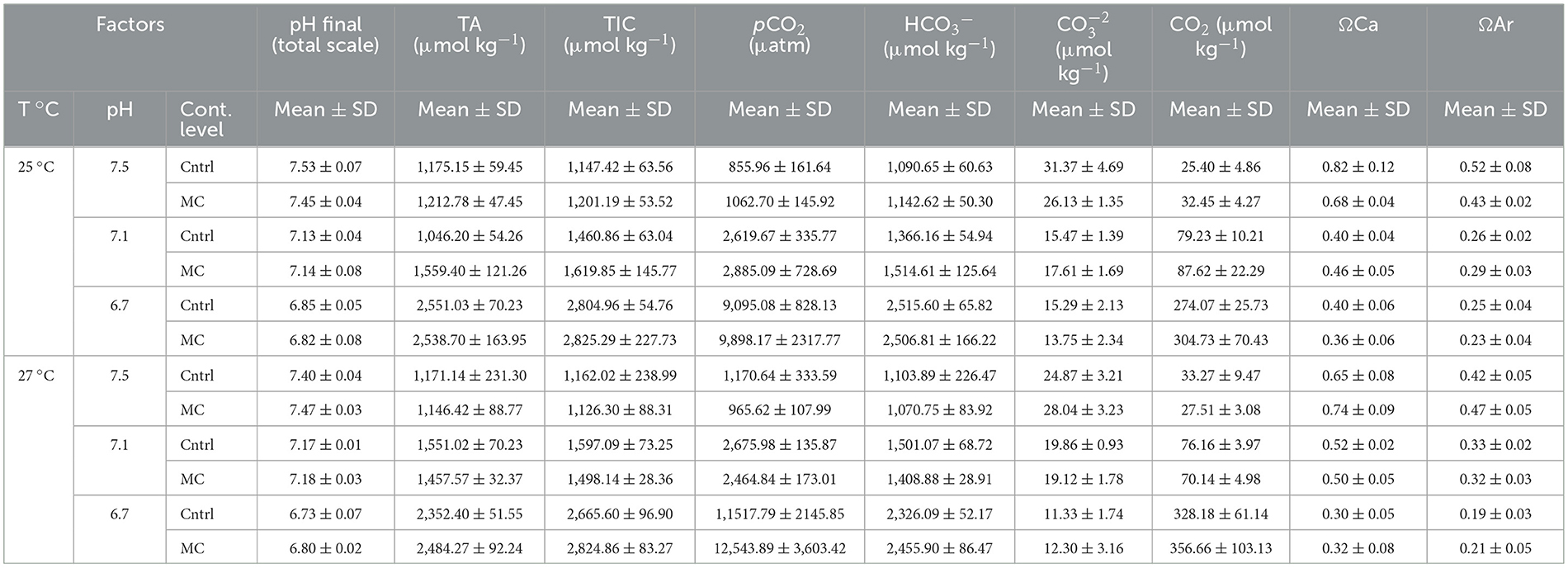
Table 2. Values of carbonate system speciation calculated at the end of the experiment with metals in sediment under different warming scenarios. Quantified values of total alkalinity (TA) in water samples and estimated concentrations of total inorganic carbon (TIC), bicarbonate (), carbonate (); partial pressure of CO2 (pCO2); saturation state for calcite (ΩCa) and aragonite (ΩAr).
3.3 Biomarkers responses
3.3.1 Interactive effects of pH, temperature, and contamination on gills tissue
The results of GLM analysis of biomarker responses on gills tissue are presented in Figure 1, and QQ Plot residuals are detailed in Supplementary Figure 1. In the comparison between the condition after 7 days of organisms acclimatization to the laboratory conditions (T0) with their respective control treatments for each pH and temperature, there was a significant difference only at pH 6.7 at the highest temperature for DNA damage (p < 0.001), and for GSH at pH 7.5 (p < 0.001) and 6.7 (p = 0.004) at 25°C, which indicates low or no “laboratory effect” on biomarkers in the gills of M. charruana.
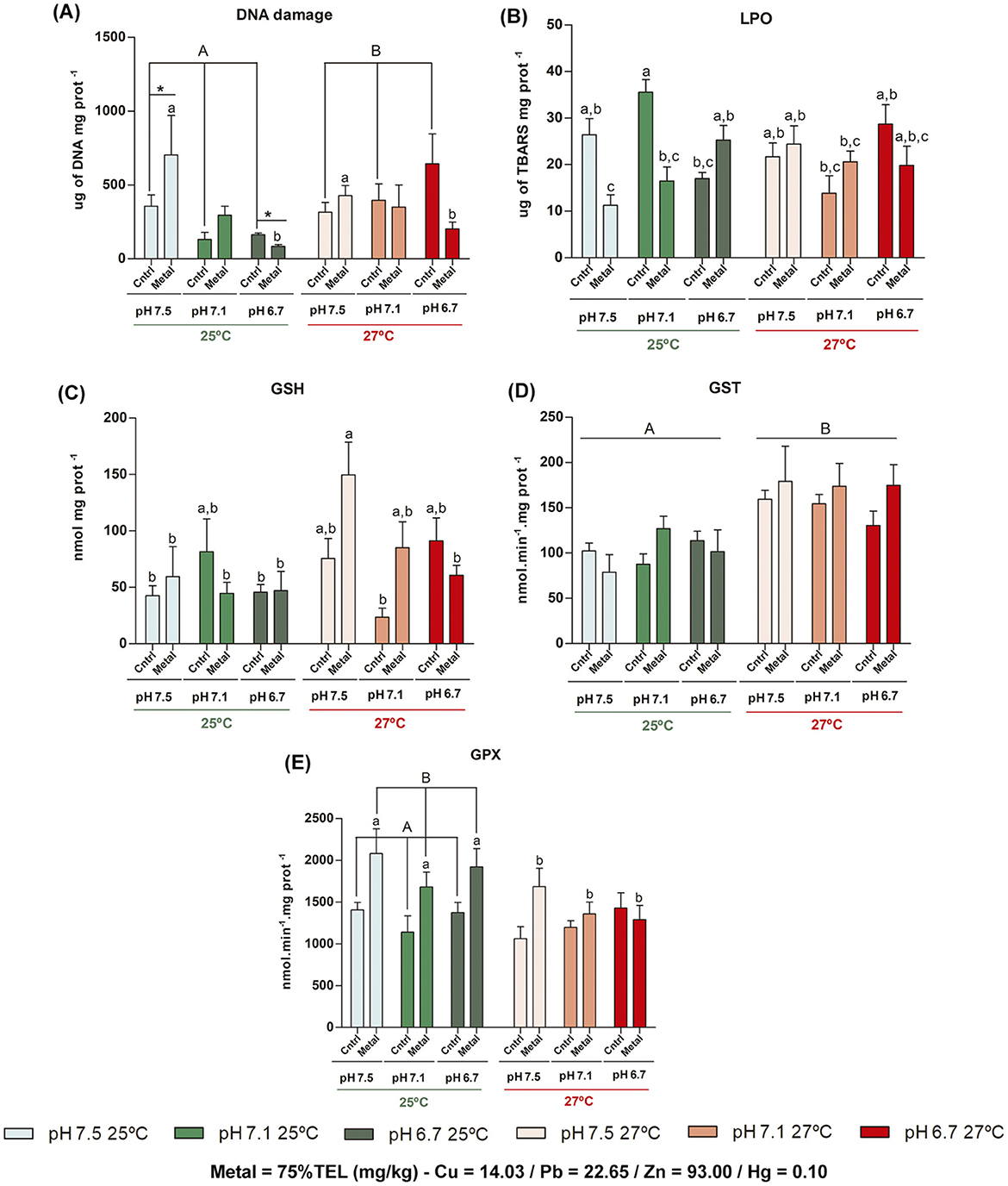
Figure 1. Means and standard deviations (n = 8) of biomarkers responses on gills tissue of Mytella charruana. (A) DNA damage, (B) Lipid peroxidation (LPO), (C) Reduced glutathione (GSH), (D) Glutathione s-transferase (GST), and (E) Glutathione peroxidase (GPx). Green-toned bars represent treatments at 25°C, and red-toned bars represent treatments at 27°C. Light green and light red bars represent treatments at pH 7.5, green and salmon red bars represent treatments at pH 7.1, and dark green and red bars represent treatments at pH 6.7. C = control treatments (without metal exposure) and Metal = Cu, Pb, Zn, and Hg sediment contaminated treatments. Capital letters represent significant differences between factors (considering the entire factor group), and lowercase letters represent significant differences between treatments (each single bar).
For DNA damage in gills, GLM analysis showed that the variation in pH/pCO2 did not show a significant individual effect, but only when combined with contamination (AIC = 1,245.23, F = 4.52, df = 2, p = 0.014), causing a decrease of DNA damage in pH 6.7, compared with pH 7.5, at 25 °C (ptukey = 0.007). Also, there was an increase in DNA damage due to the increase in temperature in the control treatments (no metal contamination) (ptukey = 0.015). Interactions among the three factors were found for levels of LPO (AIC = 638.23, F = 12.50, df = 2, p < 0.001), with reduced effects on metal-contaminated treatments in pHs 7.5 and 7.1 at the control temperature (25°C) compared with their respective controls (ptukey = 0.049 and ptukey = 0.014, respectively), however, high pCO2 decreasing LPO levels in control treatment (ptukey = 0.020) and increased in metal contamination (ptukey = 0.002). In addition, temperature induced LPO levels in pH control (7.5) at metal contamination while inhibiting them in control at pH 7.1 compared to the same treatments in temperature control (ptukey = 0.005 and 0.004, respectively). Regarding GSH levels, significant difference in the interaction between the three factors were also observed (AIC = 964.77, F = 3.13, df = 2, p = 0.049), with an increase only in the pH 7.5 with metal exposure at 27°C when comparing on all pHs at 25°C (ptukey = 0.043; 0.005; 0.007), but when combining low pH (6.7) and warming, the GSH levels were inhibited in metal-contaminated treatment (ptukey = 0.048). As for GST enzymatic activity, significant difference was found only among the temperatures tested (AIC = 973.46, F = 23.14, df = 1, p < 0.001), which increased in warming scenario (ptukey < 0.001). GPx enzymatic activity showed interaction between temperature and metal contamination (AIC = 1,296.88, F = 3.38, df = 1, p = 0.05), with an increase in GPx activity related with metal contamination in control temperature (25°C) (ptukey < 0.001), but decrease associated temperature increase (27°C) (ptukey = 0.014).
3.3.2 Interactive effects of pH, temperature, and contamination on digestive gland tissue
The results of GLM analysis of biomarker responses on digestive gland tissue of M. charruana are presented in Figure 2, and QQ Plot residuals are detailed in Supplementary Figure 2. In the comparison between the acclimatization period (T0) and the respective control treatments, there was a significant difference only in the pH 7.5 at 27°C for GPX enzymatic activity (p = 0.017). On the exposure comparisons between treatments, DNA damage exhibited a slight increase when the temperature increased (AIC = 598.71, F = 4.48, df = 1, p = 0.043). For GSH, the interaction between temperature and metal contamination was significant (AIC = 309.71, F = 13.64, df = 1, p < 0.001), showing the induction of GSH after metal exposure at 27°C, compared to 25°C (ptukey < 0.001), and among control and metal contamination treatment at the same temperature (ptukey = 0.002). Effects related with sediment contamination by metal were observed (AIC = 500.27, F = 5.26, df = 1, p = 0.029), with decreased GST enzymatic activity in metal-contaminated treatments compared to control in 25°C (ptukey = 0.013). As for GPx enzymatic activity, significant interactions between temperature and metal contamination were observed (AIC = 684.08, F = 9.77, df = 1, p = 0.004), with a decrease in metal-contaminated treatments at 25°C when compared with controls (no-spiked sediment) (ptukey = 0.013), and between control treatments (no spiked-metal sediment) at 25 and 27°C (ptukey < 0.001). Although not statistically different, there is a tendency of reduction of the GPx enzymatic activity associated with metal contamination in the more acidic treatment (pH 6.7) at 27°C compared to the same treatment at control temperature.
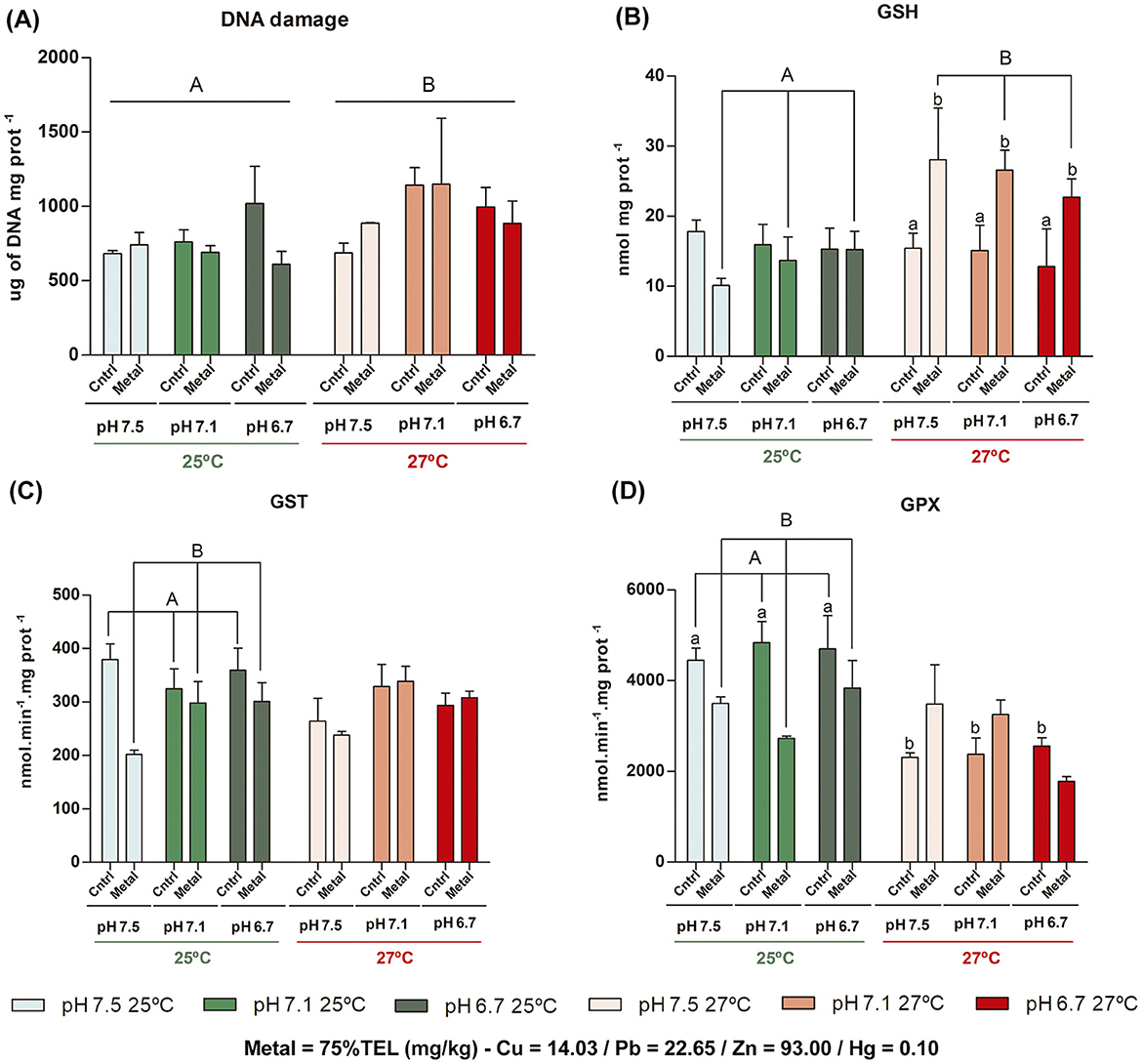
Figure 2. Means and standard deviations (n = 8) of biomarker responses on digestive gland tissue of Mytella charruana. (A) DNA damage, (B) Reduced glutathione (GSH), (C) Glutathione s-transferase (GST), and (D) Glutathione peroxidase (GPx). Green-toned bars represent treatments at 25°C, and red-toned bars represent treatments at 27°C. Light green and light red bars represent treatments at pH 7.5, green and salmon red bars represent treatments at pH 7.1, and dark green and red bars represent treatments at pH 6.7. Cntrl = control treatments (without metal exposure), and Metal = Cu, Pb, Zn, and Hg sediment contaminated treatments. Capital letters represent significant differences between factors (considering the entire factor group), and lowercase letters represent significant differences between treatments (each single bar).
3.3.3 Interactive effects of pH, temperature, and contamination on adductor muscle tissue
For the AChE enzymatic activity in muscle tissues, there were differences between the acclimatization period (T0) and the respective control treatments only in the lowest pH tested (6.7) at warm temperature (27°C) (p < 0.001). There was a significant difference among the metal contamination and temperatures (AIC = 952.60, F = 5.16, df = 1, p = 0.02), showing that the increase in enzyme cholinesterase levels in metal contaminated exposure at 27°C treatments compared to 25°C (ptukey = 0.037) (Figure 3 and QQ Plot residuals are detailed in Supplementary Figure 3).
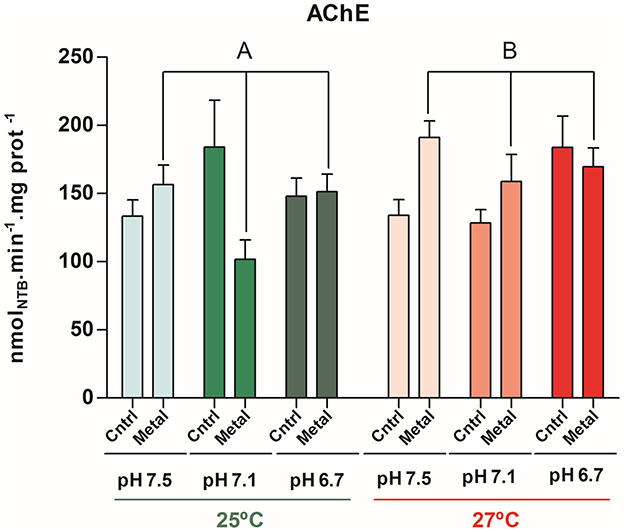
Figure 3. Means and standard deviations (n = 8) of biomarker response of neurotoxicity effects (Acetylcholine - AChE) on adductor muscle tissue of Mytella charruana. Green-toned bars represent treatments at 25°C, and red-toned bars represent treatments at 27°C. Light green and light red bars represent treatments at pH 7.5, green and salmon red bars represent treatments at pH 7.1, and dark green and red bars represent treatments at pH 6.7. Cntrl = control treatments (without metal exposure), and Metal = Cu, Pb, Zn, and Hg sediment contaminated treatments. Capital letters represent significant differences between factors.
3.3.4 PCA integration of biomarkers responses
The results of the PCA integrated all data from biomarkers responses for gills, digestive glands, and muscle tissues, and the factors tested: CO2-induced acidification, warming, and metal contamination. Three principal components (PCs) explained 74.9% of the original data variance, with coefficients ≥ |0.4| (Table 3, Figure 4). PC1 explained 30.1% of the data variance and showed positive associations among GSH and GST activity in gills, GSH in digestive gland, and AChE activity in muscle. PC2 explained 24.4% of the data variance, showing a positive association for GST enzymatic activity in digestive gland and a negative association for DNA damage and GPx enzymatic activity in gills. For PC3 there was a strong association between LPO levels in gills and GPx enzymatic activity in digestive glands, explaining 20.4% of variances. Overall, the observed PCA results suggest that temperature and metal contamination and temperature are the factors most responsible for the effects. In addition, when the three factors occur simultaneously, they may influence the effects of antioxidant enzymes and neurotoxicity.
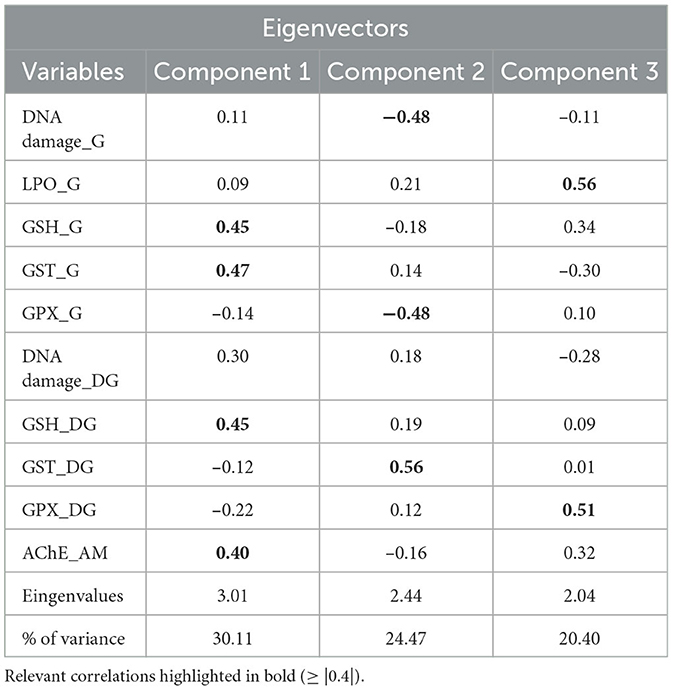
Table 3. Results of principal component analysis (PCA) integrating all data from biomarkers responses for gills (G), digestive gland (DG), and adductor muscle tissues (AM) of the mussel M. charruana exposed to CO2-induced acidification, warming, and metal contamination experimental treatments.
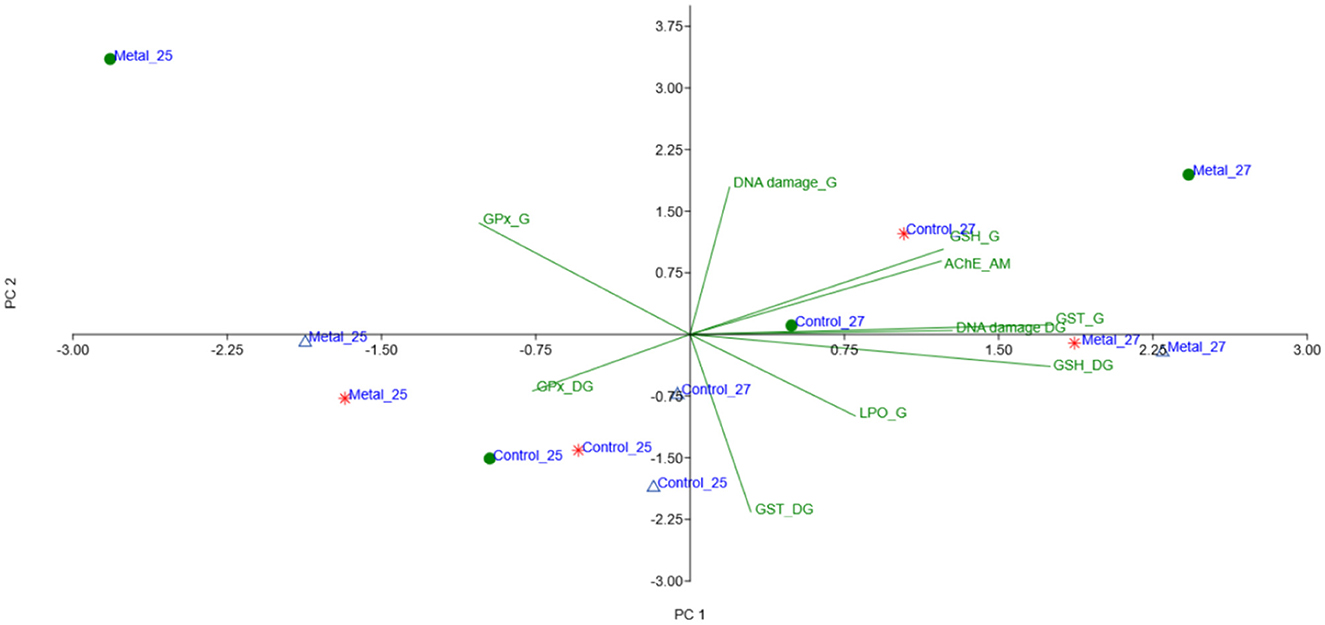
Figure 4. Biplot of PC1 and PC2 based on PCA results, integrating all data from biomarkers responses in gills, digestive glands, and muscle tissues of the mussel Mytella charruana exposed to CO2-induced acidification, warming, and metal contamination experimental treatments. G, gills; DG, digestive gland; AM, adductor muscle. Green circles = pH 7.5; Blue triangles = pH 7.1; Red asterisk = pH 6.7.
3.4 IBRv2i index
3.4.1 IBRv2i in gills tissue
The integration of biomarkers responses showed interaction among temperature and metal contamination (AIC = 424.82, F = 15.67, df = 1, p = 0.002), increasing the IBRv2i index in the control treatments at 27°C compared with 25°C (ptukey = 0.05). In addition, highest alteration IBRv2i index was observed between the control and metal-contaminated treatments at 25°C (ptukey < 0.001) and 27 °C (ptukey = 0.013) (Figure 5A and QQ Plot residuals are detailed in Supplementary Figure 4A). Therefore, these results suggest that temperature and metal contamination were the factors that most influenced the effects in gills of M. charruana.
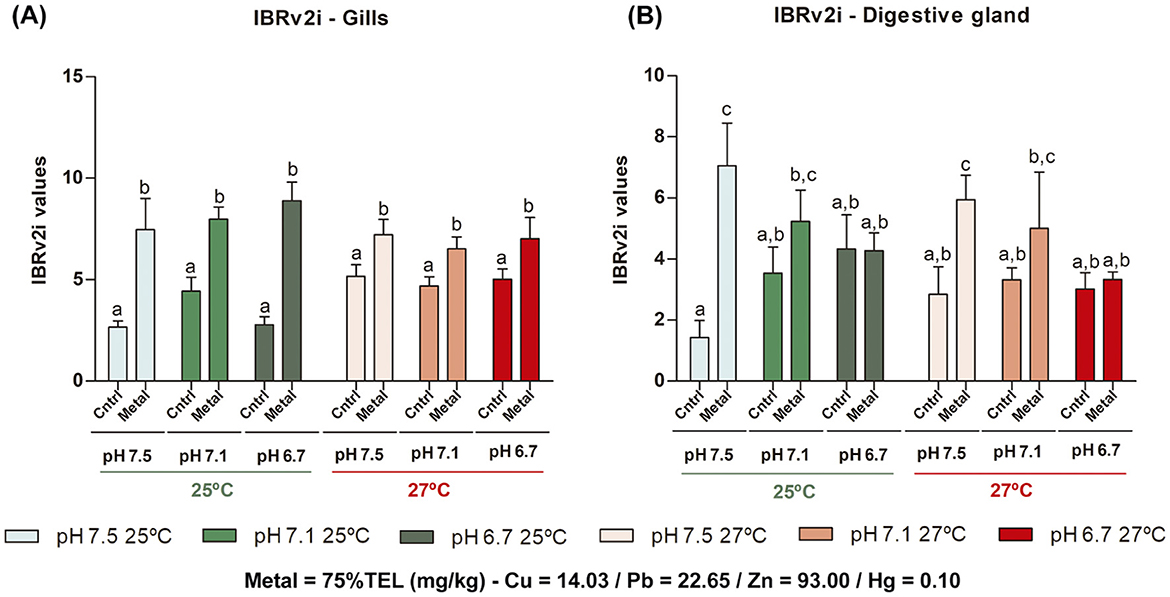
Figure 5. The Integrated Biomarker Response – version 2 individual (IBRv2i) index for (A) gills and (B) the digestive gland tissues of Mytella charruana. Green-toned bars represent treatments at 25°C, and red-toned bars represent treatments at 27°C. Light green and light red bars represent treatments at pH 7.5, green and salmon red bars represent treatments at pH 7.1, and dark green and red bars represent treatments at pH 6.7. Cntrl = control treatments (without metal exposure), and Metal = Cu, Pb, Zn, and Hg sediment contaminated treatments. Capital letters represent significant differences between factors (considering the entire treatment group within the same factor), and lowercase letters represent significant differences between treatments.
3.4.2 IBRv2i in digestive gland tissue
The IBRv2i index for the digestive gland showed a significant difference in the interaction among pH/pCO2 and metal contamination (AIC = 195.65, F = 5.78, df = 2, p = 0.002), showed an increasing in IBRv2i index along with the metal-contaminated treatment in pH 7.5 compared to the respective control (ptukey < 0.001), followed of a decrease in the lowest pH tested (6.7) in the metal-contaminated treatment (ptukey = 0.04) (Figure 5B and QQ Plot residuals are detailed in Supplementary Figure 5B).
4 Discussion
This study demonstrated that the interaction of warming and/or CO2-induced acidification with metal-contaminated sediments, even at concentrations below the threshold effect levels, could alter the antioxidant defense mechanisms of M. charruana within a short exposure period. Overall, significant effects of stressors and their interactions were observed across different soft tissues. The gills and digestive glands were particularly influenced by increased temperature and metal contamination in the sediments. Acidification is recognized as a strong pro-oxidant stressor in bivalves (Dean, 2010; Munari et al., 2018; Romero-Freire et al., 2024; Sun et al., 2025). In this study, elevated pCO2 primarily exerted an effect when combined with a temperature increase and/or metal exposure. In scenarios involving multiple stressors, including global environmental changes, it is crucial to consider (eco)toxicological interactions at cellular and physiological levels, as they may influence the overall toxicity of metals (Ivanina and Sokolova, 2015; Zeng et al., 2015).
The data showed that warming alone inhibited glutathione peroxidase (GPx) enzymatic activity in the digestive gland (without interaction with metals or increase in pCO2). GPx plays a role in the defense mechanism by catalyzing hydrogen peroxide degradation, thus preventing the accumulation of these radicals in cells (Storey, 1996; Reed, 2013). GPx and some isoforms of glutathione S-transferase (GST) reduce lipid hydroperoxides to alcohol, with the concomitant oxidation of GSH to GSSG (Regoli and Giuliani, 2014). Higher temperatures are likely to increase the release of ROS, thus increasing the risk of oxidative damage, thus an overload of the antioxidant system may occur (Benedetti et al., 2022). Therefore, defenses related to catalyzing hydrogen peroxide degradation were likely compromised, which may have caused the inhibition of GPx activity in this study, probably due to an increase in metabolic processes (Matoo et al., 2021) and/or damage in the defense mechanism. In contrast, exposure to increased temperatures induced GST enzymatic activity in the gills. To neutralize the effects of increased reactive oxygen species (ROS), bivalves can increase antioxidant defenses in cells (Jeeva Priya et al., 2017). GST contributes to detoxification by neutralizing ROS by conjugating them with glutathione so that they can be excreted (Stegeman et al., 1992). Therefore, this induction may indicate the activation of a detoxification mechanism as a result of heat stress, since GST may be one of the most active antioxidant enzymes in mussels and its increased activity could represent a compensatory mechanism for the simultaneous inhibition of other antioxidant enzymes (Vidal-Liñán et al., 2010; Cui et al., 2020), such as the GPx in this study. These findings are supported by the literature, which indicates that temperature is a dominant factor in the physiological regulation of bivalves (Matoo et al., 2021). Although mussels are generally tolerant to temperature stress, when optimum physiological ranges are extrapolated and/or when co-exposure to other stressors occurs, one of the consequences of heat stress may be increased oxidative stress due to the imbalance between the production and detoxification of ROS, such as superoxide, hydroxyl, and hydrogen peroxide, overloading antioxidant enzymes (Abele et al., 2002; Matoo et al., 2013). In line with our results, temperature was the main variable that induced oxidative stress, such as GST in the gills of the mangrove oyster Crassostrea rhizophorae in estuaries from Brazilian coast (Zanette et al., 2006).
In addition to the direct effects on organisms, temperature can also influence susceptibility to metals (Banni et al., 2014; Nardi et al., 2017; Morosetti et al., 2020), as observed in this study, owing to changes in chemical speciation. However, this influence may be limited (Sokolova and Lannig, 2008). The interactions between heat stress and metal exposure are likely driven by additive or synergistic mechanisms, as metal uptake is temperature-dependent owing to increased metabolic demand and membrane permeability (Quinn, 1988; Foulkes, 2000). Additionally, metals can disrupt regulatory mechanisms for essential redox elements and affect the thermal tolerance of organisms (Cosson et al., 2008; Sokolova and Lannig, 2008). Moreover, metals can specifically contribute to the imbalance of antioxidant enzymes by ROS production, either by donating electrons to stress markers or by catalyzing Fenton-type reactions, thereby increasing intracellular ROS levels (Yakovleva et al., 2004; Varotto et al., 2013; Regoli and Giuliani, 2014; Benedetti et al., 2016). This, in turn, can affect the capacity of antioxidant defenses (Regoli and Principato, 1995), further exacerbating oxidative stress in the exposed organisms.
In this study, metal exposure in sediment inhibited GPx and GST enzymatic activities, while increasing GSH levels in the digestive gland of M. charruana, regardless of acidification or warming. This response may indicate a shift in the cellular redox state, which contributes to the elimination of oxyradicals (Viarengo et al., 1990). Mussels can counteract the initial oxidative stress by enhancing antioxidant defense mechanisms (Mocan et al., 2010; Coppola et al., 2018). Consistent with our findings, previous studies have reported the activation of antioxidant responses following exposure to various metals (Verlecar et al., 2007; Coppola et al., 2017; Morais et al., 2023). Analysis of the antioxidant responses in the gills revealed that metal contamination significantly increased GPx enzymatic activity under controlled temperature conditions (25°C). However, when the temperature was raised to 27°C, individuals from the same treatments exhibited significant inhibition of enzymatic activity, suggesting a reduced capacity for hydroperoxide degradation in a warmed environment. These findings are consistent with those of previous studies, such as Benedetti et al. (2016), who reported that the interaction between temperature and Cd reduced GPx enzymatic activity in the digestive gland of the scallop, Adamussium colbecki. Similarly, Coppola et al. (2018) observed limited activation of antioxidant defenses in Mytillus galloprovincialis exposed to Hg at elevated temperatures (21°C), attributing this effect to metabolic downregulation mechanisms. At higher temperatures, organisms may employ strategies, such as valve closure, to reduce metabolism and serve as a defense against thermal stress. Adaptive responses have also been documented in other studies (Anestis et al., 2007; Sokolova et al., 2012; Morosetti et al., 2020). Valve closure is also a common response to other types of stress such as metal exposure (Liao et al., 2005) and low pH (Pynnönen and Huebner, 1995).
Exposure to CO2-induced acidification affects the gills of M. charruana only when combined with warming and/or metal contamination, leading to distinct response patterns. In the case of GSH levels in the gills, although GSH activity increased in the presence of metal contamination at elevated temperatures, a decrease was observed as the pH decreased. This suggests that GSH is rapidly consumed by binding to metals (or metal-induced ROS) for excretion at a rate exceeding its replenishment capacity. Such an imbalance in the antioxidant system could compromise cellular defense against oxidative stress (Franco et al., 2009; Morosetti et al., 2020). In bivalves, exposure to high pCO2 levels has been widely associated with a reduced antioxidant capacity (Wang et al., 2016; Zhang et al., 2022). However, there is no clear consensus in the literature, as some studies have reported an increase in GSH levels under high pCO2 and warming in the gills of Mytilus coruscus (Hu et al., 2015), and in combination with metal contamination and warming also in gills of A. colbecki (Benedetti et al., 2016) and Perna viridis (Verlecar et al., 2007). Conversely, Wang et al. (2016) found that elevated pCO2 (~2,000 ppm) inhibited GSH levels in the digestive gland of Crassostrea gigas, with no observed interaction with other stressors.
Lipid peroxidation is commonly used as an indicator of cellular damage to oxidative stress by the interaction of ROs with cell membrane structures or through the interaction of membranes with electrophiles (Pisoschi et al., 2021). Increased levels of LPO in co-exposure to high pCO2 and metal contamination were observed in the gills. Increased LPO levels were attributed to failures in detoxification mechanisms to prevent cellular damage in the gills of the oyster C. gigas exposed to Cu and acidification (pH 7.6) (Cao et al., 2019). Therefore, it can be attributed that the increase in LPO levels in the current study may be due to effects on antioxidant mechanisms, because despite the increase in GST enzymatic activity in the gills, there was a reduction in GSH levels and GPx enzymatic activity, which probably contributed to a lower cellular protective capacity.
Another harmful effect observed in M. charruana in this study was increased DNA damage in the digestive gland, particularly at 27°C. As discussed earlier, climate change stressors, such as warming and acidification, combined with metal contamination can elevate ROS production. This leads to the induction or inhibition of oxidative enzymes, which can have a range of detrimental effects on the bivalve physiological functions, including lipid peroxidation and DNA damage (Gagné et al., 2008; Pöhlmann et al., 2011; Tomanek, 2011; Izagirre et al., 2014; Múgica et al., 2015; Munari et al., 2018). These findings are consistent with the results of the present study, in which higher levels of LPO were observed in the gills of M. charruana exposed to metal at the lowest pH. An interesting response was observed regarding DNA damage in gills (at 25 and 27°C). Under acidification scenarios (high pCO2 levels and metal contamination), a reduction in DNA damage was observed in organisms exposed to metals. This effect, combined with the LPO results, suggests that despite the occurrence of oxidative damage, it does not reflect DNA fragmentation. Although this response may vary depending on the species and tissue analyzed. Previous studies have correlated this reduction with the activation of antioxidant defenses, reporting an increase of DNA strand breaks in gills and an inhibition in the digestive gland of M. galloprovincialis at low pH (7.0) (Munari et al., 2018). Metals can increase DNA strand breaks by producing ROS through the Fenton reaction (Stohs and Bagchi, 1995), but at the same time, the oxidative stress induced by them can be alleviated in hypercapnia (Ivanina et al., 2013). An increase in pCO2 levels may attenuate the response to oxidative stress caused by metals and warming. The relief of hypercapnia in oxidative stress is attributed to the reaction of H+ ions introduced into the medium with free radicals produced by other stressors, such as temperature and metal contamination, generating water and oxygen, decreasing the availability of ROS, and alleviating oxidative stress (Sampaio et al., 2017; Santos et al., 2022).
AChE is commonly found in nervous tissues, such as neuromuscular junctions (Silman and Sussman, 2005). The current results showed that the presence of a mixture of metals in a heated environment resulted in the significant activation of AChE in the muscle of M. charruana. The available information on the impact of co-exposure of metals and/or warming upon AChE activity is very inconsistent, varying according to the species, tissue and metal chosen. First, a recent study showed that metal ions inhibited AChE activity in M. charruana in the order Hg2+ > Pb2+ > Cd2+ > As2+ > Cu2+ > Zn2+ (Santos et al., 2022). AChE activity in bivalves can be influenced by several abiotic factors, especially temperature (Dellali et al., 2001). In the gills of Mytilus sp. AChE showed higher activity at higher temperatures (~23°C) (Pfeifer et al., 2005), whereas Kamel et al. (2012) observed inhibition of AChE in M. galloprovincialis adults exposed to 18–26°C. Regarding co-exposure to stressors simultaneously, the results also vary. Attig et al. (2014) revealed that the combination of warming (26°C) and metal (Ni) caused inhibition of the AChE enzymatic activity in the digestive gland of M. gallaprovincialis. However, according Boukadida et al. (2022) thermal stress combined with Cu and Ag significantly increased the AChE activity in the larvae of the mussel M. galloprovincialis, corroborating our results. Furthermore, the effects on AChE activity were not influenced by pCO2 in M. charruana in the present study. Qu et al. (2022) observed that the effect of CO2 and Cu on M. galloprovincialis was tissue-dependent, with a greater effect on the gills.
Exposure of multiple stressors simultaneously can affect the physiological response and stress tolerance of marine organisms, especially bivalves (Cherkasov et al., 2007; Nardi et al., 2018; Maar et al., 2018; Maulvault et al., 2019; Khan et al., 2020; Chahouri et al., 2023). In this study, the results of biomarkers in the gills and digestive gland showed a dependence of stressor interaction and the biomarker analyzed, in which the interaction of the effects can induce or inhibit oxidative stress in mussels. For instance, PCA integration showed that temperature modulated most of the antioxidant enzyme responses in all the tissues analyzed. In addition, CO2-induced acidification appears to be a stressor that affects biological responses only in interactions with temperature and/or metal contamination. In a general context, the IBR index results reinforce the biological effects of temperature and/or acidification on metal contamination in both tissues analyzed. Higher IBR values for contamination by a mixture of metals can indicate a significant effect (as seen in gills and digestive glands in the current study), even at concentrations below individual safety levels. Higher IBR values have been associated to contamination exposure (Marigómez et al., 2013; Bertrand et al., 2018) and climate changes (Maulvault et al., 2018; Marques et al., 2020). Interactions between warming and metal contamination may be associated with increased metal absorption through increased metabolism and membrane permeability, affecting the organism's susceptibility to metal contaminants (Lannig et al., 2008; Sokolova and Lannig, 2008; Banni et al., 2014; Coppola et al., 2017; Lan et al., 2020). Previous studies showed that co-exposure to metals and elevated pCO2 can regulate antioxidant mechanisms and attenuate metal-induced ROS generation, in which acidification may antagonize the negative physiological effects of metals on energy metabolism and oxidative stress in bivalves (Tomanek, 2011; Ivanina et al., 2013). Jin et al. (2021) revealed that the negative effects of metals on marine organisms can be alleviated by acidification, leading to a neutral impact of heavy metals in combination with high pCO2. Antagonistic relationships between Cu and pCO2 have also been reported (Marangoni et al., 2019; Marques et al., 2020), and it can also be explained by a CO2-related increase in H+ ions concentration in the cellular environment, which is counterbalanced by acid-base compensation to normalize the intracellular pH. This is because the presence of excess H+ ions due to acidification activates antioxidant defenses to combat ROS (Michaelidis et al., 2007; Tiedke et al., 2013; Heuer and Grosell, 2014; Sampaio et al., 2017, 2018). On the other hand, when bivalves are simultaneously exposed to multiple stressors, intracellular ROS formation can increase, leading to alterations in antioxidant mechanisms and cellular damage (Regoli and Giuliani, 2014; Freitas et al., 2019). Figueiredo et al. (2022) were observed high LPO levels for the mollusk Spisula solida exposed to La metal, warming, and acidification.
Although studies with multiple stressors are important to achieve greater comparability to natural conditions, identifying the factors responsible for this effect is a complex challenge. As discussed previously, a combination of factors can result in synergistic or antagonistic effects depending on the biological response analyzed. Understanding the effects of co-exposure to multiple stressors is essential for predicting their impacts in future scenarios; however, we strongly encourage studies that also test isolated factors and their effects beyond the interaction between them.
5 Conclusion
The results of this study suggest that climate change stressors can influence mussel responses to contamination by a mixture of metals in sediments, even at levels below the TEL; therefore, estuarine organisms tend to be more vulnerable to contamination under climate change scenarios. These findings revealed that warming and metal contamination were the main factors modulating antioxidant responses in the gills and digestive glands, as well as AChE activity in the muscle. When combined with CO2-induced acidification, these stressors also affect DNA integrity and LPO levels. Here, we provide insights into a short-term experiment on the complex interactions between predicted climate change scenarios and exposure to a mixture of metals, suggesting that estuarine organisms tend to be more vulnerable to contamination in climate change scenarios. Similar studies conducted over longer exposure periods and with more specific biological analyses are needed to better understand the mechanisms of the potential effects or adaptations of mussels to climate change scenarios in co-exposure to metal contamination. However, this study suggested that the effects of metal pollution tend to be more severe when combined with ocean warming and acidification. Understanding the effects of climate change in future scenarios on estuarine ecosystems and their biota is essential for guiding decision makers' actions and public conservation policies.
Data availability statement
The raw data supporting the conclusions of this article will be made available by the authors, without undue reservation.
Ethics statement
The animal study was approved by Declaration of Research Project without Human Involvement/Vertebs no. 2041787/2024/Postgraduate Program in Bioproducts and Bioprocesses – declaration of responsibility for the research project. Federal University of São Paulo. The study was conducted in accordance with the local legislation and institutional requirements.
Author contributions
AA: Conceptualization, Formal analysis, Investigation, Methodology, Writing – original draft. PG-C: Investigation, Methodology, Writing – review & editing. GA: Investigation, Methodology, Writing – review & editing. MF: Investigation, Methodology, Writing – review & editing. TT: Investigation, Methodology, Writing – review & editing. CF: Investigation, Methodology, Writing – review & editing. RF: Investigation, Resources, Supervision, Writing – review & editing. DA: Methodology, Resources, Writing – review & editing. RC: Conceptualization, Formal analysis, Funding acquisition, Methodology, Project administration, Supervision, Visualization, Writing – original draft, Writing – review & editing.
Funding
The author(s) declare that financial support was received for the research and/or publication of this article. The authors thank the São Paulo Research Foundation – FAPESP (Grants #2017/07351-4 and #2023/11496-9) and National Council for Scientific and Technological Development – CNPq (Grant #409498/2021-1) for funding the study. AA (#88887.501096/2020-00) and GA (#88887.000767/2024-00) thank Coordination for the Improvement of Higher Education Personnel (CAPES) for their doctoral scholarships. RC (#304844/2022-5), PGC (#304855/2022-7), RF (#313633/2021-5), and DA (#308533/2018-6 and #313420/2023-8) thank CNPq for their research productivity fellowships.
Conflict of interest
The authors declare that the research was conducted in the absence of any commercial or financial relationships that could be construed as a potential conflict of interest.
Generative AI statement
The author(s) declare that no Generative AI was used in the creation of this manuscript.
Publisher's note
All claims expressed in this article are solely those of the authors and do not necessarily represent those of their affiliated organizations, or those of the publisher, the editors and the reviewers. Any product that may be evaluated in this article, or claim that may be made by its manufacturer, is not guaranteed or endorsed by the publisher.
Supplementary material
The Supplementary Material for this article can be found online at: https://www.frontiersin.org/articles/10.3389/focsu.2025.1575728/full#supplementary-material
References
Abele, D., Heise, K., Portner, H. O., and Puntarulo, S. (2002). Temperature-dependence of mitochondrial function and production of reactive oxygen species in the intertidal mud clam Mya arenaria. J. Exp. Biol. 205, 1831–1841. doi: 10.1242/jeb.205.13.1831
Altafim, G. L., Alves, A. V., Trevizani, T. H., Figueira, R. C. L., Gallucci, F., and Choueri, R. B. (2023). Ocean warming and CO2-driven acidification can alter the toxicity of metal-contaminated sediments to the meiofauna community. Sci. Total Environ. 885:163687. doi: 10.1016/j.scitotenv.2023.163687
Alves, A. V., Ferraz, M. A., Moreno, B. B., Nobre, C. R., Antunes, R. M., Pusceddu, F. H., et al. (2022). Microscale toxicity identification evaluation (TIE) for interstitial water of estuarine sediments affected by multiple sources of pollution. Environ. Sci. Pollut. Res. 29, 1–16. doi: 10.1007/s11356-021-15389-x
Alves, A. V., Kureki, R. K., Trevizani, T. H., Figueira, R. C. L., and Choueri, R. B. (2024). Effects of metals in sediment under acidification and temperature rise scenarios on reproduction of the copepod Nitokra sp. Marine Pollut. Bull. 209:117125. doi: 10.1016/j.marpolbul.2024.117125
American Society for Testing and Materials (ASTM) (2014). “Standard test method for measuring the toxicity of sediment-associated contaminants with estuarine and marine invertebrates. E1367-03,” in Annual 346 Book of ASTM Standards (West Conshohocken, PA: American Society for Testing and Materials International), 323–317. doi: 10.1520/E1367-03R14
Anestis, A., Lazou, A., Prtner, H. O., and Michaelidis, B. (2007). Behavioral, metabolic, and molecular stress responses of marine bivalve Mytilus galloprovincialis during long-term acclimation at increasing ambient temperature. Am. J. Physiol. Regul. Integr. Comp. Physiol. 293, R911–R921. doi: 10.1152/ajpregu.00124.2007
Attig, H., Kamel, N., Sforzini, S., Dagnino, A., Jamel, J., Boussetta, H., et al. (2014). Effects of thermal stress and nickel exposure on biomarkers responses in Mytilus galloprovincialis (Lam). Marine Environ. Res. 94, 65–71. doi: 10.1016/j.marenvres.2013.12.006
Bahamon, N., Aguzzi, J., Ahumada-Sempoal, M. Á., Bernardello, R., Reuschel, C., Company, J. B., et al. (2020). Stepped coastal water warming revealed by multiparametric monitoring at NW Mediterranean fixed stations. Sensors 20:2658. doi: 10.3390/s20092658
Banaee, M., Zeidi, A., Mikušková, N., and Faggio, C. (2024). Assessing metal toxicity on crustaceans in aquatic ecosystems: a comprehensive review. Biol. Trace Elem. Res. 202, 1–19. doi: 10.1007/s12011-024-04122-7
Banni, M., Hajer, A., Sforzini, S., Oliveri, C., Boussetta, H., and Viarengo, A. (2014). Transcriptional expression levels and biochemical markers of oxidative stress in Mytilus galloprovincialis exposed to nickel and heat stress. Comp. Biochem. Physiol. Part C Toxicol. Pharmacol. 160, 23–29. doi: 10.1016/j.cbpc.2013.11.005
Basallote, M. D., Rodríguez-Romero, A., Manoela, R., Del Valls, T. Á., and Riba, I. (2015). Evaluation of the threat of marine CO2 leakage-associated acidification on the toxicity of sediment metals to juvenile bivalves. Aquat. Toxicol. 166, 63–71. doi: 10.1016/j.aquatox.2015.07.004
Belivermiş, M., Kiliç, Ö., Gezginci-Oktayoglu, S., Sezer, N., Demiralp, S., Sahin, B., et al. (2023). Physiological and gene expression responses of the mussel Mytilus galloprovincialis to low pH and low dissolved oxygen. Marine Pollut. Bull. 187:114602. doi: 10.1016/j.marpolbul.2023.114602
Belivermiş, M., Warnau, M., Metian, M., Oberhänsli, F., Teyssié, J. L., and Lacoue-Labarthe, T. (2016). Limited effects of increased CO2 and temperature on metal and radionuclide bioaccumulation in a sessile invertebrate, the oyster Crassostrea gigas. ICES J. Marine Sci. 73, 753–763. doi: 10.1093/icesjms/fsv236
Benedetti, M., Giuliani, M. E., Mezzelani, M., Nardi, A., Pittura, L., Gorbi, S., et al. (2022). Emerging environmental stressors and oxidative pathways in marine organisms: current knowledge on regulation mechanisms and functional effects. Biocell 46:37. doi: 10.32604/biocell.2022.017507
Benedetti, M., Lanzoni, I., Nardi, A., d'Errico, G., Di Carlo, M., Fattorini, D., et al. (2016). Oxidative responsiveness to multiple stressors in the key Antarctic species, Adamussium colbecki: interactions between temperature, acidification and cadmium exposure. Mar. Environ. Res. 121, 20–30. doi: 10.1016/j.marenvres.2016.03.011
Beniash, E., Ivanina, A., Lieb, N. S., Kurochkin, I., and Sokolova, I. M. (2010). Elevated level of carbon dioxide affects metabolism and shell formation in oysters Crassostrea virginica. Mar. Ecol. Prog. Ser. 419, 95–108. doi: 10.3354/meps08841
Bertrand, L., Monferrán, M. V., Mouneyrac, C., and Amé, M. V. (2018). Native crustacean species as a bioindicator of freshwater ecosystem pollution: a multivariate and integrative study of multi-biomarker response in active river monitoring. Chemosphere 206, 265–277. doi: 10.1016/j.chemosphere.2018.05.002
Bindoff, N. L., Cheung, W., Kairo, J. G., Aristegui, J., Guinder, V. A., Hallberg, R., et al. (2022). “Changing ocean, marine ecosystems, and dependent communities,” in The Ocean and Cryosphere in a Changing Climate: Special Report of the Intergovernmental Panel on Climate Change (Cambridge University Press), 447–588. doi: 10.1017/9781009157964.007
Boukadida, K., Banni, M., Romero-Ramirez, A., Clerandeau, C., Gourves, P. Y., and Cachot, J. (2022). Metal contamination and heat stress impair swimming behavior and acetylcholinesterase activity in embryo-larval stages of the Mediterranean mussel, Mytilus galloprovincialis. Mar. Environ. Res. 179:105677. doi: 10.1016/j.marenvres.2022.105677
Boyd, P. W., Collins, S., Dupont, S., Fabricius, K., Gattuso, J. P., Havenhand, J., et al. (2018). Experimental strategies to assess the biological ramifications of multiple drivers of global ocean change-a review. Glob. Change Biol. 24, 2239–2261. doi: 10.1111/gcb.14102
Boyd, R. S. (2010). Heavy metal pollutants and chemical ecology: exploring new frontiers. J. Chem. Ecol. 36, 46–58. doi: 10.1007/s10886-009-9730-5
Bradford, M. M. (1976). A rapid and sensitive method for the quantitation of microgram quantities of protein utilizing the principle of protein-dye binding. Anal. Biochem. 72, 248–254. doi: 10.1016/0003-2697(76)90527-3
Byrne, P. A., and O'halloran, J. (2001). The role of bivalve molluscs as tools in estuarine sediment toxicity testing: a review. Hydrobiologia 465, 209–217. doi: 10.1023/A:1014584607501
Caldeira, K., and Wickett, M. E. (2003). Anthropogenic carbon and ocean pH. Nature 425, 365–365. doi: 10.1038/425365a
Campana, O., Taylor, A. M., Blasco, J., Maher, W. A., and Simpson, S. L. (2015). Importance of subcellular metal partitioning and kinetics to predicting sublethal effects of copper in two deposit-feeding organisms. Environ. Sci. Technol. 49, 1806–1814. doi: 10.1021/es505005y
Canadian Council of Ministers of the Environment (CCME) (2002). Canadian Sediment Quality Guidelines for the Protection of Aquatic Life, Summary Tables. Canadian Environmental Quality Guidelines. Winnipeg. Available online at: https://ccme.ca/en/summary-table (accessed March 07, 2022).
Cao, R., Liu, Y., Wang, Q., Dong, Z., Yang, D., Liu, H., et al. (2018). Seawater acidification aggravated cadmium toxicity in the oyster Crassostrea gigas: metal bioaccumulation, subcellular distribution and multiple physiological responses. Sci. Total Environ. 642, 809–823. doi: 10.1016/j.scitotenv.2018.06.126
Cao, R., Zhang, T., Li, X., Zhao, Y., Wang, Q., Yang, D., et al. (2019). Seawater acidification increases copper toxicity: a multi-biomarker approach with a key marine invertebrate, the Pacific Oyster Crassostrea gigas. Aqua. Toxicol. 210, 167–178. doi: 10.1016/j.aquatox.2019.03.002
Cao, R., Zhang, Y., Ju, Y., Wang, W., Xi, C., Liu, W., et al. (2022). Exacerbation of copper pollution toxicity from ocean acidification: a comparative analysis of two bivalve species with distinct sensitivities. Environ. Pollut. 293:118525. doi: 10.1016/j.envpol.2021.118525
Chahouri, A., Yacoubi, B., Moukrim, A., and Banaoui, A. (2023). Bivalve molluscs as bioindicators of multiple stressors in the marine environment: recent advances. Cont. Shelf Res. 264:105056. doi: 10.1016/j.csr.2023.105056
Chandurvelan, R., Marsden, I. D., Glover, C. N., and Gaw, S. (2015). Assessment of a mussel as a metal bioindicator of coastal contamination: relationships between metal bioaccumulation and multiple biomarker responses. Sci. Total Environ. 511, 663–675. doi: 10.1016/j.scitotenv.2014.12.064
Cherkasov, A. S., Grewal, S., and Sokolova, I. M. (2007). Combined effects of temperature and cadmium exposure on haemocyte apoptosis and cadmium accumulation in the eastern oyster Crassostrea virginica (Gmelin). J. Ther. Biol. 32, 162–170. doi: 10.1016/j.jtherbio.2007.01.005
Cherkasov, A. S., Taylor, C., and Sokolova, I. M. (2010). Seasonal variation in mitochondrial responses to cadmium and temperature in eastern oysters Crassostrea virginica (Gmelin) from different latitudes. Aqua. Toxicol. 97, 68–78. doi: 10.1016/j.aquatox.2009.12.004
Clements, J. C., Hicks, C., Tremblay, R., and Comeau, L. A. (2018). Elevated seawater temperature, not pCO2, negatively affects post-spawning adult mussels (Mytilus edulis) under food limitation. Conserv. Physiol. 6:cox078. doi: 10.1093/conphys/cox078
Coppola, F., Almeida, Â., Henriques, B., Soares, A. M., Figueira, E., Pereira, E., et al. (2017). Biochemical impacts of Hg in Mytilus galloprovincialis under present and predicted warming scenarios. Sci. Total Environ. 601, 1129–1138. doi: 10.1016/j.scitotenv.2017.05.201
Coppola, F., Almeida, Â., Henriques, B., Soares, A. M., Figueira, E., Pereira, E., et al. (2018). Biochemical responses and accumulation patterns of Mytilus galloprovincialis exposed to thermal stress and Arsenic contamination. Ecotoxicol. Environ. Safety 147, 954–962. doi: 10.1016/j.ecoenv.2017.09.051
Cosson, R. P., Thiébaut, É., Company, R., Castrec-Rouelle, M., Colaço, A., Martins, I., et al. (2008). Spatial variation of metal bioaccumulation in the hydrothermal vent mussel Bathymodiolus azoricus. Mar. Environ. Res. 65, 405–415. doi: 10.1016/j.marenvres.2008.01.005
Cotovicz Júnior, L. C., Marins, R. V., and Abril, G. (2022). “Coastal ocean acidification in Brazil: a brief overview and perspective,” in Arquivos de Ciências do Mar, Fortaleza, eds. Rossi, S., Cotovicz Júnior, L. C., Sousa, P., Tavares, T., Teixeira, C. 55, 141–162. doi: 10.32360/acmar.v55iEspecial.78514
Cui, W., Cao, L., Liu, J., Ren, Z., Zhao, B., and Dou, S. (2020). Effects of seawater acidification and cadmium on the antioxidant defense of flounder Paralichthys olivaceus larvae. Sci. Total Environ. 718:137234. doi: 10.1016/j.scitotenv.2020.137234
Dean, J. B. (2010). Hypercapnia causes cellular oxidation and nitrosation in addition to acidosis: implications for CO2 chemoreceptor function and dysfunction. J. Appl. Physiol. 108, 1786–1795. doi: 10.1152/japplphysiol.01337.2009
Dellali, M., Barelli, M. G., Romeo, M., and Aissa, P. (2001). The use of acetylcholinesterase activity in Ruditapes decussatus and Mytilus galloprovincialis in the biomonitoring of Bizerta lagoon. Comp. Biochem. Physiol. Part C Toxicol. Pharmacol. 130, 227–235. doi: 10.1016/S1532-0456(01)00245-9
Depledge, M. H., Aagaard, A., and Györkös, P. (1995). Assessment of trace metal toxicity using molecular, physiological and behavioural biomarkers. Mar. Pollut. Bull. 31, 19–27. doi: 10.1016/0025-326X(95)00006-9
Dickinson, G. H., Matoo, O. B., Tourek, R. T., Sokolova, I. M., and Beniash, E. (2013). Environmental salinity modulates the effects of elevated CO2 levels on juvenile hard-shell clams, Mercenaria mercenaria. J. Exp. Biol. 216, 2607–2618. doi: 10.1242/jeb.082909
Dickson, A. G. (1990). Thermodynamics of the dissociation of boric acid in synthetic seawater from 273.15 to 318.15 K. Deep Sea Res. Part A. Oceanogr. Res. Papers 37, 755–766. doi: 10.1016/0198-0149(90)90004-F
Dickson, A. G., and Millero, F. J. (1987). A comparison of the equilibrium constants for the dissociation of carbonic acid in seawater media. Deep Sea Research Part A. Oceanographic Research Papers 34, 1733–1743. doi: 10.1016/0198-0149(87)90021-5
Duarte, C., Navarro, J. M., Acuña, K., Torres, R., Manríquez, P. H., Lardies, M. A., et al. (2014). Combined effects of temperature and ocean acidification on the juvenile individuals of the mussel Mytilus chilensis. J. Sea Res. 85, 308–314. doi: 10.1016/j.seares.2013.06.002
Ellman, G. L., Courtney, K. D., Andres Jr, V., and Featherstone, R. M. (1961). A new and rapid colorimetric determination of acetylcholinesterase activity. Biochem. Pharmacol. 7, 88–95. doi: 10.1016/0006-2952(61)90145-9
Ferraz, M. A., Kiyama, A. C., Primel, E. G., Barbosa, S. C., Castro, Í. B., Choueri, R. B., et al. (2022). Does pH variation influence the toxicity of organic contaminants in estuarine sediments? Effects of Irgarol on nematode assemblages. Sci. Total Environ. 815:152944. doi: 10.1016/j.scitotenv.2022.152944
Figueiredo, C., Grilo, T. F., Oliveira, R., Ferreira, I. J., Gil, F., Lopes, C., et al. (2022). Single and combined ecotoxicological effects of ocean warming, acidification and lanthanum exposure on the surf clam (Spisula solida). Chemosphere 302:134850. doi: 10.1016/j.chemosphere.2022.134850
Foulkes, E. C. (2000). Transport of toxic heavy metals across cell membranes (44486). Proc. Soc. Exp. Biol. Med. 223, 234–240. doi: 10.1046/j.1525-1373.2000.22334.x
Franco, R., Sánchez-Olea, R., Reyes-Reyes, E. M., and Panayiotidis, M. I. (2009). Environmental toxicity, oxidative stress and apoptosis: menage a trois. Mutat. Res. Genet. Toxicol. Environ. Mutagen. 674, 3–22. doi: 10.1016/j.mrgentox.2008.11.012
Freitas, R., De Marchi, L., Bastos, M., Moreira, A., Velez, C., Chiesa, S., et al. (2017). Effects of seawater acidification and salinity alterations on metabolic, osmoregulation and oxidative stress markers in Mytilus galloprovincialis. Ecol. Indic. 79, 54–62. doi: 10.1016/j.ecolind.2017.04.003
Freitas, R., Leite, C., Pinto, J., Costa, M., Monteiro, R., Henriques, B., et al. (2019). The influence of temperature and salinity on the impacts of lead in Mytilus galloprovincialis. Chemosphere 235, 403–412. doi: 10.1016/j.chemosphere.2019.05.221
Friedlingstein, P., O'sullivan, M., Jones, M. W., Andrew, R. M., Hauck, J., Olsen, A., et al. (2020). Global carbon budget 2020. Earth Syst. Sci. Data Discuss. 12, 1–3. doi: 10.5194/essd-12-3269-2
Gagné, F., Auclair, J., Turcotte, P., Fournier, M., Gagnon, C., Sauv,é, S., et al. (2008). Ecotoxicity of CdTe quantum dots to freshwater mussels: impacts on immune system, oxidative stress and genotoxicity. Aqua. Toxicol. 86, 333–340. doi: 10.1016/j.aquatox.2007.11.013
Gagné, F., and Blaise, C. (1995). Evaluation of the genotoxicity of environmental contaminants in sediments to rainbow trout hepatocytes. Environ. Toxicol. Water Q. 10, 217–229. doi: 10.1002/tox.2530100309
Gallucci, M. (2019). GAMLJ: general analyses for linear models. [jamovi module]. Available online at: https://gamlj.github.io/ (accessed November 12, 2024).
Garcia-Soto, C., Cheng, L., Caesar, L., Schmidtko, S., Jewett, E. B., Cheripka, A., et al. (2021). An overview of ocean climate change indicators: sea surface temperature, ocean heat content, ocean pH, dissolved oxygen concentration, arctic sea ice extent, thickness and volume, sea level and strength of the AMOC (Atlantic Meridional Overturning Circulation). Front. Mar. Sci. 8:642372. doi: 10.3389/fmars.2021.642372
Halsband, C., Thomsen, N., and Reinardy, H. C. (2024). Climate Change increases the risk of metal toxicity in Arctic zooplankton. Front. Marine Sci. 11:1510718. doi: 10.3389/fmars.2024.1510718
Hammer, Ø., Harper, D. A. T., and Ryan, P. D. (2001). Past: paleontological statistics software package for education and data analysis [software]. Available online at: https://palaeo-electronica.org/2001_1/past/past.pdf (accessed November 23, 2024).
Harvey, B. P., Gwynn-Jones, D., and Moore, P. J. (2013). Meta-analysis reveals complex marine biological responses to the interactive effects of ocean acidification and warming. Ecol. Evol. 3, 1016–1030. doi: 10.1002/ece3.516
Heuer, R. M., and Grosell, M. (2014). Physiological impacts of elevated carbon dioxide and ocean acidification on fish. Am. J. Physiol. Regul. Integr. Comp. Physiol. 307, R1061–R1084. doi: 10.1152/ajpregu.00064.2014
Hirota, J., and Szyper, J. P. (1975). Separation of total particulate carbon into inorganic and organic components 1. Limnol. Oceanogr. 20, 896–900. doi: 10.4319/lo.1975.20.5.0896
Hu, M., Li, L., Sui, Y., Li, J., Wang, Y., Lu, W., et al. (2015). Effect of pH and temperature on antioxidant responses of the thick shell mussel Mytilus coruscus. Fish Shellfish Immunol. 46, 573–583. doi: 10.1016/j.fsi.2015.07.025
Hwang, H. M., Wade, T. L., and Sericano, J. L. (2008). Residue-response relationship between PAH body burdens and lysosomal membrane destabilization in eastern oysters (Crassostrea virginica) and toxicokinetics of PAHs. J. Environ. Scie. Health Part A. 43, 1373–1380. doi: 10.1080/10934520802232006
IPCC Intergovernmental Panel on Climate Change (2014). “Climate change 2014: synthesis report,” in Contribution of Working Groups I, II and III to the Fifth Assessment Report of the Intergovernmental Panel on Climate Change, eds. Core Writing Team, R. K. Pachauri, L. A. Meyer (Geneva: IPCC Oxford University Press), 151. doi: 10.10013/epic.45156.d.001
IPCC Intergovernmental Panel on Climate Change (2021). “Summary for policymakers,” in Climate Change 2021: The Physical Science Basis. Contribution of Working Group I to the Sixth Assessment Report of the Intergovernmental Panel on Climate Change, 2, eds. V. Masson-Delmotte, P. Zhai, Y. Chen, A. Pirani, S. L. Connors, C. P'ean, et al. (New York, NY: Cambridge University Press), 3–32. doi: 10.1017/9781009157896.001
IPCC Intergovernmental Panel on Climate Change (2022). “Climate change 2022: impacts, adaptation, and vulnerability,” in Contribution of Working Group II to the Sixth Assessment Report of the Intergovernmental Panel on Climate Change, eds. H.-O. Pörtner, D. C. Roberts, M. Tignor, E. S. Poloczanska, K. Mintenbeck, A. Alegría, et al. (Cambridge, UK; New York, NY: Cambridge University Press), 3056. doi: 10.1017/9781009325844
Ivanina, A. V., Beniash, E., Etzkorn, M., Meyers, T. B., Ringwood, A. H., and Sokolova, I. M. (2013). Short-term acute hypercapnia affects cellular responses to trace metals in the hard clams Mercenaria mercenaria. Aqua. Toxicol. 140, 123–133. doi: 10.1016/j.aquatox.2013.05.019
Ivanina, A. V., and Sokolova, I. M. (2015). Interactive effects of metal pollution and ocean acidification on physiology of marine organisms. Curr. Zool. 61, 653–668. doi: 10.1093/czoolo/61.4.653
Izagirre, U., Errasti, A., Bilbao, E., Múgica, M., and Marigómez, I. (2014). Combined effects of thermal stress and Cd on lysosomal biomarkers and transcription of genes encoding lysosomal enzymes and HSP70 in mussels, Mytilus galloprovincialis. Aqua. Toxicol. 149, 145–156. doi: 10.1016/j.aquatox.2014.01.013
Jamovi Project (2022). Jamovi (Version 2.3) [Computer Software]. Sydney. Available online at: https://www.jamovi.org
Jeeva Priya, R., Anand, M., Maruthupandy, M., and Hameedha Beevi, A. (2017). Biomarker response of climate change-induced ocean acidification and hypercapnia studies on brachyurian crab Portunus pelagicus. Glob. J. Environ. Sci. Manage. 3, 165–176. doi: 10.22034/gjesm.2017.03.02.005
Jin, P., Zhang, J., Wan, J., Overmans, S., Gao, G., Ye, M., et al. (2021). The combined effects of ocean acidification and heavy metals on marine organisms: a meta-analysis. Front. Marine Sci. 8:801889. doi: 10.3389/fmars.2021.801889
Kamel, N., Attig, H., Dagnino, A., Boussetta, H., and Banni, M. (2012). Increased temperatures affect oxidative stress markers and detoxification response to benzo [a] pyrene exposure in mussel Mytilus galloprovincialis. Arch. Environ. Contam. Toxicol. 63, 534–543. doi: 10.1007/s00244-012-9790-3
Keen, J. H., Habig, W. H., and Jakoby, W. B. (1976). Mechanism for the several activities of the glutathione S-transferases. J. Biol. Chem. 251, 6183–6188. doi: 10.1016/S0021-9258(20)81842-0
Khan, F. U., Hu, M., Kong, H., Shang, Y., Wang, T., Wang, X., et al. (2020). Ocean acidification, hypoxia and warming impair digestive parameters of marine mussels. Chemosphere 256:127096. doi: 10.1016/j.chemosphere.2020.127096
Kim, B. S. M., Salaroli, A. B., de Lima Ferreira, P. A., Sartoretto, J. R., de Mahiques, M. M., and Figueira, R. C. L. (2016). Spatial distribution and enrichment assessment of heavy metals in surface sediments from Baixada Santista, Southeastern Brazil. Mar. Pollut. Bull. 103, 333–338. doi: 10.1016/j.marpolbul.2015.12.041
Lacoue-Labarthe, T., Reveillac, E., Oberhänsli, F., Teyssié, J. L., Jeffree, R., and Gattuso, J. P. (2011). Effects of ocean acidification on trace element accumulation in the early-life stages of squid Loligo vulgaris. Aqua. Toxicol. 105, 166–176. doi: 10.1016/j.aquatox.2011.05.021
Lan, W. R., Huang, X. G., Lin, L. X., Li, S. X., and Liu, F. J. (2020). Thermal discharge influences the bioaccumulation and bioavailability of metals in oysters: implications of ocean warming. Environ. Pollut. 259:113821. doi: 10.1016/j.envpol.2019.113821
Lannig, G., Cherkasov, A. S., Portner, H. O., Bock, C., and Sokolova, I. M. (2008). Cadmium-dependent oxygen limitation affects temperature tolerance in eastern oysters (Crassostrea virginica Gmelin). Am. J. Physiol. Regul. Integr. Comp. Physiol. 294, R1338–R1346. doi: 10.1152/ajpregu.00793.2007
Lannig, G., Flores, J. F., and Sokolova, I. M. (2006). Temperature-dependent stress response in oysters, Crassostrea virginica: pollution reduces temperature tolerance in oysters. Aqua. Toxicol. 79, 278–287. doi: 10.1016/j.aquatox.2006.06.017
Levitus, S., Antonov, J., and Boyer, T. (2005). Warming of the world ocean, 1955–2003. Geophy. Res. Lett. 32:L02604. doi: 10.1029/2004GL021592
Liao, C. M., Jou, L. J., and Chen, B. C. (2005). Risk-based approach to appraise valve closure in the clam Corbicula fluminea in response to waterborne metals. Environ. Pollut. 135, 41–52. doi: 10.1016/j.envpol.2004.10.015
Long, E. R. (2006). Calculation and uses of mean sediment quality guideline quotients: a critical review. Environ. Sci. Technol. 40, 1726–1736. doi: 10.1021/es058012d
Long, E. R., and MacDonald, D. D. (1998). Recommended uses of empirically derived, sediment quality guidelines for marine and estuarine ecosystems. Human Ecol. Risk Assess. 4, 1019–1039. doi: 10.1080/10807039891284956
Lough, J. M., Anderson, K. D., and Hughes, T. P. (2018). Increasing thermal stress for tropical coral reefs: 1871–2017. Sci. Rep. 8:6079. doi: 10.1038/s41598-018-24530-9
Lu, Y., Yuan, J., Lu, X., Su, C., Zhang, Y., Wang, C., et al. (2018). Major threats of pollution and climate change to global coastal ecosystems and enhanced management for sustainability. Environ. Poll. 239, 670–680. doi: 10.1016/j.envpol.2018.04.016
Luczak, C., Janquin, M. A., and Kupka, A. (1997). Simple standard procedure for the routine determination of organic matter in marine sediment. Hydrobiologia 345, 87–94. doi: 10.1023/A:1002902626798
Maar, M., Larsen, M. M., Tørring, D., and Petersen, J. K. (2018). Bioaccumulation of metals (Cd, Cu, Ni, Pb and Zn) in suspended cultures of blue mussels exposed to different environmental conditions. Estuar. Coast. Shelf Sci. 201, 185–197. doi: 10.1016/j.ecss.2015.10.010
Mangan, S., Urbina, M. A., Findlay, H. S., Wilson, R. W., and Lewis, C. (2017). Fluctuating seawater pH/p CO2 regimes are more energetically expensive than static pH/p CO2 levels in the mussel Mytilus edulis. Proc. R. Soc. B. Biol. Sci. 284:20171642. doi: 10.1098/rspb.2017.1642
Marangoni, L. F. B., Pinto, M. M. D. A. N., Marques, J. A., and Bianchini, A. (2019). Copper exposure and seawater acidification interaction: antagonistic effects on biomarkers in the zooxanthellate scleractinian coral Mussismilia harttii. Aqua. Toxicol. 206, 123–133. doi: 10.1016/j.aquatox.2018.11.005
Marigómez, I., Garmendia, L., Soto, M., Orbea, A., Izagirre, U., and Cajaraville, M. P. (2013). Marine ecosystem health status assessment through integrative biomarker indices: a comparative study after the Prestige oil spill “Mussel Watch”. Ecotoxicology 22, 486–505. doi: 10.1007/s10646-013-1042-4
Marques, J. A., Abrantes, D. P., Marangoni, L. F., and Bianchini, A. (2020). Ecotoxicological responses of a reef calcifier exposed to copper, acidification and warming: a multiple biomarker approach. Environ. Pollut. 257:113572. doi: 10.1016/j.envpol.2019.113572
Matoo, O. B., Ivanina, A. V., Ullstad, C., Beniash, E., and Sokolova, I. M. (2013). Interactive effects of elevated temperature and CO2 levels on metabolism and oxidative stress in two common marine bivalves (Crassostrea virginica and Mercenaria mercenaria). Comp. Biochem. Physiol. Part A. Mol. Integr. Physiol. 164, 545–553. doi: 10.1016/j.cbpa.2012.12.025
Matoo, O. B., Lannig, G., Bock, C., and Sokolova, I. M. (2021). Temperature but not ocean acidification affects energy metabolism and enzyme activities in the blue mussel, Mytilus edulis. Ecol. Evol. 11, 3366–3379. doi: 10.1002/ece3.7289
Matozzo, V., Chinellato, A., Munari, M., Bressan, M., and Marin, M. G. (2013). Can the combination of decreased pH and increased temperature values induce oxidative stress in the clam Chamelea gallina and the mussel Mytilus galloprovincialis? Mar. Pollut. Bull. 72, 34–40. doi: 10.1016/j.marpolbul.2013.05.004
Mattos, J. J., Siebert, M. N., and Bainy, A. C. D. (2024). Integrated biomarker responses: a further improvement of IBR and IBRv2 indexes to preserve data variability in statistical analyses. Environ. Sci. Pollut. Res. 31, 871–881. doi: 10.1007/s11356-023-31255-4
Maulvault, A. L., Barbosa, V., Alves, R., Custódio, A., Anacleto, P., Repolho, T., et al. (2017). Ecophysiological responses of juvenile seabass (Dicentrarchus labrax) exposed to increased temperature and dietary methylmercury. Sci. Total Environ. 586, 551–558. doi: 10.1016/j.scitotenv.2017.02.016
Maulvault, A. L., Camacho, C., Barbosa, V., Alves, R., Anacleto, P., Fogaça, F., et al. (2018). Assessing the effects of seawater temperature and pH on the bioaccumulation of emerging chemical contaminants in marine bivalves. Environ. Res. 161, 236–247. doi: 10.1016/j.envres.2017.11.017
Maulvault, A. L., Camacho, C., Barbosa, V., Alves, R., Anacleto, P., Pousão-Ferreira, P., et al. (2019). Living in a multi-stressors environment: an integrated biomarker approach to assess the ecotoxicological response of meagre (Argyrosomus regius) to venlafaxine, warming and acidification. Environ. Res. 169, 7–25. doi: 10.1016/j.envres.2018.10.021
Maulvault, A. L., Custodio, A., Anacleto, P., Repolho, T., Pousão, P., Nunes, M. L., et al. (2016). Bioaccumulation and elimination of mercury in juvenile seabass (Dicentrarchus labrax) in a warmer environment. Environ. Res. 149, 77–85. doi: 10.1016/j.envres.2016.04.035
Mehrbach, C., Culberson, C. H., Hawley, J. E., and Pytkowicx, R. M. (1973). Measurement of the apparent dissociation constants of carbonic acid in seawater at atmospheric pressure 1. Limnol. Oceanogr. 18, 897–907. doi: 10.4319/lo.1973.18.6.0897
Michaelidis, B., Ouzounis, C., Paleras, A., and Pörtner, H. O. (2005). Effects of long-term moderate hypercapnia on acid-base balance and growth rate in marine mussels Mytilus galloprovincialis. Mar. Ecol. Progr. Ser. 293, 109–118. doi: 10.3354/meps293109
Michaelidis, B., Spring, A., and Pörtner, H. O. (2007). Effects of long-term acclimation to environmental hypercapnia on extracellular acid-base status and metabolic capacity in Mediterranean fish Sparus aurata. Mar. Biol. 150, 1417–1429. doi: 10.1007/s00227-006-0436-8
Millero, F. J., Woosley, R., DiTrolio, B., and Waters, J. (2009). Effect of ocean acidification on the speciation of metals in seawater. Oceanography 22, 72–85. doi: 10.5670/oceanog.2009.98
Mocan, T., Clichici, S., Agoşton-Coldea, L., Mocan, L., Simon, S., Ilie, I., et al. (2010). Implications of oxidative stress mechanisms in toxicity of nanoparticles. Acta Physiol. Hung. 97, 247–255. doi: 10.1556/APhysiol.97.2010.3.1
Monserrat, J. M., Martínez, P. E., Geracitano, L. A., Amado, L. L., Martins, C. M. G., Pinho, G. L. L., et al. (2007). Pollution biomarkers in estuarine animals: critical review and new perspectives. Comp. Biochem. Physiol. Part C. Toxicol. Pharmacol. 146, 221–234. doi: 10.1016/j.cbpc.2006.08.012
Morais, T., Moleiro, P., Leite, C., Coppola, F., Pinto, J., Henriques, B., et al. (2023). Ecotoxicological impacts of metals in single and co-exposure on mussels: comparison of observable and predicted results. Sci. Total Environ. 881:163165. doi: 10.1016/j.scitotenv.2023.163165
Morosetti, B., Freitas, R., Pereira, E., Hamza, H., Andrade, M., Coppola, F., et al. (2020). Will temperature rise change the biochemical alterations induced in Mytilus galloprovincialis by cerium oxide nanoparticles and mercury? Environ. Res. 188:109778. doi: 10.1016/j.envres.2020.109778
Múgica, M., Izagirre, U., and Marigómez, I. J. A. T. (2015). Lysosomal responses to heat-shock of seasonal temperature extremes in Cd-exposed mussels. Aquat. Toxicol. 164, 99–107. doi: 10.1016/j.aquatox.2015.04.020
Munari, M., Matozzo, V., Gagn,é, F., Chemello, G., Riedl, V., Finos, L., et al. (2018). Does exposure to reduced pH and diclofenac induce oxidative stress in marine bivalves? A comparative study with the mussel Mytilus galloprovincialis and the clam Ruditapes philippinarum. Environ. Pollut. 240, 925–937. doi: 10.1016/j.envpol.2018.05.005
Nardi, A., Benedetti, M., d'Errico, G., Fattorini, D., and Regoli, F. (2018). Effects of ocean warming and acidification on accumulation and cellular responsiveness to cadmium in mussels Mytilus galloprovincialis: importance of the seasonal status. Aquat. Toxicol. 204, 171–179. doi: 10.1016/j.aquatox.2018.09.009
Nardi, A., Mincarelli, L. F., Benedetti, M., Fattorini, D., d'Errico, G., and Regoli, F. (2017). Indirect effects of climate changes on cadmium bioavailability and biological effects in the Mediterranean mussel Mytilus galloprovincialis. Chemosphere 169, 493–502. doi: 10.1016/j.chemosphere.2016.11.093
Nikinmaa, M., and Anttila, K. (2015). Responses of marine animals to ocean acidification. Clim. Change Mar. Freshwater Toxins 99–124. doi: 10.1515/9783110333596-005
Noyes, P. D., McElwee, M. K., Miller, H. D., Clark, B. W., Van Tiem, L. A., Walcott, K. C., et al. (2009). The toxicology of climate change: environmental contaminants in a warming world. Environ. Int. 35, 971–986. doi: 10.1016/j.envint.2009.02.006
Olive, P. L. (1988). DNA precipitation assay: a rapid and simple method for detecting DNA damage in mammalian cells. Environ. Mol. Mutagen. 11, 487–495. doi: 10.1002/em.2850110409
Pfeifer, S., Schiedek, D., and Dippner, J. W. (2005). Effect of temperature and salinity on acetylcholinesterase activity, a common pollution biomarker, in Mytilus sp. from the south-western Baltic Sea. J. Exp. Mar. Biol. Ecol. 320, 93–103. doi: 10.1016/j.jembe.2004.12.020
Pierrot, D. E., Wallace, D. W. R., and Lewis, E. (2006). MS Excel Program Developed for CO2 System Calculations. Oak Ridge, TN: Carbon Dioxide Information Analysis Center, O.R.N.L. U.S. Department of Energy.
Pinho, J. V., Willmer, I. Q., Conte-Junior, C. A., Lopes, A. P., Saint'Pierre, T. D., dos Santos, E. G., et al. (2024). Differential metal and metalloid contents in fresh and cooked Charu mussels (Mytella charruana) from a southeastern Brazilian estuary and associated human health risks. Food Control 166:110700. doi: 10.1016/j.foodcont.2024.110700
Pisoschi, A. M., Pop, A., Iordache, F., Stanca, L., Predoi, G., and Serban, A. I. (2021). Oxidative stress mitigation by antioxidants-an overview on their chemistry and influences on health status. Eur. J. Med. Chem. 209:112891. doi: 10.1016/j.ejmech.2020.112891
Pöhlmann, K., Koenigstein, S., Alter, K., Abele, D., and Held, C. (2011). Heat-shock response and antioxidant defense during air exposure in Patagonian shallow-water limpets from different climatic habitats. Cell Stress Chaperones 16, 621–632. doi: 10.1007/s12192-011-0272-8
Pörtner, H. O., Langenbuch, M., and Reipschläger, A. (2004). Biological impact of elevated ocean CO2 concentrations: lessons from animal physiology and earth history. J. Oceanogr. 60, 705–718. doi: 10.1007/s10872-004-5763-0
Portugal, M. C. S., Altafim, G. L., de Jesus, S. B., Alves, A. V., Rojas, L. A. V., Zanardi-Lamardo, E., et al. (2025). Toxicity of PAHs-enriched sediments on meiobenthic communities under ocean warming and CO2-driven acidification scenarios. Mar. Pollut. Bull. 212:117489. doi: 10.1016/j.marpolbul.2024.117489
Pynnönen, K. S., and Huebner, J. (1995). Effects of episodic low pH exposure on the valve movements of the freshwater bivalve Anodonta cygnea L. Water Res. 29, 2579–2582. doi: 10.1016/0043-1354(95)00111-W
Qu, Y., Zhang, T., Zhang, R., Wang, X., Zhang, Q., Wang, Q., et al. (2022). Integrative assessment of biomarker responses in Mytilus galloprovincialis exposed to seawater acidification and copper ions. Sci. Total Environ. 851:158146. doi: 10.1016/j.scitotenv.2022.158146
Quinn, G. P., and Keough, M. J. (2002). Experimental Design and Data Analysis for Biologists. Cambridge: Cambridge University Press. doi: 10.1017/CBO9780511806384
Range, P., Piló, D., Ben-Hamadou, R., Chícharo, M. A., Matias, D., Joaquim, S., et al. (2012). Seawater acidification by CO2 in a coastal lagoon environment: effects on life history traits of juvenile mussels Mytilus galloprovincialis. J. Exp. Mar. Biol. Ecol. 424, 89–98. doi: 10.1016/j.jembe.2012.05.010
Reed, D. J. (2013). Regulation of reductive processes by glutathione. Biochem. Pharmacol. 35, 7–13. doi: 10.1016/0006-2952(86)90545-9
Regoli, F., and Giuliani, M. E. (2014). Oxidative pathways of chemical toxicity and oxidative stress biomarkers in marine organisms. Mar. Environ. Res. 93, 106–117. doi: 10.1016/j.marenvres.2013.07.006
Regoli, F., and Principato, G. (1995). Glutathione, glutathione-dependent and antioxidant enzymes in mussel, Mytilus galloprovincialis, exposed to metals under field and laboratory conditions: implications for the use of biochemical biomarkers. Aquat. Toxicol. 31, 143–164. doi: 10.1016/0166-445X(94)00064-W
Roberts, D. A., Birchenough, S. N., Lewis, C., Sanders, M. B., Bolam, T., and Sheahan, D. (2013). Ocean acidification increases the toxicity of contaminated sediments. Glob. Change Biol. 19, 340–351. doi: 10.1111/gcb.12048
Rocha, T. L., Sabóia-Morais, S. M. T., and Bebianno, M. J. (2016). Histopathological assessment and inflammatory response in the digestive gland of marine mussel Mytilus galloprovincialis exposed to cadmium-based quantum dots. Aquat. Toxicol. 177:306–315. doi: 10.1016/j.aquatox.2016.06.003
Romero-Freire, A., De Marchi, L., Freitas, R., Velo, A., Babarro, J. M., and Cobelo-García, A. (2024). Ocean acidification impact on the uptake of trace elements by mussels and their biochemical effects. Aquat. Toxicol. 269:106882. doi: 10.1016/j.aquatox.2024.106882
Ross, P. M., Parker, L., and Byrne, M. (2016). Transgenerational responses of molluscs and echinoderms to changing ocean conditions. ICES J. Mar. Sci. 73, 537–549. doi: 10.1093/icesjms/fsv254
Sampaio, E., Lopes, A. R., Francisco, S., Paula, J. R., Pimentel, M., Maulvault, A. L., et al. (2017). Ocean acidification dampens warming and contamination effects on the physiological stress response of a commercially important fish. Biogeosci. Discuss. 2017, 1–27. doi: 10.5194/bg-2017-147
Sampaio, E., Lopes, A. R., Francisco, S., Paula, J. R., Pimentel, M., Maulvault, A. L., et al. (2018). Ocean acidification dampens physiological stress response to warming and contamination in a commercially-important fish (Argyrosomus regius). Sci. Total Environ. 618, 388–398. doi: 10.1016/j.scitotenv.2017.11.059
Sanchez, W., Burgeot, T., and Porcher, J. M. (2013). A novel “Integrated biomarker response” calculation based on reference deviation concept. Environ. Sci. Pollut. Res. 20, 2721–2725. doi: 10.1007/s11356-012-1359-1
Santos, G. P. C., de Assis, C. R. D., Oliveira, V. M., Cahu, T. B., Silva, V. L., Santos, J. F., et al. (2022). Acetylcholinesterase from the charru mussel Mytella charruana: kinetic characterization, physicochemical properties and potential as in vitro biomarker in environmental monitoring of mollusk extraction areas. Comp. Biochem. Physiol. Part C. Toxicol. Pharmacol. 252:109225. doi: 10.1016/j.cbpc.2021.109225
Sedlak, J., and Lindsay, R. H. (1968). Estimation of total, protein-bound, and nonprotein sulfhydryl groups in tissue with Ellman's reagent. Anal. Biochem. 25, 192–205. doi: 10.1016/0003-2697(68)90092-4
Sies, H., Koch, O. R., Martino, E., and Boveris, A. (1979). Increased biliary glutathione disulfide release in chronically ethanol-treated rats. Febs. Lett. 103, 287–290. doi: 10.1016/0014-5793(79)81346-0
Silman, I., and Sussman, J. L. (2005). Acetylcholinesterase: 'classical'and 'non-classical' functions and pharmacology. Curr. Opin. Pharmacol. 5, 293–302. doi: 10.1016/j.coph.2005.01.014
Sokolova, I. M., Frederich, M., Bagwe, R., Lannig, G., and Sukhotin, A. A. (2012). Energy homeostasis as an integrative tool for assessing limits of environmental stress tolerance in aquatic invertebrates. Mar. Environ. Res. 79, 1–15. doi: 10.1016/j.marenvres.2012.04.003
Sokolova, I. M., and Lannig, G. (2008). Interactive effects of metal pollution and temperature on metabolism in aquatic ectotherms: implications of global climate change. Clim. Res. 37, 181–201. doi: 10.3354/cr00764
Sokolova, I. M., Matoo, O. B., Dickinson, G. H., Beniash, E., Solan, M., and Whiteley, N. M. (2016). Physiological effects of ocean acidification on animal calcifiers. Stress. Mar. Environ. Physiol. Ecol. Responses Soc. Implic. 36–55. doi: 10.1093/acprof:oso/9780198718826.003.0003
Stegeman, J. J., Marius, B., Di Giulio, R. T., Lars, F., Fowler, B. A., Sanders, B. M., et al. (1992). “Molecular responses to environmental contamination: enzyme and protein systems as indicators of chemical exposure and effect,” in Biomarkers: Biochemical, Physiological and Histological markers of Anthropogenic Stress, eds. R. J. Huggett, R. A. Kimerle, P. M. Mehrle Jr., and P. M. Bergman (Boca Raton, FL: Lewis Publishers), 235–336.
Stohs, S. J., and Bagchi, D. (1995). Oxidative mechanisms in the toxicity of metal ions. Free Rad. Biol. Med. 18, 321–336. doi: 10.1016/0891-5849(94)00159-H
Storey, K. B. (1996). Oxidative stress: animal adaptations in nature. Bra. J. Med. Biol. Res. 29, 1715–1733.
Sun, Y., Ramstein, G., Fedorov, A. V., Ding, L., and Liu, B. (2025). Tropical Indian Ocean drives Hadley circulation change in a warming climate. Natl. Sci. Rev. 12:nwae375. doi: 10.1093/nsr/nwae375
Thomsen, J., Casties, I., Pansch, C., Körtzinger, A., and Melzner, F. (2013). Food availability outweighs ocean acidification effects in juvenile Mytilus edulis: laboratory and field experiments. Glob. Change Biol. 19, 1017–1027. doi: 10.1111/gcb.12109
Tiedke, J., Cubuk, C., and Burmester, T. (2013). Environmental acidification triggers oxidative stress and enhances globin expression in zebrafish gills. Biochem. Biophys. Res. Commun. 441, 624–629. doi: 10.1016/j.bbrc.2013.10.104
Tomanek, L. (2011). Environmental proteomics: changes in the proteome of marine organisms in response to environmental stress, pollutants, infection, symbiosis, and development. Annu. Rev. Mar. Sci. 3, 373–399. doi: 10.1146/annurev-marine-120709-142729
Trevisan, R., and Mello, D. F. (2024). Redox control of antioxidants, metabolism, immunity, and development at the core of stress adaptation of the oyster Crassostrea gigas to the dynamic intertidal environment. Free Rad. Biol. Med. 210, 85–106. doi: 10.1016/j.freeradbiomed.2023.11.003
Trevizani, T. H., Domit, C., Santos, M. C. D. O., and Figueira, R. C. L. (2023). Bioaccumulation of heavy metals in estuaries in the southwest Atlantic Ocean. Environ. Sci. Pollut. Res. 30, 26703–26717. doi: 10.1007/s11356-022-23974-x
Tsai, L. J., Yu, K. C., Chen, S. F., and Kung, P. Y. (2003). Effect of temperature on removal of heavy metals from contaminated river sediments via bioleaching. Water Res. 37, 2449–2457. doi: 10.1016/S0043-1354(02)00634-6
United States Environmental Protection Agency (USEPA) (1996). Method 3050B: Acid Digestion of Sediments, Sludges, and Soils. Revision 2. Washington, DC: Environmental Protection Agency. Available online at: https://www.epa.gov/esam/epa-method-3050b-acid-digestion-sediments-sludges-and-soils (accessed March 28, 2024).
United States Environmental Protection Agency (USEPA) (2001). Method 823-B-01-002: Methods for Collection, Storage and Manipulation of Sediments for Chemical and Toxicological Analyses: Technical Manual. Washington: USEPA.
United States Environmental Protection Agency (USEPA) (2007). Method 6010C (SW-846): Inductively Coupled Plasma-Atomic Emission Spectrometry, Revision 3. Washington, DC: Environmental Protection Agency. Available online at: https://archive.epa.gov/epawaste/hazard/testmethods/web/pdf/method%206010c%2c%20revision%203%20-%202007.pdf (accessed April 2, 2024).
Varotto, L., Domeneghetti, S., Rosani, U., Manfrin, C., Cajaraville, M. P., Raccanelli, S., et al. (2013). DNA damage and transcriptional changes in the gills of Mytilus galloprovincialis exposed to nanomolar doses of combined metal salts (Cd, Cu, Hg). PLoS ONE 8:e54602. doi: 10.1371/journal.pone.0054602
Verlecar, X. N., Jena, K. B., and Chainy, G. B. N. (2007). Biochemical markers of oxidative stress in Perna viridis exposed to mercury and temperature. Chem. Biol. Interact. 167, 219–226. doi: 10.1016/j.cbi.2007.01.018
Viarengo, A., Canesi, L., Pertica, M., Poli, G., Moore, M. N., and Orunesu, M. (1990). Heavy metal effects on lipid peroxidation in the tissues of Mytilus galloprovincialis lam. Comp. Biochem. Physiol. Part C Comp. Pharmacol. 97, 37–42. doi: 10.1016/0742-8413(90)90168-9
Vidal-Liñán, L., Bellas, J., Campillo, J. A., and Beiras, R. (2010). Integrated use of antioxidant enzymes in mussels, Mytilus galloprovincialis, for monitoring pollution in highly productive coastal areas of Galicia (NW Spain). Chemosphere 78, 265–272. doi: 10.1016/j.chemosphere.2009.10.060
Wang, Q., Cao, R., Ning, X., You, L., Mu, C., Wang, C., et al. (2016). Effects of ocean acidification on immune responses of the Pacific oyster Crassostrea gigas. Fish Shellfish Immunol. 49, 24–33. doi: 10.1016/j.fsi.2015.12.025
Wang, Z., Wang, Y., Zhao, P., Chen, L., Yan, C., Yan, Y., et al. (2015). Metal release from contaminated coastal sediments under changing pH conditions: implications for metal mobilization in acidified oceans. Mar Pollut Bull. 101, 707–715.doi: 10.1016/j.marpolbul.2015.10.026
Wentworth, C. K. (1922). A scale of grade and class terms for clastic sediments. J. Geol. 30, 377–392. doi: 10.1086/622910
Wills, E. D. (1987). “Evaluation of lipid peroxidation in lipids and biological membranes,” in Biochemical Toxicology: A Practical Approach, eds. K. Snell and B. Mullock (Washington: IRL Press), 127–150.
Yakovleva, I., Bhagooli, R., Takemura, A., and Hidaka, M. (2004). Differential susceptibility to oxidative stress of two scleractinian corals: antioxidant functioning of mycosporine-glycine. Comp. Biochem. Physiol. Part B Biochem. Mol. Biol. 139, 721–730. doi: 10.1016/j.cbpc.2004.08.016
Yuan, W. S., Walters, L. J., Brodsky, S. A., Schneider, K. R., and Hoffman, E. A. (2016). Synergistic effects of salinity and temperature on the survival of two nonnative bivalve molluscs, Perna viridis (Linnaeus 1758) and Mytella charruana (d'Orbigny 1846). J. Mar. Sci. 2016:9261309. doi: 10.1155/2016/9261309
Zanette, J., Monserrat, J. M., and Bianchini, A. (2006). Biochemical biomarkers in gills of mangrove oyster Crassostrea rhizophorae from three Brazilian estuaries. Comp. Biochem. Physiol. Part C Toxicol. Pharmacol. 143, 187–195. doi: 10.1016/j.cbpc.2006.02.001
Zeng, X., Chen, X., and Zhuang, J. (2015). The positive relationship between ocean acidification and pollution. Mar. Pollut. Bull. 91, 14–21. doi: 10.1016/j.marpolbul.2014.12.001
Zhang, H., Tang, D., He, J., Yang, X., Feng, Z., Fu, Y., et al. (2024). Assessment of heavy metal contamination in the surface sediments, seawater and organisms of the Pearl River Estuary, South China Sea. Sci. Total Environ. 950:175266. doi: 10.1016/j.scitotenv.2024.175266
Zhang, L., Wang, X., Zhang, W., Yin, X., Liu, Q., and Qiu, L. (2022). Impact of ocean acidification on physiology and microbiota in hepatopancreas of Pacific oyster Crassostrea gigas. J. Oceanol. Limnol. 40, 620–633. doi: 10.1007/s00343-021-0462-x
Zhang, Y., Song, J., Yuan, H., Xu, Y., He, Z., and Duan, L. (2010). Biomarker responses in the bivalve (Chlamys farreri) to exposure of the environmentally relevant concentrations of lead, mercury, copper. Environ. Toxicol. Pharmacol. 30, 19–25. doi: 10.1016/j.etap.2010.03.008
Keywords: biomarkers, ocean acidification, ocean warming, antioxidants defenses, contamination, ecotoxicology
Citation: Alves AV, Gusso-Choueri PK, Altafim GL, Ferraz MA, Trevizani TH, Felix CSA, Figueira RCL, Abessa DMdS and Choueri RB (2025) Influence of CO2-induced acidification and temperature increased on the toxicity of metals in sediment in the mussel Mytella charruana. Front. Ocean Sustain. 3:1575728. doi: 10.3389/focsu.2025.1575728
Received: 12 February 2025; Accepted: 18 March 2025;
Published: 04 April 2025.
Edited by:
Vinicius Tavares Kutter, Federal University of Pará, BrazilReviewed by:
Baoxiao Qu, Chinese Academy of Sciences (CAS), ChinaMüller L., Federal University of Rio Grande, Brazil
Copyright © 2025 Alves, Gusso-Choueri, Altafim, Ferraz, Trevizani, Felix, Figueira, Abessa and Choueri. This is an open-access article distributed under the terms of the Creative Commons Attribution License (CC BY). The use, distribution or reproduction in other forums is permitted, provided the original author(s) and the copyright owner(s) are credited and that the original publication in this journal is cited, in accordance with accepted academic practice. No use, distribution or reproduction is permitted which does not comply with these terms.
*Correspondence: Aline Vecchio Alves, YWxpbmVlLnZlY2NoaW9hbHZlc0BnbWFpbC5jb20=