- 1AZTI, Marine Research, Basque Research and Technology Alliance (BRTA), Herrera Kaia, Portualdea z/g, Pasaia, Gipuzkoa, Spain
- 2Departament de Biologia Marina i Oceanografia, Institut de Ciències del Mar (CSIC), Pg. Marítim de la Barceloneta, Barcelona, Spain
- 3Marine Biology Station Piran, National Institute of Biology, Piran, Slovenia
- 4Continuous Plankton Recorder Survey Department, Marine Biological Association, Plymouth, United Kingdom
- 5Environmental Monitoring and Biodiversity Conservation Department, ISPRA, Italian Institute for Environmental Protection and Research (ISPRA), Rome, Italy
- 6Marine Scotland Science, Marine Laboratory, Aberdeen, United Kingdom
- 7Department of Marine Sciences, University of the Aegean, Mytilene, Greece
- 8Department of Ecoscience, Aarhus University, Roskilde, Denmark
- 9Hellenic Centre for Marine Research, Institute of Oceanography, Athens, Greece
- 10Ikerbasque – Basque Foundation for Science, Bilbao, Spain
- 11National Institute for Marine Research and Development “Grigore Antipa”, Constanta, Romania
- 12Faculty of Oceanography and Geography, University of Gdansk, Gdyńia, Poland
- 13Department of Ecology, School of Biology, Aristotle University of Thessaloniki, Thessaloniki, Greece
Marine harmful algal blooms (HABs), caused by various aquatic microalgae, pose significant risks to ecosystems, some socio-economic activities and human health. Traditionally managed as a public health issue through reactive control measures such as beach closures, seafood trade bans or closure of mollusc production areas, the multifaceted linkages of HABs with environmental and socio-economic factors require more comprehensive ecosystem-based management approach tools to support policies. This study promotes a coordinated understanding and implementation of HAB assessment and management under the Marine Strategy Framework Directive (MSFD), targeting the achievement of Good Environmental Status (GES) in European marine waters. We introduce two novel tools: GES4HABs (GES for HABs) decision tree, and MAMBO (environMental mAtrix for the Management of BlOoms), a decision support matrix. These tools aim to streamline HABs reporting and prioritize resource allocation and management interventions. The GES4HABs decision tree defines a sequence of decision steps to identify HAB management strategies according to their state (evaluated against predefined baselines) and causes (anthropic or natural). MAMBO is proposed to address different HABs and their interaction with human and environmental pressures. The matrix utilizes two axes: natural trophic status and level of human influence, capturing major aspects such as nutrient supply. While acknowledging the limitations of this simplified framework, MAMBO categorizes marine regions into quadrants of varying management viability. Regions with high human influence and eutrophic conditions are identified as most suitable for effective management intervention, whereas regions with minimal or mixed human influence are deemed less amenable to active management. In addition, we explore and describe various indicators, monitoring methods and initiatives that may be relevant to support assessments of HAB status and associated pressures and impacts in the MSFD reporting. Finally, we provide some recommendations to promote the consideration of HABs in ecosystem-based management strategies, intensify efforts for harmonizing and defining best practices of analysis, monitoring and assessment methodologies, and foster international and cross-sectoral coordination to optimize resources, efforts and roles.
1 Introduction
The term “harmful algal blooms” (HABs) refers to ecologically, socio-economically or health-related detrimental events caused by a wide range of taxonomically, physiologically, and ecologically distinct microalgae and macroalgae. Focusing on microalgae, of the approximately 3,400 to 4,000 known species worldwide, only a mere 1–2% are categorized as harmful (Shumway et al., 2018).
Although HABs are best known for their adverse impacts on public health, aquaculture, fisheries, infrastructure, and recreational and tourism activities (Anderson et al., 2012; Brown et al., 2019; Kouakou and Poder, 2019; Young et al., 2020; Karlson et al., 2021; Lenzen et al., 2021), several adverse effects on marine organisms, including molluscs, fish, seabirds, reptiles and marine mammals, are increasingly documented (Landsberg, 2002; Zohdi and Abbaspour, 2019; Rattner et al., 2022). All these impacts significantly contribute to changes in marine ecosystems, their associated services, and human wellbeing (Masó and Garcés, 2006).
HABs are typically classified into three broad categories based on their “mechanisms of harm”: (i) low biomass toxin-producers, which can contaminate seafood, water, and generate aerosols even at low biomass levels, (ii) high-biomass toxin-producers, which can produce similar harmful effects when reaching high concentrations, and (iii) high-biomass non-toxic species, that can cause either hypoxic/anoxic conditions or unpleasant/nuisance foam or gelatinous masses, among other effects (Anderson et al., 2017; Karlson et al., 2021). A comprehensive list of the most frequently described adverse effects (impacts) of HABs on ecosystem services has been compiled in the Supplementary material (Supplementary Table S1).
Identifying the causative factors behind HAB events is a complex endeavor. Most HABs are natural phenomena that have historically occurred in various regions worldwide before human activities altered coastal and marine ecosystems (Hallegraeff et al., 2003). HABs involve a change of phytoplankton assemblages, which can arise are in response to chemical, biological or habitat alterations (Smayda, 2008). Various ecological mechanisms have been suggested to elucidate HABs, including biological life strategies such as mixotrophic behavior, swimming ability, allelopathy effects, multi-resource competition, and prey avoidance (Choi et al., 2023). In some instances, certain types of HABs from across the globe have been associated with distinct anthropogenic pressures, such as nutrient loads (Riegman et al., 1992; Glibert et al., 2005; Heisler et al., 2008; Harrison et al., 2012), intensified human activities (e.g., aquaculture and navigation, see Hallegraeff et al., 2021), habitat modifications (Garcés and Camp, 2012), and climate change (Anderson, 2014; Wells et al., 2015; Glibert and Burkholder, 2018; Glibert, 2020).
The first legislations on HABs appeared in the mid-1990s: for instance, the local Galician Government legally established the monitoring network for marine biotoxins in bivalve molluscs grown in rafts in the Galician Rías (NW Spain) in 1995 (Pazos and Maneiro, 1999), and the US “Harmful Algal Bloom and Hypoxia Research and Control Act” was enacted in 1998. In Europe, HABs are currently managed primarily as a public health issue (Food and Hygiene Regulations [Regulation (EC) 853/2004, 2004; Regulation (EC) 2074/2005, 2005; Regulation (EU) 2019/627, 2019; Regulation (EU) 2021/1709, 2021]), Bathing Water Directive (BWD) (European Commission, 2006), but are also considered within the Water Framework Directive (WFD) (European Commission, 2000). These regulations mandate the monitoring of marine biotoxins and toxic phytoplankton while establishing specific thresholds to trigger control measures, such as beach closures or seafood trade bans (European Food Safety Authority (EFSA), 2009; Serret et al., 2019). Nevertheless, the significant interconnections between HABs and various environmental and socio-economic issues (see Supplementary material 1) highlight the need of a holistic, ecosystem-based approach to manage HABs through appropriate instruments and policies. Furthermore, neglecting HABs management in environmental policies may lead to future environmental challenges if affected socio-economic actors resort to unregulated mitigation measures such as algicide application, ultrasound, clay disposal or biological treatment (Silliman, 2022).
In this context, the European Marine Strategy Framework Directive (MSFD; European Commission, 2008) represents a significant milestone by introducing an ecosystem-based management approach (EBMA) for the sustainable utilization of marine resources and ecosystem services across Europe. The MSFD aims to ensure that, through its implementation by the EU Member States, in coordination with the Regional Sea Conventions (RSCs), a “Good Environmental Status” (GES) of the EU's marine waters is achieved by 2020 (now, 2026) (European Commission, 2020).
In practice, the MSFD is implemented through a six-year adaptive management cycle, starting with (i) an initial assessment of the status of the marine environment and its essential features and characteristics, (ii) an analysis of the prevailing pressures and impacts, and (iii) an economic and social analysis of the sea use (Art. 8 MSFD). In parallel, the determination of GES (Art. 9 MSFD) and a set of environmental targets and associated indicators (Art. 10 MSFD) was established. GES is defined by eleven descriptors that elucidate the conditions indicative of GES attainment. The indicators and reference values are used to assess compliance with GES and to establish adapted monitoring programmes (Art. 11 MSFD) and programmes of measures (Art. 13 MSFD) for preserving or restoring GES conditions. The six-year management cycle allows Member States to periodically review the suitability and effectiveness of their GES determination, environmental targets, indicators, and measures.
HABs received limited attention in the 2012 MSFD initial assessments (Palialexis et al., 2014). In the 2018 reporting phase, only few Member States initiated reporting on HABs (cyanobacteria in the Baltic Sea and southern North Sea, Noctiluca scintillans in the Black Sea, and Phaeocystis spp. in the southern North Sea) (Tornero Alvarez et al., 2023). Although progress is being made, the diverse array of environmental and socio-economic problems associated with HABs in European coastal and offshore waters is inadequately reflected in the reporting (Tornero Alvarez et al., 2023). Indeed, in the European Seas, abiotic conditions such as nutrient availability and hydrodynamics vary significantly between the open sea and coastal areas. This leads to differences in the types of phytoplankton species present and the frequency of their blooms, which can range from seasonal to unpredictable (Garcés and Camp, 2012). The nearshore zones, covered by the WFD assessment, frequently exhibit signs of eutrophication due to substantial nutrient inputs are often sites of phytoplankton blooms and the presence of harmful algal (HA) species (Bricker et al., 2008). Although most of the potential HAB impacts are expected to occur in nearshore areas designated under the WFD and MSFD, some may extend and impact to offshore regions exclusively covered by MSFD.
In this context, the aim of this study is to pave the way for the integration of HABs into EBMA, with a specific focus on the MSFD (without forgetting lessons learnt from WFD). The main objective is to provide policy- and decision-makers with technical guidance and tools that can enhance the monitoring, assessment and management of HABs in marine environments.
The study outcomes include the proposal of two new conceptual decision support tools (a decision tree and a conceptual matrix), an exploration of existing and alternative indicators and monitoring methods potentially useful for HABs, and recommendations for fostering EBMA strategies.
2 Proposed conceptual decision support tools for guiding HAB management
Within the MSFD framework we found the following principles as most relevant for contextualizing HABs (European Commission, 2017, 2020, 2022):
• The MSFD primarily focuses on assessing the overall environmental status of marine ecosystems, with a particular emphasis on evaluating the impacts of human activities.
• GES is not conceived to reflect a pristine status but should encompass prevailing environmental conditions, including natural variability, climate change, past human activities, their pressures and impacts as well as the ecosystem's resilience and capacity for recovery (Claussen et al., 2011).
• Climate change should be regarded as a “shifting baseline” to be integrated into GES determination (Elliott et al., 2015). Even if climate change is acknowledged as a significant pressure across all European marine regions (European Commission, 2020), assessing climate change effects is not a specific objective of the MSFD. Thus, it is important to distinguish wider climate-change impacts from more localized effects caused by other anthropogenic pressures.
• Member States can, based on risk analysis, focus their efforts on the main problems and areas. The exclusion of low-risk areas and issues does not preclude the maintenance of surveillance monitoring for early detection of future deviations.
• The new MSFD framework requires the setting of quantitative “threshold values” grounded in the best available science, aiming for consistent and comparable outcomes among Member States (European Commission, 2017).
• The (re)use of existing monitoring, standards, and methods stipulated in other EU legislation is recommended to avoid redundant processes and unnecessary reporting burden on member states (European Commission, 2020).
Considering these requirements, we have developed a decision tree, hereafter referred to as GES4HABs, and a decision support matrix, hereafter referred to as MAMBO (environMental mAtrix for the Management of BlOoms). GES4HABs breaks down complex decisions into a sequence of more manageable steps, rendering the decision-making process easier to understand and follow (Figure 1). MAMBO is nested within GES4HABs and assists in identifying HABs that are more amenable to management actions, thereby directing efforts and resources efficiently (Figure 2). Hereafter, the consecutive steps and criteria encompassed in GES4HABs, and MAMBO are described.
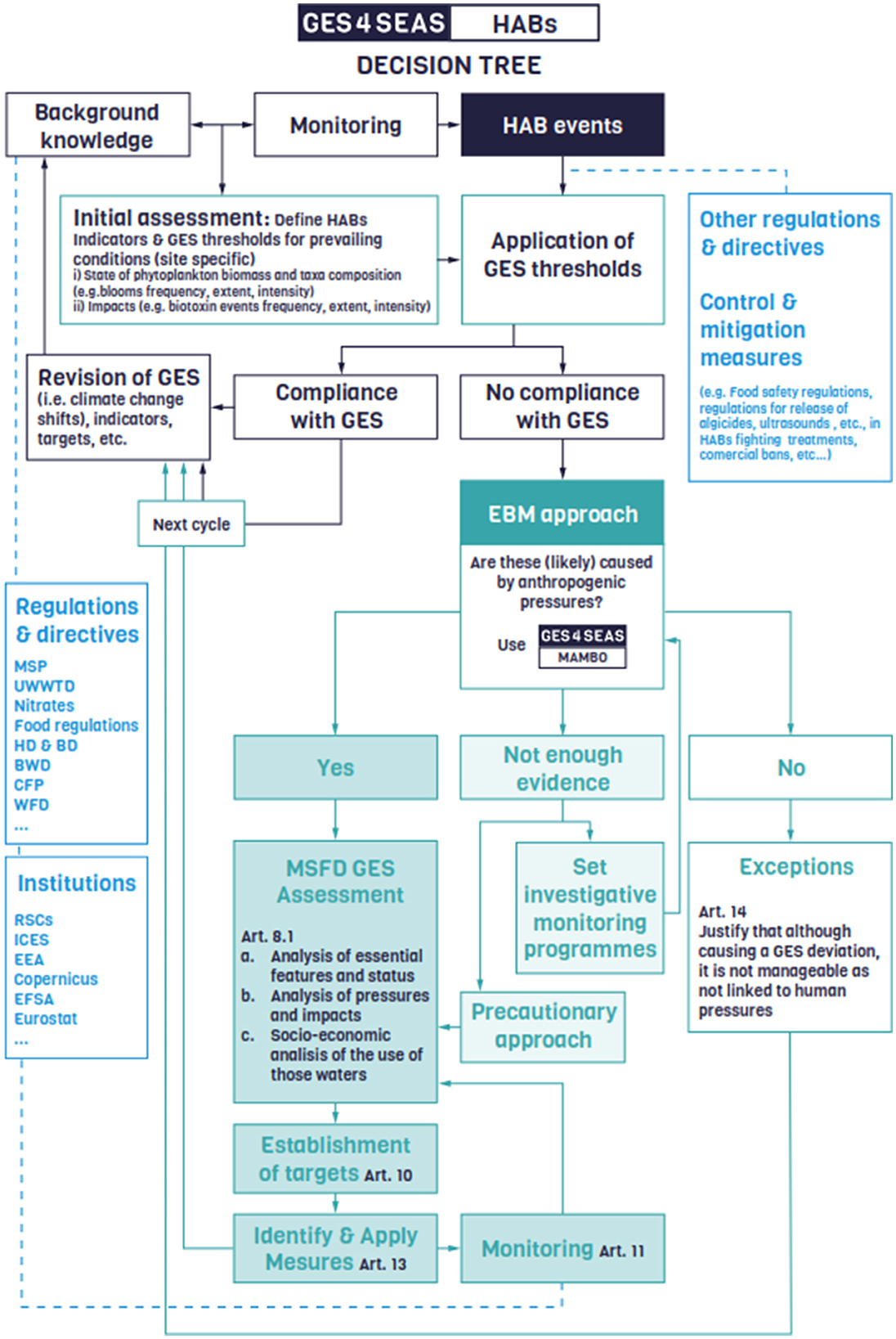
Figure 1. GES4HABs Decision tree to guide policy makers and Marine Strategy Framework Directive (MSFD) stakeholders on the steps and decisions to support management and MSFD reporting actions related to different Harmful Algal Blooms (HABs) in their jurisdiction areas. GES, Good Environmental Status; EBM, Ecosystem-Based Management; RSC, Regional Seas Conventions; ICES, International Council for the Exploration of the Sea; EEA, European Environment Agency; EFSA, European Food Safety Authority; Eurostat, Statistical office of the European Union; MSP, Maritime spatial planning; WFD, Water Framework Directive; UWWTD, Urban Waste Water Treatment Directive; Nitrates, Nitrates Directive; HD and BD, Habitats Directive and Birds Directive; BWD, Bathing Water Directive; CFP, Common Fisheries Policy; MAMBO, environMental mAtrix for the Management of BlOoms (see Figure 2).
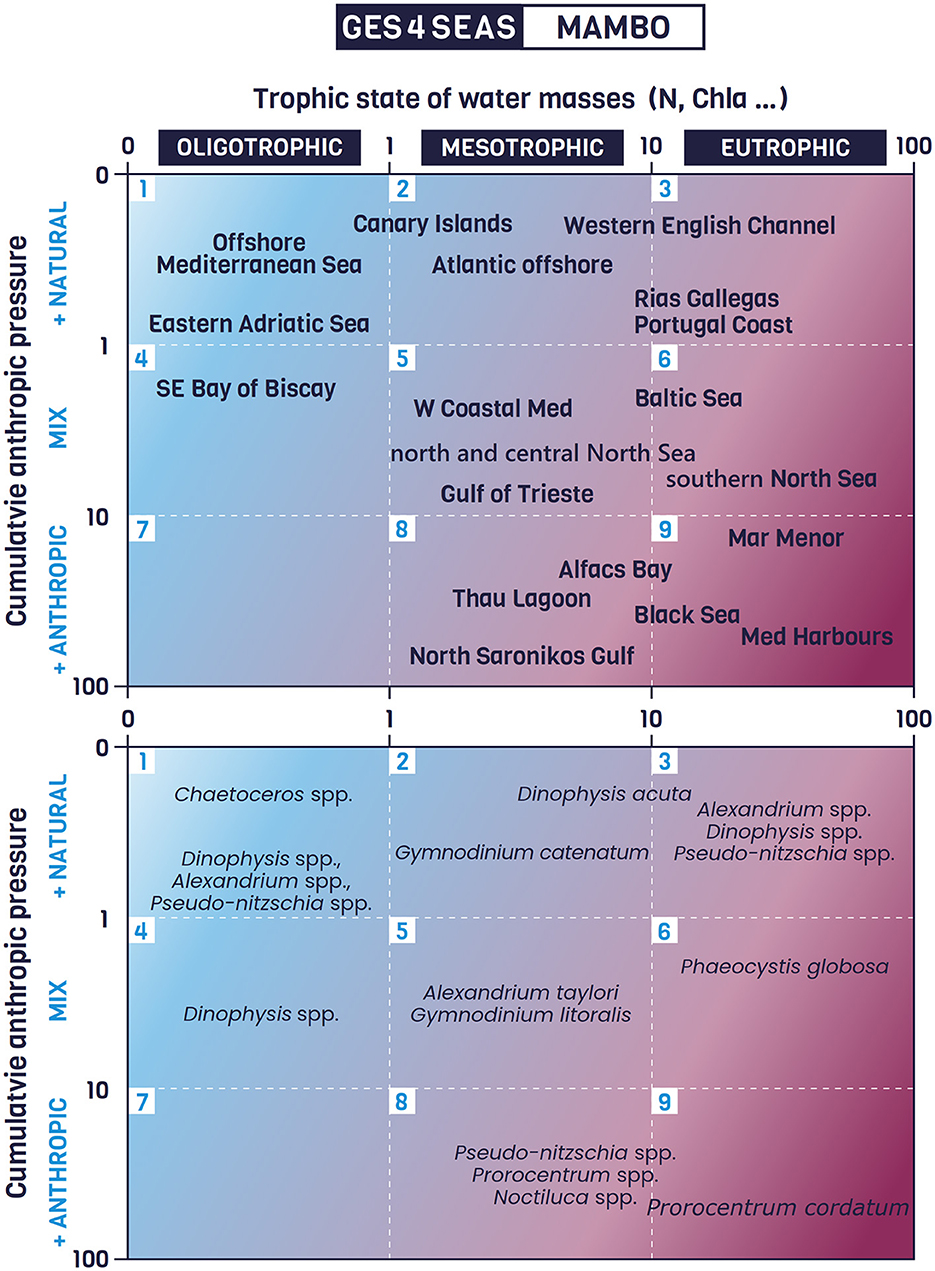
Figure 2. Top: Representation of some marine geographical regions in the MAMBO (environMental mAtrix for the Management of BlOoms) matrix according to their associated trophic state (X axis) and anthropic influence level (Y axis). Bottom: Representation of some examples of Harmful Algal species occurrences in relation to the European marine geographical regions previously represented (Top). The examples added are not meant to hold a comprehensive list of HAB events in European seas but to provide different examples of harmful algae presence and/or events in Europe and exemplify their position in the MAMBO matrix. More examples can be found in Supplementary Table S2.
2.1 GES4HABs entry point: the initial assessment
Although reactive local control measures and regulatory limits will always be necessary to mitigate the impacts and risks of HABs [e.g., Food and Hygiene Regulations [(EC) 853/2004; (EC) 2074/2005; (EU) 2019/627; (EU) 2021/1709], Serret et al. (2019); Bathing Water Directive (European Commission, 2000)] (Figure 1), upstream management based on EBMAs, such as the MSFD, can assist in identifying the causes and impacts of HABs, assess their status against the expected prevailing conditions, and define appropriate management measures. Ultimately, EBMA aims to preserve or enhance ecological integrity, resilience, the provision of ecosystem services, stakeholder engagement and accountability, and transdisciplinary integrated management (Delacámara et al., 2020).
The first step in implementing this approach involves leveraging the local experts' background knowledge of HABs, along with existing monitoring data and infrastructure, to conduct an initial/preliminary assessment (Figure 1). During this initial assessment, appropriate indicators, reference conditions (e.g., those corresponding to the prevailing environmental conditions that determine GES), and thresholds (e.g., those indicating the boundaries between GES and non-GES) should be established to assess HAB events in a given area (WG GES, 2011).
This initial phase could also serve to identify inadequate or inconsistent monitoring efforts and techniques needed to establish effective indicators and reference points (Zampoukas et al., 2014). This may be particularly relevant in the context of rapidly changing conditions due to climate change and the expanding and intensifying human footprint in the assessed areas (e.g., due to aquaculture, coastal modifications, shipping, mining, fishing, and recreation).
The required indicators for this initial assessment can focus on the status of HABs (e.g., phytoplankton biomass, taxa composition, frequency, extent, and duration of blooms) or their impacts (e.g., biotoxin concentrations, frequency and number of closed mariculture sites, and organism mortality) (Figure 1). This choice should be driven by the characteristics of the HABs occurring in the area, the available monitoring networks, and possibilities for inter-comparison (see Section 3 for further information on HAB-related indicators).
While this initial assessment is challenging and resource-intensive, it is essential to (i) identify and account for HAB occurrences in the assessed areas, (ii) determine whether HABs deviate from prevailing conditions, and (iii) anticipate potential new HABs or shifts in baselines due to global change.
The second step commences with a new reporting phase, during which the HABs recorded in the reporting period should be compared to the reference values for prevailing conditions (GES thresholds) set in the initial assessment (Figure 1). If HABs fall within these thresholds, reporters can confirm compliance with GES, justify the lack of need for additional management measures, and maintain the existing surveillance monitoring and response control measures. Conversely, if the assessment indicates a departure from GES, a potential concern arises, leading to the next question: Are these HABs (likely) linked to anthropogenic causes and therefore manageable within the policy framework? (Figure 1).
2.2 Use of MAMBO to address the complexity of environmental factors and human influence in the management of HABs
At present, establishing clear links between HABs and anthropogenic pressures is challenging due to several factors: (i) the complexity of the mechanisms that trigger HABs, (ii) the interaction of multiple pressures, both human and natural, and their cumulative effects (additive, synergistic, or antagonistic), and (iii) changing conditions due to climate change.
In this context, MAMBO is proposed to pragmatically delineate the manageable environment within which policy makers and environmental managers can direct their efforts and resources. MAMBO intersects the natural trophic status of marine waters, ranging from oligotrophic to highly eutrophic systems (X axis), with their level of anthropic influence (Y axis) (Figure 2). For the quantitative application of MAMBO, these axes can be customized with different metrics. The 'trophic state' axis could be determined by average chlorophyll a concentration values or any other metric deemed appropriate to represent prevailing conditions. Similarly, different metrics could be chosen for the cumulative anthropic pressure axis. This choice may be based upon user preferences, data availability, and regional characteristics (e.g., freshwater content, land use indices, or other anthropic indicators).
These axes were chosen because most connections between human activities and HABs are related to nutrient status. Although the link with over enrichment of nutrients is more often associated with high-biomass HABs, the effect of nutrients in promoting HABs (including toxic HABs) is neither uniform nor straightforward (Masó and Garcés, 2006; Smayda, 2008; Gowen et al., 2012). Establishing a direct link between trophic status and HABs is challenging, especially when attributing this status to either natural or anthropogenic sources is required. In addition, this link is often context dependent with the co-occurrence of other factors or pressures (Smayda, 2008; Davidson et al., 2014). For instance, nutrient reduction policies leading to oligotrophication could increase Paralytic Shellfish Poisoning (PSP) events in warm shelf systems (Walsh et al., 2011) and Mediterranean lagoons (Collos et al., 2009). Additionally, changes in nutrient stoichiometry may favor some harmful species, as observed in areas of intensive bivalve cultivation (Brown et al., 2019) or after dam construction (Humborg et al., 1997). Apart from climate change, other anthropic pressures may also contribute to HABs, such as the transmission of species via ballast waters (Brown et al., 2019; Karlson et al., 2021), the decline of top predators due to fishing (Walsh et al., 2011), the introduction of cysts in the water column due to dredging operations (Carrada et al., 1991; Feki et al., 2022), and the construction of structures that affect hydrodynamics, such as harbors and dikes (Garcés and Camp, 2012; Karlson et al., 2021). These pressures may even interact to produce larger effects (Ferreira et al., 2011).
Recognizing that the selected axes do not fully capture the complex interactions between different pressures and HABs but only the most relevant ones, MAMBO can depict different quadrants associated with different levels of management viability. When different geographical marine regions and different HAB species occurrences are represented in MAMBO (Figure 2), those falling within highly anthropized and eutrophic quadrants (quadrants numbered 5, 6, 8 and 9) have the potential for effective management intervention. In the remaining quadrants (1, 2, 3, 4 and 7), active management interventions would be less viable because HABs are more likely to be driven by natural phenomena occurring in areas with mixed or no anthropogenic pressures.
Several examples of HABs in European geographical areas, found in the reviewed literature, have been placed within the MAMBO matrix quadrants (Figure 2) and briefly described in Supplementary Table S2 to illustrate MAMBO functionality. For example, in quadrants 2 and 3, where HABs have a natural origin, there is the occurrence of Gymnodinium catenatum blooms in Portugal and the Galician Rias in Spain. The G. catenatum blooms are triggered at the end of the coastal upwelling seasons when nutrient-depleted, warm surface water is found offshore, while coastal upwelling keeps the nearshore waters cold, nutrient-rich, and with a rich community of diatoms. When the upwelling subsides, warmer offshore waters move toward the coast, resulting in a temperature increase that favors the blooms of G. catenatum. In some cases, an inshore poleward current may transport populations of dinoflagellates to the Rias from waters off northern Portugal (Sordo et al., 2001; Pitcher and Fraga, 2015). These natural triggers place these blooms outside the scope of management, making monitoring and early warning systems the most appropriate tools for mitigating their impacts.
Alexandrium taylori and Gymnodinium litoralis blooms, which are influenced by both natural and anthropogenic factors, are illustrative examples of quadrant 5. These are common in Mediterranean coastal waters, and result from a combination of factors, including nutrient enrichment, enhanced growth, and limited water renewal. Nutrients are mainly supplied by groundwater, rivers and seasonal Mediterranean streams, while local summer winds maintain high cell densities in coastal waters (Garcés et al., 1999; Basterretxea et al., 2005; Reñé et al., 2011; Garcés and Camp, 2012). Both increased growth rates and reduced wind-driven water renewal are critical in modulating these blooms. While nutrient inputs can be controlled, hydrographic mechanisms cannot.
In quadrant 9, the harmful algal species Prorocentrum cordatum has traditionally been associated with eutrophication, mainly from riverine nutrients exported to the coast (Glibert et al., 2008). The authors demonstrate that the species is prevalent in regions with high levels of dissolved inorganic nitrogen and phosphorus significantly originated from anthropogenic sources, such as fertilizers and manures.
It is worth highlighting that a single HAB species or groups may appear in different quadrants. For example, Pseudo-nitzschia is a genus of diatoms that exhibits remarkable adaptability, thriving in a variety of environmental conditions (Hasle, 2002; Hubbard et al., 2023). Even if commonly found in upwelling systems, such as the Galician Rias, it also thrives in eutrophic areas where excess nutrients from human activities create favorable conditions for its growth, such as Alfacs Bay in the Mediterranean. This diatom genus even appears in open waters with an oligotrophic status, demonstrating its ability to bloom in diverse environments. This adaptability illustrates MAMBO's effectiveness in defining manageable conditions for the same microalgae involved, emphasizing that the bloom's origin is often more pertinent than the species involved.
Moreover, the ecosystem positions in MAMBO are dynamic. For example, European assessments of eutrophication indicate that phosphorus levels in rivers are decreasing, thereby reducing fluxes to coastal areas such as the Mediterranean and southern North Sea (Ludwig et al., 2009). Therefore, as WFD measures take effect, the trophic status of areas within the MAMBO matrix may shift away from anthropogenic influences. However, the gaps of knowledge on these changes hinder our ability to predict what will be the trends of the trophic status and generation of HABs in the European seas. For example, in the offshore Mediterranean Sea, oligotrophication is expected to continue due to reduced continental inputs and increased water column stability under global change. However, the extent to which these processes will be influenced by extreme weather events or increased atmospheric deposition is not known.
Anyhow, the outcomes from MAMBO will define the next steps in the GES4HABs decision flow (Figure 1): HABs identified as manageable should be included in a comprehensive assessment informing on their status, their related pressures and impacts (MSFD Article 8.1b) and the human activities involved (Article 8.1c). This assessment will support the designation of appropriate measures to restore GES conditions (Articles 10, 13, 11). For HABs located in quadrants 1 to 3, an exception (Article 14) could be considered for the implementation of new management measures, justifying the likely natural and unmanageable nature of the identified GES deviation. In cases with insufficient evidence to rule out or confirm HAB linkages with anthropogenic pressures, additional investigative monitoring should be supported to clarify these questions. Alternatively, a precautionary approach can be adopted, assuming probable linkage to anthropogenic causes, and initiate a full assessment and management cycle as in the first case. The following sections provide a list and brief descriptions of the indicators and monitoring methods that can be used to achieve this.
3 Currently used indicators and alternatives
For both initial assessment and the subsequent reporting cycles, it is essential to identify suitable indicators under the relevant MSFD descriptors and criteria that evaluate the state, associated pressures and impacts of HABs. Although the MSFD currently addresses HABs solely within the eutrophication descriptor (D5C3 criterion) and their potential impacts on the “D1C6-pelagic broad habitat” state criterion (European Commission, 2017), it formally omits HABs unrelated to anthropic eutrophication (European Commission, 2022). This omission occurs despite their evident connections with other ecological challenges (e.g., mass mortalities or disruption of ecosystem services). Further details on these connections between HABs and the MSFD descriptors are provided in Supplementary Table S3.
In addition to the thematic context, indicators should be defined alongside quantifiable metrics or indices and their associated thresholds to ensure operational, transparent, and efficient assessments.
Criteria for indicator selection within a normative framework should: (i) have limited sensitivity to natural variation (Heink and Kowarik, 2010), (ii) reflect pressure-state-impact linkages with other indicators (Dale and Beyeler, 2001; Niemeijer and de Groot, 2008; Marques et al., 2009; Birk et al., 2012), (iii) consider the feasible/required sampling and analysis capabilities, (iv) account for the temporal, spatial, and taxonomic resolutions of underlying data and their associated uncertainties (Racault et al., 2014), and (v) allow for intercomparison and intercalibration at the pan-European level (Poikane et al., 2014). Threshold values, Ecological Quality Ratios (EQRs), trends, or supplementary information are crucial for properly interpreting the indicator results (Cusack et al., 2008).
3.1 Indicators for pressures causing HABs
The main abiotic pressures identified to cause HABs, as for phytoplankton in general, are nutrients (both macronutrients and micronutrients), light, temperature, water column stability (Anderson et al., 1998; Litchman and Klausmeier, 2008; Facey et al., 2019), pH (Shapiro, 1984; Raven et al., 2020) and oxygen concentration (Heisler et al., 2008; Ryan et al., 2009; Kudela et al., 2010; Pitcher and Fraga, 2015; McCabe et al., 2016; Xiao et al., 2019). The conditions that trigger HABs can result from changes in the seabed and hydrography, as well as species translocations caused by human activities. Thus, criteria and indicators used under MSFD descriptors other than D5 (e.g., D2, non-indigenous species; D4, food-webs; D6, seafloor integrity; and D7, hydrography) could be relevant for assessing the pressures contributing to HABs. However, currently used indicators may require adaptation to appropriately assess HAB causes.
For instance, many HABs are associated with certain inorganic nutrient ratios, forms or composition regardless of the total nutrient availability (Glibert and Burkholder, 2006; Heisler et al., 2008). Some mixotrophic or heterotrophic harmful algal species, seem to be stimulated by the availability of organic forms of nitrogen or phosphorus (Herndon and Cochlan, 2007; Cochlan et al., 2008; Kudela et al., 2008, 2010; Loureiro et al., 2011; Davidson et al., 2014), whereas other HAB forming species consume predominantly particulate rather than dissolved nutrients (e.g., Jeong et al., 2005). Another example is the ability of some harmful algal species to fix and convert gaseous nitrogen, enabling them to succeed and grow in nitrogen-depleted conditions (Litchman, 2023).
The development of HABs is often also related with the dynamics of the whole ecological system and the adaptive strategies of certain species (e.g., against predation) (Flynn, 2008). Therefore, multiparametric indicators or methods have been also proposed based on the multitrait characteristics associated with different HABs (Litchman, 2023), the combined mixing-irradiance-nutrient conditions (Smayda and Reynolds, 2003), or interestingly, indicators focusing on other ecological groups like zooplankton to improve forecasting of biotoxins from harmful algae blooms (Trapp et al., 2021).
Other abiotic indicators on irradiance levels (at the surface or reaching the seabed), or on the dissolved oxygen profiles, could be interesting if linkages between particular conditions and certain HABs are revealed. Finally, to report on hydrographical or seabed alterations potentially promoting HABs, indicators reflecting these changes (anomalies, inflection points) or special conditions (extreme events, stratification or upwelling indices, residence times, etc.) could be eligible.
3.2 Indicators for HAB state
These indicators parallel those used to assess phytoplankton, but are specialized to address potentially harmful phytoplankton taxa. They also take into account the frequency or probability of their associated occurrence (e.g., seasonal, occasional, potential). The commonly used indicators for phytoplankton and phytobenthos provide information on their composition, structure or functions, as detailed in Supplementary Table S4.
Indicators addressing composition commonly rely on metrics such as presence, abundance or biomass of phytoplankton and phytobenthos taxa, often quantified as the cell counts per volume or weight. For certain toxigenic HABs, the identification to species level is required to differentiate between toxic and non-toxic species within the same genus (e.g., Alexandrium and Pseudo-nitzschia). However, the mere presence of a HAB species does not necessarily indicate an outbreak, or a toxic event. Moreover, if the HABs anthropogenic origin is inconclusive, the indicator may not suffice to trigger management actions, according to GES4HABs (Figure 1).
The presence and abundance of phytoplankton toxic species are currently reported under various regulatory frameworks such as Food Regulation (EU) 2019/627, Bathing Water Directive, as well as in OSPAR assessments from 2003, 2008 and 2017 (OSPAR Commission, 2003, 2008, 2017). Some Member States also reported data for abundances of noxious taxa, such as Phaeocystis spp. and Noctiluca spp., under the MSFD, while HELCOM assessments include data on bloom-forming cyanobacteria genera. Most of these abundance indicators have associated thresholds, sometimes established at the national level (e.g., Chorus, 2012; Funari et al., 2015; Serret et al., 2019), which are periodically revised for accuracy and relevance. These thresholds serve as benchmarks for initiating regulatory actions and are adaptable to align with updated scientific knowledge and environmental conditions.
Indicators related to phytoplankton structure include information on the coexistence of different phytoplankton groups. These groups can be structured either taxonomically (i.e., diatoms/dinoflagellates), by size (microplankton, nanoplankton, picoplankton, etc.), based on their pigment signatures (Bustillos-Guzmán et al., 2004; Havskum et al., 2004; Not et al., 2007; Hayward et al., 2023), or according to their functional traits such as autotrophs-to-heterotrophs ratios or ecological assemblages (Nogueira and Figueiras, 2005; Weithoff and Beisner, 2019; Lehtinen et al., 2021; Litchman, 2023). This structuring of indicators offers a multifaceted approach to understanding phytoplankton communities and their ecological roles.
A high variety of multi-metric indices that incorporate phytoplankton community information are utilized in eutrophication assessments, including national WFD reporting (Tett et al., 2008; Devlin et al., 2009; Giordani et al., 2009; Spatharis and Tsirtsis, 2010; Lugoli et al., 2012; Facca et al., 2014; Ní Longphuirt et al., 2019), and HELCOM, OSPAR, or UNEP-MAP assessments. These indices often come with established thresholds and are linked to nutrient levels and other eutrophication pressures.
Finally, indicators focusing on bloom frequency, amplitude, peak, spatial extent, and phenology are rarely used because the high temporal resolution required for phytoplankton data. However, such indicators do exist for variables like chlorophyll a.
Chlorophyll a (chl-a) concentration, due to its ease of sampling and measurement, as well as its correlation with nutrient inputs, is the most used proxy of phytoplankton biomass. While it may be unsuitable for determining the abundance at species level, this indicator can be useful in depicting the extent and frequency of phytoplankton blooms and contextualizing the relation between widespread coastal eutrophication and the increase of HABs (Heisler et al., 2008; Xiao et al., 2019).
3.3 Indicators for HAB impacts
The indicators addressing the impact levels of different HABs should clearly address the corresponding types of impacts they produce (see Supplementary Table S1).
3.3.1 Impacts on ecosystems and marine wildlife
Indicators addressing impacts on ecosystems can only be efficient if the occurrence and extent of HAB events are directly connected with the ecosystem status. On a global scale, there have been numerous wildlife mortality events associated with HABs (Rattner et al., 2022) but only in a few cases, robust evidence of direct causation has been provided (e.g., domoic acid: Fritz et al., 1992; Work et al., 1993; microcystin: Miller et al., 2010; aetokthonotoxin: Breinlinger et al., 2021). So far, there is no historical evidence of lasting population level consequences associated with persistent HABs (Rattner et al., 2022).
The controlled studies of algal toxin effects in wildlife have focused on acute impacts such as mass mortality events involving marine mammals, seabirds and charismatic megafauna, but far more data and studies are needed to assess the hazard of various algal toxins to wildlife. In this context, diagnostic guidance or protocols (toxic doses, target organs, molecular biomarkers, microscopic lesions, signs of intoxication, etc.) for linking algal toxin exposure to morbidity and mortality of different species or groups, would be a valuable resource to define suitable indicators and thresholds. It is noteworthy that such information (e.g., tissue residues, molecular biomarkers, histopathological lesions, behavioral effects, delineation of various intoxication syndromes) is available for domoic acid and other toxins in marine mammals (Lefebvre et al., 2012; Cook et al., 2015; Broadwater et al., 2018). In the interim, toxin content in water or seafood vectors might be employed to predict risk to wildlife. Analyses of toxins on stranded dead animals or ongoing stomach content analyses for litter assessments [OSPAR Ecological Quality Objective (EcoQO)] could be an opportunity for that.
Using indicators addressing the abundances of some vector species serving as “sentinels” or “bio-indicators” like filter-feeding invertebrates, top predators or confined fishes could also help to support early detection of toxic HAB episodes or record the cumulative effects of their occurrence given their often ephemeral and local frequency (Backer and Miller, 2016).
For eutrophication impacts, some phytoplankton species have also been tested as bio-indicators in the Baltic Sea, due to their positive linear relationship with nutrient concentration (Höglander et al., 2013), e.g., Cyclotella choctawhatcheeana and Cylindrotheca closterium (Jaanus et al., 2009), and Planktothrix agardhii (Carstensen and Heiskanen, 2007).
Impacts on biodiversity may also be caused by high biomass and mucilage/foam producing HABs, by reducing water column oxygenation, light penetration and viscosity inducing mass mortalities to benthic communities and species like gorgonians, corals, and sponges. In these cases, the status of the potentially affected species may be assessed to monitor these impacts (Özalp, 2021).
3.3.2 Impacts on human health
Most of the monitoring and management efforts on HAB impacts are related to human health either by direct exposition or toxic seafood consumption. The European Commission has already established specific laws for the toxin content of bivalves of planktic origin entering the market for human consumption and the marine toxin limits allowed before legal sale [Regulation (EC) 853/2004, 2004]. These regulations are applicable by seafood producers and by food security administrations.
The detection of marine biotoxins in other seafood vectors (rarely covered by the monitoring programs) is already being done in European countries such as Portugal, UK, Croatia and Spain (Ben-Gigirey et al., 2012, 2020; Silva et al., 2013, 2018, 2020; Dean et al., 2020), to prevent further seafood intoxications. For example, the possible presence of PSP toxins in cephalopods, echinoderms and tunicates and the increased interest in the exploitation of marine live resources other than bivalves have promoted a revision of monitoring strategies introducing non-traditional vectors [Regulation (EC) 853/2004, 2004]. These regulations also include the maximum PSP toxin concentrations allowed in echinoderms, tunicates and marine gastropods. However, more studies are needed to evaluate the potential risks they could pose for human health as well as their impacts on food webs. On top of that, more data on the presence of emerging marine toxins in the EU marine invertebrates are also necessary for risk assessment studies on these non-traditional vectors (Ruiz-Villarreal et al., 2022).
The impacts of HABs on human health can also be evaluated as societal costs that in precedent studies (Sanseverino et al., 2016) have focused on medical costs (medical cares and medical investigations) and individual expenses (lost wages, lost vacation time, transportation of patients to the hospital, etc.).
3.3.3 Impacts on socio-economic activities
Indeed, while substantial research is directed toward understanding, quantifying, and forecasting HAB occurrences (HAB state indicators), less attention has been given to understanding, quantifying, and preparing for the socio-economic impacts that these events generate each year (Trainer, 2020). There are some examples of comprehensive assessments of the economic losses due to HABs (including direct and indirect costs) (Hoagland and Scatasta, 2006; Sanseverino et al., 2016; Martino et al., 2020; Karlson et al., 2021). While reasonable estimates are often possible for harvest and job or wage losses associated with decreased yields of seafood products (direct losses), as well as the medical costs associated with acute poisonings (induced losses), other, often much larger costs are more difficult to assess. These include impacts on associated industries, which may turn to alternate sources or activities to partially compensate for HAB-related losses in revenue, changes in seafood availability including losses of subsistence harvest potential, losses in recreational and tourism revenues, and losses of consumer confidence in the safety or quality of the product that undercuts demand and thus the price (Trainer, 2020).
Many articles analyzed the consequences of seafood trade bans at different scales (Basti et al., 2018). Dyson and Huppert (2010) used an Input-Output model to estimate the detrimental impact of beach closures on recreational razor clam fisheries. Díaz et al. (2019) studied the economic loss of the salmon farming industry in South Chile caused by HAB events, where the economic damage was deemed particularly strong in PSP outbreaks. Red tides are also largely studied through their economic impacts on different industries, using monitoring data (Larkin and Adams, 2007). More recently, Theodorou et al. (2020) evaluated the consequences of HAB-related mussel farming site closures in the Mediterranean Sea and concluded that the risk depends on the season (summertime being the most critical) when it occurs, with a limited financial risk at certain non-critical periods. Park et al. (2013) studied the economic impact and mitigation strategies of HABs in Korea, where the aquaculture industry suffered a total loss of USD $121 million from the early 1980s to the early 2010s, with a predominance of Cochlodinium polykrikoides events since 1990. In Southern Europe, Rodríguez Rodríguez et al. (2011) looked at mussel cultivation in Galicia in the presence of red tides. They estimated the correlation between the time length of area shutdowns and the quantity of unsold output. They showed that there was no systematic effect: losses depend on specific market circumstances and authors highlighted the importance of organizational solutions to mitigate commercial risks. More recently, Martino et al. (2020) used a production function to investigate the effect of HABs on the Scottish shellfish market. They showed a significant but non-linear relationship between DSP and shellfish production.
Most of the available studies on socioeconomic losses on tourism caused by HABs are found in the US (Sanseverino et al., 2016). However, indicators like the number of beach closures, the expenditures on foam cleaning or barriers deployments in recreational waters, or the decrease of visitors may be useful to assess these impacts. Some of this information is already collected by public national or European statistical agencies (i.e., EUROSTAT) and/or other stakeholders like civil protection servants. On the contrary, information on damages caused by HABs on infrastructures like pumping systems or desalination plants may be more difficult to gather.
Considering the need for further monitoring and research to fill research gaps and assessment requirements related with HABs (Guillotreau et al., 2021) an evaluation of monitoring costs could evidence the benefits of such investments when compared to the accumulated costs of their negative impacts. Often, when considering the total costs of environmental management, from monitoring to management programs, monitoring costs constitute only a small proportion that becomes even smaller when adding the benefits achieved from efficient management (Nygård et al., 2016).
4 Current and novel monitoring programs and methods
The selection of indicators related to HABs will be highly reliant on the monitoring methods employed (including sampling and analysis procedures), and the required/feasible spatio-temporal extents and resolutions. Anderson et al. (2019) examined several regional programs in the USA, European Union, and Asia and concluded that there is no one-size-fits-all approach.
HABs occur in sunlit pelagic oceans, at the surface or at subsurface in open seas, coastal and upwelling regions and estuaries. Traditional phytoplankton sampling employs discrete in situ water samples, collected using Niskin bottles or nets at various depths that are filtered and preserved for laboratory analysis through multiple analytical techniques (Karlson et al., 2010). In their guidelines for monitoring the toxin-producing phytoplankton in bivalve mollusc harvesting areas, Serret et al. (2019) recommend sampling the full range of depths where shellfish are grown.
Non-motile resting cysts of HAB species that settle and accumulate in seabed, are collected using devices like sediment grabs, cores or pumps. Comprehensive guidelines for HABs and cyst sampling can be found in Hallegraeff et al. (2003). Such cysts are often found in finer grain sediments, with low wave and wind exposure like protected harbors and bays. Recent formed cysts are found in the surface sediment layers with progressively older cysts, sometimes, decades older, found with increasing depth.
No standard procedures exist for benthic HAB sampling due to the diversity of substrates that cells grow on (macroalgae, seagrass, sand, pebbles, rocks, coral, and coral rubble). Tracking their growth is challenging due to planktonic/benthic alternation stages and high spatio-temporal variability (Berdalet et al., 2017). Commonly, cells are shaken off the substrates and filtered to collect detached cells (Yasumoto et al., 1980; Hoppenrath et al., 2023). The use of artificial substrate to recruit benthic cells over 24 hours followed by counting as a proxy measurement of benthic HAB species abundance has been recommended (Tester et al., 2014; Jauzein et al., 2016; Mangialajo et al., 2017).
Samples are processed in the laboratory for the identification and abundance quantification of different taxa. Currently, the most prevalent technique employs morphological identification through optical microscopy, conducted by expert microalgae taxonomists. This method is time-consuming, expensive, and limited to identifying larger phytoplankton species (>5μM). Additionally, there is a declining pool of expert taxonomists, further complicating the process (McQuatters-Gollop et al., 2017). In this sense, advanced open international training courses and certificates in phytoplankton taxonomy, such as those organized by the IOC-UNESCO, are essential to promote the availability of qualified taxonomists and interlaboratory intercalibration exercises.
In any case, new approaches for more rapid, cost effective and precise microalgae cell counting and identification are being continuously developed and proposed to support HAB monitoring.
4.1 Analyses for HAB identification and cell abundance estimation
There are extensive reviews for the established detection methods for harmful microalgae found in Anderson et al. (2001), Hallegraeff et al. (2003), and Liu et al. (2022), and detailed methodological guide by Karlson et al. (2010). The described methods include morphological structure-based detection methods (optical microscopy, inverse optical microscopy and scanning electron microscopy, automated image identification and classification), analytical detection techniques (high-performance liquid chromatography, absorption spectral analysis and fluorescence spectral analysis), immunofluorescence assay, immunosensing assay, enzyme-linked immunosorbent assay; and nucleic acid-based detection methods (fluorescence in situ hybridization, sandwich hybridization assay, polymerase chain reaction techniques, metabarcoding, isothermal amplification technology, and microarrays). Liu et al. (2022) also provided information on the principles, advantages, weaknesses and suitability of these methods for the detection and identification of harmful microalgae.
The analytical detection or chemotaxonomic analysis may contribute to study the distribution and composition of different phytoplankton classes with specific pigment signatures (Schlüter et al., 2000; Henriksen, 2002). It is rarely used now for HABs monitoring as pigment signatures do not specifically relate to taxonomic identity. However, Bustillos-Guzmán et al. (2004) found that 76% and 84% of dinoflagellate and diatom cell density was explained by their specific pigment signature variation suggesting that pigment analysis could be very useful in delineating taxa or potential toxin-producing groups, particularly in combination with remote sensing near real-time or predictive models.
FlowCAM (Sieracki et al., 1998) is an automated identification device that includes a flow cytometer with a camera and a microscope which is widely used in several studies for analyzing fixed and fresh phytoplankton samples both in the laboratory or onboard ships. It is effective at detecting some harmful algae by image, often using an imaging training set (Buskey and Hyatt, 2006). For toxigenic Alexandrium catenella, it was shown that mean abundances as defined by FlowCam were comparable to those defined by molecular-probe and microscopy (Ayala et al., 2023). However, it is limited to microscopic-level species distinction. This method is still in development for harmful algae detection, so there is much heterogeneity in methodological reporting (e.g., FlowCam unit, sample preparation, run settings, improved post-processing of images). Harmonized protocols and guidelines are needed to enhance the quality, interpretability, and repeatability of FlowCam results (Owen et al., 2022).
Molecular methods are frequently used for quantifying marine organisms including toxin-producing microalgae via species-specific qPCR/droplet digital PCR (dPCR) methods, or to determine phytoplankton community biodiversity (with metabarcoding or amplicon sequencing methods) (Scorzetti et al., 2009; Pearson et al., 2021). This trend is likely to continue thanks to improved standardization and technological development (Goodwin et al., 2016; Medlin and Orozco, 2017; Jerney et al., 2023), lower sample processing costs, and relatively straightforward sample collection and preservation from water filters (Jerney et al., 2023) or phytoplankton net or sediment samples. Species-specific PCR or dPCR based methods are relatively accurate and sensitive but require specialist knowledge of the relevant species (or toxin genes), and assays (primer pairs) targeting these species (or genes). Extensive public sequence databases exist from Genbank, PR2, BOLD, Midori or Phyto (Murray et al., 2011; Casabianca et al., 2014; Pearson et al., 2021; Yarimizu et al., 2021). Routine monitoring using qPCR or dPCR is already used in the French Atlantic and Mediterranean coasts, the Bay of Biscay, UK, Ireland, the US and New Zealand to provide HAB early warnings (Drouet et al., 2021; Pearson et al., 2021). Metabarcoding methods have also been already widely used to study the dynamics of HAB species and their spatial distribution (Dzhembekova et al., 2022; Gaonkar and Campbell, 2023). Both qPCR/dPCR (Perini et al., 2019) and metabarcoding (Wang et al., 2022) can also be successfully applied to assess the distribution and abundance of toxic dinoflagellate cysts (Perini et al., 2019).
Biosensor technology is applied to all these methods to miniaturize platforms for the detection of multiple targets, for in situ rapid detection to increase detection frequency and reduce manual costs (Medlin et al., 2020; Chin Chwan Chuong et al., 2022; McNamee et al., 2023).
Most of the above methods, in addition to the requirement for high-tech equipment and trained staff, depend on (i) the development/availability of ancillary data (like libraries of genes, taxonomic images, or pigment signatures), (ii) powerful algorithms/models to reliably identify microalgae species based on the features analyzed, (iii) standardized protocols or guidelines [e.g., Karlson et al., 2010; U. S. Integrated Ocean Observing System (IOOS), 2017; Serret et al., 2019] and (iv) intercomparisons of results from different methods (e.g. Not et al., 2007; McNamee et al., 2023).
4.2 Analysis methods for HAB toxin detection and quantification
In Europe, an official Standard Operating Procedure (SOP) exists for the determination of several biotoxins in live bivalve molluscs: the amnesic shellfish poisoning toxin (Commission Regulation, 2005), the okadaic acid, as well as some azaspiracids and yessotoxins (Commission Implementing Regulation (EU) No 2019/627), and the PSP toxins ordered by the Commission Implementing Regulation (EU) 2021/1709. The SOP establishes the reference methods to use by official authorities for seafood at any stage of the food chain and for internal checks by food business operators (FBO). These methods were validated under the coordination of the European Union Reference Laboratory for marine biotoxins (EU-RL) in an inter-laboratory validation study carried out by the Member States. However, the recent findings of the presence of emerging azaspiracids, spirolides, pinnatoxins, gymnodimines, palitoxins, ciguatoxins, brevetoxins, and tetrodotoxins in European coastal seas require additional monitoring, analytical guidelines and regulatory guidance to face new potential risks caused by these substances (Otero and Silva, 2022). There are already ongoing activities to develop and validate workflows for the identification and characterization of emergent marine toxins, and the organisms producing them, in environmental samples based on the next-generation sequencing (NGS), shotgun metagenomic sequencing and computational analysis for OneHealth surveillance and food safety risk assessment (García-Cazorla and Vasconcelos, 2022).
Other non-bivalve marine organisms such as echinoderms, tunicates and marine gastropod species may act as toxin vectors in the marine food web (Ben-Gigirey et al., 2020) and have already been responsible for some past poisoning incidents (Costa et al., 2017). Regulation (EC) 853/2004, 2004 stipulates that testing requirements for live bivalve molluscs should apply equally to live echinoderms, live tunicates and live marine gastropods. However, the accumulation of toxins in marine food web is incomplete, and there is still a need to revise which animals act as toxin vectors, and improve recommended guidelines for toxin determination across a wide range of complex variable matrices, including the required sample size and sampling frequency, the highest toxin levels per group, etc.
Emerging studies are also investigating the analysis of concentrations of marine biotoxins in seawater (Bosch-Orea et al., 2021), in aerosols (Ciminiello et al., 2014) and in sediments (Liu et al., 2019) as a toxin reservoir and potential accumulation paths for benthic organisms. Recent advances have been made for portable toxin sensing and biosensing assays for on-site rapid detection of different chemicals including some marine biotoxins (Sohrabi et al., 2021).
4.3 Automated sampling methods and platforms
Significant progress has been made in the development of automated sampling devices that can increase spatial coverage and frequency of sampling (Boss et al., 2022; Nichols and Hogan, 2022) required for an indicator-based assessment of GES on the number, extent, and duration of HABs.
Automated (discrete or continuous) sampling and sensing technologies can be mounted on scientific survey ships, ships-of-opportunity, moored platforms and buoys, land based, remotely operated aerial and underwater platforms, or in autonomous surface or underwater vehicles (Ruiz-Villarreal et al., 2022).
For instance, the Continuous Plankton Recorder (CPR), although originally designed for zooplankton sampling (~270 μm mesh) in 1931, filters 3 m3 of water that retain high densities of phytoplankton trapped in the mesh (Batten, 2003; Richardson et al., 2006). Although there are very few HAB genera that can be effectively sampled using the CPR, a recent study has demonstrated its value in characterizing changing temporal and spatial patterns of Pseudo-nitzschia species using high- throughput sequences from DNA of CPR samples in the North Pacific (Stern et al., 2018). Six decades of data from the CPR have revealed distinct North Sea phytoplankton community events (Bresnan et al., 2013), showing Atlantic-scale decline in harmful dinoflagellates and stable or increasing harmful diatoms, in line with overall dinoflagellate and diatom trends linked to ocean temperature and wind (Hinder et al., 2012). The limitations of CPR data include its collection only of subsurface samples at 4–10 m with poor taxonomic resolution of some harmful algae taxa. However, samples collected by the CPR Survey since 1958 are stored and carefully curated, providing a bank of samples available for future analysis using new and innovative methodologies.
The MBARI Environmental Sample Processor (ESP) (Moore et al., 2021) collects and analyses seawater samples to identify the presence of organisms and/or biological toxins. The instrument uses an electromechanical fluidic system to autonomously collect and filter water samples. Then it either preserves and archives the sample for use after the ESP is recovered or directly applies molecular detection technology to investigate the biology of the sample in near real-time. ESP was deployed in the Pacific Northwest to provide near real-time surveillance of growth and toxicity of Pseudo-nitzschia (Scholin et al., 2009), as well as Alexandrium catenella and Domoic acid by ELISA (Ryan et al., 2011). The ESP device can now be deployed on long-range AUVs for extended spatial sampling and post-collection eDNA sequencing (Truelove et al., 2022).
The Imaging FlowCytobot (IFCB) is an in situ automated submersible imaging flow cytometer that generates high resolution images of particles in-flow taken from the aquatic environment. An IFCB deployed in Rhode Island has been recently used to generate a daily-resolution time series of Pseudo-nitzschia spp. and Dinophysis spp. (Agarwal et al., 2023). Other flow cytometers and Imaging Flow Cytometers have been deployed in US (Fischer et al., 2020), Scandinavia (Kraft et al., 2021), Hong Kong (Guo et al., 2021), France, and Scotland (Davidson et al., 2021; Ruiz-Villarreal et al., 2022).
The Scripps Plankton Camera system1 is an underwater microscope with real-time image processing and object detection, A classifier has been developed to find potential HABs. Seven potential HAB formers were detected with an image classifier model (Orenstein et al., 2020).
Autonomous Surface Vehicles (ASVs) or Autonomous Underwater Vehicles (AUVs) encompass a wide range of surface and subsurface platforms but suffer from limited payload space for complex instruments required to process samples although there are some new prototypes proposed for sample collection (e.g., Zhang et al., 2019; Truelove et al., 2022). These ASVs typically include sensors to acquire physical and/or chemical data, and/or aggregated biological variables such chl-a, phycocyanin pigments, UAVs can also be equipped with multispectral (Becker et al., 2019) or hyperspectral sensors (Shang et al., 2017), digital cameras (Cheng et al., 2020; Chan et al., 2022) or echosounders (Benoit-Bird et al., 2018). AUVs and ASV have been tested for surficial water sampling in continental and marine waters (e.g., Hanlon et al., 2022; Ruiz-Villarreal et al., 2022). These data can be eligible to support indicators related with some abiotic and biotic pressures on HABs.
However, although very promising for high frequency data, these complex systems have significant constraints related to the acquisition and maintenance costs, staff training, and data management and subsequent data processing notably:
• In situ deployed systems may require two or three equipment units for continuous monitoring including maintenance, calibration or training. Land based systems exist, with seawater being continuously pumped with easier access for maintenance (Ruiz-Villarreal et al., 2022).
• Real-time analysis options require a physical link to land (cables) or a wide bandwidth network or radio connection and access to significant data processing capability.
• The sensors onboard these autonomous platforms need to be light (miniaturized) and low power demanding. While performant probes are already available for some physical parameters (Sun et al., 2021) like temperature, pressure, light, and fluorescence (Roesler et al., 2017), challenges remain to produce accurate, long-range, and sensitive data for salinity (Gu et al., 2022), dissolved oxygen (Wei et al., 2019), or pH (Okazaki et al., 2017). Finally, measurement of nutrients is the most challenging to be measured by in situ sensors (even when not miniaturized), due to their low stability. Sensors for marine nutrients are classified into colorimetric, optical and electrochemical devices. However, most of these devices present several weaknesses as the low accuracy, short duration, narrow detection concentration range and poor repeatability (Liu et al., 2023), Many novel and higher performance sensors are under development to overcome the above-mentioned weaknesses. For instance, Beaton et al. (2022) propose low-cost nutrient analyzers that during the tested in-field profile measurements yielded results comparable to laboratory-based analyses.
Despite all these challenges, technological innovation and product development are advancing very rapidly to make these technologies more reliable and widely accessible to users.
Besides the in situ sensing devices, remote sensing technologies on board aerial and satellite platforms can also provide useful information for indicators or drivers related to HABs. These include Sea Surface Temperature (SST) from infrared sensors, and ocean-color based products such as chl-a and turbidity (Groom et al., 2019; Nichols and Hogan, 2022). These parameters are measured over large areas at higher temporal ranges and frequencies, that can assess temporal dynamics (seasonality, anomalies, extreme events, trends, etc.), and spatial distribution and evolution (i.e., blooms and river plumes extensions). The main limitation of remote sensing is that cloud or ice coverage obscuring image acquisition in some areas and seasons (above all in winter and in northern and equatorial latitudes), and lack of subsurface data. Whilst parameters like SSTs are reliable, the estimation of chl-a and other ocean-color metrics are often uncertain in optically complex waters found mainly near the coast. New algorithms and processing methods are continuously being proposed to overcome these difficulties and provide improved products not only for chl-a but also for the identification of HAB risk areas. For instance, a web alert system to track the development, magnitude and spread of HABs (Karenia mikimotoi, Phaeocystis globosa, Pseudo-nitzschia spp.) in the French-English Channel with satellite data has been developed within the INTERREG-VA FCE project S-3 EUROHAB2 Preliminary results indicate that HAB risk maps of Karenia mikimotoi and Phaeocystis globosa from the NASA satellite MODIS-Aqua are comparable to in situ cell abundances, whereas the Pseudo-nitzschia risk maps are less accurate. Similar studies using satellite data for HAB risk identifications have been proposed for P. globosa and K. mikimotoi in the southern North Sea and western English Channel (Kurekin et al., 2014), for Pseudo-nitzschia in the Galician upwelling area (Torres Palenzuela et al., 2019), for Karenia brevis in the Gulf of Mexico (Stumpf et al., 2003; Cannizzaro et al., 2008; Carvalho et al., 2011), for Cochlodinium polykrikoids in the Persian Gulf (Ghanea et al., 2016), for diatom blooms in the East China Sea (Tao et al., 2015), and for distinct phytoplankton assemblages (Smith and Bernard, 2019, 2020), and cyanobacterial-dominance blooms (Matthews et al., 2012) in the Benguela upwelling area. Remote sensing data is used for calculating the cyanobacterial bloom index pre-core indicator in the HELCOM region of the Baltic Sea for reporting GES status (HELCOM, 2018).
Besides physico-chemical parameters, other remote sensing products can be useful to support indicators on human print like coastline changes (Murray et al., 2019) or location of aquaculture sites (Themistocleous, 2021) from high resolution true-color images, or vessel densities and fishing effort from Automatic Identification Systems (AIS) (Robbins et al., 2022).
4.4 Integrated approaches: models and early warning systems
Many of the above monitoring approaches are often included or complemented in more complete monitoring and research frameworks to integrate information on different biological and environmental features related to HABs. Often this information is integrated in modeling tools than can help to (i) investigate, characterize and quantify the links between different parameters, HABs and their impacts, (ii) discern the contribution of natural vs. anthropogenic causes, (iii) delineate areas with higher risks for HABs based on historical data, (iv) predict, in real- or near real-time, the risk of HABs, and (v) make climatic projections.
There are a multitude of different model types and approaches potentially useful to support HAB research and management, such as ecological and food web models, biogeochemistry models, statistical and machine learning models, physical numerical models, Lagrangian particle tracking models, spatial plan models, etc. (Glibert et al., 2010; Fernandes-Salvador et al., 2021). These models can, at different reliability levels, assess areas with the highest risk likelihood of HAB events over short periods, help to optimize monitoring plans (e.g., with less sampling effort in situations of low probability of HABs), assess/manage the compatibility of different marine uses, aid the preparedness for contingency responses, or extrapolate in situ observations/indicators within the grid to better depict the spatio-temporal variability of the pelagic habitat (Magliozzi et al., 2023).
Although models can be very practical tools, it is important to bear in mind that they cannot substitute monitoring data, especially when public health is compromised. Models are a form of secondary monitoring that use multiple data sources. Their reliability depends on accurate and representative data for their development and calibration, implementation, and validation.
Ralston and Moore (2020) provide a large review of statistical and process-based models that have been developed for different HAB species in different areas of the world. An example of benthic harmful algae model comes from Asnaghi et al. (2017), using Quantile Random Forests model to predict the concentration of Ostreopsis ovata in the Ligurian Sea. Valbi et al. (2019) developed a Random Forest model trained with molecular data to predict the presence of A. minutum in the NW Adriatic Sea. Cheng et al. (2021) developed an iterative Random Forests along the California coast to identify phytoplankton abundance and microbial community structure in response to coastal conditions and land-sea nutrient fluxes.
Early warning systems (EWS) incorporate region-specific knowledge of HAB risk, observations and/or models, which are operationalized (nowcast or forecast modes) to provide communication, by an official source, of authoritative, timely, accurate, and actionable warnings on the likelihood of HAB occurrence and the risk of potential HAB-related impacts of concern. These should consider preparedness protocols at all relevant levels to respond to early warnings with timely actions (FAO, 2023).
Different EWS for HABs exist in Europe (Ireland, Scotland, England, France, Spain and Portugal) ranging from weekly bulletins based on expert analysis and identification systems. EWS can involve particle tracking models and/or remote sensing data (Lin et al., 2021), statistical (e.g., Generalized Additive Models, GAMs) and machine learning models, or mechanistic full-low trophic ecosystem models (Fernandes-Salvador et al., 2021). One such example is ShellEye3 that combine remote sensing, modeled hydrographic data, local algae and biotoxin modeled data to forecast water quality for Scottish shellfisheries that can benefit science-based development of harmful algae indicators.
Very recently, FAO (2023) published a Technical Guidance for the Implementation of Early Warning Systems for HABs that includes examples of several case studies of HABs and EWSs, but mostly provides a complete roadmap for authorities and institutions in countries or regions to commence building an EWS or expand the existing ones.
4.5 International cooperation, monitoring synergies and data management
The information collected in the former sections evidence that there are already several scientific studies and monitoring programs related to HABs that involve different stakeholders (scientists, regulators, managers, industry, and general public) and organizations (European Commission, EFSA, RSCs, ICES, FAO, IOC, etc.). Nevertheless, the resources and initiatives are still quite disconnected among the three main foci of concern for marine HABs: seafood toxins and aquaculture, cyanobacteria and eutrophication, and recreational water quality. Bridging these areas could serve to optimize monitoring efforts and plans, analysis methods and protocols, and information exchange and maintenance (raw data, indicators and metadata).
So far, collective databases with relevant data for HABs can be found in:
• The IOC-ICES-PICES Harmful Algal Event Database (HAEDAT)4 (Bresnan et al., 2021). Developed in the 1990s it contains more than 8,000 entries on harmful algal events associated with monitoring programmes and ad hoc reports from across the globe. It is a part of the IOC International Ocean Data exchange (IODE), and collects, harmonizes, stores and publishes HAB events reported on a voluntary basis by a variety of scientific working groups including the ICES-IOC Working Group on Harmful Algal Bloom Dynamics (WGHABD). The “harmful algal events” considered in HAEDAT must be associated with a negative impact or management action. This information is sensitive to monitoring and reporting effort and efficiency and requires expert interpretation.
• To complement the records of HAEDAT, in 2017, international HAB experts were trained to report on occurrences of toxic algae from scientific publications in the OBIS (Ocean Biodiversity Information System), which now supports HAB OBIS5, a global database with 18,864 harmful algae occurrences reported incorporating databases mentioned in this review. Such data address questions on the probability of change in HAB frequencies, intensities, and geographic ranges. HAEDAT and HAB OBIS data supported the first Global HAB Status Report (Hallegraeff, 2021). While the results and conclusions are likely to be modified as more data become available, these databases encourage reporting and further contribute to these initiatives.
• Databases for RSCs' assessments (HELCOM, OSPAR, UNEP-MAP, BSC) collect and harmonize data provided by different contracting parties. These are made publicly available alongside supporting indicators. In addition to the raw data, some regional sea conventions have made progress in harmonizing indicators and assessment metadata and documentation including guidelines for monitoring, analysis, data processing, quality control, and thresholds. Zampoukas et al. (2014) provide details on phytoplankton monitoring programs (among other elements), related to RSCs (HELCOM, OSPAR, UNEP-MAP) and other marine related EU legislation. All the monitoring guidelines of HELCOM are public6.
• There are currently 120 marine LTERs (Long-term Ecological Research Sites) in European seas measuring key microscopic phytoplankton and in situ chl-a on a regular basis among other environmental parameters. The data collected in these LTERs conform to LTER European data policy, of which one of the guiding principles is to “focus on Open Source products as well as to foster an Open Access policy wherever possible and useful” (Kunkel et al., 2019). As such, LTER data can be of inestimable value also for HAB characterization. Phytoplankton, Zooplankton, ocean hydrography and nutrients for Northern European countries are compiled and available at ICES7, including historic data.
• The Coastal and Oceanic Plankton Ecology, Production and Observation Database (COPEPOD)8, developed by the US National Marine Fisheries Service provides quality-reviewed, globally distributed plankton (phytoplankton, zooplankton and microbial) data with co-sampled environmental hydrographic and meteorological data. Although it includes 193,696 worldwide observations on phytoplankton since the mid-nineties, it was last updated in 2019 and, moreover, much of the historical phytoplankton data is only qualitative (“absent/present/rare/common”). However, it has the great advantage of discovering many phytoplankton historical surveys and monitored sites and providing time series visualizations of phytoplankton and concurrent environmental conditions. Access to raw data often needs to be requested to contributors.
• EMODnet Biology9 provides open and free access to interoperable data and products on marine species (angiosperms, benthos, birds, fish, macroalgae, mammals, reptiles, phytoplankton, zooplankton). It also includes nutrient data. Although EMODNET collects data from different providers (RSCs, research institutes, OBIS, etc.), it also releases maps on the temporal and spatial distribution of species/taxa and species traits in European regional seas.
• Toxin datasets from Food Operators could be very valuable to support assessments and scientific studies beyond their primary objectives for seafood controls especially if combined with phytoplankton counts. In many cases the food operators are reluctant to release these data, especially in real-time, and in some member states toxin data is not available. These hampers investigations linking harmful algae and toxin production. Moreover, toxins are not measured by agencies during the closing periods for cost reasons and because public health is already guaranteed. As a result, information on new appearing toxins, toxin maxima values or detoxification kinetics is not available.
• Phytoplankton and toxin data reported for the BWD in bathing waters with HAB risks are made publicly available through different national portals on recreational water quality.
Besides the effort needed to collect HAB related data, it is important to think on coordinated work for finding common data models and formats, distribution mechanisms and repositories, metadata content (quality controls, lineage, authorship, etc.) and formats, etc. All these best practices should be in line with the Open Science and FAIR (Findable, Accessible, Interoperable, Reusable)10 principles meant to optimize the reuse of data. To meet these demands, the data management efforts should benefit from the participation of professional data management and IT experts, and sustained resources to ensure the good continuity of these collaborative initiatives.
Minelli et al. (2021) concluded from their study on open access to research projects and data that, despite the initial and still existing mistrust, it is more than just a best practice because it improves the transparency of research (thus increasing the credibility of researchers, the reproducibility of science and the re-use of products), supports many international initiatives and regulations, and encourages collaboration between scientists from different fields and laboratories. However, the (re)use of these data in a regulatory context needs to be carefully evaluated for relevance and reliability, ideally by using the same criteria for the different studies producing them (Brock et al., 2021).
5 Conclusions and recommendations to move forward
HABs (like pests or storms) are natural phenomena, but their changing patterns are often a reflection of an ecosystem alteration. Therefore, HABs cannot be eliminated, but only prevented or mitigated. While conventional management focuses on mitigating local impacts, a shift toward EBMAs is essential to prevent to some extent and counteract HAB crises more effectively and cost-efficiently.
The MSFD stands as a milestone in ecosystem-based marine management in Europe, with the aim of achieving GES. Despite its holistic approach and two concluded reporting cycles, HABs have so far received limited attention from Member States (Palialexis et al., 2014; Tornero Alvarez et al., 2023). However, current efforts aim to build on the lessons learned from the WFD and the two reporting cycles of the MSFD, and to promote best practices for integrating HABs into MSFD assessments, recognizing their relevance to marine ecosystems and socio-economic issues.
To improve HAB monitoring, assessment, and management, we have developed new tools and compiled several key recommendations.
5.1 Local tailored solutions with harmonized best practices
There are many different cases of HABs, with different impacts on socio-economic and/or ecosystem components and triggered by different and often combined causes. Therefore, there is no one single solution for the assessment and management of marine HABs in different affected areas, and specific tailored solutions are needed.
Indeed, most of the proposed phytoplankton indicators for eutrophication are site-specific due to the heterogeneous response of marine phytoplankton to nutrient loads and the different monitoring approaches used by Member States. This heterogeneity poses a challenge for comparing results across regions (Garmendia et al., 2013), as demonstrated in the WFD intercalibration exercise (Poikane et al., 2014), where only chl-a was found suitable for biomass-based indicators in coastal and transitional waters by most member states (European Commission, 2018).
However, this does not preclude the need for international and cross-sectoral coordination to (i) share knowledge and data, and (ii) define harmonized or standardized best practices to support joint large-scale assessments and synergistic and optimized strategies. These best practices may relate to monitoring programmes, monitoring methods, analysis protocols, indicator metrics, assessment methods, data management and flows, division of responsibilities, etc.
5.2 Monitoring needs for better understanding HABs, their causes and their impacts
Although the understanding of HABs has increased rapidly over the last two decades, there are still many gaps in our knowledge of their specific causes, toxicity triggers, frequency, extent, duration, impacts on biodiversity, etc.
To meet these knowledge needs, sustained long-term monitoring programmes with appropriate strategies are essential. Monitoring of HABs should have appropriate temporal, spatial and taxonomic resolution, and the spatial distribution of monitored areas should be designed/adapted to avoid: (i) over-representation bias due to the concentration of monitoring sites (e.g., in aquaculture areas), and (ii) spatial gaps in under-monitored areas with potential risk of HABs. The data generated must be long-term (10-year horizon), quality controlled and stored according to international data and metadata standards.
In addition to the detection of HABs, sustained monitoring efforts will enable the establishment of baselines of environmental factors and biodiversity components, the rate and extent of environmental change, the detection of hazards and environmental disturbances, and the estimation of recovery times.
Traditional methods of monitoring HABs, involving in situ sampling and taxonomic identification and cell counting in laboratories, are currently the best available option but remain costly, time-consuming and inadequate for the vast geographical scope of the MSFD. New technologies are being developed to provide more cost-effective solutions, such as genomic methods, automated samplers, remote sensing, early warning systems, artificial intelligence models, etc. The future use of the results of these novel techniques will need to undergo intercalibration processes in order to be reliably used in the assessment and management processes (Stauffer et al., 2019).
5.3 Integration with the MSFD framework
To consider the principles of the MSFD, this study proposes a GES4HABs decision matrix to assist MSFD reporters in deciding whether to include HABs in the MSFD and adopt management decisions. Within this process, the MAMBO matrix helps to distinguish between manageable and unmanageable circumstances around HAB outbreaks. If HABs are found suitable for inclusion in the MSFD assessment cycles, then the full assessment procedure should be engaged. In this context, it is extremely important to identify, demonstrate and quantify the links between HABs, the pressures causing them and their impacts on public health and on the different components of the ecosystem and socioeconomic activities, and to select the most appropriate indicators and thresholds to reflect these links. This remains an important prerequisite for targeting the best management measures to effectively reduce the occurrence of HABs and their impacts.
To date many of these management measures proposed by European environmental instruments (like MSFD, WFD, ND, UWWTD, etc) have focused on nutrient reduction objectives but have largely overlooked measures to counter habitat degradation in coastal areas. This oversight contributes to the simplification of European coastal habitats and ecosystems, allowing harmful algal blooms to persist.
5.4 International and cross-sectoral cooperation to increase synergies and optimize resources and efforts
Cooperation fora and roles need to be better defined to integrate new knowledge on different HABs and scale up from multiple national assessments to regional or global observing system for HABs (Anderson et al., 2019). To this end, the complementarities between the resources and organizations currently dedicated to HAB management (food safety and public health authorities, environmental managers, scientific organizations, food producers, marine spatial planners, etc.) should be closely examined to build bridges of cooperation, avoid duplication, optimize efforts, and focus new resources to fill the identified gaps.
5.5 Preparedness and anticipation for adaptability and sustainability of ecosystems
Although the general perception of a global increase of HABs needs further and more refined substantiation (Hallegraeff et al., 2021), the rapidly changing environmental conditions due to climate change and the expansion of the human footprint in European coastal and marine waters strongly support the need to actively intensify efforts toward ecosystem based management strategies that although complex and challenging can provide solutions to avoid increasing vulnerability to future changes, and reinforce our preparedness and anticipation capacities for adaptability.
Author contributions
YS: Conceptualization, Investigation, Writing—original draft, Writing—review and editing. EG: Conceptualization, Investigation, Writing—original draft, Writing—review and editing. JF: Conceptualization, Investigation, Writing—original draft, Writing—review and editing. RS: Conceptualization, Investigation, Writing—original draft, Writing—review and editing. MR: Conceptualization, Investigation, Writing—original draft, Writing—review and editing. EM: Conceptualization, Investigation, Writing—review and editing. EB: Conceptualization, Investigation, Writing—review and editing. GT: Conceptualization, Investigation, Writing—review and editing. HJ: Conceptualization, Investigation, Writing—review and editing. NS: Conceptualization, Investigation, Writing—review and editing. AR: Conceptualization, Investigation, Writing—original draft, Writing—review and editing. JC: Conceptualization, Investigation, Writing—review and editing. ÁB: Conceptualization, Funding acquisition, Supervision, Writing—review and editing. JGR: Investigation, Writing—original draft. ES: Conceptualization, Writing—review and editing. KP: Conceptualization, Writing—review and editing. RD: Conceptualization, Writing—review and editing. AL: Writing—original draft. LF: Writing—review and editing. PB: Conceptualization, Writing—review and editing. LB: Writing—original draft, Writing—review and editing. EA: Writing—review and editing. JK: Writing—review and editing. AM: Writing—review and editing. SK: Investigation, Supervision, Writing—original draft, Writing—review and editing, Conceptualization.
Funding
The author(s) declare financial support was received for the research, authorship, and/or publication of this article. This manuscript is a result of the joint activity of two projects funded by the European Union, under the Horizon Europe program: GES4SEAS (Achieving Good Environmental Status for maintaining ecosystem services, by assessing integrated impacts of cumulative pressures; grant agreement no. 101059877; www.ges4seas.eu) and ACTNOW (Advancing understanding of cumulative impacts on European marine biodiversity, ecosystem functions and services for human wellbeing, grant agreement No. 101060072). JF was financially supported by the Slovenian Research and Innovation Agency (research core funding no. P1-0237). This work by EG, NS, AR, and JC acknowledges the ‘Severo Ochoa Centre of Excellence' accreditation (CEX2019-000928-S) funded by AEI 10.13039/501100011033 to the Institut de Ciencies del Mar, CSIC.
Acknowledgments
This review contributes to the state of the art objective of the Horizon Europe GES4SEAS project (Achieving Good Environmental Status for maintaining ecosystem services by assessing integrated impacts of cumulative pressures) and has been produced through a collective work including experts from the GES4SEAS and ACTNOW (Advancing understanding of Cumulative Impacts on European marine biodiversity, ecosystem functions and services for human wellbeing) project consortia and additional invited external experts on HABs. We would like to thank Science Crunchers for their artwork on the figures representing the GES4HABs decision tree and the MAMBO matrices.
Conflict of interest
The authors declare that the research was conducted in the absence of any commercial or financial relationships that could be construed as a potential conflict of interest.
The author(s) declared that they were an editorial board member of Frontiers, at the time of submission. This had no impact on the peer review process and the final decision.
Publisher's note
All claims expressed in this article are solely those of the authors and do not necessarily represent those of their affiliated organizations, or those of the publisher, the editors and the reviewers. Any product that may be evaluated in this article, or claim that may be made by its manufacturer, is not guaranteed or endorsed by the publisher.
Supplementary material
The Supplementary Material for this article can be found online at: https://www.frontiersin.org/articles/10.3389/.2023.1298800/full#supplementary-material
Footnotes
2. ^https://www.s3eurohab.eu/portal/
5. ^https://obis.org/node/33dec23c-af65-4fb1-a437-79543c562ef0
6. ^https://helcom.fi/action-areas/monitoring-and-assessment/monitoring-guidelines/
7. ^https://www.ices.dk/data/dataset-collections/Pages/Plankton.aspx
8. ^https://www.st.nmfs.noaa.gov/copepod/about/databases.html
References
Agarwal, V., Chávez-Casillas, J., and Mouw, C. B. (2023). Sub-monthly prediction of harmful algal blooms based on automated cell imaging. Harmful Algae 122, 102386. doi: 10.1016/j.hal.2023.102386
Anderson, C. R., Berdalet, E., Kudela, R. M., Cusack, C. K., Silke, J., O'Rourke, E., et al. (2019). Scaling up from regional case studies to a global harmful algal bloom observing system. Front. Mar. Sci. 6, 250. doi: 10.3389/fmars.2019.00250
Anderson, D. (2014). “HABs in a changing world: a perspective on harmful algal blooms, their impacts and research and management in a dynamic era of climatic and environmental change,” in eds. H. G. Kim, B. Reguera, G. M. Hallegraeff, C. K. Lee, M. S. Han, and J. K. Choi. Harmful Algae 2012, Proceedings of the 15th International Conference on Harmful Algae, Changwon, Gyeongnam, Korea, 29 October−2 November 2012. Copenhagen: International Society for the Study of Harmful Algae, p. 3–17. Available at: https://issha.org/wp-content/uploads/2018/05/ICHA15-Proceedings-1.pdf
Anderson, D. M., Andersen, P., Bricelj, V. M., Cullen, J. J., and Rensel, J. E. (2001). Monitoring and Management Strategies for Harmful Algal Blooms in Coastal Waters. Paris, France: APEC Report # 201-MR-01.1, Asia Pacific Economic Programme, and Intergovernmental Oceanographic Commission of UNESCO, Technical Series No. 59. Available online at: https://unesdoc.unesco.org/ark:/48223/pf0000140545
Anderson, D. M., Boerlage, S. F. E., and Dixon, M. B. (2017). Harmful Algal Blooms (HABs) and Desalination: A Guide to Impacts, Monitoring, and Management. Paris, Intergovernmental Oceanographic Commission of UNESCO, IOC Manuals and Guides No. 78, p. 539.
Anderson, D. M., Cembella, A., and Hallegraeff, G. M. (1998). Physiological Ecology of Harmful Algal Blooms. Available online at: https://epic.awi.de/id/eprint/10883/ (accessed March 8, 2023).
Anderson, D. M., Cembella, A. D., and Hallegraeff, G. M. (2012). Progress in understanding harmful algal blooms: paradigm shifts and new technologies for research, monitoring, and management. Ann. Rev. Mar. Sci. 4, 143–176. doi: 10.1146/annurev-marine-120308-081121
Asnaghi, V., Pecorino, D., Ottaviani, E., Pedroncini, A., Bertolotto, R. M., and Chiantore, M. (2017). A novel application of an adaptable modeling approach to the management of toxic microalgal bloom events in coastal areas. Harmful Algae 63, 184–192. doi: 10.1016/j.hal.2017.02.003
Ayala, Z. R., Judge, S., Anglès, S., and Greenfield, D. I. (2023). A comparison between the FlowCam 8100, microscopy, and sandwich hybridization assay for quantifying abundances of the saxitoxin-producing dinoflagellate, Alexandrium catenella. Harmful Algae 125, 102423. doi: 10.1016/j.hal.2023.102423
Backer, L., and Miller, M. (2016). Sentinel Animals in a One Health Approach to Harmful Cyanobacterial and Algal Blooms. Vet. Sci. 3, 8. doi: 10.3390/vetsci3020008
Basterretxea, G., Garcés, E., Jordi, A., Mas,ó, M., and Tintoré, J. (2005). Breeze conditions as a favoring mechanism of Alexandrium taylori blooms at a Mediterranean beach. Estuar. Coast. Shelf Sci. 62, 1–12. doi: 10.1016/j.ecss.2004.07.008
Basti, L., Hégaret, H., and Shumway, S. E. (2018). “Harmful algal blooms and shellfish”. in Shumway, S. E., Burkholder, J. M., and Morton, S. L. (Eds.). Harmful Algal Blooms: A Compendium Desk Reference. John Wiley and Sons, Ltd., p. 135–190. doi: 10.1002/9781118994672.ch4
Batten, S. D. (2003). Phytoplankton biomass from continuous plankton recorder data: an assessment of the phytoplankton colour index. J. Plankton Res. 25, 697–702. doi: 10.1093/plankt/25.7.697
Beaton, A. D., Schaap, A. M., Pascal, R., Hanz, R., Martincic, U., Cardwell, C. L., et al. (2022). Lab-on-chip for in situ analysis of nutrients in the deep sea. ACS Sens. 7, 89–98. doi: 10.1021/acssensors.1c01685
Becker, R. H., Sayers, M., Dehm, D., Shuchman, R., Quintero, K., Bosse, K., et al. (2019). Unmanned aerial system based spectroradiometer for monitoring harmful algal blooms: a new paradigm in water quality monitoring. J. Great Lakes Res. 45, 444–453. doi: 10.1016/j.jglr.2019.03.006
Ben-Gigirey, B., Rodríguez-Velasco, M. L., and Gago-Martínez, A. (2012). Extension of the validation of AOAC official methodSM 2005.06 for dc-GTX2,3: interlaboratory study. J. AOAC Int. 95, 111–121. doi: 10.5740/jaoacint.10-446
Ben-Gigirey, B., Rossignoli, A. E., Riobó, P., and Rodríguez, F. (2020). First report of paralytic shellfish toxins in marine invertebrates and fish in Spain. Toxins 12, 723. doi: 10.3390/toxins12110723
Benoit-Bird, K. J., Patrick Welch, T., Waluk, C. M., Barth, J. A., Wangen, I., McGill, P., et al. (2018). Equipping an underwater glider with a new echosounder to explore ocean ecosystems: Echosounder equipped glider. Limnol. Oceanogr. Meth. 16, 734–749. doi: 10.1002/lom3.10278
Berdalet, E., Tester, P., Chinain, M., Fraga, S., Lemée, R., Litaker, W., et al. (2017). Harmful algal blooms in benthic systems: recent progress and future research. Oceanography 30, 36–45. doi: 10.5670/oceanog.2017.108
Birk, S., Bonne, W., Borja, A., Brucet, S., Courrat, A., Poikane, S., et al. (2012). Three hundred ways to assess Europe's surface waters: an almost complete overview of biological methods to implement the Water Framework Directive. Ecol. Indic. 18, 31–41. doi: 10.1016/j.ecolind.2011.10.009
Bosch-Orea, C., Sanchís, J., and Farré, M. (2021). Analysis of highly polar marine biotoxins in seawater by hydrophilic interaction liquid chromatography coupled to high resolution mass spectrometry. MethodsX 8, 101370. doi: 10.1016/j.mex.2021.101370
Boss, E., Waite, A. M., Karstensen, J., Trull, T., Muller-Karger, F., Sosik, H. M., et al. (2022). Recommendations for plankton measurements on oceanSITES moorings with relevance to other observing sites. Front. Mar. Sci. 9, 929436. doi: 10.3389/fmars.2022.929436
Breinlinger, S., Phillips, T. J., Haram, B. N., Mare,š, J., Martínez Yerena, J. A., Hrouzek, P., et al. (2021). Hunting the eagle killer: A cyanobacterial neurotoxin causes vacuolar myelinopathy. Science 371, eaax9050. doi: 10.1126/science.aax9050
Bresnan, E., Arévalo, F., Belin, C., Branco, M. A. C., Cembella, A. D., Clarke, D., et al. (2021). Diversity and regional distribution of harmful algal events along the Atlantic margin of Europe. Harmful Algae 102, 101976. doi: 10.1016/j.hal.2021.101976
Bresnan, E., Davidson, K., Edwards, M., Fernand, L., Gowen, R., Hall, A., et al. (2013). Impacts of climate change on harmful algal blooms. MCCIP Sci. Rev. 2013, 236–243.
Bricker, S. B., Longstaff, B., Dennison, W., Jones, A., Boicourt, K., Wicks, C., et al. (2008). Effects of nutrient enrichment in the nation's estuaries: a decade of change. Harmful Algae, 8(1), 21–32. doi: 10.1016/j.hal.2008.08.028
Broadwater, M. H., Van Dolah, F. M., and Fire, S. E. (2018). “Vulnerabilities of Marine Mammals to Harmful Algal Blooms” in Harmful Algal Blooms: A Compendium Desk Reference, eds. S. E. Shumway, J. M., Burkholder, and S. L. Morton. New York, NY: John Wiley and Sons, Ltd, 191–222.
Brock, T. C. M., Elliott, K. C., Gladbach, A., Moermond, C., Romeis, J., Seiler, T., et al. (2021). Open Science in regulatory environmental risk assessment. Integ. Environm. Assessm. Manage. 17, 1229–1242. doi: 10.1002/ieam.4433
Brown, A. R., Lilley, M., Shutler, J., Lowe, C., Artioli, Y., Torres, R., et al. (2019). Assessing risks and mitigating impacts of harmful algal blooms on mariculture and marine fisheries. Rev. Aquac. 12, 1663–1688. doi: 10.1111/raq.12403
Buskey, E. J., and Hyatt, C. J. (2006). Use of the FlowCAM for semi-automated recognition and enumeration of red tide cells (Karenia brevis) in natural plankton samples. Harmful Algae 5, 685–692. doi: 10.1016/j.hal.2006.02.003
Bustillos-Guzmán, J., Gárate-Lizárraga, I., López-Cortés, D., and Hernández-Sandoval, F. (2004). The use of pigment “fingerprints” in the study of harmful algal blooms. Rev. Biol. Trop. 52, 17–26.
Cannizzaro, J. P., Carder, K. L., Chen, F. R., Heil, C. A., and Vargo, G. A. (2008). A novel technique for detection of the toxic dinoflagellate, Karenia brevis, in the Gulf of Mexico from remotely sensed ocean color data. Cont. Shelf Res. 28, 137–158. doi: 10.1016/j.csr.2004.04.007
Carrada, G. C., Casotti, R., Modigh, M., and Saggiomo, V. (1991). Presence of Gymnodinium catenatum (Dinophyceae) in a coastal Mediterranean lagoon. J. Plankton Res. 13, 229–238. doi: 10.1093/plankt/13.1.229
Carstensen, J., and Heiskanen, A. (2007). Phytoplankton responses to nutrient status: application of a screening method to the northern Baltic Sea. Mar. Ecol. Prog. Ser. 336, 29–42. doi: 10.3354/meps336029
Carvalho, G. A., Minnett, P. J., Banzon, V. F., Baringer, W., and Heil, C. A. (2011). Long-term evaluation of three satellite ocean color algorithms for identifying harmful algal blooms (Karenia brevis) along the west coast of Florida: a matchup assessment. Remote Sens. Environ. 115, 1–18. doi: 10.1016/j.rse.2010.07.007
Casabianca, S., Perini, F., Casabianca, A., Battocchi, C., Giussani, V., Chiantore, M., et al. (2014). Monitoring toxic Ostreopsis cf. ovata in recreational waters using a qPCR based assay. Mar. Pollut. Bull. 88, 102–109. doi: 10.1016/j.marpolbul.2014.09.018
Chan, S. N., Fan, Y. W., and Yao, X. H. (2022). Mapping of coastal surface chlorophyll-a concentration by multispectral reflectance measurement from unmanned aerial vehicles. J. Hydro-environ. Res. 44, 88–101. doi: 10.1016/j.jher.2022.08.003
Cheng, K. H., Chan, S. N., and Lee, J. H. W. (2020). Remote sensing of coastal algal blooms using unmanned aerial vehicles (UAVs). Mar. Pollut. Bull. 152, 110889. doi: 10.1016/j.marpolbul.2020.110889
Cheng, Y., Bhoot, V. N., Kumbier, K., Sison-Mangus, M. P., Brown, J. B., Kudela, R., et al. (2021). A novel random forest approach to revealing interactions and controls on chlorophyll concentration and bacterial communities during coastal phytoplankton blooms. Sci. Rep. 11, 19944. doi: 10.1038/s41598-021-98110-9
Chin Chwan Chuong, J. J., Rahman, M., Ibrahim, N., Heng, L. Y., Tan, L. L., and Ahmad, A. (2022). Harmful microalgae detection: biosensors versus some conventional methods. Sensors 22, 3144. doi: 10.3390/s22093144
Choi, J.-G., Lippmann, T. C., and Harvey, E. L. (2023). Analytical population dynamics underlying harmful algal blooms triggered by prey avoidance. Ecol. Modell. 481, 110366. doi: 10.1016/j.ecolmodel.2023.110366
Chorus, I. (2012). Current approaches to Cyanotoxin risk assessment, risk management and regulations in different countries. Federal Environment Agency (Umweltbundesamt), Germany. Available online at: http://www.uba.de/uba-info-medien-e/4390.html (accessed May 15, 2023).
Ciminiello, P., Dell'Aversano, C., Iacovo, E. D., Fattorusso, E., Forino, M., Tartaglione, L., et al. (2014). First finding of Ostreopsis cf. ovata toxins in marine aerosols. Environ. Sci. Technol. 48, 3532–3540. doi: 10.1021/es405617d
Claussen, U., Connor, D., de Vree, L., Leppänen, J., Percelay, J., Kapari, M., et al (2011). Common Understanding of (Initial) Assessment, Determination of Good Environmental Status (GES) and Establishment of Environmental Targets (Articles 8, 9 and 10 MSFD). Available online at: https://circabc.europa.eu/sd/d/ce7e2776-6ac6-4a41-846f-a04832c32da7/05_info_Common_understanding_final.pdf
Cochlan, W. P., Herndon, J., and Kudela, R. M. (2008). Inorganic and organic nitrogen uptake by the toxigenic diatom Pseudo-nitzschia australis (Bacillariophyceae). Harmful Algae 8, 111–118. doi: 10.1016/j.hal.2008.08.008
Collos, Y., Bec, B., Jauzein, C., Abadie, E., Laugier, T., Lautier, J., et al. (2009). Oligotrophication and emergence of picocyanobacteria and a toxic dinoflagellate in Thau lagoon, southern France. J. Sea Res. 61, 68–75. doi: 10.1016/j.seares.2008.05.008
Cook, P. F., Reichmuth, C., Rouse, A. A., Libby, L. A., Dennison, S. E., Carmichael, O. T., et al. (2015). Algal toxin impairs sea lion memory and hippocampal connectivity, with implications for strandings. Science 350, 1545–1547. doi: 10.1126/science.aac5675
Costa, P. R., Costa, S. T., Braga, A. C., Rodrigues, S. M., and Vale, P. (2017). Relevance and challenges in monitoring marine biotoxins in non-bivalve vectors. Food Cont. 76, 24–33. doi: 10.1016/j.foodcont.2016.12.038
Cusack, C., O'Beirn, F. X., King, J. J., Silke, J., Keirse, G., Whyte, B. I., et al (2008). Water “Framework Directive: Marine Ecological Tools for Reference, Intercalibration and Classification (METRIC). Final report for the ERTDI-funded project: 2005-W-MS-36,” in STRIVE Report Series No. 7, Environmental Protection Agency. Available online at: https://oar.marine.ie/handle/10793/1266 (accessed April 3, 2023).
Dale, V. H., and Beyeler, S. C. (2001). Challenges in the development and use of ecological indicators. Ecol. Indic. 1, 3–10. doi: 10.1016/S1470-160X(01)00003-6
Davidson, K., Gowen, R. J., Harrison, P. J., Fleming, L. E., Hoagland, P., and Moschonas, G. (2014). Anthropogenic nutrients and harmful algae in coastal waters. J. Environ. Manage. 146, 206–216. doi: 10.1016/j.jenvman.2014.07.002
Davidson, K., Whyte, C., Aleynik, D., Dale, A., Gontarek, S., Kurekin, A. A., et al. (2021). HABreports: online early warning of harmful algal and biotoxin risk for the scottish shellfish and finfish aquaculture industries. Front. Mar. Sci. 8, 631732. doi: 10.3389/fmars.2021.631732
Dean, K. J., Hatfield, R. G., Lee, V., Alexander, R. P., Lewis, A. M., Maskrey, B. H., et al. (2020). Multiple new paralytic shellfish toxin vectors in offshore north sea benthos, a deep secret exposed. Mar. Drugs 18, 400. doi: 10.3390/md18080400
Delacámara, G., O'Higgins, T. G., Lago, M., and Langhans, S. (2020). “Ecosystem-based management: moving from concept to practice”, in Ecosystem-Based Management, Ecosystem Services and Aquatic Biodiversity: Theory, Tools and Applications, eds. T. G. O'Higgins, M. Lago, and T. DeWitt. Cham: Springer, 39–60. doi: 10.1007/978-3-030-45843-0_3
Devlin, M., Barry, J., Painting, S., and Best, M. (2009). Extending the phytoplankton tool kit for the UK Water Framework Directive: indicators of phytoplankton community structure. Hydrobiologia 633, 151–168. doi: 10.1007/s10750-009-9879-5
Díaz, P. A., Álvarez, G., Varela, D., Pérez-Santos, I., Díaz, M., Molinet, C., et al. (2019). Impacts of harmful algal blooms on the aquaculture industry: Chile as a case study. PiP 6, 39–50. doi: 10.1127/pip/2019/0081
Drouet, K., Jauzein, C., Herviot-Heath, D., Hariri, S., Laza-Martinez, A., Lecadet, C., et al. (2021). Current distribution and potential expansion of the harmful benthic dinoflagellate Ostreopsis cf. siamensis towards the warming waters of the Bay of Biscay, North-East Atlantic. Environ. Microbiol. 23, 4956–4979. doi: 10.1111/1462-2920.15406
Dyson, K., and Huppert, D. D. (2010). Regional economic impacts of razor clam beach closures due to harmful algal blooms (HABs) on the Pacific coast of Washington. Harmful Algae 9, 264–271. doi: 10.1016/j.hal.2009.11.003
Dzhembekova, N., Moncheva, S., Slabakova, N., Zlateva, I., Nagai, S., Wietkamp, S., et al. (2022). New knowledge on distribution and abundance of toxic microalgal species and related toxins in the Northwestern Black Sea. Toxins 14, 685. doi: 10.3390/toxins14100685
Elliott, M., Borja, Á., McQuatters-Gollop, A., Mazik, K., Birchenough, S., Andersen, J. H., et al. (2015). Force majeure: will climate change affect our ability to attain Good Environmental Status for marine biodiversity? Mar. Pollut. Bull. 95, 7–27. doi: 10.1016/j.marpolbul.2015.03.015
European Commission (2000). Directive 2000/60/EC of the European Parliament and of the Council of 23 October 2000 Establishing a Framework for Community Action in the Field of Water Policy, p. 1–73
European Commission (2006). Directive 2006/7/EC of the European Parliament and of the Council of 15 February 2006 Concerning the Management of Bathing Water Quality and Repealing Directive 76/160/EEC, p. 37–51
European Commission (2008). “Directive 2008/56/EC of the European Parliament and the Council of 17 June 2008 establishing a framework for community action in the field of marine environmental policy,” in Official Journal of the European Union L164, 19-40. Available online at: http://data.europa.eu/eli/dir/2008/56/oj (accessed March 8, 2023).
European Commission (2017). Commission Decision (EU) 2017/848 of 17May 2017 Laying Down Criteria and Methodological Standards on Good Environmental Status of Marine Waters and Specifications and Standardised Methods for Monitoring and Assessment, and Repealing Decision 2010/477/EU. Available online at: https://eur-lex.europa.eu/legal-content/EN/TXT/?uri=CELEX%3A32017D0848
European Commission (2018). Commission Decision (EU) 2018/229 of 12 February 2018 Establishing, Pursuant to Directive 2000/60/EC of the European Parliament and of the Council, the Values of the Member State Monitoring System Classifications as a Result of the Intercalibration Exercise and Repealing Commission Decision 2013/480/EU. Available online at: https://eur-lex.europa.eu/legal-content/EN/TXT/?uri=CELEX%3A32018D0229
European Commission (2020). Report from the Commission to the European Parliament and the Council on the implementation of the Marine Strategy Framework Directive (Directive 2008/56/EC). Brussels: European Commission. Available online at: https://eur-lex.europa.eu/legal-content/EN/TXT/PDF/?uri=CELEX:52020DC0259 (accessed April 3, 2023).
European Commission (2022). Article 8 MSFD Assessment Guidance. Available at: https://circabc.europa.eu/d/d/workspace/SpacesStore/d2292fb4-ec39-4123-9a02-2e39a9be37e7/GD19%20-%20MSFDguidance_2022_Art.8Assessment(1).pdf (accessed April 3, 2023).
European Food Safety Authority (EFSA) (2009). Marine biotoxins in shellfish – Summary on regulated marine biotoxins. EFSA J. 1306, 1–23. doi: 10.2903/j.efsa.2009.1306
Facca, C., Bernardi Aubry, F., Socal, G., Ponis, E., Acri, F., Bianchi, F., et al. (2014). Description of a Multimetric Phytoplankton Index (MPI) for the assessment of transitional waters. Mar. Pollut. Bull. 79, 145–154. doi: 10.1016/j.marpolbul.2013.12.025
Facey, J. A., Apte, S. C., and Mitrovic, S. M. (2019). A review of the effect of trace metals on freshwater cyanobacterial growth and toxin production. Toxins 11, 643. doi: 10.3390/toxins11110643
FAO IOC and I. A. E. A.. (2023). Joint technical guidance for the implementation of early warning systems for harmful algal blooms. FAO Fisheries and Aquaculture Technical Paper, No. 690.
Feki, N., Khannous, L., Keskes, F. A., Ben Slama, A., and Levacher, D. (2022). Mobility of trace metals and microbiological pollution from dredged sediments to the Gulf of Gabes, Tunisia. Environ. Monit. Assess. 194, 815. doi: 10.1007/s10661-022-10451-8
Fernandes-Salvador, J. A., Davidson, K., Sourisseau, M., Revilla, M., Schmidt, W., Clarke, D., et al. (2021). Current status of forecasting toxic harmful algae for the north-east atlantic shellfish aquaculture industry. Front. Mar. Sci. 8, 666583. doi: 10.3389/fmars.2021.666583
Ferreira, J. G., Andersen, J. H., Borja, A., Bricker, S. B., Camp, J., Cardoso Da Silva, M., et al. (2011). Overview of eutrophication indicators to assess environmental status within the European Marine Strategy Framework Directive. Estuar. Coast. and Shelf Sci. 93, 117–131. doi: 10.1016/j.ecss.2011.03.014
Fischer, A. D., Hayashi, K., McGaraghan, A., and Kudela, R. M. (2020). Return of the “age of dinoflagellates” in Monterey Bay: Drivers of dinoflagellate dominance examined using automated imaging flow cytometry and long-term time series analysis. Limnol. Oceanogr. 65, 2125–2141. doi: 10.1002/lno.11443
Flynn, K. J. (2008). Attack is not the best form of defense: lessons from harmful algal bloom dynamics. Harmful Algae 8, 129–139. doi: 10.1016/j.hal.2008.08.007
Fritz, L., Quilliam, M. A., Wright, J. L. C., Beale, A. M., and Work, T. M. (1992). An outbreak of domoic acid poisoning attributed to the pennate diatom pseudonitzschia australis. J. Phycol. 28, 439–442. doi: 10.1111/j.0022-3646.1992.00439.x
Funari, E., Manganelli, M., and Testai, E. (2015). Ostreospis cf. ovata blooms in coastal water: Italian guidelines to assess and manage the risk associated to bathing waters and recreational activities. Harmful Algae 50, 45–56. doi: 10.1016/j.hal.2015.10.008
Gaonkar, C. C., and Campbell, L. (2023). Metabarcoding reveals high genetic diversity of harmful algae in the coastal waters of Texas, Gulf of Mexico. Harmful Algae 121, 102368. doi: 10.1016/j.hal.2022.102368
Garcés, E., and Camp, J. (2012). “Habitat changes in the Mediterranean Sea and the consequences for harmful algal blooms formation”, in eds. N. Stambler, N. Life in the Mediterranean Sea: A Look at Habitat Changes. Hauppauge, NY: Nova Science Publishers, 519–541.
Garcés, E., Delgado, M., Mas,ó, M., and Camp, J. (1999). In situ growth rate and distribution of the ichthyotoxic dinoflagellate Gyrodinium corsicum Paulmier in an estuarine embayment (Alfacs Bay, NW Mediterranean Sea). J. Plankton Res. 21, 1977–1991. doi: 10.1093/plankt/21.10.1977
García-Cazorla, Y., and Vasconcelos, V. (2022). Emergent marine toxins risk assessment using molecular and chemical approaches. EFSA J. 20, e200422. doi: 10.2903/j.efsa.2022.e200422
Garmendia, M., Borja, Á., Franco, J., and Revilla, M. (2013). Phytoplankton composition indicators for the assessment of eutrophication in marine waters: present state and challenges within the European directives. Mar. Pollut. Bull. 66, 7–16. doi: 10.1016/j.marpolbul.2012.10.005
Ghanea, M., Moradi, M., and Kabiri, K. (2016). A novel method for characterizing harmful algal blooms in the Persian Gulf using MODIS measurements. Adv. Space Res. 58, 1348–1361. doi: 10.1016/j.asr.2016.06.005
Giordani, G., Zaldívar, J. M., and Viaroli, P. (2009). Simple tools for assessing water quality and trophic status in transitional water ecosystems. Ecol. Indic. 9, 982–991. doi: 10.1016/j.ecolind.2008.11.007
Glibert, P., Seitzinger, S., Heil, C., Burkholder, J., Parrow, M., Codispoti, L., et al. (2005). The Role of eutrophication in the global proliferation of harmful algal blooms. Oceanography 18, 198–209. doi: 10.5670/oceanog.2005.54
Glibert, P. M. (2020). Harmful algae at the complex nexus of eutrophication and climate change. Harmful Algae 91, 101583. doi: 10.1016/j.hal.2019.03.001
Glibert, P. M., Allen, J. I., Bouwman, A. F., Brown, C. W., Flynn, K. J., Lewitus, A. J., et al. (2010). Modeling of HABs and eutrophication: Status, advances, challenges. J. Mar. Syst. 83, 262–275. doi: 10.1016/j.jmarsys.2010.05.004
Glibert, P. M., and Burkholder, J. M. (2006). “The complex relationships between increases in fertilization of the earth, coastal eutrophication and proliferation of harmful algal blooms”, in Ecology of Harmful Algae Ecological Studies, eds. E. Granéli, and J. T. Turner. Berlin, Heidelberg: Springer, p. 341–354. doi: 10.1007/978-3-540-32210-8_26
Glibert, P. M., and Burkholder, J. M. (2018). “Causes of Harmful Algal Blooms”, in Harmful Algal Blooms: A Compendium Desk Reference, eds. S. E. Shumway, J. M. Burkholder, and S. L. Morton, S. L. New York: John Wiley and Sons, Ltd, 1–38. doi: 10.1002/9781118994672.ch1
Glibert, P. M., Mayorga, E., and Seitzinger, S. (2008). Prorocentrum minimum tracks anthropogenic nitrogen and phosphorus inputs on a global basis: Application of spatially explicit nutrient export models. Harmful Algae 8, 33–38. doi: 10.1016/j.hal.2008.08.023
Goodwin, S., McPherson, J. D., and McCombie, W. R. (2016). Coming of age: ten years of next-generation sequencing technologies. Nat. Rev. Genet. 17, 333–351. doi: 10.1038/nrg.2016.49
Gowen, R. J., Tett, P., Bresnan, E., Davidson, K., McKinney, A., Milligan, P. J. H., et al. (2012). “Anthropogenic nutrient enrichment and blooms of harmful phytoplankton”, in Oceanography and Marine Biology: An Annual Review, eds. R. N., Gibson, R. J. A. Atkinson, J. D. M. Gordon, R. N. Hughes. Boca Raton: CRC Press, Taylor and Francis Group.
Groom, S., Sathyendranath, S., Ban, Y., Bernard, S., Brewin, R., Brotas, V., et al. (2019). Satellite Ocean Colour: Current Status and Future Perspective. Front. Mar. Sci. 6, 485. doi: 10.3389/fmars.2019.00485
Gu, L., He, X., Zhang, M., and Lu, H. (2022). Advances in the Technologies for Marine Salinity Measurement. J. Mar. Sci. Eng. 10, 2024. doi: 10.3390/jmse10122024
Guillotreau, P., Bihan, V. L., Morineau, B., and Pardo, S. (2021). The vulnerability of shellfish farmers to HAB events: an optimal matching analysis of closure decrees. Harmful Algae 101, 101968. doi: 10.1016/j.hal.2020.101968
Guo, J., Ma, Y., and Lee, J. H. W. (2021). Real-time automated identification of algal bloom species for fisheries management in subtropical coastal waters. J. Hydro-environ. Res. 36, 1–32. doi: 10.1016/j.jher.2021.03.002
Hallegraeff, G. M. (2021). Global HAB Status Report. a Scientific Summary for Policy Makers. Paris: UNESCO (IOC Information Document, 1399), 14. Available online at: https://unesdoc.unesco.org/ark:/48223/pf0000378691 (accessed March 8, 2023).
Hallegraeff, G. M., Anderson, D. M., Belin, C., Bottein, M.-Y. D., Bresnan, E., Chinain, M., et al. (2021). Perceived global increase in algal blooms is attributable to intensified monitoring and emerging bloom impacts. Commun. Earth Environ. 2, 1–10. doi: 10.1038/s43247-021-00178-8
Hallegraeff, G. M., Anderson, D. M., Cembella, A. D., and Enevoldsen, H. O. (2003). “Manual on Harmful Marine Microalgae,” in Monographs on Oceanographic Methodology, 11. Paris, UNESCO, p. 793.
Hanlon, R., Jacquemin, S. J., Birbeck, J. A., Westrick, J. A., Harb, C., Gruszewski, H., et al. (2022). Drone-based water sampling and characterization of three freshwater harmful algal blooms in the United States. Front. Remote Sens. 3, 949052. doi: 10.3389/frsen.2022.949052
Harrison, P. J., Xu, J., Yin, K., Liu, H. B., Lee, J. H. W., Anderson, D. M., et al. (2012). “Is there a link between N: P ratios and red tides in Tolo Harbour, Hong Kong?,” Pagou, P., and Hallegraeff, G. (Eds.). Proceedings of 14th International Conference on Harmful Algae, Hersonissos-Crete, Greece, 1–5 November 2010. International Society for the Study of Harmful Algae and Intergovernmental Oceanographic Commission of UNESCO 2013, p. 90–92.
Hasle, G. R. (2002). Are most of the domoic acid-producing species of the diatom genus Pseudo-nitzschia cosmopolites? Harmful Algae 1, 137–146. doi: 10.1016/S1568-9883(02)00014-8
Havskum, H., Schlüter, L., Scharek, R., Berdalet, E., and Jacquet, S. (2004). Routine quantification of phytoplankton groups—microscopy or pigment analyses? Mar. Ecol. Prog. Ser. 273, 31–42. doi: 10.3354/meps273031
Hayward, A., Pinkerton, M. H., and Gutierrez-Rodriguez, A. (2023). phytoclass: A pigment-based chemotaxonomic method to determine the biomass of phytoplankton classes. Limnol. Oceanogr. Meth. 21, 220–241. doi: 10.1002/lom3.10541
Heink, U., and Kowarik, I. (2010). What are indicators? On the definition of indicators in ecology and environmental planning. Ecol. Indic. 10, 584–593. doi: 10.1016/j.ecolind.2009.09.009
Heisler, J., Glibert, P. M., Burkholder, J. M., Anderson, D. M., Cochlan, W., Dennison, W. C., et al. (2008). Eutrophication and harmful algal blooms: a scientific consensus. Harmful Algae 8, 3–13. doi: 10.1016/j.hal.2008.08.006
HELCOM (2018). Cyanobacteria Bloom Index (CyaBI). HELCOM pre-core indicator report. Available online at: https://helcom.fi/wp-content/uploads/2019/08/Cyanobacterial-bloom-index-HELCOM-pre-core-indicator-2018.pdf
Henriksen, P. (2002). Effects of nutrient-limitation and irradiance on marine phytoplankton pigments. J. Plankton Res. 24, 835–858. doi: 10.1093/plankt/24.9.835
Herndon, J., and Cochlan, W. P. (2007). Nitrogen utilization by the raphidophyte Heterosigma akashiwo: growth and uptake kinetics in laboratory cultures. Harmful Algae 6, 260–270. doi: 10.1016/j.hal.2006.08.006
Hinder, S. L., Hays, G. C., Edwards, M., Roberts, E. C., Walne, A. W., and Gravenor, M. B. (2012). Changes in marine dinoflagellate and diatom abundance under climate change. Nat. Clim. Chang. 2, 271–275. doi: 10.1038/nclimate1388
Hoagland, P., and Scatasta, S. (2006). “The Economic Effects of Harmful Algal Blooms”, in Ecology of Harmful Algae Ecological Studies, eds. E. Granéli, and J. T. Turner. Berlin, Heidelberg: Springer, 391–402.
Höglander, H., Karlson, B., Johansen, M., Walve, J., and Andersson, A. (2013). Overview of coastal phytoplankton indicators and their potential use in Swedish waters. Havsmiljöinstitutet, Sweden: Deliverable 3.3-1, WATERS Report no. 2013:5.. Available online at: https://waters.gu.se/digitalAssets/1457/1457765_3.3_1_coastal_phytoplankton_indicators.pdf
Hoppenrath, M., Chomerat, N., Horiguchi, T., Murray, S. A., and Rhodes, L. (2023). Marine benthic dinoflagellates: their relevance for science and society (2nd revised edition). Frankfurt am Main, Germany: Senckenberg Gesellschaft für Naturforschung.
Hubbard, K. A., Villac, M. C., Chadwick, C., DeSmidt, A. A., Flewelling, L., Granholm, A., et al. (2023). Spatiotemporal transitions in Pseudo-nitzschia species assemblages and domoic acid along the Alaska coast. PLOS ONE 18, e0282794. doi: 10.1371/journal.pone.0282794
Humborg, C., Ittekkot, V., Cociasu, A., and Bodungen, B.v. (1997). Effect of Danube River dam on Black Sea biogeochemistry and ecosystem structure. Nature 386, 385–388. doi: 10.1038/386385a0
Jaanus, A., Toming, K., Hällfors, S., Kaljurand, K., and Lips, I. (2009). Potential phytoplankton indicator species for monitoring Baltic coastal waters in the summer period. Hydrobiologia 629, 157–168. doi: 10.1007/s10750-009-9768-y
Jauzein, C., Fricke, A., Mangialajo, L., and Lemée, R. (2016). Sampling of Ostreopsis cf. ovata using artificial substrates: Optimization of methods for the monitoring of benthic harmful algal blooms. Mar. Pollut. Bull. 107, 300–304. doi: 10.1016/j.marpolbul.2016.03.047
Jeong, H., Yoo, Y., Park, J., Song, J., Kim, S., Lee, S., et al. (2005). Feeding by phototrophic red-tide dinoflagellates: five species newly revealed and six species previously known to be mixotrophic. Aquatic Microb. Ecol. 40, 133–150. doi: 10.3354/ame040133
Jerney, J., Hällfors, H., Jakobsen, H., Jurgensone, I., Karlson, B., Kremp, A., et al. (2023). DNA Metabarcoding - Guidelines to Monitor Phytoplankton Diversity and Distribution in Marine and Brackish Waters. Copenhagen: Nordisk Ministerråd, 68. doi: 10.6027/temanord2023-505
Karlson, B., Andersen, P., Arneborg, L., Cembella, A., Eikrem, W., John, U., et al. (2021). Harmful algal blooms and their effects in coastal seas of Northern Europe. Harmful Algae 102, 101989. doi: 10.1016/j.hal.2021.101989
Karlson, B., Cusack, C., and Bresnan, E. (2010). Microscopic and Molecular Methods for Quantitative Phytoplankton Analysis. Paris: UNESCO (IOC Manuals and Guides, No. 55), 110.
Kouakou, C. R. C., and Poder, T. G. (2019). Economic impact of harmful algal blooms on human health: a systematic review. J. Water Health 17, 499–516. doi: 10.2166/wh.2019.064
Kraft, K., Seppälä, J., Hällfors, H., Suikkanen, S., Ylöstalo, P., Anglès, S., et al. (2021). First application of IFCB High-Frequency Imaging-in-Flow Cytometry to Investigate Bloom-Forming Filamentous Cyanobacteria in the Baltic Sea. Front. Marine Sci. 8, 594144. doi: 10.3389/fmars.2021.594144
Kudela, R. M., Lane, J. Q., and Cochlan, W. P. (2008). The potential role of anthropogenically derived nitrogen in the growth of harmful algae in California, USA. Harmful Algae 8, 103–110. doi: 10.1016/j.hal.2008.08.019
Kudela, R. M., Seeyave, S., and Cochlan, W. P. (2010). The role of nutrients in regulation and promotion of harmful algal blooms in upwelling systems. Progr. Oceanogr. 85, 122–135. doi: 10.1016/j.pocean.2010.02.008
Kunkel, R., Peterseil, J., Oggioni, A., Wohner, C., Watkins, J., Minić, V., et al. (2019). European Long-Term Ecosystem and Socio-Ecological Research Infrastructure, D3.2 Governance and Data Policy for Sharing and Publishing of Data. European Long-Term Ecosystem and Socio-Ecological Research Infrastructure (eLTER); Annexes, 38. doi: 10.25607/OBP-1778
Kurekin, A. A., Miller, P. I., and Van der Woerd, H. J. (2014). Satellite discrimination of Karenia mikimotoi and Phaeocystis harmful algal blooms in European coastal waters: Merged classification of ocean colour data. Harmful Algae 31, 163–176. doi: 10.1016/j.hal.2013.11.003
Landsberg, J. H. (2002). The effects of harmful algal blooms on aquatic organisms. Rev. Fish. Sci. 10, 113–390. doi: 10.1080/20026491051695
Larkin, S. L., and Adams, C. M. (2007). Harmful algal blooms and coastal business: economic consequences in Florida. Soc. Nat. Resour. 20, 849–859. doi: 10.1080/08941920601171683
Lefebvre, K. A., Frame, E. R., Gulland, F., Hansen, J. D., Kendrick, P. S., Beyer, R. P., et al. (2012). A novel antibody-based biomarker for chronic algal toxin exposure and sub-acute neurotoxicity. PLoS ONE 7, e36213. doi: 10.1371/journal.pone.0036213
Lehtinen, S., Suikkanen, S., Hällfors, H., Tuimala, J., and Kuosa, H. (2021). Phytoplankton morpho-functional trait variability along coastal environmental gradients. Microorganisms 9, 2477. doi: 10.3390/microorganisms9122477
Lenzen, M., Li, M., and Murray, S. A. (2021). Impacts of harmful algal blooms on marine aquaculture in a low-carbon future. Harmful Algae 110, 102143. doi: 10.1016/j.hal.2021.102143
Lin, J., Miller, P. I., Jönsson, B. F., and Bedington, M. (2021). Early warning of harmful algal bloom risk using satellite ocean color and lagrangian particle trajectories. Front. Mar. Sci. 8, 736262. doi: 10.3389/fmars.2021.736262
Litchman, E. (2023). Understanding and predicting harmful algal blooms in a changing climate: a trait-based framework. Limnol. Oceanogr. Lett. 8, 229–246. doi: 10.1002/lol2.10294
Litchman, E., and Klausmeier, C. A. (2008). Trait-based community ecology of phytoplankton. Annu. Rev. Ecol. Evol. Syst. 39, 615–639. doi: 10.1146/annurev.ecolsys.39.110707.173549
Liu, F., Zhang, C., Wang, Y., and Chen, G. (2022). A review of the current and emerging detection methods of marine harmful microalgae. Sci. Total Environ. 815, 152913. doi: 10.1016/j.scitotenv.2022.152913
Liu, Y., Lu, H., and Cui, Y. (2023). A review of marine in situ sensors and biosensors. J. Mar. Sci. Eng. 11, 1469. doi: 10.3390/jmse11071469
Liu, Y., Zhang, P., Du, S., Lin, Z., Zhou, Y., Chen, L., et al. (2019). Sediment as a potential pool for lipophilic marine phycotoxins with the case study of Daya Bay of China. Mar. Drugs 17, 623. doi: 10.3390/md17110623
Loureiro, S., Re,ñé, A., Garcés, E., Camp, J., and Vaqu,é, D. (2011). Harmful algal blooms (HABs), dissolved organic matter (DOM), and planktonic microbial community dynamics at a near-shore and a harbour station influenced by upwelling (SW Iberian Peninsula). J. Sea Res. 65, 401–413. doi: 10.1016/j.seares.2011.03.004
Ludwig, W., Dumont, E., Meybeck, M., and Heussner, S. (2009). River discharges of water and nutrients to the Mediterranean and Black Sea: major drivers for ecosystem changes during past and future decades? Progr. Oceanogr. 80, 199–217. doi: 10.1016/j.pocean.2009.02.001
Lugoli, F., Garmendia, M., Lehtinen, S., Kauppila, P., Moncheva, S., Revilla, M., et al. (2012). Application of a new multi-metric phytoplankton index to the assessment of ecological status in marine and transitional waters. Ecol. Indic. 23, 338–355. doi: 10.1016/j.ecolind.2012.03.030
Magliozzi, C., Palma, M., Druon, J.-N., Palialexis, A., McQuatters-Gollop, A., Varkitzi, I., et al. (2023). Status of pelagic habitats within the EU-Marine Strategy Framework Directive: proposals for improving consistency and representativeness of the assessment. Mar. Policy 148, 105467. doi: 10.1016/j.marpol.2022.105467
Mangialajo, L., Fricke, A., Perez-Gutierrez, G., Catania, D., Jauzein, C., and Lemee, R. (2017). Benthic Dinoflagellate Integrator (BEDI): a new method for the quantification of Benthic Harmful Algal Blooms. Harmful Algae 64, 1–10. doi: 10.1016/j.hal.2017.03.002
Marques, J. C., Salas, F., Patricio, J., Teixeira, H., and Neto, J. (2009). Ecological Indicators for Coastal and Estuarine Environmental Assessment. Southampton; Boston, MA: WIT Press. Available online at: https://www.witpress.com/books/978-1-84564-209-9
Martino, S., Gianella, F., and Davidson, K. (2020). An approach for evaluating the economic impacts of harmful algal blooms: The effects of blooms of toxic Dinophysis spp. on the productivity of Scottish shellfish farms. Harmful Algae 99, 101912. doi: 10.1016/j.hal.2020.101912
Masó, M., and Garcés, E. (2006). Harmful microalgae blooms (HAB); problematic and conditions that induce them. Mar. Pollut. Bull. 53, 620–630. doi: 10.1016/j.marpolbul.2006.08.006
Matthews, M. W., Bernard, S., and Robertson, L. (2012). An algorithm for detecting trophic status (chlorophyll-a), cyanobacterial-dominance, surface scums and floating vegetation in inland and coastal waters. Remote Sens. Environ. 124, 637–652. doi: 10.1016/j.rse.2012.05.032
McCabe, R. M., Hickey, B. M., Kudela, R. M., Lefebvre, K. A., Adams, N. G., Bill, B. D., et al. (2016). An unprecedented coastwide toxic algal bloom linked to anomalous ocean conditions. Geophys. Res. Lett. 43. doi: 10.1002/2016GL070023
McNamee, S. E., Medlin, L. K., Kegel, J., McCoy, G. R., Raine, R., Barra, L., et al. (2023). Distribution, occurrence and biotoxin composition of the main shellfish toxin producing microalgae within European waters: a comparison of methods of analysis. Harmful Algae 55, 112–120. doi: 10.1016/j.hal.2016.02.008
McQuatters-Gollop, A., Johns, D. G., Bresnan, E., Skinner, J., Rombouts, I., Stern, R., et al. (2017). From microscope to management: the critical value of plankton taxonomy to marine policy and biodiversity conservation. Mar. Policy 83, 1–10. doi: 10.1016/j.marpol.2017.05.022
Medlin, L., and Orozco, J. (2017). Molecular techniques for the detection of organisms in aquatic environments, with emphasis on harmful algal bloom species. Sensors 17, 1184. doi: 10.3390/s17051184
Medlin, L. K., Gamella, M., Mengs, G., Serafín, V., Campuzano, S., Pingarrón, J. M., et al. (2020). Advances in the detection of toxic algae using electrochemical biosensors. Biosensors 10, 207. doi: 10.3390/bios10120207
Miller, M. A., Kudela, R. M., Mekebri, A., Crane, D., Oates, S. C., Tinker, M. T., et al. (2010). Evidence for a novel marine harmful algal bloom: cyanotoxin (microcystin) transfer from land to sea otters. PLoS ONE 5, e12576. doi: 10.1371/journal.pone.0012576
Minelli, A., Sarretta, A., Oggioni, A., Bergami, C., Bastianini, M., Bernardi Aubry, F., et al. (2021). Opening marine long-term ecological science: lesson learned from the LTER-Italy site northern adriatic sea. Front. Mar. Sci. 8, 659522. doi: 10.3389/fmars.2021.659522
Moore, S. K., Mickett, J. B., Doucette, G. J., Adams, N. G., Mikulski, C. M., Birch, J. M., et al. (2021). An autonomous platform for near real-time surveillance of harmful algae and their toxins in dynamic coastal shelf environments. J. Mar. Sci. Eng. 9, 336. doi: 10.3390/jmse9030336
Murray, N. J., Phinn, S. R., DeWitt, M., Ferrari, R., Johnston, R., Lyons, M. B., et al. (2019). The global distribution and trajectory of tidal flats. Nature 565, 222–225. doi: 10.1038/s41586-018-0805-8
Murray, S. A., Wiese, M., Stüken, A., Brett, S., Kellmann, R., Hallegraeff, G., et al. (2011). sxtA-based quantitative molecular assay to identify saxitoxin-producing harmful algal blooms in marine waters. Appl. Environ. Microbiol,. 77, 7050–7057. doi: 10.1128/AEM.05308-11
Nichols, D., and Hogan, T. (2022). “Remote sensing technologies to monitor harmful algal blooms in offshore aquaculture,” in World Aquaculture, 20–25. Available online at: https://mclanelabs.com/wp-content/uploads/2022/07/2022.WordAquaculture.D.Nichols.pdf
Niemeijer, D., and de Groot, R. S. (2008). A conceptual framework for selecting environmental indicator sets. Ecol. Indic. 8, 14–25. doi: 10.1016/j.ecolind.2006.11.012
Ní Longphuirt, S., McDermott, G., O'Boyle, S., Wilkes, R., and Stengel, D. B. (2019). Decoupling abundance and biomass of phytoplankton communities under different environmental controls: a new multi-metric index. Front. Mar. Sci. 6, 312. doi: 10.3389/fmars.2019.00312
Nogueira, E., and Figueiras, F. G. (2005). The microplankton succession in the Ría de Vigo revisited: species assemblages and the role of weather-induced, hydrodynamic variability. J. Marine Syst. 54, 139–155. doi: 10.1016/j.jmarsys.2004.07.009
Not, F., Zapata, M., Pazos, Y., Campaña, E., Doval, M., and Rodríguez, F. (2007). Size-fractionated phytoplankton diversity in the NW Iberian coast: a combination of microscopic, pigment and molecular analyses. Aquatic Microbial Ecol. 49, 255–265. doi: 10.3354/ame01144
Nygård, H., Oinonen, S., Hällfors, H. A., Lehtiniemi, M., Rantajärvi, E., and Uusitalo, L. (2016). Price vs. value of marine monitoring. Front. Mar. Sci. 3, 205. doi: 10.3389/fmars.2016.00205
Okazaki, R. R., Sutton, A. J., Feely, R. A., Dickson, A. G., Alin, S. R., Sabine, C. L., et al. (2017). Evaluation of marine pH sensors under controlled and natural conditions for the Wendy Schmidt Ocean Health XPRIZE: Evaluation of marine pH sensors. Limnol. Oceanogr. Meth. 15, 586–600. doi: 10.1002/lom3.10189
Orenstein, E. C., Ratelle, D., Briseño-Avena, C., Carter, M. L., Franks, P. J. S., Jaffe, J. S., et al. (2020). The Scripps Plankton Camera system: a framework and platform for in situ microscopy. Limnol. Oceanogr. Meth,. 18, 681–695. doi: 10.1002/lom3.10394
OSPAR Commission (2003). OSPAR Integrated Report 2003 on the Eutrophication Status of the OSPAR Maritime Area Based Upon the First Application of the Comprehensive Procedure. London: OSPAR Commission.
OSPAR Commission (2008). Second Integrated Report on the Eutrophication Status of the OSPAR Maritime Area. London: OSPAR Commission.
OSPAR Commission (2017). Third Integrated Report on the Eutrophication Status of the OSPAR Maritime Area. London: OSPAR Commission.
Otero, P., and Silva, M. (2022). Emerging marine biotoxins in european waters: potential risks and analytical challenges. Mar. Drugs 20, 199. doi: 10.3390/md20030199
Owen, B. M., Hallett, C. S., Cosgrove, J. J., Tweedley, J. R., and Moheimani, N. R. (2022). Reporting of methods for automated devices: a systematic review and recommendation for studies using FlowCam for phytoplankton. Limnol. Oceanogr. Meth. 20, 400–427. doi: 10.1002/lom3.10496
Özalp, H. B. (2021). A preliminary assessment of the mass mortality of some benthic species due to the mucilage phenomenon of 2021 in the Çanakkale Strait (Dardanelles) and North Aegean Sea. J. Black Sea Mediterr. Environ. 27, 154–166. Available online at: https://blackmeditjournal.org/wp-content/uploads/3-2021_2_154-166.pdf
Palialexis, A., Tornero, V., Barbone, E., Gonzalez, D., Hanke, G., Cardoso, A. C., et al. (2014). In-Depth Assessment of the EU Member States' Submissions for the Marine Strategy Framework Directive under articles 8, 9 and 10. Luxembourg: EUR 26473 EN, Publications Office of the European Union.
Park, T. G., Lim, W. A., Park, Y. T., Lee, C. K., and Jeong, H. J. (2013). Economic impact, management and mitigation of red tides in Korea. Harmful Algae 30, S131–S143. doi: 10.1016/j.hal.2013.10.012
Pazos, Y., and Maneiro, J. (1999). “Algal bloom detection, monitoring and prediction in the Galician Rías (NW Spain)”, in Algal Bloom Detection, Monitoring and Prediction. 3rd Workshop on Public Health, eds. G. Catena, and E. Funari E. Roma: Istituto Superiore di Sanitá, 95.
Pearson, L. A., D'Agostino, P. M., and Neilan, B. A. (2021). Recent developments in quantitative PCR for monitoring harmful marine microalgae. Harmful Algae 108, 102096. doi: 10.1016/j.hal.2021.102096
Perini, F., Bastianini, M., Capellacci, S., Pugliese, L., DiPoi, E., Cabrini, M., et al. (2019). Molecular methods for cost-efficient monitoring of HAB (harmful algal bloom) dinoflagellate resting cysts. Mar. Pollut. Bull. 147, 209–218. doi: 10.1016/j.marpolbul.2018.06.013
Pitcher, G. C., and Fraga, S. (2015). “Harmful Algal Bloom events in the Canary Current Large Marine Ecosystem”, in Oceanographic and biological features in the Canary Current Large Marine Ecosystem, eds. L. Valdés, and I. Déniz-González, I. Paris: IOC Technical Series, No. 115, 175–182. Available online at: https://aquadocs.org/handle/1834/9187 (accessed April 3, 2023).
Poikane, S., Zampoukas, N., Borja, A., Davies, S. P., van de Bund, W., and Birk, S. (2014). Intercalibration of aquatic ecological assessment methods in the European Union: Lessons learned and way forward. Environ. Sci. Policy 44, 237–246. doi: 10.1016/j.envsci.2014.08.006
Racault, M.-F., Platt, T., Sathyendranath, S., A irba, E., Martinez Vicente, V., and Brewin, R. (2014). Plankton indicators and ocean observing systems: support to the marine ecosystem state assessment. J. Plankton Res. 36, 621–629. doi: 10.1093/plankt/fbu016
Ralston, D. K., and Moore, S. K. (2020). Modeling harmful algal blooms in a changing climate. Harmful Algae 91, 101729. doi: 10.1016/j.hal.2019.101729
Rattner, B. A., Wazniak, C. E., Lankton, J. S., McGowan, P. C., Drovetski, S. V., and Egerton, T. A. (2022). Review of harmful algal bloom effects on birds with implications for avian wildlife in the Chesapeake Bay region. Harmful Algae 120, 102319. doi: 10.1016/j.hal.2022.102319
Raven, J. A., Gobler, C. J., and Hansen, P. J. (2020). Dynamic CO2 and pH levels in coastal, estuarine, and inland waters: theoretical and observed effects on harmful algal blooms. Harmful Algae 91, 101594. doi: 10.1016/j.hal.2019.03.012
Regulation (EC) 853/2004 (2004). Regulation (EC) No. 853/2004 of the European Parliament and of the Council of 29 April 2004 laying down specific hygiene rules for food of animal origin. Available at: https://eur-lex.europa.eu/legal-content/EN/TXT/PDF/?uri=CELEX:02004R0853-20230215
Regulation (EC) 2074/2005 (2005). Regulation (EC) 2074/2005 of 5 December 2005 laying down implementing measures for certain products under Regulation (EC) No 853/2004 of the European Parliament and of the Council and for the organisation of official controls under Regulation (EC) No 854/2004 of the European Parliament and of the Council and Regulation (EC) No 882/2004 of the European Parliament and of the Council, derogating from Regulation (EC) No 852/2004 of the European Parliament and of the Council and amending Regulations (EC) No 853/2004 and (EC) No 854/2004 (Text with EEA relevance) OJ L 338, 22.12.2005, 27–59.
Regulation (EU) 2019/627 (2019). Commission Implementing Regulation (EU) 2019/627 of 15 March 2019 laying down uniform practical arrangements for the performance of official controls on products of animal origin intended for human consumption in accordance with Regulation (EU) 2017/625 of the European Parliament and of the Council and amending Commission Regulation (EC) No 2074/2005 as regards official controls. Available online at: https://eur-lex.europa.eu/legal-content/EN/TXT/PDF/?uri=CELEX:02019R0627-20230109&qid=1694612476662 (accessed April 3, 2023).
Regulation (EU) 2021/1709 (2021). Commission Implementing Regulation (EU) 2021/1709 of 23 September 2021 amending Implementing Regulation (EU) 2019/627 as regards uniform practical arrangements for the performance of official controls on products of animal origin. Available online at: https://eur-lex.europa.eu/legal-content/EN/TXT/PDF/?uri=CELEX:32021R1709&qid=1694615974495 (accessed April 3, 2023).
Reñé, A., Satta, C. T., Garcés, E., Massana, R., Zapata, M., Anglès, S., et al. (2011). Gymnodinium litoralis sp. nov. (Dinophyceae), a newly identified bloom-forming dinoflagellate from the NW Mediterranean Sea. Harmful Algae 12, 11–25. doi: 10.1016/j.hal.2011.08.008
Richardson, A. J., Walne, A. W., John, A. W. G., Jonas, T. D., Lindley, J. A., Sims, D. W., et al. (2006). Using continuous plankton recorder data. Progr. Oceanogr. 68, 27–74. doi: 10.1016/j.pocean.2005.09.011
Riegman, R., Noordeloos, A. A. M., and Cadée, G. C. (1992). Phaeocystis blooms and eutrophication of the continental coastal zones of the North Sea. Mar. Biol. 112, 479–484. doi: 10.1007/BF00356293
Robbins, J. R., Bouchet, P. J., Miller, D. L., Evans, P. G. H., Waggitt, J., Ford, A. T., et al. (2022). Shipping in the north-east Atlantic: Identifying spatial and temporal patterns of change. Mar. Pollut. Bull. 179, 113681. doi: 10.1016/j.marpolbul.2022.113681
Rodríguez Rodríguez, G., Villasante, S., and Carme García-Negro, M. D. (2011). Are red tides affecting economically the commercialization of the Galician (NW Spain) mussel farming? Mar. Policy 35, 252–257. doi: 10.1016/j.marpol.2010.08.008
Roesler, C., Uitz, J., Claustre, H., Boss, E., Xing, X., Organelli, E., et al. (2017). Recommendations for obtaining unbiased chlorophyll estimates from in situ chlorophyll fluorometers: a global analysis of WET Labs ECO sensors. Limnol. Oceanogr. Meth. 15, 572–585. doi: 10.1002/lom3.10185
Ruiz-Villarreal, M., Sourisseau, M., Anderson, P., Cusack, C., Neira, P., Silke, J., et al. (2022). Novel methodologies for providing in situ data to HAB early warning systems in the european atlantic area: the PRIMROSE experience. Front. Mar. Sci. 9, 791329. doi: 10.3389/fmars.2022.791329
Ryan, J., Greenfield, D., Marin, R. I., Preston, C., Roman, B., Jensen, S., et al. (2011). Harmful phytoplankton ecology studies using an autonomous molecular analytical and ocean observing network. Limnol. Oceanogr. 56, 1255–1272. doi: 10.4319/lo.2011.56.4.1255
Ryan, J. P., Fischer, A. M., Kudela, R. M., Gower, J. F. R., King, S. A., Marin, R., et al. (2009). Influences of upwelling and downwelling winds on red tide bloom dynamics in Monterey Bay, California. Cont. Shelf Res. 29, 785–795. doi: 10.1016/j.csr.2008.11.006
Sanseverino, I., Conduto, D., Pozzoli, L., Dobricic, S., and Lettieri, T. (2016). Algal bloom and its economic impact. Luxembourg: EUR 27905 EN, Publications Office of the European Union.
Schlüter, L., Møhlenberg, F., Havskum, H., and Larsen, S. (2000). The use of phytoplankton pigments for identifying and quantifying phytoplankton groups in coastal areas: testing the influence of light and nutrients on pigment/chlorophyll a ratios. Mar. Ecol. Prog. Ser. 192, 49–63. doi: 10.3354/meps192049
Scholin, C., Doucette, G., Jensen, S., Roman, B., Pargett, D., Marin, R. Iii, et al. (2009). Remote detection of marine microbes, small invertebrates, harmful algae, and biotoxins using the environmental sample processor (ESP). Oceanography 22, 158–167. doi: 10.5670/oceanog.2009.46
Scorzetti, G., Brand, L. E., Hitchcock, G. L., Rein, K. S., Sinigalliano, C. D., and Fell, J. W. (2009). Multiple simultaneous detection of Harmful Algal Blooms (HABs) through a high throughput bead array technology, with potential use in phytoplankton community analysis. Harmful Algae 8, 196–211. doi: 10.1016/j.hal.2008.05.003
Serret, P., Aligizaki, K., Davidson, K., Caricato, P., Gago, A., Jakobsen, H., et al. (2019). Monitoring of Toxin-producing Phytoplankton in Bivalve Mollusc Harvesting Areas Guide to Good Practice: Technical Application. Vigo: European Union Reference Laboratory for Marine Biotoxins.
Shang, S., Lee, Z., Lin, G., Hu, C., Shi, L., Zhang, Y., et al. (2017). Sensing an intense phytoplankton bloom in the western Taiwan Strait from radiometric measurements on a UAV. Remote Sens. Environ. 198, 85–94. doi: 10.1016/j.rse.2017.05.036
Shapiro, J. (1984). Blue-green dominance in lakes: the role and management significance of pH and CO2. Int. Revue ges. Hydrobiol. Hydrogr. 69, 765–780. doi: 10.1002/iroh.19840690602
Shumway, S. E., Burkholder, J. M., and Morton, S. L. (2018). Harmful Algal Blooms: A Compendium Desk Reference. New York: John Wiley and Sons, Ltd.
Sieracki, C., Sieracki, M., and Yentsch, C. (1998). An imaging-in-flow system for automated analysis of marine microplankton. Mar. Ecol. Prog. Ser. 168, 285–296. doi: 10.3354/meps168285
Silliman, J. (2022). Harmful algal blooms: No good, just the bad and the ugly. Eos 103. doi: 10.1029/2022EO220440
Silva, M., Barreiro, A., Rodriguez, P., Otero, P., Azevedo, J., Alfonso, A., et al. (2013). New Invertebrate vectors for PST, spirolides and okadaic acid in the North Atlantic. Marine Drug. 11, 1936–1960. doi: 10.3390/md11061936
Silva, M., Rey, V., Barreiro, A., Kaufmann, M., Neto, A. I., Hassouani, M., et al. (2018). Paralytic shellfish toxins occurrence in non-traditional invertebrate vectors from north atlantic waters (Azores, Madeira, and Morocco). Toxins 10, 362. doi: 10.3390/toxins10090362
Silva, M., Rodríguez, I., Barreiro, A., Kaufmann, M., Neto, A. I., Hassouani, M., et al. (2020). Lipophilic toxins occurrence in non-traditional invertebrate vectors from North Atlantic Waters (Azores, Madeira, and Morocco): Update on geographical tendencies and new challenges for monitoring routines. Marine Pollut. Bullet. 161, 111725. doi: 10.1016/j.marpolbul.2020.111725
Smayda, T. J. (2008). Complexity in the eutrophication–harmful algal bloom relationship, with comment on the importance of grazing. Harmful Algae 8, 140–151. doi: 10.1016/j.hal.2008.08.018
Smayda, T. J., and Reynolds, C. S. (2003). Strategies of marine dinoflagellate survival and some rules of assembly. J. Sea Res. 49, 95–106. doi: 10.1016/S1385-1101(02)00219-8
Smith, M., and Bernard, S. (2019). “Satellite ocean colour based Harmful Algal Bloom identification for improved risk assessment and mitigation”, ESS Open Archive, 7.
Smith, M. E., and Bernard, S. (2020). Satellite ocean color based harmful algal bloom indicators for aquaculture decision support in the Southern Benguela. Front. Mar. Sci. 7, 61. doi: 10.3389/fmars.2020.00061
Sohrabi, H., Hemmati, A., Majidi, M. R., Eyvazi, S., Jahanban-Esfahlan, A., Baradaran, B., et al. (2021). Recent advances on portable sensing and biosensing assays applied for detection of main chemical and biological pollutant agents in water samples: a critical review. Trends Analyt. Chem. 143, 116344. doi: 10.1016/j.trac.2021.116344
Sordo, I., Barton, E. D., Cotos, J. M., and Pazos, Y. (2001). An inshore poleward current in the nw of the iberian peninsula detected from satellite images, and its relation with G. catenatum and D. acuminata blooms in the Galician Rias. Estuar. Coast. Shelf Sci. 53, 787–799. doi: 10.1006/ecss.2000.0788
Spatharis, S., and Tsirtsis, G. (2010). Ecological quality scales based on phytoplankton for the implementation of Water Framework Directive in the Eastern Mediterranean. Ecol. Indic. 10, 840–847. doi: 10.1016/j.ecolind.2010.01.005
Stauffer, B. A., Bowers, H. A., Buckley, E., Davis, T. W., Johengen, T. H., Kudela, R., et al. (2019). Considerations in harmful algal bloom research and monitoring: perspectives from a consensus-building workshop and technology testing. Front. Mar. Sci. 6, 399. doi: 10.3389/fmars.2019.00399
Stern, R., Moore, S., Trainer, V., Bill, B., Fischer, A., and Batten, S. (2018). Spatial and temporal patterns of Pseudo-nitzschia genetic diversity in the North Pacific Ocean from the Continuous Plankton Recorder survey. Marine Ecol. Prog. Series 606, 7–28. doi: 10.3354/meps12711
Stumpf, R. P., Culver, M. E., Tester, P. A., Tomlinson, M., Kirkpatrick, G. J., Pederson, B. A., et al. (2003). Monitoring Karenia brevis blooms in the Gulf of Mexico using satellite ocean color imagery and other data. Harmful Algae 2, 147–160. doi: 10.1016/S1568-9883(02)00083-5
Sun, K., Cui, W., and Chen, C. (2021). Review of Underwater Sensing Technologies and Applications. Sensors 21, 7849. doi: 10.3390/s21237849
Tao, B., Mao, Z., Lei, H., Pan, D., Shen, Y., Bai, Y., et al. (2015). A novel method for discriminating Prorocentrum donghaiense from diatom blooms in the East China Sea using MODIS measurements. Remote Sens. Environ. 158, 267–280. doi: 10.1016/j.rse.2014.11.004
Tester, P. A., Kibler, S. R., Holland, W. C., Usup, G., Vandersea, M. W., Leaw, C. P., et al. (2014). Sampling harmful benthic dinoflagellates: comparison of artificial and natural substrate methods. Harmful Algae 39, 8–25. doi: 10.1016/j.hal.2014.06.009
Tett, P., Carreira, C., Mills, D. K., Van Leeuwen, S., Foden, J., Bresnan, E., et al. (2008). Use of a Phytoplankton Community Index to assess the health of coastal waters. ICES J. Mar. Sci. 65, 1475–1482. doi: 10.1093/icesjms/fsn161
Themistocleous, K. (2021). “Monitoring aquaculture fisheries using Sentinel-2 images by identifying plastic fishery rings,” in Proceedings of the Earth Resources and Environmental Remote Sensing/GIS Applications XII, eds. K. Schulz, K. G. Nikolakopoulos, and U. Michel, 30.
Theodorou, J. A., Moutopoulos, D. K., and Tzovenis, I. (2020). Semi-quantitative risk assessment of Mediterranean mussel (Mytilus galloprovincialis L.) harvesting bans due to harmful algal bloom (HAB) incidents in Greece. Aquac. Econ. Manag. 24, 273–293. doi: 10.1080/13657305.2019.1708994
Tornero Alvarez, M. V., Palma, M., Boschetti, S., Cardoso, A. C., Druon, J., Kotta, M., et al. (2023). Marine Strategy Framework Directive: Review and Analysis of eu member states' 2020 Reports on Monitoring Programmes (MSFD Article 11). Luxembourg: EUR 31181 EN. Publications Office of the European Union.
Torres Palenzuela, J. M., González Vilas, L., Bellas, F. M., Garet, E., González-Fernández, Á., and Spyrakos, E. (2019). Pseudo-nitzschia blooms in a coastal upwelling system: remote sensing detection, toxicity and environmental variables. Water 11, 1954. doi: 10.3390/w11091954
Trainer, V. L. (2020). GlobalHAB. evaluating, reducing and mitigating the cost of harmful algal blooms: a compendium of case studies. PICES Sci. Rep. 59, 107. Available online at: https://meetings.pices.int/publications/scientific-reports/Report59/Rpt59.pdf (accessed March 8, 2023).
Trapp, A., Heuschele, J., and Selander, E. (2021). Eavesdropping on plankton—can zooplankton monitoring improve forecasting of biotoxins from harmful algae blooms? Limnol. Oceanogr. 66, 3455–3471. doi: 10.1002/lno.11891
Truelove, N. K., Patin, N. V., Min, M., Pitz, K. J., Preston, C. M., Yamahara, K. M., et al. (2022). Expanding the temporal and spatial scales of environmental DNA research with autonomous sampling. Environ. DNA 4, 972–984. doi: 10.1002/edn3.299
U. S. Integrated Ocean Observing System (IOOS) (2017). Manual for Real-Time Quality Control of Phytoplankton Data.
Valbi, E., Ricci, F., Capellacci, S., Casabianca, S., Scardi, M., and Penna, A. (2019). A model predicting the PSP toxic dinoflagellate Alexandrium minutum occurrence in the coastal waters of the NW Adriatic Sea. Sci. Rep. 9, 4166. doi: 10.1038/s41598-019-40664-w
Walsh, J. J., Tomas, C. R., Steidinger, K. A., Lenes, J. M., Chen, F. R., Weisberg, R. H., et al. (2011). Imprudent fishing harvests and consequent trophic cascades on the West Florida shelf over the last half century: a harbinger of increased human deaths from paralytic shellfish poisoning along the southeastern United States, in response to oligotrophication? Cont. Shelf Res. 31, 891–911. doi: 10.1016/j.csr.2011.02.007
Wang, Z., Liu, L., Tang, Y., Li, A., Liu, C., Xie, C., et al. (2022). Phytoplankton community and HAB species in the South China Sea detected by morphological and metabarcoding approaches. Harmful Algae 118, 102297. doi: 10.1016/j.hal.2022.102297
Wei, Y., Jiao, Y., An, D., Li, D., Li, W., and Wei, Q. (2019). Review of dissolved oxygen detection technology: from laboratory analysis to online intelligent detection. Sensors 19, 3995. doi: 10.3390/s19183995
Weithoff, G., and Beisner, B. E. (2019). Measures and approaches in trait-based phytoplankton community ecology – from freshwater to marine ecosystems. Front. Mar. Sci. 6, 40. doi: 10.3389/fmars.2019.00040
Wells, M. L., Trainer, V. L., Smayda, T. J., Karlson, B. S. O., Trick, C. G., Kudela, R. M., et al. (2015). Harmful algal blooms and climate change: learning from the past and present to forecast the future. Harmful Algae 49, 68–93. doi: 10.1016/j.hal.2015.07.009
WG GES (2011). Common Understanding of (Initial) Assessment, Determination of Good Environmental Status (GES) and Establishment of Environmental Targets (Articles 8, 9 and 10 MSFD). Available online at: https://circabc.europa.eu/d/a/workspace/SpacesStore/ae13d0d6-8787-4d62-b2b6-1718cf760fe8/CommonUnderstandingArt.8-9-10_Nov2011.doc (accessed April 3, 2023).
Work, T. M., Barr, B., Beale, A. M., Fritz, L., Quilliam, M. A., and Wright, J. L. C. (1993). Epidemiology of domoic acid poisoning in brown pelicans (Pelecanus occidentalis) and Brandt's Cormorants (Phalacrocorax penicillatus) in California. J. Zoo Wildl. Med. 24, 54–62.
Xiao, X., Agust,í, S., Pan, Y., Yu, Y., Li, K., Wu, J., et al. (2019). Warming amplifies the frequency of harmful algal blooms with eutrophication in chinese coastal waters. Environ. Sci. Technol. 53, 13031–13041. doi: 10.1021/acs.est.9b03726
Yarimizu, K., Sildever, S., Hamamoto, Y., Tazawa, S., Oikawa, H., Yamaguchi, H., et al. (2021). Development of an absolute quantification method for ribosomal RNA gene copy numbers per eukaryotic single cell by digital PCR. Harmful Algae 103, 102008. doi: 10.1016/j.hal.2021.102008
Yasumoto, T., Oshima, Y., Murakami, Y., Nakajima, I., Bagnis, R., and Fukuyo, Y. (1980). Toxicity of benthic dinoflagellates found in coral reef. Nippon Suisan Gakkaishi 46, 327–331. doi: 10.2331/suisan.46.327
Young, N., Sharpe, R. A., Barciela, R., Nichols, G., Davidson, K., Berdalet, E., et al. (2020). Marine harmful algal blooms and human health: a systematic scoping review. Harmful Algae 98, 101901. doi: 10.1016/j.hal.2020.101901
Zampoukas, N., Palialexis, A., Duffek, A., Graveland, J., Giorgi, G., Hagebro, C., et al. (2014). Technical guidance on monitoring for the Marine Strategy Framework Directive. Luxembourg: EUR 26499 EN, Publications Office of the European Union.
Zhang, Y., Ryan, J. P., Kieft, B., Hobson, B. W., McEwen, R. S., Godin, M. A., et al. (2019). Targeted sampling by autonomous underwater vehicles. Front. Mar. Sci. 6, 415. doi: 10.3389/fmars.2019.00415
Keywords: decision support tools, ecosystem-based management, indicators, marine monitoring, eutrophication, marine biotoxins, environmental assessment, pressures and impacts
Citation: Sagarminaga Y, Garcés E, Francé J, Stern R, Revilla M, Magaletti E, Bresnan E, Tsirtsis G, Jakobsen HH, Sampedro N, Reñé A, Camp J, Borja Á, Rodríguez JG, Spada E, Pagou K, De Angelis R, Lanzén A, Ferrer L, Borrello P, Boicenco L, Kobos J, Mazaris A and Katsanevakis S (2023) New tools and recommendations for a better management of harmful algal blooms under the European Marine Strategy Framework Directive. Front. Ocean Sustain. 1:1298800. doi: 10.3389/focsu.2023.1298800
Received: 22 September 2023; Accepted: 08 November 2023;
Published: 07 December 2023.
Edited by:
Louis Celliers, Climate Service Center Germany (GERICS), GermanyReviewed by:
Yolanda Pazos, Instituto Tecnolóxico para o Control do Medio Mariño de Galicia (INTECMAR), SpainNatacha Nogueira, Governo Regional da Madeira, Portugal
Copyright © 2023 Sagarminaga, Garcés, Francé, Stern, Revilla, Magaletti, Bresnan, Tsirtsis, Jakobsen, Sampedro, Reñé, Camp, Borja, Rodríguez, Spada, Pagou, De Angelis, Lanzén, Ferrer, Borrello, Boicenco, Kobos, Mazaris and Katsanevakis. This is an open-access article distributed under the terms of the Creative Commons Attribution License (CC BY). The use, distribution or reproduction in other forums is permitted, provided the original author(s) and the copyright owner(s) are credited and that the original publication in this journal is cited, in accordance with accepted academic practice. No use, distribution or reproduction is permitted which does not comply with these terms.
*Correspondence: Yolanda Sagarminaga, eXNhZ2FybWluYWdhQGF6dGkuZXM=