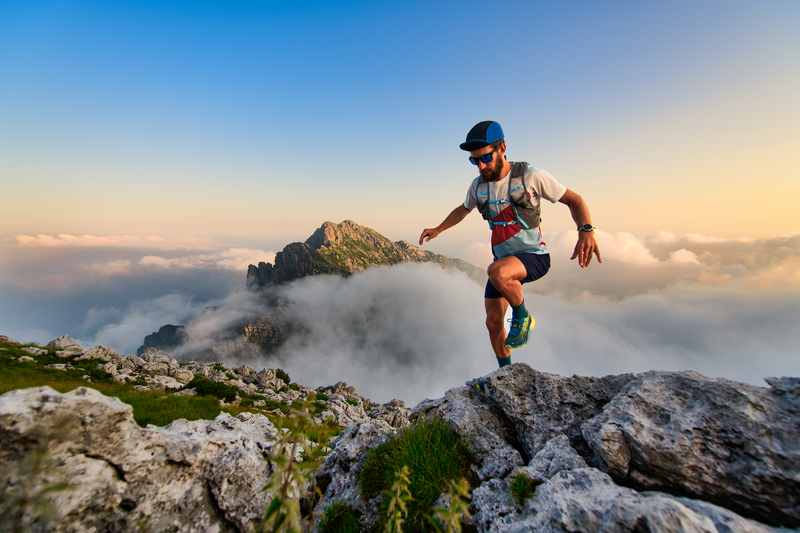
94% of researchers rate our articles as excellent or good
Learn more about the work of our research integrity team to safeguard the quality of each article we publish.
Find out more
ORIGINAL RESEARCH article
Front. Nutr. , 03 February 2025
Sec. Nutrition, Psychology and Brain Health
Volume 12 - 2025 | https://doi.org/10.3389/fnut.2025.1440373
This article is part of the Research Topic Nutrients, Neurotransmitters and Brain Energetics - Volume II View all 12 articles
Introduction: Dietary interventions such as caloric restriction (CR) exert positive effects on brain health. Unfortunately, poor compliance hinders the success of this approach. A proposed alternative is resveratrol (Rsv), a CR-mimetic known to promote brain health. Direct comparison between the effects of Rsv and CR on brain health is lacking, with limited knowledge on their sex-specific effects. Therefore, we aimed to compare and unravel the sex-specific impact of these dietary interventions on spontaneous brain activity.
Methods: Here, we used resting-state fMRI to investigate functional connectivity (FC) changes in five prominent resting-state brain networks (RSNs) in healthy 4 month old male and female F344 rats supplemented to either 40% CR or daily Rsv supplementation (10 mg/kg, oral) for the duration of 1 month.
Results: Our results demonstrated a decreased body weight (BW) in CR rats, as well as an increase in body weight in male Rsv supplemented rats, compared to female Rsv supplemented rats, whereas this difference between sexes was not observed in the control or CR groups. Furthermore, we found that both CR or Rsv supplementation induce a female-specific decrease of FC between the subcortical network and hippocampal network, and between the subcortical network and lateral cortical network. Moreover, Rsv supplementation lowered FC within the hippocampal network and between the hippocampal and the default mode like network, the lateral cortical network and the sensory network—an effect not observed for the CR rats.
Discussion: Our findings reveal that both CR and Rsv induce a similar female-specific decrease of FC in RSNs associated with memory and emotion, all the while CR and Rsv induce dissimilar changes in body weight and other within- and between-RSN FC measures. Altogether, this study provides insight into the effects and comparability of short-term CR and Rsv supplementation on brain connectivity within- and between-RSNs in both male and female F344 rats, providing a FC reference for future research of dietary effects.
Due to the constant exponential growth of the elderly population world-wide, the search for preventative strategies to preserve cognitive function and promote healthy aging have become increasingly important (1). Lifestyle modifications, including regular exercise and dietary adjustments, are known to play a crucial role in this endeavor. One proposed dietary adjustment is caloric restriction (CR) (2), defined as a significant reduction of energy intake without the risk of malnutrition. Through a variety of pathways, short- and long-term CR has been shown to positively influence brain health by reducing neuroinflammation (3) and oxidative stress (4), while simultaneously enhancing neurovascular function (5). In animal models of aging and age-related neurodegenerative diseases, CR has been shown to prevent neuronal degradation (6, 7), enhance neurogenesis in the hippocampus (8), as well as prevent against the age-related decline in motor coordination (9) and learning (7). These findings collectively highlight the potential of CR as a promising approach to maintain and improve brain health.
Despite the proven positive effect of CR on brain health, poor compliance to drastic, life-long CR hinders its success as a therapeutic approach (10). In light of this challenge, CR-mimetics have emerged as a potential alternative strategy. CR-mimetics aim to activate the same pathways and mechanisms and are therefore hypothesized to mimic the beneficial effects of CR on brain health without the life-long commitment to reduced caloric intake (11, 12). One of the most extensively studied CR-mimetics is resveratrol (Rsv), a polyphenol and phytoalexin, present in high concentrations in, for example, blue berries and red grapes. Rsv has shown to exert positive effects on neuroinflammation (13) and oxidative stress (14). The hypothesis of Rsv serving a similar function as CR is further solidified through reported findings from studies in animal models of aging and age-related neurodegenerative diseases (15). Here, Rsv is reported to preserve cognitive function (16) and neurovascular coupling (17) in aging mice, and exert neuroprotective effects in neurodegenerative diseases such as Alzheimer’s (18) and Parkinson’s Disease (19) through similar pathways as reported for CR. These findings further support the hypothesis that Rsv may serve as a viable alternative to CR in promoting brain health and potentially delaying the onset of aging and age-related neurodegenerative diseases.
Resting-state functional MRI (rsfMRI), a non-invasive neuroimaging method, has proven itself as a powerful tool to assess brain function (20). With rsfMRI, one measures the blood-oxygenation level dependent (BOLD)-contrast, indirectly reflecting fluctuations in neuronal activity via neurovascular coupling while the brain is at rest. The correlation between the BOLD signal time series of brain regions is expressed as functional connectivity (FC), where regions that exhibit highly correlated BOLD signals form the resting-state networks (RSNs) (21). The most prominent RSN in humans is the default mode network (DMN), typically anticorrelated to the frontoparietal network (FPN) (22). Changes in FC as a result of CR and Rsv have been reported in regions belonging to the hippocampal network (Hipp), FPN and the DMN (23–26), emphasizing the potential of rsfMRI to assess changes in brain function as a result of dietary interventions.
While these findings confirm that CR or Rsv supplementation alters brain connectivity, there is a lack of research directly comparing the effects of these dietary interventions. Moreover, even though both CR and Rsv supplementation have been hypothesized to exert effects on brain function in a sex-dependent manner (27, 28), research on the possible sex-specific effects that both dietary interventions can exert on brain connectivity, is sparce. Clinical trials assessing dietary effects are subject to challenges due to low patient adherence, high susceptibility to confounding variables, high patient dropout rates and limited follow-up periods (29). The use of animal models can overcome these challenges, aiding to provide critical insight into the sex-specific effects of CR or Rsv on brain connectivity. RsfMRI is well established in rodents, showing similar findings compared to humans regarding RSNs. Rodent analogs of the human DMN and FPN, along with other major RSNs, have been identified as the default mode-like network (DMLN), the lateral cortical network (LCN), the hippocampal (Hipp), the sensory (Sens) and subcortical (SubC) network (30). In this study, we hypothesized that CR or Rsv supplementation induces changes in connectivity within and between these RSNs in a sex-specific manner. Therefore, we aimed to characterize and compare the sex-specific effects of short-term CR and Rsv supplementation on brain connectivity, using rsfMRI to assess FC changes within and between the five prominent rodent RSNs as a result of dietary intervention.
F344 rats (RRID: RGD_60994, Charles River, Italy) used within this study were bred in-house. A total of 42 male and female F344 rats (n = 21/sex) were single-housed at 3 months of age for the duration of the experiment, and kept under controlled environmental conditions [12-h light/dark cycle, (22 ± 2)°C, 40–60% humidity]. Water was provided at libitum. All procedures were in accordance with the guidelines approved by the European Ethics Committee (decree 2010/63/EU), and were approved by the Committee on Animal Care and Use at the University of Antwerp, Belgium (ECD: 2021-59).
Three-month-old rats were randomly divided into 3 groups (Figure 1), receiving dietary intervention and Rsv supplementation for the duration of 4 weeks, until the age of 4 months. The first group served as controls. The second group received daily Rsv (10 mg/kg, oral, High Potency Trans-Resveratrol 600, Doctor’s Best®, California, United States) supplementation. The third group was exposed to a gradual weekly decrease in caloric uptake by 40%. To allow adaptation to restricted feeding, the animals were fed 1 week with 15% CR, a second week with 27.5% CR and 40% CR thereafter. Food fortified with vitamins and minerals was provided to avoid malnutrition (Rat/Mouse Fortified, Ssniff®, Germany). CR of 15, 27.5, and 40% was calculated based on averaged food intake of Ctrl (male/female n = 3/3) and Rsv (male/female n = 3/2) rats, adjusted for age and sex. Control and Rsv supplemented rats were provided standard chow (Ssniff®, Germany) ad libitum. Rsv solubilized in 99.8% ethanol (Thermo Fisher Scientific), was applied to dried apple chips (JR Farm, Germany) and supplemented daily to the rats in the Rsv group. To minimize the difference between conditions, all CR and control rats were supplemented with a daily dose of dried apple chips with vehicle only (99.8% ethanol). This volume of ethanol was determined through averaging the daily Rsv volumes, adjusted for age and sex.
Figure 1. Overview of the experimental dietary treatment paradigm and study design. Number of animals per sex and group are given. Dietary intervention and supplementation of the apple chips starts at 3 months of age (3M). RsfMRI data are acquired at 4 months of age (4M). The apple represents the supplementation of Rsv or its vehicle using dried apple chips. Created with Biorender.com.
Subjects were weighed prior to the start of the dietary intervention and each week afterwards for the duration of the experiment. The percentage body weight (BW) change respective to the starting weight was calculated for each subject.
RsfMRI data of the rats were acquired at 4 months of age, after 1 month of CR or Rsv supplementation. Rats were anesthesia using an isoflurane-medetomidine protocol: an established anesthesia protocol for rsfMRI in rodents (31–34). Anesthesia was induced using 5% isoflurane (IsoFlo®, Zoetis, United States), administered with a gaseous mixture of 200 mL/min O2 and 400 mL/min N2. For further animal handling and positioning, the isoflurane level was reduced to 3%. After positioning, a subcutaneous bolus injection of medetomidine (0.05 mg/kg, Domitor®, Vetoquinol, France) was administered. Continuous subcutaneous infusion of medetomidine (0.1 mg/kg/h) started 15 min after bolus administration, with the isoflurane level being lowered to 0.4%, to be maintained throughout the whole MRI session. Physiological parameters (breathing rate, heart rate, O2 saturation and body temperature) of the animal were closely monitored during the entire procedure (MR-compatible Small Animal Monitoring, Gating, and heating system, SA Instruments). Body temperature was maintained at 37 (± 0.5) °C using a feedback-controlled warm air circuitry (MR-compatible Small Animal Heating System, SA Instruments Inc., United States).
MRI data were acquired on a 7 T Pharmascan MR scanner (Bruker®, Germany) with a volume resonator coil for radiofrequency excitation and a 2 × 2 channel receiver head radiofrequency coil for signal detection. To ensure uniform slice positioning between subjects, multi-slice T2-weighted (T2w) TurboRARE images were acquired in three directions (echo time (TE): 33 ms, repetition time (TR): 1800 ms, RARE factor: 8, field of view (FOV): (35 × 35) mm2, matrix: [256 × 256]). Whole-brain rsfMRI data were acquired 40 min after the bolus of medetomidine using a single shot gradient echo, echo-planar imaging (EPI) sequence (TE: 18 ms, TR: 600 ms, FOV (30 × 30) mm2, matrix [96 × 96], 12 coronal slices of 1 mm, slice gap: 0.1 mm, 1000 repetitions), for a total of 10 min. An anatomical 3D image was acquired for registration purposes, with a T2w-TurboRARE sequence (TR: 1,800 ms, TE: 36 ms, RARE factor: 16, FOV: (35 × 35 × 16) mm3, acquisition matrix: [256 × 256 × 32], reconstruction matrix: [256 × 256 × 64]). At the end of the scan session, a subcutaneous injection of 0.1 mg/kg atipamezole (Antisedan®, Pfizer, Germany) was administered to counteract the effects of the medetomidine anesthesia and the animals were allowed to recover under a heating lamp. All animals recovered within 15–20 min after the end of the scan session.
All preprocessing steps were performed with MATLAB R2020a (Mathworks, Natick, MA) and ANTs (Advanced Normalization Tools). The rsfMRI data were padded using an in-house MATLAB script. Debiasing, realignment, normalization, co-registration and smoothing of the data was performed using SPM 12 software (Statistical Parametric Mapping). First, subject-specific 3Ds were debiased after which a study specific 3D-template was created from a subset of animals (male/female: Ctrl n = 2/2, Rsv n = 2/2, CR n = 2/3) in ANTs. All individual 3Ds were normalized to the study-specific 3D template using a global 12-parameter affine transformation followed by a non-linear deformation protocol. The rsfMRI EPI images were realigned to the first EPI image using a 6-parameter rigid body spatial transformation estimated using a least-squares approach. RsfMRI data were co-registered to the animal’s respective 3D image using a global 12-parameter affine transformation with mutual information used as similarity metric and normalized to the study-specific template using the combined transformation parameters. RsfMRI data were smoothed in-plane using a Gaussian kernel with full width at half maximum of twice the voxel size and filtered (0.01–0.2 Hz) with a Butterworth band-pass filter. Finally, quadratic detrending was performed on the filtered images. During the entire process, a total of five subjects [Ctrl (male/female n = 2/1), CR (male n = 2)] were removed from further analysis due to poor quality of the data.
Region of interest (ROI)-based FC analysis was performed on the preprocessed rsfMRI data. A neuroanatomical atlas comprised of 71 anatomical parcels (Fischer 3441), was warped onto the study-specific template and down-sampled (ANTs) to match the EPI space. Out of the 71 parcels, 43 unilateral gray matter ROIs (for both the left (L) and right (R) hemisphere for each region) were selected, excluding regions sensitive to susceptibility artifacts, transient effects and small size. These selected ROIs represent the five prominent rodent RSNs: the default mode-like network, the hippocampal network (Hipp), the sensory network (Sens), the lateral cortical network (LCN) and the subcortical network (SubC) (Supplementary Table S1). For each subject, Pearson correlation coefficients between the ROI-averaged BOLD signal timeseries of each pair of ROIs were calculated and Fisher z-transformed yielding subject-wise 43 × 43 FC matrices. FC within each network was calculated by averaging across FC values between all ROIs belonging to the network. Similarly, between-network FC was calculated by averaging across FC values between all pairs of ROIs belonging to both networks.
The ROI-based and network-based FC matrices were subjected to an one-sample t-test (FDR corrected, Benjamini-Hochberg procedure, p < 0.05), within group (per sex and treatment). All data was tested for normality using the Shapiro–Wilk test determining the goodness of a normal fit. All data was normally distributed. The BW at week 0, the percentile change in BW at 4 weeks of treatment and the outcomes of the network-based FC were analyzed using a two-way ANOVA (treatment, sex, treatment*sex). In case of a significant treatment*sex interaction, post-hoc tests were performed using Student’s t-test with FDR correction (Benjamini-Hochberg procedure, p < 0.05). When no significant treatment*sex interaction was present, the interaction was removed and the model was recalculated using only the main effects (treatment and sex). In case of a significant treatment effect, post-hoc tests were performed on all groups using a Tukey HSD test. Outlier detection was performed using a principal component analysis, per sex and treatment. Subjects with a Hotelling T2 statistics index higher than the 95% confidence interval were marked as outliers. Subjects with more than 8 out of 15 within- and between-network FC values marked as outliers were excluded. Statistical analyzes were performed using JMP Pro 17 (SAS Institute Inc.) and MATLAB R2020a (Mathworks, Natick, MA). Graphical representation of the data was created using GraphPad Prism (version 9.4.1. for Windows, GraphPad Software, San Diego, California United States) and Adobe Illustrator (Adobe Inc.).
In order to test the randomness of the treatment groups prior to dietary intervention, we tested for the effects of treatment and sex on body weight at week 0. No significant effects were found for treatment (p = 0.4324) or the interaction treatment*sex (p = 0.6471), but, as expected, we did find a significant sex effect (p < 0.0001) on BW, where males had a significantly higher weight compared to females (Figure 2A).
Figure 2. Effects of dietary intervention on body weight (BW). (A) Mean ± SD BW in grams (g) at baseline, prior to the start of the dietary interventions (week 0). The colors of the bars are indicative of the group each subject was assigned to [control (Ctrl), resveratrol (Rsv), caloric restricted (CR)]. Solid bars represent females and open bars represent the males. Dots represent individual subject data points. (B) Percentage change in BW (mean ± SD) with respect to baseline, over the course of the 4 week treatment, Ctrl, Rsv and CR group. Bar graph (insert) shows the percentage change in BW (mean ± SD) at week 4. Asterisks indicate the levels of statistical significance: **p < 0.01, ****p < 0.0001.
Next, we aimed to determine the effect of short-term dietary intervention on BW, by evaluating the percentile change in BW over the course of treatment, from the start of the intervention (Figure 2B). Over the course of the intervention, we observed a positive percentage change of BW in both Ctrl and Rsv rats indicative of weight gain, while observing a negative percentage BW change in the CR rats, indicative of weight loss. Statistical analysis of the percent change in BW at week 4 demonstrated a significant treatment*sex interaction effect (p = 0.031). Post-hoc analysis revealed that after 4 weeks the percentage BW change was significantly different in CR female rats [(mean ± SD) %, (−9.18 ± 1.41)%] when compared to female Ctrl [(6.94 ± 2.98)%, p < 0.0001] and female Rsv [(7.92 ± 1.44)%, p < 0.0001] rats. Similarly, we observed a significant difference in male CR rats (−11.51 ± 3.73)% compared to male Ctrl [(9.95 ± 1.67)%, p < 0.0001] and male Rsv [(10.33 ± 1.71)%, p < 0.0001] rats. Moreover, male Rsv supplemented rats had a higher percentage increase in BW compared to the female Rsv supplemented rats (p = 0.009). This sex-difference was not observed in the Ctrl (p = 0.1084) or CR (p = 0.0739) groups.
Next, we investigated the effect of dietary intervention on brain connectivity. To evaluate these effects on FC between ROIs and within and between networks, we first calculated average ROI-based FC matrices between the 43 predefined ROIs, per sex and treatment (Figure 3A), providing insight into the distribution of FC across the groups. Our analysis revealed that within group, FC of regions associated with the DMLN, Hipp, Sens and LCN were statistically significant and thus robustly represented in each of the groups. ROI-based FC, mainly within the SubC [caudate putamen (CPu), medial septum (MS), thalamus (Thal), hypothalamus (Hyp) and nucleus Accumbens (nAcc)], were often not statistically significant in the males, irrespective of treatment. The total number of significant connections was higher in females when compared to males in each group. An additional graphical representation of the ROI-based FC matrices was generated (Supplementary Figure S1), showing strong inter-hemispheric FC within the DMLN and LCN, and intra-hemispheric FC between DMLN, Hipp and Sens. These observations were primarily noted for the Ctrl and CR groups and to a lesser extent in the Rsv supplemented group.
Figure 3. Functional connectivity (FC) in male and female Ctrl, Rsv supplemented and CR rats. (A) ROI-based FC matrices displaying the mean FC between ROI pairs for males (lower half) and females (upper half) per treatment group (Ctrl = control, CR = caloric restriction, Rsv = resveratrol, zFC = z-scored functional connectivity). The 43 regions of interest with left (L) and right (R) hemispheric location are plotted on the x and y axes (Supplementary Table S1). Non-significant connections (p > 0.05, one-sample t-test, FDR corrected per group) are blacked out. (B) Anatomical representation of the RSNs used in the network-based FC analysis. Annotated per image are the anatomical Bregma depths. (C) Mean network-based FC in females (top row) and males (bottom row) per treatment group. Colors indicate the strength of FC. Off-diagonal connections represent between-network FC, whereas the diagonals represent within network FC: DMLN (default mode-like network), Hipp (hippocampal network), Sens (sensory network), LCN (lateral cortical network), SubC (subcortical network). All within- and between-network FC values were significantly different from 0 as determined with a one-sample t-test (FDR corrected, Benjamini-Hochberg procedure, p < 0.05).
Next, we grouped the ROIs based on their anatomical location and known implications into five RSNs (Figure 3B) and calculated, similar to the ROI-based FC matrices, average network-based FC matrices per sex and treatment (Figure 3C). Despite the earlier mentioned lack of significance in regions associated with the SubC, all between- and within-network FC measures were significantly higher than zero within each group [p < 0.05, one-sample t-test, FDR corrected (Supplementary Tables S2, S3)].
To visualize the effects of dietary intervention on ROI-based and network-based FC, we calculated the mean difference between the treatment groups per sex, highlighting the global changes in FC as a result of dietary intervention (Figures 4A,B).
Figure 4. Mean difference in FC in male and female Ctrl, Rsv supplemented and CR rats. (A) ROI-based FC matrices displaying the mean difference in FC between ROI pairs for males (lower half) and females (upper half) between the treatment groups (Ctrl = control, CR = caloric restriction, Rsv = resveratrol). Non-significant connections (calculated on the mean FC, p > 0.05, one-sample t-test, FDR corrected per group) are blacked out. (B) Mean difference in network-based FC in females (top row) and males (bottom row) between treatment groups. In both panel A and B, positive (red-color) and negative (blue-color) values indicate, respectively, higher and lower connectivity in the first group relative to the second group. Diagonal and off-diagonal elements represent differences in within- and between-network FC respectively: DMLN (default mode-like network), Hipp (hippocampal network), Sens (sensory network), LCN (lateral cortical network), SubC (subcortical network).
To further investigate if FC is altered due to dietary intervention within- and between-RSNs, data was subjected to statistical analysis (two-way ANOVA). We observed a significant interaction effect of treatment*sex in the Hipp-SubC (p = 0.0250), SubC-DMLN (p = 0.0116) and SubC (p = 0.0215) (Figure 5A). Hipp-SubC FC was higher in female Ctrl rats when compared to male Ctrl rats (p = 0.0229), female Rsv rats (p = 0.0038) and female CR rats (p = 0.019). Similarly, in the SubC-DMLN, FC was higher in female Ctrl rats compared to male Ctrl rats (p = 0.0364), female Rsv rats (p = 0.0364) and female CR rats (p = 0.0083) and in addition we observed a difference between male CR and male Rsv rats (p = 0.0495). Finally, we also observed this interaction effect in the SubC, where FC was higher in female Ctrl rats compared to male Ctrl rats (p = 0.0225), female Rsv rats (p = 0.0225), and female CR rats (p = 0.0225).
Figure 5. Resting-state network (RSN) connectivity alterations in male and female Ctrl, Rsv supplemented and CR rats. Network-based FC demonstrating significant interaction (A) or treatment (B) effects. Bar graphs show the group-level FC within (e.g., Hipp) or between (e.g., Hipp-Sens) FC. Solid bars represent females and open bars representing the males. Group means ± SEM are presented together with the individual subject data points (dots). Significant interaction effects of treatment*sex (FDR corrected, Benjamini-Hochberg procedure, p < 0.05) are noted by the red dotted line. Significant treatment effects (post-hoc Tukey HSD, p < 0.05) are annotated by the red solid line. Asterisks indicate the levels of statistical significance: *p < 0.05, **p < 0.01, ***p < 0.001, ****p < 0.0001.
Moreover, we found a significant treatment effect demonstrating lower FC in the Rsv group, compared to the Ctrl group in the Hipp network (p = 0.0036), Hipp-DMLN (p = 0.0030), Hipp-LCN (p = 0.0474), Hipp-Sens (p = 0.0055), SubC-LCN (p = 0.0339), and SubC-Sens (p = 0.0076). Post-hoc analysis (Figure 5B) consistently revealed a lower FC in the Rsv group, when compared to the Ctrl group [Hipp network (p = 0.0025), Hipp-DMLN (p = 0.0020), Hipp-LCN (p = 0.0375), Hipp-Sens (p = 0.0043), SubC-LCN (p = 0.0266) and SubC-Sens (p = 0.0062)]—an effect we did not observe in the CR group (Supplementary Tables S4–S6).
To our knowledge, this is the first study to characterize and compare RSN FC in both male and female F344 rats subjected to short-term CR or Rsv supplementation, comparing not only the effects of CR and Rsv on RSN FC, but also highlighting their sex-specific effects. Our results demonstrated decreased BW in CR rats, as well as increased in BW in male Rsv supplemented rats, compared to female Rsv supplemented rats, whereas this difference between sexes was not observed in the Ctrl or CR groups. Furthermore, we found that both CR or Rsv supplementation induced a female-specific decrease of FC between SubC-Hipp, Sub-DMLN, and SubC. Moreover, Rsv supplementation lowered FC within the Hipp network and between Hipp-DMLN, Hipp-LCN, and Hipp-Sens, as well as between the SubC-LCN and SubC-Sens—an effect not observed for the CR rats.
The core mechanism of CR bases itself on the reduction in energy expenditure, in which the slowing of the metabolic rate is hypothesized to improve metabolic health and extend lifespan. The selective use of energy is reflected in the reduction of biomass and thus BW. As energy for activity and basic function maintenance cannot change, limited energy resources are allocated to biomass resulting in weight loss (35). This weight loss has been reported in a plethora of preclinical (36) and clinical (37, 38) studies and is in line with our own findings, as we observe a significant decrease in BW in our male and female CR rats.
Along with the loss of biomass, energy expenditure declines until eventually the energy intake matches the new lower BW. This adaptive process allows for the maintenance of important body functions including metabolic homeostasis, breathing, heart rate, and most importantly, activity of the central nervous system (37). By this principle, RSN FC should remain mostly unaltered, as energy expenditure allocated to neuronal activity is maintained throughout CR. In a mixed-sex human cohort, whole-brain RSN FC analysis has shown to not be altered as a result of dietary intervention through a hypocaloric Mediterranean diet (39). Female-only human cohorts that have been submitted to this hypocaloric Mediterranean diet or CR, revealed a decrease of FC in regions associated with food reward (40), memory consolidation (23), self-perception and emotional functions (41). These processes are known to be modulated by hormones, with fluctuations in FC coinciding with the female menstrual cycle (42–44). This sex-related decrease in FC supports our own findings, as our results similarly show lower FC in CR females when compared to Ctrl females, between the Hipp-SubC, SubC-DMLN and within the SubC, which are networks involved with learning, memory and emotion, suggesting an underlying sex-specific mechanism of CR (45) on RSN FC. However, to our knowledge, the effects of CR on FC have not been explored in male-only cohorts. Consequently, we cannot conclusively determine if these observations are indeed sex-specific. Further exploration of the sex-specific mechanisms of CR on brain activity is therefore detrimental to understand and optimize its therapeutic potential, tailoring interventions to individual needs.
CR-mimetics are compounds that are able to increase life- and/or health-span and ameliorate age-associated diseases in model organisms, in a CR-like manner (12). Rsv as a proposed CR-mimetic has been implicated with some controversy, with conflicting reports regarding the aforementioned criteria. Rsv supplementation studies in humans do not yield overwhelmingly positive data regarding aging-related benefits (46), even though Rsv has been reported to address age- and disease-related underlying mechanisms such as systemic inflammation and oxidative stress (47, 48). Studies in model organisms using CR or Rsv on age- and obesity-related biomarkers have found small, non-existent, and even opposing effects of Rsv compared to CR mechanisms (11, 49). One of the obesity-related biomarkers in which contradicting results have been reported is BW (50). A handful of preclinical trials in rats and mice supplemented daily with Rsv (5–24 mg/kg, oral, 30–45 days), have shown unaltered (51, 52) or reduced BW (53). In this study we did not observe a significant effect of Rsv supplementation (10 mg/kg, oral) on the percentage change of BW when compared to Ctrl. However, we did observe a higher percentage increase of BW for male Rsv supplemented rats relative to their starting weight, when compared to Rsv supplemented females. This difference of percentage change in BW between males and females is absent in the Ctrl and CR group. To our knowledge, there is currently no literature to support or contradict our observation of this sex-specific effect of Rsv regarding BW. Therefore, further investigation into this aspect and the effect of Rsv on BW in different sexes is warranted.
Supporting the narrative that Rsv is a CR-mimetic, we observed a female-specific decrease in the Hipp-SubC and SubC-LCN connectivity as a result of Rsv supplementation, similar to the decrease observed in female CR rats when compared to Ctrl. However, unlike CR, we observed a treatment effect of Rsv, decreasing FC within the Hipp and between the Hipp, the SubC, and other RSNs compared to Ctrl rats. Despite limited literature on the effects of Rsv on FC, published works in humans manage to directly contradict our reported lowering of FC because of Rsv supplementation. They report increased FC between the hippocampus and other regions including the right and lateral angular cortex, anterior cingulate cortex, precuneus and lateral occipital cortex, speculated to be linked to improved memory retention and attenuated hippocampal atrophy (25, 26). One possible explanation for the observed differential effects of Rsv on FC lies within the experimental design, more specifically, the dosage of Rsv. In both the mentioned human trials as well as our study, Rsv was supplemented orally daily, for the duration of a month. However, their Rsv dosage was 200 mg/kg: 20 times more compared to our daily dosage (10 mg/kg). Rsv is known to exert a hormesis dose-dependent effect (54, 55), with this effect referring to the biphasic response of a cell or organism to a compound. Often, a stimulating effect can be observed at low doses (often associated with beneficial effects), while an inhibitory effect is exerted at high doses (often toxic) (56, 57). The biphasic effect of Rsv has been shown in a plethora of (pre)clinical studies, with a multitude of them reporting differential outcomes depending on the supplied Rsv dosage (58–61). As Rsv demonstrates hormesis in various biological models, further work is required to understand its dose-relationship in context of its exerted effects, highlighting the importance of carefully considering dosage when designing studies involving Rsv supplementation and interpreting their outcomes.
The BOLD signal, used in this study as an indirect measure of neuronal activity, is based on neurovascular coupling, making it heavily dependent on changes in cerebral blood flow (CBF), cerebral blood volume (CBV) and the rate of oxygen consumption in response to changes in neuronal activity (62, 63). Unarguably, it is clear that vascular and neuronal signals both contribute to the BOLD signal (64) and while changes in BOLD are caused by neuronal activation through neurovascular coupling, they can also arise from other physiological processes that affect blood oxygenation or volume (65). CR and Rsv are both known to influence the vasculature by modulating vasodilation and CBF, through the increase of endothelial nitric oxide (NO) production (66, 67). NO plays a big role in cellular oxygen supply and demand, through regulation of the vascular tone and blood flow, as well as modulating mitochondrial oxygen consumption (68). With the increased production of endothelial NO, CR has been shown to decrease arterial blood pressure (69), improve endothelium-dependent vasodilation (70) and increase CBF (20). Similarly, Rsv supplementation leads to a more efficient endothelium-dependent vasodilation (71) and consequently, an improved CBF (72). Considering these vascular effects, it is possible they contribute to changes in the BOLD signal, which should be considered when interpreting BOLD outcomes in the context of interventions like CR and Rsv supplementation. Future work is encouraged to unravel the vascular contribution to the BOLD signal in the context of these interventions.
Sex differences in RSNs have been thoroughly investigated in humans and have shown to be confounded by environmental and sociocultural factors (73). However, sex-differences are often driven by biological factors such as hormones (e.g., estrogen) that are known to exert region-specific effects in the brain, making them potential contributors to the wide range of differences in FC observed (74, 75). Studies often show greater FC in (sub)cortical regions in females compared to males (76) and in addition, females generally show greater between-network FC whereas men have greater within-network FC (77). RsfMRI studies in rats have revealed sex-differences in brain connectivity patterns, with females exhibiting stronger hypothalamus connectivity, while males show more prominent striatum-related connectivity (78). These cumulative findings highlight the importance of taking sex into consideration as a factor when interpreting rsfMRI results in rodents, providing valuable insights into the large-scale functional organization of the brain across sexes.
As with the majority of studies, the design of the current study is subject to limitations. First, the rsfMRI scans in this study were performed in isoflurane- and medetomidine-anesthetized rats. Anesthesia indeed affect neural activity and are likely to have an impact on FC patterns as observed in rsfMRI studies. The combination of isoflurane and medetomidine specifically is known to reduce FC in the subcortical structures such as the hippocampus and (hypo)thalamus. However, studies have shown that FC under these anesthetics shows a very similar FC pattern as is observed in awake rats (79). Moreover, the combination of isoflurane and medetomidine has shown its own advantages, allowing visualization of interactions in cortical and sub-cortical structures, and the interaction between cortical, striatal and thalamic components (80, 81) and is therefore proposed as the most suitable anesthetic alternative to awake imaging for rsfMRI analysis in rats (31). Secondly, rats were supplemented Rsv daily using dried apple chips to assure oral consumption. Unfortunately, most studies have shown that the oral bioavailability of resveratrol is low (<1%) (82). Consequently, this may be the reason for discrepancies between in vivo and in vitro studies regarding Rsv efficacy and mechanisms of action (83). The limited bioavailability is often accredited to several factors including poor water solubility, limited chemical stability and high metabolism (18). However, as a polyphenol, Rsv exhibits a lipophilic nature, allowing the compound to cross the blood–brain barrier and thus reach neural tissue (84), providing the speculated beneficial effects as observed in in vivo studies. Studies have been able to detect very low Rsv concentrations in neuronal tissue (85, 86), or report that the concentration of Rsv was too low to be detected (51), despite all establishing a wide range of beneficial effects of the compound. Thus, the data suggests that even though there is limited bioavailability of the compound, it is able to exert biological effect. It is essential to consider these limitations and explore alternative delivery methods or formulations to enhance bioavailability. Additionally, further research is needed to elucidate the precise mechanisms underlying the observed effects of Rsv to validate its therapeutic potential.
Continued research into the effects of CR and Rsv on FC using advanced neuroimaging techniques like rsfMRI holds promise for advancing our understanding of dietary interventions in the promotion of brain health. Our work provides valuable insights into the effects and comparability of short-term dietary interventions using CR and Rsv supplementation on RSNs and BW in both male and female F344 rats. With this, we established a benchmark of the sex-specific impact of CR or Rsv as a dietary intervention on spontaneous brain activity, providing an FC reference for future research of dietary effects.
The raw data supporting the conclusions of this article will be made available by the authors, without undue reservation.
The animal study was approved by Committee on Animal Care and Use at the University of Antwerp, Belgium (ECD: 2021-59). The study was conducted in accordance with the local legislation and institutional requirements.
JR: Conceptualization, Data curation, Formal analysis, Investigation, Project administration, Validation, Visualization, Writing – original draft, Writing – review & editing. MB: Conceptualization, Formal analysis, Project administration, Software, Supervision, Validation, Visualization, Writing – review & editing. TV: Formal analysis, Software, Supervision, Validation, Writing – review & editing. JA: Methodology, Resources, Software, Writing – review & editing. LK: Conceptualization, Investigation, Writing – review & editing. DB: Supervision, Visualization, Writing – review & editing. MA: Methodology, Resources, Software, Supervision, Validation, Visualization, Writing – review & editing. MV: Conceptualization, Methodology, Project administration, Resources, Supervision, Validation, Writing – review & editing.
The author(s) declare that financial support was received for the research, authorship, and/or publication of this article. This study was supported by the Fund of Scientific Research Flanders (FWO-G045420N) and Stichting Alzheimer Onderzoek (SAO-FRA 2020/027, granted to Georgios A. Keliris). The computational resources and services used in this work were provided by the HPC core facility CalcUA of the University of Antwerp, the VSC (Flemish Supercomputer Center), funded by the Hercules Foundation and the Flemish Government department EWI. Funding for heavy scientific equipment was provided by the Flemish Impulse funding under grant agreement number 42/FA010100/1230 (granted to Annemie Van der Linden).
We would like to acknowledge Dr. Georgios A. Keliris for his initial contributions to the project proposal, which lead to the SAO-granted funding.
The authors declare that the research was conducted in the absence of any commercial or financial relationships that could be construed as a potential conflict of interest.
All claims expressed in this article are solely those of the authors and do not necessarily represent those of their affiliated organizations, or those of the publisher, the editors and the reviewers. Any product that may be evaluated in this article, or claim that may be made by its manufacturer, is not guaranteed or endorsed by the publisher.
The Supplementary material for this article can be found online at: https://www.frontiersin.org/articles/10.3389/fnut.2025.1440373/full#supplementary-material
1. Pressley, JC, Trott, C, Tang, M, Durkin, M, and Stern, Y. Dementia in community-dwelling elderly patients: a comparison of survey data, medicare claims, cognitive screening, reported symptoms, and activity limitations. J Clin Epidemiol. (2003) 56:896–905. doi: 10.1016/S0895-4356(03)00133-1
2. Liu, JK. Antiaging agents: safe interventions to slow aging and healthy life span extension. Nat Prod Bioprospect. (2022) 12:18. doi: 10.1007/s13659-022-00339-y
3. Fontana, L, Ghezzi, L, Cross, AH, and Piccio, L. Effects of dietary restriction on neuroinflammation in neurodegenerative diseases. J Exp Med. (2021) 218:e20190086. doi: 10.1084/jem.20190086
4. Hyun, DH, Emerson, SS, Jo, DG, Mattson, MP, and de Cabo, R. Calorie restriction up-regulates the plasma membrane redox system in brain cells and suppresses oxidative stress during aging. Proc Natl Acad Sci USA. (2006) 103:19908–12. doi: 10.1073/pnas.0608008103
5. Parikh, I, Guo, J, Chuang, KH, Zhong, Y, Rempe, RG, Hoffman, JD, et al. Caloric restriction preserves memory and reduces anxiety of aging mice with early enhancement of neurovascular functions. Aging. (2016) 8:2814–26. doi: 10.18632/aging.101094
6. Hadad, N, Unnikrishnan, A, Jackson, JA, Masser, DR, Otalora, L, Stanford, DR, et al. Caloric restriction mitigates age-associated hippocampal differential CG and non-CG methylation. Neurobiol Aging. (2018) 67:53–66. doi: 10.1016/j.neurobiolaging.2018.03.009
7. Portero-Tresserra, M, Galofré-López, N, Pallares, E, Gimenez-Montes, C, Barcia, C, Granero, R, et al. Effects of caloric restriction on spatial object recognition memory, hippocampal neuron loss and Neuroinflammation in aged rats. Nutrients. (2023) 15:1572. doi: 10.3390/nu15071572
8. Hornsby, AKE, Redhead, YT, Rees, DJ, Ratcliff, MSG, Reichenbach, A, Wells, T, et al. Short-term calorie restriction enhances adult hippocampal neurogenesis and remote fear memory in a Ghsr-dependent manner. Psychoneuroendocrinology. (2016) 63:198–207. doi: 10.1016/j.psyneuen.2015.09.023
9. Maswood, N, Young, J, Tilmont, E, Zhang, Z, Gash, DM, Gerhardt, GA, et al. Caloric restriction increases neurotrophic factor levels and attenuates neurochemical and behavioral deficits in a primate model of Parkinson's disease. Proc Natl Acad Sci USA. (2004) 101:18171–6. doi: 10.1073/pnas.0405831102
10. Martin, CK, Höchsmann, C, Dorling, JL, Bhapkar, M, Pieper, CF, Racette, SB, et al. Challenges in defining successful adherence to calorie restriction goals in humans: results from CALERIE™ 2. Exp Gerontol. (2022) 162:111757. doi: 10.1016/j.exger.2022.111757
11. Chiba, T, Tsuchiya, T, Komatsu, T, Mori, R, Hayashi, H, and Shimokawa, I. Development of calorie restriction mimetics as therapeutics for obesity, diabetes, inflammatory and neurodegenerative diseases. Curr Genomics. (2010) 11:562–7. doi: 10.2174/138920210793360934
12. Madeo, F, Carmona-Gutierrez, D, Hofer, SJ, and Kroemer, G. Caloric restriction Mimetics against age-associated disease: targets, mechanisms, and therapeutic potential. Cell Metab. (2019) 29:592–610. doi: 10.1016/j.cmet.2019.01.018
13. dos Santos, MG, da Luz, DB, de Miranda, FB, de Aguiar, RF, Siebel, AM, Arbo, BD, et al. Resveratrol and Neuroinflammation: Total-scale analysis of the scientific literature. Forum Nutr. (2024) 4:165–80. doi: 10.3390/nutraceuticals4020011
14. Omidian, M, Abdolahi, M, Daneshzad, E, Sedighiyan, M, Aghasi, M, Abdollahi, H, et al. The effects of resveratrol on oxidative stress markers: a systematic review and Meta-analysis of randomized clinical trials. Endocr Metab Immune. (2020) 20:718–27. doi: 10.2174/1871530319666191116112950
15. Lam, YY, Peterson, CM, and Ravussin, E. Resveratrol vs. calorie restriction: data from rodents to humans. Exp Gerontol. (2013) 48:1018–24. doi: 10.1016/j.exger.2013.04.005
16. Oomen, CA, Farkas, E, Roman, V, van der Beek, EM, Luiten, PG, and Meerlo, P. Resveratrol preserves cerebrovascular density and cognitive function in aging mice. Front Aging Neurosci. (2009) 1:4. doi: 10.3389/neuro.24.004.2009
17. Toth, P, Tarantini, S, Tucsek, Z, Ashpole, NM, Sosnowska, D, Gautam, T, et al. Resveratrol treatment rescues neurovascular coupling in aged mice: role of improved cerebromicrovascular endothelial function and downregulation of NADPH oxidase. Am J Physiol Heart Circ Physiol. (2014) 306:H299–308. doi: 10.1152/ajpheart.00744.2013
18. Ahmed, T, Javed, S, Javed, S, Tariq, A, Samec, D, Tejada, S, et al. Resveratrol and Alzheimer's disease: mechanistic insights. Mol Neurobiol. (2017) 54:2622–35. doi: 10.1007/s12035-016-9839-9
19. Dos Santos, MG, Schimith, LE, Andre-Miral, C, Muccillo-Baisch, AL, Arbo, BD, and Hort, MA. Neuroprotective effects of resveratrol in in vivo and in vitro experimental models of Parkinson's disease: a systematic review. Neurotox Res. (2022) 40:319–45. doi: 10.1007/s12640-021-00450-x
20. Lin, AL, Parikh, I, Hoffman, JD, and Ma, D. Neuroimaging biomarkers of caloric restriction on Brain metabolic and vascular functions. Curr Nutr Rep. (2017) 6:41–8. doi: 10.1007/s13668-017-0187-9
21. Bajic, D, Craig, MM, Mongerson, CRL, Borsook, D, and Becerra, L. Identifying rodent resting-state Brain networks with independent component analysis. Front Neurosci. (2017) 11:685. doi: 10.3389/fnins.2017.00685
22. Fox, MD, Snyder, AZ, Vincent, JL, Corbetta, M, Van Essen, DC, and Raichle, ME. The human brain is intrinsically organized into dynamic, anticorrelated functional networks. Proc Natl Acad Sci USA. (2005) 102:9673–8. doi: 10.1073/pnas.0504136102
23. Prehn, K, Jumpertz von Schwartzenberg, R, Mai, K, Zeitz, U, Witte, AV, Hampel, D, et al. Caloric restriction in older adults-differential effects of weight loss and reduced weight on Brain structure and function. Cereb Cortex. (2017) 27:1765–78. doi: 10.1093/cercor/bhw008
24. Huhn, S, Beyer, F, Zhang, R, Lampe, L, Grothe, J, Kratzsch, J, et al. Effects of resveratrol on memory performance, hippocampus connectivity and microstructure in older adults - a randomized controlled trial. NeuroImage. (2018) 174:177–90. doi: 10.1016/j.neuroimage.2018.03.023
25. Witte, AV, Kerti, L, Margulies, DS, and Floel, A. Effects of resveratrol on memory performance, hippocampal functional connectivity, and glucose metabolism in healthy older adults. J Neurosci. (2014) 34:7862–70. doi: 10.1523/JNEUROSCI.0385-14.2014
26. Kobe, T, Witte, AV, Schnelle, A, Tesky, VA, Pantel, J, Schuchardt, JP, et al. Impact of resveratrol on glucose control, hippocampal structure and connectivity, and memory performance in patients with mild cognitive impairment. Front Neurosci. (2017) 11:105. doi: 10.3389/fnins.2017.00105
27. Juybari, KB, Sepehri, G, Meymandi, MS, Vakili Shahrbabaki, SS, Moslemizadeh, A, Saeedi, N, et al. Sex dependent alterations of resveratrol on social behaviors and nociceptive reactivity in VPA-induced autistic-like model in rats. Neurotoxicol Teratol. (2020) 81:106905. doi: 10.1016/j.ntt.2020.106905
28. Baer, SB, Dorn, AD, and Osborne, DM. Sex differences in response to obesity and caloric restriction on cognition and hippocampal measures of autophagic-lysosomal transcripts and signaling pathways. BMC Neuroscience. (2024) 25:1. doi: 10.1186/s12868-023-00840-1
29. Mirmiran, P, Bahadoran, Z, and Gaeini, Z. Common limitations and challenges of dietary clinical trials for translation into clinical practices. Int J Endocrinol Metab. (2021) 19:e108170. doi: 10.5812/ijem.108170
30. Xu, N, LaGrow, TJ, Anumba, N, Lee, A, Zhang, X, Yousefi, B, et al. Functional connectivity of the Brain across rodents and humans. Front Neurosci. (2022) 16:816331. doi: 10.3389/fnins.2022.816331
31. Grandjean, J, Desrosiers-Gregoire, G, Anckaerts, C, Angeles-Valdez, D, Ayad, F, Barriere, DA, et al. A consensus protocol for functional connectivity analysis in the rat brain. Nat Neurosci. (2023) 26:673–81. doi: 10.1038/s41593-023-01286-8
32. van den Berg, M, Adhikari, MH, Verschuuren, M, Pintelon, I, Vasilkovska, T, Van Audekerke, J, et al. Altered basal forebrain function during whole-brain network activity at pre- and early-plaque stages of Alzheimer's disease in TgF344-AD rats. Alzheimers Res Ther. (2022) 14:148. doi: 10.1186/s13195-022-01089-2
33. Vasilkovska, T, Adhikari, MH, Van Audekerke, J, Salajeghe, S, Pustina, D, Cachope, R, et al. Resting-state fMRI reveals longitudinal alterations in brain network connectivity in the zQ175DN mouse model of Huntington's disease. Neurobiol Dis. (2023) 181:106095. doi: 10.1016/j.nbd.2023.106095
34. De Waegenaere, S, van den Berg, M, Keliris, GA, Adhikari, MH, and Verhoye, M. Early altered directionality of resting brain network state transitions in the TgF344-AD rat model of Alzheimer's disease. Front Hum Neurosci. (2024) 18:1379923. doi: 10.3389/fnhum.2024.1379923
35. Hou, C, Bolt, KM, and Bergman, A. A general model for ontogenetic growth under food restriction. Proc R Soc B Biol Sci. (2011) 278:2881–90. doi: 10.1098/rspb.2011.0047
36. Taormina, G, and Mirisola, MG. Calorie restriction in mammals and simple model organisms. Biomed Res Int. (2014) 2014:308690:1–10. doi: 10.1155/2014/308690
37. Most, J, and Redman, LM. Impact of calorie restriction on energy metabolism in humans. Exp Gerontol. (2020) 133:110875. doi: 10.1016/j.exger.2020.110875
38. Trepanowski, JF, Canale, RE, Marshall, KE, Kabir, MM, and Bloomer, RJ. Impact of caloric and dietary restriction regimens on markers of health and longevity in humans and animals: a summary of available findings. Nutr J. (2011) 10:107. doi: 10.1186/1475-2891-10-107
39. Gaynor, AM, Varangis, E, Song, S, Gazes, Y, Noofoory, D, Babukutty, RS, et al. Diet moderates the effect of resting state functional connectivity on cognitive function. Sci Rep. (2022) 12:16080. doi: 10.1038/s41598-022-20047-4
40. Jakobsdottir, S, van Nieuwpoort, IC, van Bunderen, CC, de Ruiter, MB, Twisk, JW, Deijen, JB, et al. Acute and short-term effects of caloric restriction on metabolic profile and brain activation in obese, postmenopausal women. Int J Obes. (2016) 40:1671–8. doi: 10.1038/ijo.2016.103
41. Garcia-Casares, N, Bernal-Lopez, MR, Roe-Vellve, N, Gutierrez-Bedmar, M, Fernandez-Garcia, JC, Garcia-Arnes, JA, et al. Brain functional connectivity is modified by a hypocaloric Mediterranean diet and physical activity in obese women. Nutrients. (2017) 9:685. doi: 10.3390/nu9070685
42. Lisofsky, N, Martensson, J, Eckert, A, Lindenberger, U, Gallinat, J, and Kuhn, S. Hippocampal volume and functional connectivity changes during the female menstrual cycle. NeuroImage. (2015) 118:154–62. doi: 10.1016/j.neuroimage.2015.06.012
43. Hidalgo-Lopez, E, Mueller, K, Harris, T, Aichhorn, M, Sacher, J, and Pletzer, B. Human menstrual cycle variation in subcortical functional brain connectivity: a multimodal analysis approach. Brain Struct Funct. (2020) 225:591–605. doi: 10.1007/s00429-019-02019-z
44. Hjelmervik, H, Hausmann, M, Osnes, B, Westerhausen, R, and Specht, K. Resting states are resting traits - an fMRI study of sex differences and menstrual cycle effects in resting state cognitive control networks. PLoS One. (2014) 9:e103492. doi: 10.1371/journal.pone.0103492
45. Suchacki, KJ, Thomas, BJ, Ikushima, YM, Chen, KC, Fyfe, C, Tavares, AAS, et al. The effects of caloric restriction on adipose tissue and metabolic health are sex- and age-dependent. eLife. (2023) 12:12. doi: 10.7554/eLife.88080
46. Bhullar, KS, and Hubbard, BP. Lifespan and healthspan extension by resveratrol. Biochim Biophys Acta. (2015) 1852:1209–18. doi: 10.1016/j.bbadis.2015.01.012
47. Ramirez-Garza, SL, Laveriano-Santos, EP, Marhuenda-Munoz, M, Storniolo, CE, Tresserra-Rimbau, A, Vallverdu-Queralt, A, et al. Health effects of resveratrol: results from human intervention trials. Nutrients. (2018) 10:1892. doi: 10.3390/nu10121892
48. Chung, JH, Manganiello, V, and Dyck, JRB. Resveratrol as a calorie restriction mimetic: therapeutic implications. Trends Cell Biol. (2012) 22:546–54. doi: 10.1016/j.tcb.2012.07.004
49. Pallauf, K, Günther, I, Kühn, G, Chin, D, de Pascual-Teresa, S, and Rimbach, G. The potential of resveratrol to act as a caloric restriction mimetic appears to be limited: insights from studies in mice. Adv Nutr. (2021) 12:995–1005. doi: 10.1093/advances/nmaa148
50. Hillsley, A, Chin, V, Li, A, and McLachlan, CS. Resveratrol for weight loss in obesity: an assessment of randomized control trial designs in ClinicalTrials.gov. Nutrients. (2022) 14:1424. doi: 10.3390/nu14071424
51. Karuppagounder, SS, Pinto, JT, Xu, H, Chen, HL, Beal, MF, and Gibson, GE. Dietary supplementation with resveratrol reduces plaque pathology in a transgenic model of Alzheimer's disease. Neurochem Int. (2009) 54:111–8. doi: 10.1016/j.neuint.2008.10.008
52. Raskovic, A, Cucuz, V, Torovic, L, Tomas, A, Gojkovic-Bukarica, L, Cebovic, T, et al. Resveratrol supplementation improves metabolic control in rats with induced hyperlipidemia and type 2 diabetes. Saudi Pharm J. (2019) 27:1036–43. doi: 10.1016/j.jsps.2019.08.006
53. Sharma, R, Sharma, NK, and Thungapathra, M. Resveratrol regulates body weight in healthy and ovariectomized rats. Nutr Metab. (2017) 14:30. doi: 10.1186/s12986-017-0183-5
54. Calabrese, EJ, Mattson, MP, and Calabrese, V. Resveratrol commonly displays hormesis: occurrence and biomedical significance. Hum Exp Toxicol. (2010) 29:980–1015. doi: 10.1177/0960327110383625
55. Shaito, A, Posadino, AM, Younes, N, Hasan, H, Halabi, S, Alhababi, D, et al. Potential adverse effects of resveratrol: a literature review. Int J Mol Sci. (2020) 21:2084. doi: 10.3390/ijms21062084
57. Calabrese, EJ, and Baldwin, LA. Hormesis: the dose-response revolution. Annu Rev Pharmacol Toxicol. (2003) 43:175–97. doi: 10.1146/annurev.pharmtox.43.100901.140223
58. Plauth, A, Geikowski, A, Cichon, S, Wowro, SJ, Liedgens, L, Rousseau, M, et al. Hormetic shifting of redox environment by pro-oxidative resveratrol protects cells against stress. Free Radic Biol Med. (2016) 99:608–22. doi: 10.1016/j.freeradbiomed.2016.08.006
59. Juhasz, B, Mukherjee, S, and Das, DK. Hormetic response of resveratrol against cardioprotection. Exp Clin Cardiol. (2010) 15:e134–8.
60. Dey, A, Guha, P, Chattopadhyay, S, and Bandyopadhyay, SK. Biphasic activity of resveratrol on indomethacin-induced gastric ulcers. Biochem Biophys Res Commun. (2009) 381:90–5. doi: 10.1016/j.bbrc.2009.02.027
61. Posadino, AM, Giordo, R, Cossu, A, Nasrallah, GK, Shaito, A, Abou-Saleh, H, et al. Flavin oxidase-induced ROS generation modulates PKC biphasic effect of resveratrol on endothelial cell survival. Biomol Ther. (2019) 9:209. doi: 10.3390/biom9060209
62. Drew, PJ. Vascular and neural basis of the BOLD signal. Curr Opin Neurobiol. (2019) 58:61–9. doi: 10.1016/j.conb.2019.06.004
63. Hillman, EMC. Coupling mechanism and significance of the BOLD signal: a status report. Annu Rev Neurosci. (2014) 37:161–81. doi: 10.1146/annurev-neuro-071013-014111
64. Tsvetanov, KA, Henson, RNA, and Rowe, JB. Separating vascular and neuronal effects of age on fMRI BOLD signals. Philos Trans R Soc Lond B Biol Sci. (2021) 376:20190631. doi: 10.1098/rstb.2019.0631
65. Tong, Y, Hocke, LM, Fan, X, Janes, AC, and Frederick, B. Can apparent resting state connectivity arise from systemic fluctuations? Front Hum Neurosci. (2015) 9:285. doi: 10.3389/fnhum.2015.00285
66. Al Attar, AA, Fahed, GI, Hoballah, MM, Pedersen, S, El-Yazbi, AF, Nasser, SA, et al. Mechanisms underlying the effects of caloric restriction on hypertension. Biochem Pharmacol. (2022) 200:115035. doi: 10.1016/j.bcp.2022.115035
67. Xia, N, Forstermann, U, and Li, HG. Resveratrol and endothelial nitric oxide. Molecules. (2014) 19:16102–21. doi: 10.3390/molecules191016102
68. Tejero, J, Shiva, S, and Gladwin, MT. Sources of vascular nitric oxide and reactive oxygen species and their regulation. Physiol Rev. (2019) 99:311–79. doi: 10.1152/physrev.00036.2017
69. Kord-Varkaneh, H, Nazary-Vannani, A, Mokhtari, Z, Salehi-sahlabadi, A, Rahmani, J, Clark, CCT, et al. The influence of fasting and energy restricting diets on blood pressure in humans: a systematic review and Meta-analysis. High Blood Press Cardiovasc Prev. (2020) 27:271–80. doi: 10.1007/s40292-020-00391-0
70. Mattagajasingh, I, Kim, CS, Naqvi, A, Yamamori, T, Hoffman, TA, Jung, SB, et al. SIRT1 promotes endothelium-dependent vascular relaxation by activating endothelial nitric oxide synthase. Proc Natl Acad Sci USA. (2007) 104:14855–60. doi: 10.1073/pnas.0704329104
71. Breuss, JM, Atanasov, AG, and Uhrin, P. Resveratrol and its effects on the vascular system. Int J Mol Sci. (2019) 20:1523. doi: 10.3390/ijms20071523
72. Kennedy, DO, Wightman, EL, Reay, JL, Lietz, G, Okello, EJ, Wilde, A, et al. Effects of resveratrol on cerebral blood flow variables and cognitive performance in humans: a double-blind, placebo-controlled, crossover investigation. Am J Clin Nutr. (2010) 91:1590–7. doi: 10.3945/ajcn.2009.28641
73. Becker, JB, McClellan, ML, and Reed, BG. Sex differences, gender and addiction. J Neurosci Res. (2017) 95:136–47. doi: 10.1002/jnr.23963
74. Biswal, BB, Mennes, M, Zuo, XN, Gohel, S, Kelly, C, Smith, SM, et al. Toward discovery science of human brain function. Proc Natl Acad Sci USA. (2010) 107:4734–9. doi: 10.1073/pnas.0911855107
75. Alfano, V, Cavaliere, C, Di Cecca, A, Ciccarelli, G, Salvatore, M, Aiello, M, et al. Sex differences in functional brain networks involved in interoception: an fMRI study. Front Neurosci. (2023) 17:1130025. doi: 10.3389/fnins.2023.1130025
76. Gong, G, Rosa-Neto, P, Carbonell, F, Chen, ZJ, He, Y, and Evans, AC. Age- and gender-related differences in the cortical anatomical network. J Neurosci. (2009) 29:15684–93. doi: 10.1523/JNEUROSCI.2308-09.2009
77. Chung, YS, Calhoun, V, and Stevens, MC. Adolescent sex differences in cortico-subcortical functional connectivity during response inhibition. Cogn Affect Behav Neurosci. (2020) 20:1–18. doi: 10.3758/s13415-019-00718-y
78. Li, Q, and Zhang, N. Sex differences in resting-state functional networks in awake rats. Brain Struct Funct. (2023) 228:1411–23. doi: 10.1007/s00429-023-02657-4
79. Paasonen, J, Stenroos, P, Salo, RA, Kiviniemi, V, and Grohn, O. Functional connectivity under six anesthesia protocols and the awake condition in rat brain. NeuroImage. (2018) 172:9–20. doi: 10.1016/j.neuroimage.2018.01.014
80. Grandjean, J, Schroeter, A, Batata, I, and Rudin, M. Optimization of anesthesia protocol for resting-state fMRI in mice based on differential effects of anesthetics on functional connectivity patterns. NeuroImage. (2014) 102:838–47. doi: 10.1016/j.neuroimage.2014.08.043
81. Bukhari, Q, Schroeter, A, Cole, DM, and Rudin, M. Resting state fMRI in mice reveals anesthesia specific signatures of Brain functional networks and their interactions. Front Neural Circuits. (2017) 11:11. doi: 10.3389/fncir.2017.00005
82. Walle, T. Bioavailability of resveratrol. Ann N Y Acad Sci. (2011) 1215:9–15. doi: 10.1111/j.1749-6632.2010.05842.x
83. Kulkarni, SS, and Canto, C. The molecular targets of resveratrol. Biochim Biophys Acta. (2015) 1852:1114–23. doi: 10.1016/j.bbadis.2014.10.005
84. Figueira, I, Garcia, G, Pimpao, RC, Terrasso, AP, Costa, I, Almeida, AF, et al. Polyphenols journey through blood-brain barrier towards neuronal protection. Sci Rep. (2021) 11:11456. doi: 10.1038/s41598-021-96179-w
85. Asensi, M, Medina, I, Ortega, A, Carretero, J, Baño, MC, Obrador, E, et al. Inhibition of cancer growth by resveratrol is related to its low bioavailability. Free Radical Biol Med. (2002) 33:387–98. doi: 10.1016/S0891-5849(02)00911-5
Keywords: caloric restriction, resveratrol, resting-state functional MRI, resting-state networks, functional connectivity
Citation: van Rooij JRA, van den Berg M, Vasilkovska T, Van Audekerke J, Kosten L, Bertoglio D, Adhikari MH and Verhoye M (2025) Short-term caloric restriction or resveratrol supplementation alters large-scale brain network connectivity in male and female rats. Front. Nutr. 12:1440373. doi: 10.3389/fnut.2025.1440373
Received: 29 May 2024; Accepted: 13 January 2025;
Published: 03 February 2025.
Edited by:
Rubem C. A. Guedes, Federal University of Pernambuco, BrazilReviewed by:
Qixiang Lin, Emory University, United StatesCopyright © 2025 van Rooij, van den Berg, Vasilkovska, Van Audekerke, Kosten, Bertoglio, Adhikari and Verhoye. This is an open-access article distributed under the terms of the Creative Commons Attribution License (CC BY). The use, distribution or reproduction in other forums is permitted, provided the original author(s) and the copyright owner(s) are credited and that the original publication in this journal is cited, in accordance with accepted academic practice. No use, distribution or reproduction is permitted which does not comply with these terms.
*Correspondence: Judith R. A. van Rooij, anVkaXRoLnZhbnJvb2lqQHVhbnR3ZXJwZW4uYmU=; Marleen Verhoye, bWFybGVlbi52ZXJob3llQHVhbnR3ZXJwZW4uYmU=
Disclaimer: All claims expressed in this article are solely those of the authors and do not necessarily represent those of their affiliated organizations, or those of the publisher, the editors and the reviewers. Any product that may be evaluated in this article or claim that may be made by its manufacturer is not guaranteed or endorsed by the publisher.
Research integrity at Frontiers
Learn more about the work of our research integrity team to safeguard the quality of each article we publish.