- 1Key Laboratory of Prevention and Treatment of Cardiovascular and Cerebrovascular Diseases, Ministry of Education, Gannan Medical University, Ganzhou, China
- 2School of Public Health and Health Management, Gannan Medical University, Ganzhou, China
- 3State Center of Quality Testing and Inspection for Camellia Products, Ganzhou, China
- 4Key Laboratory of Development and Utilization of Gannan Characteristic Food Function Component of Ganzhou, Gannan Medical University, Ganzhou, China
Edible plant oils are widely used in cooking, cosmetics, health supplement capsules, and other industries, due to their various health-promoting effects. There is increasing evidence that edible plant oils can modulate gut microbiota during their health-promoting effects in animal experiments and cohort or clinical studies. However, the information concerning the gut microbiota modulation of edible plant oils during their health-promoting effects is scattered. In this article, the research progress on gut microbiota modulation of edible plant oils (especially camellia oil, olive oil, and flaxseed oil) is summarized. Meanwhile, a summary on correlations between modulated gut microbiota and changed biochemical indexes is provided. The alterations of edible plant oils on gut microbiota-derived metabolites and the correlations between altered metabolites and modulated gut microbiota as well as changed biochemical indexes are reviewed. Furthermore, the prospects for gut microbiota modulation of edible plant oils during their health-promoting effects are put forward. Existing literature has shown that edible plant oils could modulate gut microbiota during their health-promoting effects, and some differential gut microbiota biomarkers were gained. Some similarities and differences existed while the oils exhibited health-promoting actions. Dosage and treatment time have influences on gut microbiota modulation of edible plant oils. Different edible plant oils exhibited different behaviors in modulating gut microbiota, and edible plant oils were mostly different in modulating gut microbiota compared to edible animal oils. Moreover, the modulated gut microbiota was significantly correlated with the changed biochemical indexes. Furthermore, edible plant oils altered SCFAs and other gut microbiota-derived metabolites. The altered metabolites were obviously correlated with the modulated gut microbiota and changed biochemical indexes. This review is helpful to the future research and application of edible plant oils in health-promoting effects from the perspective of gut microbiota.
1 Introduction
Edible plant oils, obtained from the seeds, pulps, fruits, or plumules of certain plants, are an important source of dietary fat and represent as much as 25% of human caloric intake in developed countries (1). They are widely used in cooking, food, pharmaceutical, cosmetics, and other industries (2). With the increase in the world population, the demand for edible oils is increasing, showing the annual growth rate of global demand for edible plant oils as 5.14% from 2020 to 2025 (3). In 2022, the global consumption of edible plant oils was 212.82 million tons, showing a 1.25-fold increase compared to 2014 (4). A more important reason behind this was the health-promoting effects of them, due to their special fatty acid composition and abundant bioactive components.
Compared with most animal oils, edible plant oils are rich in unsaturated fatty acids and lacking cholesterol. Moreover, there are a diversity of bioactive compounds in edible plant oils, such as sterol, squalene, polyphenols, and tocopherols. As research continues, more attention has been increasingly paid to the edible plant oils’ health-promoting effects, including antioxidant (5), anti-inflammatory (6), regulation of lipid metabolism (7), anti-Alzheimer’s disease (8), and anti-cancer (9) activities, as well as other activities. For example, camellia oil has been reported to possess antioxidant, anti-cancer, anti-inflammatory, antibacterial, antihypertensive, hypoglycemic, cardioprotective, and immunoregulatory activities (10). Olive oil has been reviewed to have anti-inflammatory, chemopreventive, antimicrobial, hepatoprotective, kidney protection, and anti-neurodegenerative effects (11). Flaxseed oil has been summarized to have antioxidant, anti-inflammatory, anti-obesity, and bone osteoporosis improvement actions (12). Coconut oil has been reported to possess hypocholesterolemic, anti-cancer, antihepatosteatotic, antidiabetic, antioxidant, anti-inflammatory, antimicrobial, and skin moisturizing properties (13).
The trillions of microorganisms in the human intestine are important regulators of health (14). Gut microbiota is considered as an environmental factor that interacts with diet and may also have an impact on health outcomes, many of which involve metabolites produced by the microbiota from dietary components that can impact the host (15). In recent years, a lot of edible plant oils have been demonstrated to modulate gut microbiota during their health-promoting effects in numerous animal experiments (16–18) and cohort or clinical studies (19–21). For instance, camellia oil has been demonstrated to exhibit anti-fatigue properties by modulating the gut microbial composition of mice, which were received with Rotarod test and Treadmill test (16). Olive oil-enriched diet has been indicated to increase the abundance of lactic acid bacteria in overweight/obese subjects (19). Perilla oil has been found to relieve constipation and enhance diversity of gut microbiota in sedentary healthy female (21). Among them, camellia oil, olive oil, and flaxseed oil are three of the common edible plant oils on the market. Especially, these three oils have been extensively reported to modulate gut microbiota during their health-promoting effects.
Currently, the research progress on health-promoting effects of edible plant oils has been summarized in some literature (10–12, 22). Moreover, there were several reviews that partly summarized some findings about the modulations of edible plant oils (such as olive oil, flaxseed oil, safflower oil, and palm oil) on gut microbiota during their health-promoting effects (23–25). However, the information concerning the gut microbiota modulation of edible plant oils during their health-promoting effects is still scattered. It is necessary to conduct a comprehensive review on this aspect for better understanding of the health-promoting effects of edible plant oils from the perspective of gut microbiota.
Herein, the research progress on gut microbiota modulation of edible plant oils (especially camellia oil, olive oil, and flaxseed oil) is reviewed. Meanwhile, the correlations between modulated gut microbiota and changed biochemical indexes are summarized. The alterations of edible plant oils on gut microbiota-derived metabolites and the correlations between altered metabolites and modulated gut microbiota as well as changed biochemical indexes are reviewed. Furthermore, the prospects for gut microbiota modulation of edible plant oils in exhibiting health-promoting effects are put forward.
2 Overview of chemical composition in edible plant oils
Camellia oil is extracted from the seeds of Camellia oleifera Abel. The unsaturated fatty acid content of camellia oil is as high as 85–97%, consisting mainly of oleic (71.42–90%) and linoleic (7–14%) acids. And the saturated fatty acid content is usually approximately 10–13%, consisting mainly of palmitic acid (7–9%), stearic acid (1–3%), and a small amount of palmitoleic acid (26). Meanwhile, it also contains a multitude of bioactive components, such as sterol (2860.18–4748.39 mg/kg), squalene (122.02–248.24 mg/kg), polyphenols (20.56–88.56 mg/kg), sasanquasaponin (38.5 mg/kg), tocopherols (α-tocopherol, 153–771 mg/kg; γ-tocopherol, 9.4–59 mg/kg; δ-tocopherol, 0.27–28 mg/kg) and other functional substances (26, 27).
Olive oil is obtained from the fruit of Olea europaea L. It is composed of ~98–99% of fatty acids, mainly triacylglycerol esters of oleic acid (55–83%), palmitic acid (7.5–20%), linoleic acid (3.5–21%), and other fatty acids such as stearic acid (0.5–5%) (28). The unsaponifiable fraction of olive oil includes triterpenic dialcohols and acids (20–200 mg/kg), sterols (1000–5,000 mg/kg), squalene (1,000–8,000 mg/kg), pigments (5–30 mg/kg), and phenolic compounds (50–1,000 mg/kg) (9, 29).
Flaxseed oil is gained from the seeds of Linum usitatissimum L. It has high unsaturated fatty acids (>70%), mainly composed of linolenic acid (53.36–65.84%), linoleic acid (10.14–16.39%), oleic acid (10.03–12.37%), stearic acid (3.98–9.85%), and palmitic acid (2.41–7.97%) (12, 30). In addition, it contains many bioactive compounds, including sterols (0.25–0.3%), polyphenols (15.69–47.68 mg/kg), cyclic polypeptides (188.6–643.8 mg/kg), tocopherol (374–563.7 mg/kg), lignans (0–32, 28 mg/kg), chlorophyll (1.45–2.08 mg/kg), carotenoid (2.89–3.45 mg/kg), and other compounds (12).
Other edible plant oils are also rich in unsaturated fatty acids, mainly composed of oleic acid (soybean oil, 15–36%; peony seed oil, 20.5–45.1%; walnut oil, 10–20%; etc.), linoleic acids (soybean oil, 42.8–56.1%; peony seed oil, 16.5–33.6%; walnut oil, 55–70%; etc.) and linolenic acids (soybean oil, 2–14%; peony seed oil, 28.1–46.9%; walnut oil, 10–18%; etc.) (2). Meanwhile, other edible plant oils contain many bioactive ingredients, including phytosterols (safflower oil, 243.7 mg/100 g; peony seed oil, 154.5 mg/100 g; walnut oil, 115.7 mg/100 g; etc.), phenolic compounds (safflower oil, 231.40 mg/kg; Torreya grandis seed oil, 12,630 mg/kg; almond oil, 644.54 mg/kg; etc.), tocopherol (sea buckthorn seed oil, 898.1 mg/kg; tomato seed oil, 345.8 mg/kg; rice bran oil, 322.7 mg/kg; etc.), squalene (walnut oil, 12 mg/100 g; peony seed oil, 4 mg/100 g; rice bran oil, 24 mg/100 g; etc.), and β-carotene (tomato seed oil, 765.7 mg/kg; sea buckthorn seed oil, 55.3 mg/kg; corn oil, 0.07 mg/kg; etc.) (22).
3 Gut microbiota modulation of edible plant oils
3.1 Camellia oil modulation
Camellia oil has been demonstrated to modulate gut microbiota during its health-promoting effects (8, 16, 31–42), as shown in Table 1 and Figure 1. In terms of anti-ulcerative colitis effect, supplementation of camellia oil increased the abundances of Firmicutes and/or Firmicutes/Bacteroidetes ratio, and/or decreased that of Bacteroidetes in acetic acid and dextran sulfate sodium-induced rats or mice, at phylum level (32, 33). And, at the genus level, camellia oil upregulated the amounts of Bacteroides and Lactobacillus and downregulated those of Alistipes and Lachnospiraceae NK4A136 group in dextran sulfate sodium-induced colitis mice (33). Moreover, Actinobacteria, Bacteroidetes, Firmicutes, and Proteobacteria were differential gut microbiota biomarkers for camellia oil in anti-ulcerative colitis against acetic acid-induced colitis rats (32).
Regarding anti-Alzheimer’s disease action, camellia oil added Lactobacillales and reduced Enterobacteriaceae, with Lactobacillus as the differential gut microbiota biomarker, toward aluminum chloride-induced rats (34). At the phylum level, camellia oil enhanced Bacteroidetes and Proteobacteria and lowered Firmicutes (36). At genus level, camellia oil elevated the abundances of Bacteroides pectinophilus group, Eubacterium xylanophilum group, Intestinimonas, [Eubacterium]_coprostanoligenes_group and Lactobacillus_johnsonii, and decreased those of Lachnospira, Ruminiclostridium, Lachnospiraceae_ND3007_group and Bacteroides (8, 36). On the other hand, camellia oil intervention upregulated Bacteroidetes and downregulated Firmicutes while exerted anti-Alzheimer’s disease action on Aβ25–35-induced mice (35).
To anti-obese activity, camellia oil enhanced Bacteroidetes and reduced Firmicutes and Firmicutes/Bacteroidetes ratio in high-fat diet-induced mice, at the phylum level (37, 38). At the genus level, camellia oil increased the abundances of six bacteria (Lactobacillus, Bacteroides, Alloprevotella, Alistipes, Blautia, etc.), and decreased those of six bacteria (Allobaculum, Lachnospiraceae NK4A136 group, Helicobacter, Lactobacillus, Bacteroides, etc.) in high-fat diet-induced mice (37, 38). Moreover, 12 types of bacteria (Actinobacteria, Lactobacillaceae, Coriobacteriaceae, Bacilli, Coriobacteriia, etc.) were differential gut microbiota biomarkers for camellia oil that exerted anti-obese effect against high-fat diet-induced mice (37).
Other effects included anti-atherosclerosis (40), gastroprotection (41), hepatoprotection (42), anti-fatigue (16), and hypolipidemic effects (39). Camellia oil upregulated the abundances of Bacteroidetes, Tenericutes, Alloprevotella, and/or Desulfobacterota, and downregulated those of Firmicutes, Actinobacteria, Verrucomicrobia, Turicibacter, and Proteobacteria along with Firmicutes/Bacteroidetes ratio at phylum level, in high-fat diet or ethanol-induced ApoE−/− mice and/or normal mice. At the genus level, camellia oil enhanced the abundances of nine bacteria (Bacteroides, Alistipes, Alloprevotella, Lactobacillus, g_UCG-010, etc.) and lowered those of seven bacteria (Dubosiella, Faecalibaculum, Coriobacteriaceae UCG-002, Lactobacillus, Turicibacter, etc.) (16, 39–42). Moreover, Dorea, Olsenella, and Bosea were identified to be differential gut microbiota biomarkers for camellia oil that exhibited gastroprotection on ethanol-induced mice (41). Six bacteria (Faecalibaculum, Defluviitaleaceae UCG, Streptococcus, Enterorhabdus, Bilophila, etc.) were differential gut microbiota biomarkers for camellia oil exerted anti-atherosclerosis effect against high-fat diet-induced ApoE−/− mice (40).
Some differences and similarities in gut microbiota modulation could be found while camellia oil exhibited health-promoting effects in animal experiments. In terms of the similarities, at the phylum level, camellia oil treatment mostly caused increment in Bacteroidetes (16, 35–39, 41, 42) and reduction in Firmicutes (16, 35–39, 42). These led to the decrease of Firmicutes/Bacteroidetes ratio (37–40). Meanwhile, the abundance of Actinobacteria was lowered while camellia oil exerted gastroprotection (41) and anti-fatigue (16) effects. At the genus level, the amount of Bacteroides was raised as camellia oil exhibiting anti-ulcerative colitis (33), gastroprotection (41), hepatoprotection (42), and anti-obese (37) effects. The abundance of Alloprevotella was elevated, while camellia oil showed anti-fatigue (16), anti-atherosclerosis (40), hepatoprotection (42), and anti-obese (37, 38) activities. That of Alistipes was enhanced as camellia oil displayed anti-fatigue (16), anti-obese (38), and hypolipidemic (39) effects. On the contrary, the abundance of Dubosiella was decreased as camellia oil revealed anti-fatigue (16) and anti-atherosclerosis (40) activities. That of Lachnospiraceae NK4A136 group was reduced, while camellia oil showed anti-ulcerative colitis (33) and anti-obese (37) actions. The amount of Lactobacillus was downregulated while camellia oil generated anti-atherosclerosis and anti-obese effects (37, 38).
Regarding the differences, at the phylum level, the abundance of Firmicutes was increased and/or that of Bacteroidetes was decreased, while camellia oil exerted anti-ulcerative colitis action (32, 33). Whereas, opposite phenomena were observed as camellia oil showed gastroprotection (41), anti-fatigue (16), anti-atherosclerosis (40), anti-Alzheimer’s disease (35, 36), hepatoprotection (42), anti-obese (37, 38), and hypolipidemic effects (39). Correspondingly, the Firmicutes/Bacteroidetes ratio was enlarged while camellia oil exerted anti-ulcerative colitis action (33), while it was shrunk as the oil revealed anti-atherosclerosis (40) and anti-obese (37, 38) effects. Meanwhile, the amount of Proteobacteria was added and reduced while camellia oil exhibited anti-Alzheimer’s disease action (36) and hypolipidemic effect (39), respectively. At the genus level, the abundance of Bacteroides was decreased while camellia oil showed anti-Alzheimer’s disease (8) and anti-obese (38) effects, whereas it was increased as this oil displayed anti-ulcerative colitis (33), gastroprotection (41), hepatoprotection (42) and anti-obese (37) activities. Meanwhile, the abundance of Lactobacillus was upregulated while camellia oil displayed anti-ulcerative colitis (33), anti-fatigue (16), and hypolipidemic (39) effects, and it was downregulated as camellia oil exerted anti-atherosclerosis action (40). That of Lachnospiraceae NK4A136 group was decreased while camellia oil exhibited anti-ulcerative colitis (33) and anti-obese (37) activities, and it was increased as the oil showed anti-atherosclerosis action (40). The amount of Alistipes was reduced while camellia oil revealed anti-ulcerative colitis effect (33), while it was enhanced as this oil exerted anti-fatigue (16), anti-obese (38), and hypolipidemic (39) effects. The abundance of Dubosiella was decreased while camellia oil showed anti-fatigue (16) and anti-atherosclerosis (40) activities, whereas it was increased as the oil exerted hypolipidemic effect (39).
Overall, camellia oil could modulate gut microbiota during their many health-promoting effects. Moreover, differential gut microbiota biomarkers have been screened for it and showed anti-ulcerative colitis, anti-Alzheimer’s disease, anti-obese, and gastroprotection activities. Furthermore, some differences and similarities in gut microbiota modulation have been found while this oil exhibited different health-promoting effects.
3.2 Olive oil modulation
Olive oil has been proven to modulate gut microbiota during its health-promoting effects (19, 43–50), as illustrated in Table 2 and Figure 2. In terms of attenuate metabolic syndrome action, olive oil increased the abundances of six bacteria (Eubacteriaceae, Bifidobacteriaceae, Anaeroplasmataceae, Erysipelotrichaceae, Clostridiaceae_1, etc.) and decreased those of seven bacteria (Corynebacteriaceae, Enterococcaceae, Aerococcaceae, Staphylococcaceae, Coriobacteriaceae, etc.) at family level in high-fructose/fat diet-induced rats or normal mice (43, 45). At the phylum level, olive oil enhanced the amount of Proteobacteria in normal mice (44). At the genus level, olive oil elevated the amounts of eight bacteria (Olsenella, Bifidobacterium, [Eubacterium]_fissicatena_group, Ruminococcaceae_UCG-014, Allobaculum, etc.), and lowered those of 12 bacteria (Bilophila, Aerococcus, Staphylococcus, uncultured_bacterium_f_Coriobacteriaceae, Faecalitalea, etc.) (43–45). Moreover, six types of bacteria (c__Gammaproteobacteria, o__Enterobacteriales, f__Enterobacteriaceae, s__uncultured_bacterium_g_Blautia, s__uncultured_bacterium_g_Allobaculum, etc.) were screened to be differential gut microbiota biomarkers for olive oil and showed attenuate metabolic syndrome activity against high-fructose/fat diet-induced rats (43).
Regarding the other above-mentioned health-promoting effects, olive oil boosted the abundances of Actinobacteria, Bacteroidetes, Verrucomicrobia, Cyanobacteria, and Deferibacteres as well as Bacteroidetes/Firmicutes ratio, and declined that of Firmicutes at the phylum level (47, 48, 50). At the genus level, olive oil raised the amounts of 12 bacteria (Clostridiaceae, Bacteroides, Muribaculum, Akkermansia, Ruminococcaceae_UCG_005, etc.), and reduced those of seven bacteria (Burkholderiaceae, Lachnospira, Eubacterium_xylanophilum_group, Mogibacterium, Dethiosulfovibrionaceae, etc.), while exerted anti-food allergy, anti-diabetes, anti-HIV, and immunoregulation effects (47–50). On the other hand, olive oil supplement added the abundances of Lactobacillus sp., Clostridia XIVa, and Universal as exerted anti-hypertension activity (46), and aggrandized the number of Lactic acid bacteria while exhibited anti-obese action (19). Moreover, for olive oil revealed anti-food allergy effect, 11 types of bacteria (f__Bifidobacteriaceae, o__Bifidobacteriales, o__Micrococcales, c__Actinobacteria, f__Streptococcaceae, etc.) were identified to be differential gut microbiota biomarkers (47). Olive oil exhibited anti-diabetes activity, and 11 types of bacteria (Bacteroides, Muribaculaceae, Alistipes, Lachnoclostridium, Tyzzerella, etc.) were characterized as differential gut microbiota biomarkers (48). As to olive oil showed anti-HIV action, g__Gardnerella was the differential gut microbiota biomarker (49).
Some similarities in gut microbiota modulation could be seen while olive oil exhibited different health-promoting effects in animal experiments. At the phylum level, the abundance of Firmicutes was reduced and that of Bacteroides was enhanced, while olive oil exerted anti-diabetes and immunoregulation effects (48, 50).
In short, olive oil could modulate gut microbiota during their health-promoting effects. Moreover, differential gut microbiota biomarkers have been gained for exerting attenuate metabolic syndrome, anti-food allergy, anti-diabetes, and anti-HIV actions. Furthermore, some similarities in gut microbiota modulation have been discovered as this oil exhibited anti-diabetes and immunoregulation effects.
3.3 Flaxseed oil modulation
Flaxseed oil has been discovered to modulate gut microbiota during its health-promoting effects (18, 50–63), as displayed in Table 3 and Figure 3. In terms of regulation of lipid metabolism effect, flaxseed oil treatment increased the abundances of Actinobacteria, Proteobacteria, and Spirochaeta, and decreased those of Firmicutes, Saccharibacteria, and Verrucomicrobia at the phylum level in Albas cashmere goats (52, 53). At the genus level, flaxseed oil elevated the amounts of 17 bacteria (Uc_Ruminococcaceae, Uc_Lachnospiraceae, Oscillospira, Ruminococcus, Ruminococcus_2, etc.), and declined those of 10 bacteria (Sporosarcina, Mogibacteriaceae, Clostridium, unclassified_f_Peptostreptococcaceae, Clostridium_sensu_stricto_1, etc.) (51–53). Moreover, six types of bacteria (Ruminococcus, Anaerotruncus, Ruminococcaceae, Lachnospiraceae, Dehalobacterium, etc.) have been screened to be differential gut microbiota biomarkers for flaxseed oil and showed lipid metabolism regulation against high-fat diet-induced mice (51). While Lachnospiraceae_NK3A20_group has been identified as a differential gut microbiota biomarker for flaxseed oil-regulated lipid metabolism in Albas cashmere goats (53). Regarding anti-diabetes action, flaxseed oil supplement enhanced Bacteroidetes, and reduced Firmicutes and Firmicutes/Bacteroidetes ratio at phylum level in streptozotocin-nicotinamide-induced rats (55). At the genus level, flaxseed oil upregulated Alistipes and downregulated Blautia. While the study of Xia et al. (56) has revealed that flaxseed oil treatment raised the abundance of six bacteria (Bacteroidetes, Muribaculaceae, Streptococcaceae, Lactococcus, Streptococcus, etc.). To anti-atherosclerosis activity, flaxseed oil lessened the number of Eubacterium and Firmicutes/Bacteroidetes ratio at the phylum level in TMAO and high-fat diet-induced ApoE−/− mice (57, 58). At the genus level, flaxseed oil expanded the abundances of Alistipes and Odoribacter and diminished the amounts of seven bacteria (Intestinimonas, Bilophila, Anaerotruncus, Oscillibacter, Lachnoclostridium, etc.).
For aforementioned other effects, flaxseed oil aggrandized the abundances of Actinobacteria, Melainabacteria, Bacteroidetes, Proteobacteria, Verrucomicrobia, and/or Firmicutes and lowered those of Firmicutes, Proteobacteria, Actinobacteria and/or Spirochaetes as well as Firmicutes/Bacteroidetes ratio, at the phylum level (18, 50, 59, 61–63). At genus level, flaxseed oil increased the amounts of 19 bacteria (Allobaculum, Coriobacteriaceae spp., Parabacteroides, uncultured_bacterium_f_Lachnospiraceae, Ruminococcaceae_UCG-005, etc.) and decreased those of 12 bacteria (Clostridiales spp., uncultured_bacterium_f_Desulfovibrionaceae, Akkermansia, Romboutsia, Prevotella_9, etc.). Moreover, for the anti-aging action of flaxseed oil on D-galactose-induced rats, 11 types of bacteria (p_Firmicutes, o_Clostridiales, c_Clostridia, f_Lachnospiraceae, s_uncultured_bacterium_f_Lachnospiracea, etc.) have been identified to be differential gut microbiota biomarkers (60).
Some similarities and differences in gut microbiota modulation could be observed, while flaxseed oil showed different health-promoting effects in animal experiments. At the phylum level, the abundance of Firmicutes was downregulated while flaxseed oil exerted immunoregulation (50), regulation of lipid metabolism (53), and anti-diabetes (55) effects. Meanwhile, Firmicutes/Bacteroidetes ratio was diminished as this oil exhibited anti-diabetes (55), anti-atherosclerosis (58), and ameliorating polycystic ovary syndrome (61) activities. While that of Firmicutes was upregulated as flaxseed oil revealed anti-hepatocellular carcinoma action (18). The amount of Bacteroidetes was added while flaxseed oil displayed anti-diabetes (55) and anti-ulcerative colitis (63) effects. The number of Actinobacteria was increased while flaxseed oil reflected regulation of lipid metabolism (52) and improving intrauterine growth retardation (62) effects, whereas it was decreased as this oil showed ameliorating polycystic ovary syndrome action (61). The abundance of Proteobacteria was raised while flaxseed oil exerted regulation of lipid metabolism (53) and anti-ulcerative colitis (63) effects, while it was shrunk as flaxseed oil exhibited hepatoprotection (59), ameliorating polycystic ovary syndrome (61) and anti-hepatocellular carcinoma (18) actions. The amount of Verrucomicrobia was reduced while flaxseed oil revealed regulation of lipid metabolism effect (53), while it was elevated as this oil emerged anti-ulcerative colitis action (63). At the genus level, the number of Allobaculum was augmented, while flaxseed oil showed immunoregulation (50) and ameliorating polycystic ovary syndrome (61) effects. The amount of Lachnoclostridium was downregulated while flaxseed oil revealed anti-atherosclerosis effect (58), and that was upregulated as the oil showed anti-ulcerative colitis action (63). The number of Alistipes was enhanced as flaxseed oil displayed anti-diabetes (55) and anti-atherosclerosis (57) activities. The abundance of Enterorhabdus was declined while flaxseed oil exerted anti-atherosclerosis (58) and anti-hepatocellular carcinoma (18) actions. The amount of Romboutsia was decreased as flaxseed oil exhibited anti-aging (60) and anti-ulcerative colitis (63) activities. The abundance of Ruminococcaceae_UCG-005 was upregulated while flaxseed oil showed anti-aging effect (60) and was downregulated as this oil exhibited anti-ulcerative colitis activity (63). The quantity of Lactobacillus was enhanced while flaxseed oil exerted ameliorating polycystic ovary syndrome (61) and anti-ulcerative colitis (63) effects. The number of Bifidobacterium was enhanced, while flaxseed oil revealed regulation of lipid metabolism (52), ameliorating polycystic ovary syndrome (61), and improving intrauterine growth retardation (62) activities. The abundance of Blautia was decreased while flaxseed oil exhibited anti-diabetes effect (55), while it was increased as the oil exerted improving intrauterine growth retardation action (62). The amount of Streptococcus was declined, while flaxseed oil showed regulation of lipid metabolism (52) and ameliorating polycystic ovary syndrome (61) activities. The number of Turicibacter was added while flaxseed oil displayed regulation of lipid metabolism (52) and anti-hepatocellular carcinoma (18) effects. The abundance of Enterorhabdus was lowered while flaxseed oil reflected anti-atherosclerosis (58) and anti-hepatocellular carcinoma (18) actions.
In a word, flaxseed oil could modulate gut microbiota during their many health-promoting effects. Moreover, differential gut microbiota biomarkers have been acquired for their revealed lipid metabolism regulation and anti-aging effect. Furthermore, some differences and similarities in gut microbiota modulation have been found while this oil exhibited different health-promoting effects.
3.4 Other oil modulations
There were other edible plant oils which could modulate gut microbiota during their health-promoting effects, as shown in Table 4 and Figure 4. In terms of walnut oil, it increased the abundances of S24-7, Lachnospiraceae and Ruminococcaceae, and decreased that of Moraxellaceae at family level while exerted anti-Alzheimer’s disease effect on scopolamine-induced mice (64). At the phylum level, walnut oil elevated the amounts of Firmicutes, Actinobacteria, and/or Bacteroidetes, and declined those of Proteobacteria and/or Epsilonbacteraeota while revealed anti-ulcerative colitis action in dextran sulfate sodium-induced colitis mice (65) and anti-Alzheimer’s disease activity in scopolamine-induced mice (64). At the genus level, walnut oil enhanced the quantities of seven bacteria (Lactobacillus, Lachnospiraceae_NK4A136_group, Faecalibaculum, Bifidobacterium, [Ruminococcus]_torques_group, etc.), and reduced those of six bacteria (Helicobacter, Bacteroides, Clostridium_sensu_stricto_1, Staphylococcus, Desulfovibrio, etc.) (65). Moreover, Ruminococcaceae and Mogibacteriaceae were chosen to be differential gut microbiota biomarkers for walnut oil, which exhibited anti-Alzheimer’s disease effect against scopolamine-induced mice (64).
Regarding soybean oil, it raised the abundances of Bacteroidetes, Deferribacteres, and Proteobacteria, and shrunk those of Firmicutes, Tenericutes, and TM7 at the phylum level while revealed anti-atherosclerosis action to normal mice (66). Moreover, 11 kinds of bacteria (Prevotella, unclassified S24-7, Mucispirillum, Dehalobacterium, Ruminococcus, etc.) were identified as the differential gut microbiota biomarkers. On the other hand, the amounts of six bacteria (Akkermansia muciniphila, Turicibacter, Thermicanus, Clostridium saccharogumia, Lactobacillus pontis, etc.) were upregulated, and that of Coprobacillus was downregulated, while soybean oil exhibited attenuate metabolic syndrome effect (67) against high-fat diet-induced mice and increase insulin sensitivity and prevent fatty liver activities in normal mice (68). To sea buckthorn seed/pulp oil, it increased the number of Bacteroidetes and decreased that of Firmicutes as well as Firmicutes/Bacteroidetes ratio at the phylum level, while exhibited hypocholesterolemic effect on high-cholesterol diet-induced hamsters (69). At the genus level, it enhanced the amounts of nine bacteria (norank_f_Bacteroidales_S24-7_group, Allobaculum, norank_o_Mollicutes_RF9, Alistipes, Bacteroides, etc.), and lowered those of 13 bacteria (Acietatifactor, Lactobacillus, norank_f_Ruminococcaceae, Mucispirillum, Anaeroplasma, etc.), while exerted hypocholesterolemic (69) and immunoenhancement (70) effects. Moreover, Tannerellaceae and Parabacteroides were characterized as differential gut microbiota biomarkers while sea buckthorn pulp oil exhibited immunoenhancement effect toward cyclophosphamide-induced mice (70). Otherwise, sea buckthorn seed and pulp oil enlarged the abundances of Oscillibacter and Roseburia, and shrunk that of Lactobacillus while exerted ameliorate non-alcoholic fatty liver disease effects in high-fat diet-induced mice (71).
Coconut oil could regulate gut microbiota during exerted improvements in diabetes (72), improving the gastrointestinal tract (73), anti-obese (17, 74, 75), and regulation of lipid metabolism (76) effects. At the phylum level, coconut oil increased the abundances of Firmicutes, Actinobacteria, and/or Bacteroidetes along with Firmicutes/Bacteroidetes ratio, and decreased those of Bacteroidetes, Spirochaetes, and/or Firmicutes (72, 74, 76). At the genus level, the amounts of Lactobacillus, Allobaculum, and Erysipelotrichaceae_UCG-006 were augmented, and those of Treponema and Turicibacter were declined, while coconut oil reflected improved diabetes (72) and regulation of lipid metabolism (76) activities. On the other hand, coconut oil upregulated the numbers of six kinds of bacteria (Alloprevotella, Bifidobacteriales, Lactobacilli, Enterobacteriaceae, Escherichia, etc.), and downregulated those of Corynebacterium, Pseudomonadales, Psychrobacter, Mitsuokella, and E. coli, while exhibited improving gastrointestinal tract (73) and anti-obese (17, 75) activities.
Perilla oil, respectively, increased the abundances of Clostridiaceae and Lachnospiraceae while exhibited relieved constipation toward sedentary healthy female (21) and exerted improved gut function to athletes (77) at the family level. At the phylum level, the amounts of Bacteroidetes, Spirochaetes, and Verrucomicrobia were raised, and those of Firmicutes and Proteobacteria as well as Firmicutes/Bacteroidetes ratio were lessened, while perilla oil exerted anti-non-alcoholic fatty liver disease (78) and improved gut function (77) actions. At the genus level, the numbers of eight bacteria (Alistipes, Alloprevotella, Parabacteroides, Rikenella, Roseburia, etc.) were enhanced, while those of 11 bacteria (unidentified_Ruminococcaceae, Lachnoclostridium, unidentified_Lachnospiraceae, Oscillibacter, Blautia, etc.) were lowered, as perilla oil displayed anti-diabetes (79) and anti-non-alcoholic fatty liver disease (78) activities.
Peony seed oil declined the amounts of Proteobacteria and Deferribacteres accompanied by Firmicutes/Bacteroidetes ratio at the phylum level, while exhibited hypocholesterolemic (80) and alleviated hyperlipidemia and hyperglycemia (81) effects. At the genus level, peony seed oil increased the abundances of unclassified_f_Ruminococcaceae, Ruminococcus_2, Lactobacillus, Prevotella and/or Parabacteroides, and decreased those of 10 bacteria (unclassified_f_Erysipelotrichaceae, Peptococcus, Christensenellaceae_R-7_group, norank_o_Mollicutes_RF9, norank_f_Eubacteriaceae, etc.) (80, 81). Moreover, Bacteroides, Turicibacter, and Allobaculum were screened to be differential gut microbiota biomarkers, while peony seed oil alleviated hyperlipidemia and hyperglycemia against high-fat diet-induced mice (81). Decaisnea insignis seed oil enhanced the amounts of Saccharibacteria and/or Bacteroidetes and declined those of Firmicutes and Proteobacteria at phylum level, while showed hepatoprotection against alcohol or L-carnitine-induced mice (82, 83). At the genus level, the abundances of eight bacteria (Lactobacillus, Conexibacter, Pepeococcus, Ruminoccoceae_UCG_004, Akkermansia, etc.) were elevated, while those of Helicobacter and Erysipelotrichaceae were lowered (82, 83). Moreover, Lactobacillus_gasseri was identified to be differential gut microbiota biomarker for Decaisnea insignis seed oil that exhibited hepatoprotection on alcohol-induced mice (82). Sacha inchi oil increased the amount of Firmicutes, and decreased that of Bacteroidetes at the phylum level, while exerted hypolipidemic effect in high-fat diet-induced rats (84). At the genus level, it enhanced the abundance of Alistipes, and shrunk those of Unidentified Enterobacteriaceae, Bacteroides, and Lachnoclostridium (84). Moreover, six bacteria (Roseburia, Turicibacter, Butyrivibrio, Unidentified Enterobacteriaceae, Escherichia, etc.) were chosen to be differential gut microbiota biomarkers. While, sacha inchi oil upregulated the number of Bacteroidetes, and downregulated that of Firmicutes as well as Firmicutes/Bacteroidetes ratio at the phylum level, as exhibited regulation of lipid metabolism effect against high-fat diet-induced mice (85). Moreover, Deferribacteraceae, Deferribacterales, and Deferribacteres were identified to be differential gut microbiota biomarkers. Millet/riceberry bran oil increased the amounts of Verrucomicrobia, Saccharibacteria, and Bacteroidetes, and decreased that of Firmicutes along with Firmicutes/Bacteroidetes ratio at the phylum level, while exhibited attenuate metabolic syndrome effect (86) or anti-cancer activity (87). At genus level, the numbers of six bacteria (Ruminococcaceae UCG-013, Ruminococcaceae UCG-014, Adlercreutzia, Enterorhabdus, Papillibacter, etc.) were upregulated, and those of Eubacterium coprostanoligenes, Ruminoclostridium 6 and Bacteroides were downregulated, while riceberry bran oil exerted anti-cancer action on diethylnitrosamine and 1,2-dimethylhydrazine-induced rats (87). Moreover, eight bacteria (Akkernansia, unclassified_f__Lachnospiraceae, Prevotellaceae UCG_001, Erysipelatoclostridium, Ruminococcaceae UCG_009, etc.) were screened to be differential gut microbiota biomarkers, as millet bran oil showed attenuate metabolic syndrome effect (86). Torreya grandis oil augmented the abundances of Firmicutes and Actinobacteria along with Firmicutes/Bacteroidetes ratio, and declined those of Bacteroidetes, Proteobacteria, and Verrucomicrobiota at the phylum level, while exhibited anti-obese (88) and anti-Alzheimer’s disease (89) effects. At the family level, Torreya grandis oil aggrandized the amounts of Erysipelotrichaceae, Coriobacteriaceae, Lactobacillaceae, and Bifidobacteriaceae, and reduced that of Porphyromonadaceae, while exerted anti-obese effect on high-fat diet-induced mice (88). At the species level, Torreya grandis oil raised the numbers of eight bacteria (Allobaculum, Bifidobacterium, Olsenella, Parasutterella, unclassified Ruminococcaceae, etc.), while showed anti-Alzheimer’s disease action against scopolamine-induced mice (89).
Schizochytrium sp. L. oil (85), safflower oil (90), peanut oil (43), kiwifruit seed oil (91), okra seed oil (92), wild melon seed oil (93), tomato seed oil (94) or almond oil (95) could also modulate gut microbiota during their health-promoting effects. At the phylum level, the abundances of Bacteroidetes and/or Deferribacteres as well as Firmicutes/Bacteroidetes ratio were increased, and those of Firmicutes, Bacteroidetes, Proteobacteria, and/or Actinobacteria along with Firmicutes/Bacteroidetes ratio were decreased (43, 85, 90–95). At the genus level, the abundances of 33 bacteria (Blautia, Olsenella, Peptoclostridium, Ruminococcaceae_UCG-009, [Eubacterium]_fissicatena_group, etc.) were enhanced, while these oils displayed the health-promoting effects (43, 90–96). On the contrary, those of 39 bacteria (Barnesiella, Bilophila, Leuconostoc, [Eubacterium]_nodatum_group, Lactococcus, etc.) were reduced. Moreover, for Schizochytrium sp. L. oil exerted regulation of lipid metabolism effect on high-fat diet-induced mice, Rhodospirillales and Alphaproteobacteria were identified to be differential gut microbiota biomarkers (85). In terms of peanut oil exhibited attenuate metabolic syndrome action against high-fat/high sucrose diet-induced rats, 10 types of bacteria (g__Faecalibaculum, s__uncultured_bacterium_g_Faecalibaculum, p__Proteobacteria, f_Clostridiaceae_1, g__Ruminococcaceae_UCG_014, etc.) were screened to be differential gut microbiota biomarkers (43). To kiwifruit seed oil showed anti-obese action on high-fat diet-induced mice, Pseudoflavonifractor, Flavonifractor, Intestinimonas, Romboutsia and Olsenlla were characterized to be differential gut microbiota biomarkers (91). Regarding tomato seed oil showed anti-hyperlipidemia effect against high-fat diet-induced mice, norank_o_Gastranaerophilale, Phascolarctobacterium, and Lactobacillus were chosen to be differential gut microbiota biomarkers (94).
Overall, there were other oils which could modulate gut microbiota during their health-promoting effects. Moreover, differential gut microbiota biomarkers have been acquired for some of them to show health-promoting effects.
3.5 Influences of dosage and treatment time of edible plant oils
Different dosages of camellia oil, olive oil, and flaxseed oil have been demonstrated to exhibit different behaviors in modulating gut microbiota during their health-promoting effects (16, 51, 56, 63, 68). In terms of camellia oil, 2 and 4 mL/kg BW of it significantly enriched the abundances of Faecalibacterium, Muribaculaceae, and Coriobacteriaceae_UCG_002 in exercise mice, while 6 mL/kg BW of it obviously enhanced the amounts of Desulfobacteria and Faecalibaculum (16). Regarding olive oil, Akkermansia muciniphila was present at the dose of 7%, whereas Mucispirillum schaedleri was the most abundant at that of 21%, as it increased insulin sensitivity and prevented fatty liver in normal mice (68). For flaxseed oil, 400 mg/kg BW of it increased the quantities of Lactobacillus and Lachnospiraceae_NK4A136_group, while 1,600 mg/kg BW of it added the number of Prevotellaceae_UCG-001 during its anti-ulcerative colitis effect in dextran sulfate sodium-induced rats (63).
Moreover, peony seed oil (80, 81), perilla oil (77, 79), Torreya grandis oil (88, 89), and other oils (69, 91, 93–95) have also been proven to generate different modulations on gut microbiota because of different doses during their health-promoting effects. For example, 1.5 mL of peony seed oil upregulated the proportions of Lactobacillus and Prevotella, while 1.0 mL of it elevated the presence of Parabacteroides and reduced the prevalence of Ruminococcaceae_Ruminococcu and Mucispirillum, as alleviated hyperlipidemia and hyperglycemia in high-fat diet-induced mice (81). For perilla oil exhibited anti-diabetes activity against high-fat diet-induced KKAy mice, Dubosiella and Turicibacter were the most abundant genus in low-dose (0.67 g/kg BW) group relative to middle-dose (1.33 g/kg BW) and high-dose (2.00 g/kg BW) groups, while Lactobacillus was the most abundant genus in the high-dose group compared to the low-dose and middle-dose groups (79). 250, 550, and 850 mg/kg of Torreya grandis oil decreased Porphyromonadaceae to 15.57, 10.96, and 6.97%, increased Erysipelotrichaceae to 20.32, 36.55, and 38.75%, and enhanced Coriobacteriaceae to 7.65, 1.94, and 3.07% during their anti-obese effects in high-fat diet-induced mice (88). 50% of sea buckthorn seed oil boosted the number of norank_f_Bacteroidales_S24-7_group, whereas 100% of it increased the amounts of Allobaculum and norank_o_Mollicutes_RF9 in high-cholesterol diet-induced hamsters (69). 5.9% of tomato seed oil significantly changed the abundances of Anaerotruncus and Alistipes, while 11.8% of it obviously altered the amounts of Lactobacillus, Rikenella, and Enterorhabdus in high-fat diet-induced mice (94). The abundances of Pseudoflavonifractor, Flavonifractor, Intestinimonas, Romboutsia, and Olsenlla were markedly increased in the high-dose (3.0 mL/kg) kiwifruit seed oil group as compared to the low-dose (1.0 mL/kg) group, in high-fat diet-induced mice (91). 8 g/kg BW of almond oil notably changed the amounts of Ruminococcaceae_UCG-014, Lactobacillus, and Lachnospiraceae_NK4A136_group, 4 g/kg BW of it significantly altered the quantity of Bacteroides, and 2 g/kg BW of it obviously changed the abundances of Clostridium_sensu_stricto_1 and Fusicatenibacter in streptozotocin-induced rats (95). 9.5% of wild melon seed oil showed significant differences in Clostridiales_vadinBB60_group and Streptococcaceae in comparison with 4.75% of it during its hypocholesterolemic effect in high-cholesterol diet-induced hamsters (93).
On the other hand, treatment time has been indicated to influence the modulations of edible plant oils on gut microbiota in three animal studies (67, 90, 97). The study taken by Hidalgo et al. (97) has shown that 6 weeks of olive oil intervention could not significantly alter the gut microbiota of normal ICR mice, whereas 12 weeks of that showed significant changes. The investigation of Danneskiold-Samsøe et al. (90) has indicated that significant differences were found in the abundances of Bilophila, Olsenella, Clostridium XIVa, Parasutterella, and Pseudoflavonifactor in the cecum as well as Allobaculum and Parasutterella in the colon at different treatment time points (2, 5, 10, and 40 weeks) of safflower oil in normal C57BL/6 J mice. Another study by Patrone et al. (67) has revealed that lower proportions of Thermicanus, Akkermansia muciniphila, Lactobacillus pontis, Eubacterium biforme, Turicibacter, but higher Proteus, Ruminococcus flavefaciens, Clostridium perfringen, Allobaculum and Deltaproteobacteria of high-fat diet-induced mice were found in coconut oil group as compared with soybean oil group at 2 weeks. While at 8 weeks, Lactobacillus reuteri, F16, Anaerofustis, Allobaculum, and Deltaproteobacteria were significantly higher, whereas Akkermansia muciniphila was lower in coconut oil group compared to soybean oil group.
3.6 Comparisons between edible plant oils and edible animal oils
Comparisons between different edible plant oils on gut microbiota modulations have been made in some studies. Compared with camellia oil, different modulations of corn oil, olive oil, soybean oil, perilla oil, and sunflower oil on the gut microbiota of mice or rats were seen while exhibited hepatoprotection, anti-ulcerative colitis, anti-obese, and anti-Alzheimer’s disease effects (31, 32, 36, 38, 42). For example, Lee et al. (32) have found that Prevotella was higher and Actinobacteria, Bacteroidetes, Firmicutes, and Proteobacteria were lower in camellia oil-treated ulcerative rats compared to soybean oil-treated ulcerative rats. Meanwhile, Actinobacteria and Firmicutes were higher and Bacteroidetes and Proteobacteria were lower in camellia oil-treated ulcerative rats compared to olive oil-treated ulcerative rats. Relative to olive oil, distinctions in gut microbiota modulation of mice, rats, or grouper have also been discovered in soybean oil, coconut oil, corn oil, and peanut oil as exerted increase insulin sensitivity and prevent fatty liver, attenuate metabolic syndrome, and improve gut health activities (43, 68, 98). For instance, as exhibited increase insulin sensitivity and prevent fatty liver effect on normal mice, 7% soybean oil treatment displayed a significant increase in Akkermansia muciniphila, whereas 7% olive oil consumption showed significant increments in 10 species (primarily Bacteroides acidifaciens, and Faecalibacterium prausnitzii), and 7% coconut oil administration had significant enhancements in eight species (particularly Mucispirillum schaedleri) (68). In comparison with flaxseed oil, different gut microbiota modulations of corn oil, olive oil, and soybean oil were generated in pigs, rats, and mice during their hepatoprotection, immunoregulation, anti-diabetes, and improving intrauterine growth retardation actions (50, 55, 59, 62). For example, as exerted anti-diabetes activity against streptozotocin-nicotinamide-induced rats, Blautia was higher and Alistipes was lower in corn oil group, as compared to flaxseed oil group (55). Additionally, distinctions of gut microbiota modulation to mice or suckling calves were found among soybean oil, peanut oil, coconut oil, palm oil, Schizochytrium sp. L. oil, sacha inchi oil, sea buckthorn pulp oil, sea buckthorn seed oil, walnut oil, and/or sunflower oil (65, 67, 71, 76, 85). For instance, significant differences of Lactobacillus, Oscillibacter, and/or Roseburia were observed among soybean oil, peanut oil, sea buckthorn seed oil, and sea buckthorn pulp oil treatments, during their ameliorate non-alcoholic fatty liver disease actions (71).
On the other hand, some studies have compared the gut microbiota modulations between edible plant oils and edible animal oils (including fish oil and lard oil) in vivo experiments (7, 57, 71, 78, 85, 98–100). In terms of comparisons with fish oil, the differential bacteria of Schizochytrium sp. L. oil group were Rhodospirillales and Alphaproteobacteria, whereas those of sacha inchi oil group were Deferribacteraceae, Deferribacterales, and Deferribacteres in high-fat diet-induced mice (85). Perilla oil has been reported to have similar gut microbiota modulations with fish oil in high-fat diet-induced rats (78). However, flaxseed oil was weaker than fish oil in modulating the gut microbiota of TMAO-induced ApoE−/− mice (57). And, soybean oil, fish oil, and lard oil showed differential influences on the gut microbiota of middle-aged rats (100). Corn oil, olive oil, and fish oil had significantly different modulations on the amounts of Photobacterium, Romboutsia, Epulopiscium, Clostridium_sensu_stricto_1, Staphylococcus and/or Leuconostoc in grouper (98). Compared with lard oil, significant changes in Blautia were produced by peanut oil, sea buckthorn seed oil, and sea buckthorn pulp oil, in high-fat diet-induced mice (71). Soybean oil, olive oil, and lard oil treatments exhibited obviously different modulations on the gut microbiota of mice at phylum, class, order, and family levels (7). Corn oil and lard oil revealed notably different alterations in the gut microbiota of hamsters at phylum, family, and genus levels (99).
4 Correlations between gut microbiota and biochemical indexes affected by edible plant oils
Significant correlations between modulated gut microbiota and changed biochemical indexes were found while edible plant oils exhibited health-promoting effects, as summarized in Table 5.
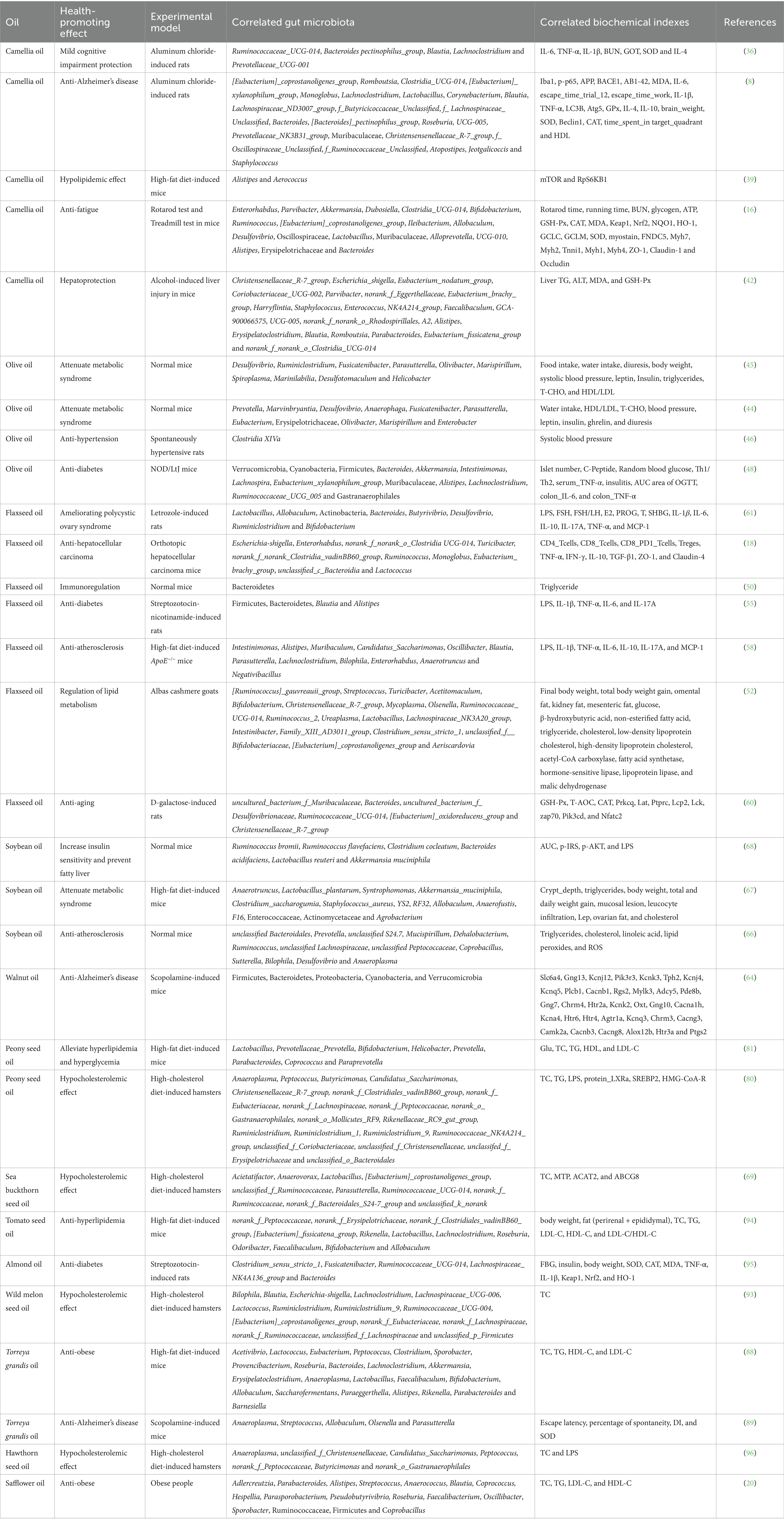
Table 5. Correlations between modulated gut microbiota and changed biochemical indexes by edible plant oils.
4.1 Affected by camellia oil
For camellia oil exerted mild cognitive impairment protection on aluminum chloride-induced rats (36), Bacteroides pectinophilus_group was positively correlated with TNF-α, IL-1β, and IL-6. Blautia was positively correlated with BUN, TNF-α, and IL-6. Lachnoclostridium and Prevotellaceae_UCG-001 were positively correlated with GOT and TNF-α, respectively. Ruminococcaceae_UCG-014 was negatively correlated with SOD and IL-4. For camellia oil exhibited anti-Alzheimer’s disease effect against aluminum chloride-induced rats (8), eight bacteria (Romboutsia, Clostridia_UCG-014, [Eubacterium]_coprostanoligenes_group, [Eubacterium]_xylanophilum_group, Monoglobus, etc.) had significantly positive correlations with 11 changed biochemical indexes (Iba1, p-p65, APP, BACE1, AB1-42, etc.), and had obviously negative relations with another 11 changed biochemical indexes (LC3B, Atg5, GPx, IL-4, IL-10, etc.). While 16 kinds of bacteria (Blautia, Lachnospiraceae_ND3007_group, f_Butyricicoccaceae_Unclassified, f_ Lachnospiraceae_Unclassified, Bacteroides, etc.) showed opposite correlations with these biochemical indexes.
Camellia oil revealed hypolipidemic effect on high-fat diet-induced mice (39), Alistipes was positively correlated with mTOR and RpS6KB1, and Aerococcus was negatively correlated with RpS6KB1. As this oil showed anti-fatigue action toward mice received with Rotarod test and Treadmill test (16), 19 bacteria (Enterorhabdus, Parvibacter, Akkermansia, Dubosiella, Clostridia_UCG-014, etc.) had significant correlations with 25 changed biochemical indexes (Rotarod time, Running time, BUN, glycogen, ATP, etc.) in serum, liver, muscle, and/or colon. Among them, Muribaculaceae was one of the differential gut microbiota biomarkers for camellia oil. Regarding this oil exhibited hepatoprotection against alcohol-induced liver injury in mice (42), Enterococcus and NK4A214_group were negatively correlated with liver TG, while Faecalibaculum was positively correlated with liver TG. Staphylococcus, Enterococcus, Romboutsia, and Eubacterium_fissicatena_group were negatively correlated with GSH-Px, whereas Harryflintia and Parvibacter were positively correlated with GSH-Px. Alistipes was negatively correlated with MDA, while Eubacterium_brachy_group and Parvibacter were positively correlated with MDA. norank_f_Eggerthellaceae, Parvibacter, and Coriobacteriaceae_UCG-002 were negatively correlated with ALT, whereas 14 bacteria (GCA-900066575, Staphylococcus, UCG-005, norank_f_norank_o_Rhodospirillales, Eubacterium_nodatum_group, etc.) were positively correlated with ALT.
In a word, significant correlations between modulated gut microbiota and changed biochemical indexes occurred during camellia oil exhibited mild cognitive impairment protection, anti-Alzheimer’s disease, hypolipidemic, anti-fatigue, and hepatoprotection effects.
4.2 Affected by olive oil
For olive oil exerted attenuate metabolic syndrome effect on normal mice (45), Desulfovibrio was positively correlated with food intake, water intake, diuresis, and T-CHO. Ruminiclostridium was positively correlated with systolic blood pressure and HDL/LDL and was negatively correlated with water intake. Fusicatenibacter was positively correlated with water intake and diuresis and was negatively correlated with body weight, T-CHO, and HDL/LDL. Parasutterella was negatively correlated with insulin and HDL/LDL. Olivibacter was positively correlated with triglycerides and was negatively correlated with food intake. Marispirillum was negatively correlated with leptin, and Spiroplasma was negatively correlated with food intake and HDL/LDL. Marinilabilia was positively correlated with food intake, water intake, and diuresis. Desulfotomaculum was negatively correlated with food intake and T-CHO. Helicobacter was positively correlated with water intake and diuresis and was negatively correlated with leptin. In another study, as olive oil exhibited attenuate metabolic syndrome activity in normal mice (44), Prevotella was negatively correlated with T-CHO, while Anaerophaga was positively correlated with T-CHO. Marvinbryantia and Eubacterium were positively correlated with leptin, while Marispirillum was negatively correlated with leptin. Desulfovibrio was positively correlated with water intake, blood pressure, insulin, and diuresis. Fusicatenibacter was negatively correlated with HDL/LDL and T-CHO. Parasutterella was positively correlated with T-CHO and was negatively correlated with HDL/LDL. Erysipelotrichaceae was positively correlated with T-CHO and was negatively correlated with ghrelin. Olivibacter was positively correlated with T-CHO and ghrelin. Enterobacter was negatively correlated with water intake. Regarding olive oil showed anti-hypertension effect against spontaneously hypertensive rats, Clostridia XIVa was negatively correlated with systolic blood pressure (46). For olive oil revealed anti-diabetes action toward NOD/LtJ mice (48), Lachnospira and Eubacterium_xylanophilum_group were positively correlated with Random blood glucose, Th1/Th2, insulities, and AUC area of OGTT. Muribaculaceae was negatively correlated with serum_TNF-α. Verrucomicrobia and Akkermansia were negatively correlated with colon_TNF-α. Cyanobacteria and Gastranaerophilales were negatively correlated with insulitis, AUC area of OGTT, and colon_TNF-α. Alistipes was positively correlated with C-peptide and was negatively correlated with AUC area of OGTT. Intestinimonas was negatively correlated with insulitis. Ruminococcaceae_UCG_005 was positively correlated with islet number and negatively correlated with Random blood glucose and AUC area of OGTT. Lachnoclostridium was negatively correlated with insulitis and AUC area of OGTT. Bacteroides was negatively correlated with colon_IL-6. Among them, Muribaculaceae, Akkermansia, Gastranaerophilales, Alistipes, Intestinimonas, Ruminococcaceae_UCG_005, Lachnoclostridium, and Bacteroides were differential gut microbiota biomarkers for olive oil.
Overall, notable correlations between modulated gut microbiota and changed biochemical indexes existed during olive oil exerted attenuate metabolic syndrome, anti-hypertension, and anti-diabetes actions.
4.3 Affected by flaxseed oil
For flaxseed oil exhibited ameliorating polycystic ovary syndrome effect on letrozole-induced rats (61), Firmicutes/Bacteroidetes ratio was positively correlated with E2, PROG, T, IL-1β, TNF-α, and MCP-1 in plasma and with IL-6, IL-17A, and MCP-1 in the ovary and was negatively correlated with IL-10 in the ovary. Actinobacteria was positively correlated with LPS, FSH/LH, E2, PROG, T, IL-6, and TNF-α in plasma and with IL-1β, IL-6, IL-17A, and TNF-α in the ovary and was negatively correlated with FSH and IL-10 in plasma. Lactobacillus was positively correlated with FSH and E2 in plasma and was negatively correlated with LPS, FSH/LH, T, and IL-6 in plasma and with IL-1β, TNF-α, and MCP-1 in ovary. Bacteroides was positively correlated with LPS, FSH/LH, IL-1β, IL-6, and TNF-α in plasma and with IL-1β, IL-6, IL-17A, and TNF-α in the ovary. Allobaculum was positively correlated with PROG in plasma and was negatively correlated with IL-17A and MCP-1 in plasma and with MCP-1 in ovary. Butyrivibrio was positively correlated with FSH, E2, PROG, SHBG, and IL-10 in plasma, and was negatively correlated with LPS, FSH/LH, T, IL-1β, IL-6, and MCP-1 in plasma and with IL-1β and MCP-1 in the ovary. Desulfovibrio was positively correlated with E2, PROG, SHBG, and IL-10 in plasma and with IL-10 in the ovary, and was negatively correlated with LPS, FSH/LH, T, IL-1β, IL-6, IL-17A, TNF-α, and MCP-1 in plasma and IL-17A, TNF-α and with MCP-1 in the ovary. Ruminiclostridium was positively correlated with FSH, PROG, and IL-10 in plasma, and was negatively correlated with T in plasma. Bifidobacterium was positively correlated with FSH, E2, and IL-10 in plasma and with IL-10 and IL-17A in the ovary, and was negatively correlated with LPS, FSH/LH, T, IL-1β, IL-6, TNF-α, and MCP-1 in plasma and with IL-6 in the ovary.
As flaxseed oil exerted anti-hepatocellular activity against orthotopic hepatocellular carcinoma mice (18), Escherichia-shigella was negatively correlated with CD8_Tcells and Claudin-4. Enterorhabdus was positively correlated with IFN-γ and was negatively correlated with CD8_PD1_Tcells and IL-10. norank_f_norank_o_Clostridia UCG-014 was positively correlated with CD4_Tcells, CD8_Tcells and IFN-γ, and was negatively correlated with CD8_PD1_Tcells and IL-10. Turicibacter was positively correlated with TNF-α and was negatively correlated with IL-10. norank_f_norank_Clostridia_vadinBB60_group was positively correlated with CD4_Tcells, CD8_Tcells and IFN-γ, and was negatively correlated with CD8_PD1_Tcells and IL-10. Ruminococcus was positively correlated with CD4_Tcells and TNF-α and was negatively correlated with IL-10. Monoglobus was positively correlated with CD4_Tcells, CD8_Tcells, IFN-γ, and ZO-1, and was negatively correlated with CD8_PD1_Tcells and TGF-β1. Eubacterium_brachy_group was positively correlated with IL-10 and TGF-β1 and was negatively correlated with CD8_Tcells. unclassified_c_Bacteroidia was positively correlated with CD4_Tcells, CD8_Tcells and IFN-γ, and was negatively correlated with TGF-β1. Lactococcus was negatively correlated with ZO-1 and Claudin-4.
Regarding flaxseed oil showed immunoregulation in normal mice (50), Bacteroidetes was negatively correlated with plasma triglyceride concentration. For flaxseed oil revealed anti-diabetes effect toward streptozotocin-nicotinamide-induced rats (55), Firmicutes was positively correlated with LPS, IL-1β, TNF-α, IL-6, and IL-17A, Bacteroidetes was negatively correlated with LPS, IL-1β, TNF-α, and IL-17A, Blautia was positively correlated with LPS, IL-1β, TNF-α, and IL-6, and Alistipes was negatively correlated with LPS and TNF-α. As flaxseed oil displayed anti-atherosclerosis action against high-fat diet-induced ApoE−/− mice (58), Intestinimonas was positively correlated with LPS and IL-1β in plasma and with IL-6 and IL-1β in the aorta. Oscillibacter was positively correlated with IL-17A in plasma and with TNF-α, IL-6, IL-1β, and IL-17A in the aorta, and was negatively correlated with IL-10. Parasutterella was positively correlated with IL-6. Lachnoclostridium was positively correlated with LPS in plasma and with TNF-α in the aorta. Bilophila was positively correlated with LPS and IL-1β in plasma and with TNF-α, IL-6, and IL-1β in the aorta. Enterorhabdus was positively correlated with TNF-α in the aorta. Anaerotruncus was positively correlated with IL-1β in plasma and with TNF-α in the aorta. Negativibacillus was positively correlated with LPS and IL-1β in plasma.
For flaxseed oil reflected regulation of lipid metabolism activity on Albas cashmere goats (52), 19 bacteria ([Ruminococcus]_gauvreauii_group, Streptococcus, Turicibacter, Acetitomaculum, Bifidobacterium, etc.) exhibited significant correlations with 17 changed biochemical indexes (final body weight, total body weight gain, omental fat, kidney fat, mesenteric fat, etc.).
As flaxseed oil exhibited anti-aging effect against D-galactose-induced rats (60), uncultured_bacterium_f_Muribaculaceae was negatively correlated with Nfatc2. Christensenellaceae_R-7_group was negatively correlated with Prkcq, Ptprc, Lcp2, Lck, zap70, and Pik3cd. Bacteroides was positively correlated with T-AOC in serum, while [Eubacterium]_oxidoreducens_group was negatively correlated with T-AOC in serum. uncultured_bacterium_f_Desulfovibrionaceae was negatively correlated with GSH-Px in the liver. Ruminococcaceae_UCG-014 was positively correlated with T-AOC in the liver, and [Eubacterium]_oxidoreducens_group was negatively correlated with T-AOC in the liver. [Eubacterium]_oxidoreducens_group was negatively correlated with CAT in the liver.
In short, obvious correlations between modulated gut microbiota and changed biochemical indexes appeared during flaxseed oil displayed ameliorating polycystic ovary syndrome, anti-hepatocellular, immunoregulation, anti-diabetes, anti-atherosclerosis, regulation of lipid metabolism, and anti-aging effect activities.
4.4 Affected by other oils
For soybean oil exhibited increase insulin sensitivity and prevented fatty liver effect on normal mice (68), Ruminococcus bromii, Ruminococcus flavefaciens, and Clostridium cocleatum were positively correlated with p-IRS and p-AKT, and were negatively correlated with AUC. Bacteroides acidifaciens was positively correlated with LPS, and Lactobacillus reuteri and Akkermansia muciniphila were negatively correlated with LPS. As soybean oil exerted attenuate metabolic syndrome action against high-fat diet-induced mice (67), Anaerotruncus was positively correlated with body weight and ovarian fat. Lactobacillus_plantarum, Akkermansia_muciniphila, and Clostridium_saccharogumia were positively correlated with Lep. Syntrophomonas was positively correlated with mucosal lesion, leucocyte infiltration, and Lep. Staphylococcus aureus was positively correlated with total and daily weight gain, body weight, and ovarian fat. YS2 was positively correlated with cholesterol and ovarian fat and was negatively correlated with Crypt_depth. RF32 and Enterococcaceae were negatively and positively correlated with Crypt_depth, respectively. Allobaculum was positively correlated with Lep and cholesterol. Anaerofustis was positively correlated with triglycerides and cholesterol; F16 was positively correlated with cholesterol. Actinomycetaceae was negatively correlated with cholesterol and body weight, and Agrobacterium was negatively correlated with total and daily weight gain. Regarding soybean oil showed anti-atherosclerosis activity in normal mice (66), 13 bacteria (unclassified Bacteroidales, Prevotella, unclassified S24.7, Mucispirillum, Dehalobacterium, etc.) had significant correlations with triglycerides, cholesterol, linoleic acid, lipid peroxides and/or ROS in serum, peritoneal macrophages, or aorta. Among them, Prevotella, unclassified S24.7, Mucispirillum, Dehalobacterium, Ruminococcus, unclassified Peptococcaceae, Coprobacillus, Bilophila, Desulfovibrio, and Anaeroplasma were differential gut microbiota biomarkers for soybean oil.
For peony seed oil exhibited hypocholesterolemic effect against high-cholesterol diet-induced hamsters (80), eight bacteria (Butyricimonas, norank_f_Peptococcaceae, norank_o_Gastranaerophilales, unclassified_f_Coriobacteriaceae, Peptococcus, etc.) were positively correlated with TC, while Anaeroplasma, Candidatus_Saccharimonas, Rikenellaceae_RC9_gut_group, and Ruminiclostridium_1 were negatively correlated with TC. Six bacteria (norank_f_Eubacteriaceae, norank_o_Mollicutes_RF9, Candidatus_Saccharimonas, norank_f_Clostridiales_vadinBB60_group, Rikenellaceae_RC9_gut_group, etc.) were negatively correlated with TG. Seven bacteria (Butyricimonas, norank_f_Peptococcaceae, norank_o_Gastranaerophilales, Papillibacter, Peptococcus, etc.) were positively correlated with LPS, while Anaeroplasma and Candidatus_Saccharimonas were negatively correlated with LPS. Six bacteria (Christensenellaceae_R-7_group, norank_f_Lachnospiraceae, norank_o_Mollicutes_RF9, Ruminiclostridium, Ruminococcaceae_NK4A214_group, etc.) were negatively correlated with protein_LXRa. unclassifed_f_Erysipelotrichaceae was positively correlated with SREBP2. norank_f_Peptococcaceae, Peptococcus, Ruminococcaceae_NK4A214_group and unclassified_f_Christensenellaceae were positively correlated with HMG-CoA-R. As sea buckthorn seed oil exerted hypocholesterolemic effect on high-cholesterol diet-induced hamsters (69), Acietatifactor, Anaerovorax, Lactobacillus, [Eubacterium]_coprostanoligenes_group and unclassified_f_Ruminococcaceae were positively correlated with TC, while Parasutterella and Ruminococcaceae_UCG-014 were negatively correlated with TC. Norank_f_Rumincoccaceae and unclassified_k_norank were positively correlated with MTP, while norank_f_Bacteroidales_S24-7_group was negatively correlated with MTP. Lactobacillus was positively correlated with ACAT2, and unclassified_k_norank was positively correlated with ABCG8. Regarding hawthorn seed oil reflected hypocholesterolemic effect on high-cholesterol diet-induced hamsters (96), unclassified_f_Christensenellaceae, Peptococcus, norank_f_Peptococcaceae, Butyricimona, and norank_o_Gastranaerophilales were positively correlated with TC, and Anaeroplasma and Candidatus_Saccharimonas were negatively correlated with TC. Unclassified_f_Christensenellaceae and norank_o_Gastranaerophilales were positively correlated with LPS, while Anaeroplasma and Candidatus_Saccharimonas were negatively correlated with LPS. For wild melon seed oil reflected hypocholesterolemic effect toward high-cholesterol diet-induced hamsters (93), 13 bacteria (Bilophila, Blautia, Escherichia-shigella, Lachnoclostridium, Lachnospiraceae_UCG-006, etc.) were positively correlated with TC, whereas norank_f_Eubacteriaceae and unclassified_p_Firmicutes were negatively correlated with TC.
Regarding walnut oil displayed anti-Alzheimer’s disease effect toward scopolamine-induced mice (64), Firmicutes, Bacteroidetes, Proteobacteria, Cyanobacteria, and Verrucomicrobia had significant correlations with differential genes enriched in the cortisol synthesis and secretion pathway, oxytocin signaling pathway, cholinergic signaling pathway, and serotonergic signaling pathway. Torreya grandis oil revealed anti-Alzheimer’s disease action against scopolamine-induced mice (89), and Anaeroplasma was positively correlated with escape latency. Streptococcus was negatively correlated with percentage of spontaneity. Allobaculum and Olsenella were positively correlated with DI. Parasutterella was positively correlated with SOD.
For Torreya grandis oil showed anti-obese activity on high-fat diet-induced mice (88), 23 bacteria (Acetivibrio, Lactococcus, Eubacterium, Peptococcus, Clostridium, etc.) had significant correlations with serum TC, TG, HDL-C, and LDL-C. As safflower oil exhibited anti-obese effect on obese people (20), 17 types of bacteria (Adlercreutzia, Parabacteroides, Alistipes, Streptococcus, Anaerococcus, etc.) exhibited notable correlations with serum TC, TG, LDL-C, and HDL-C.
Regarding tomato seed oil showed anti-hyperlipidemia action against high-fat diet-induced mice (94), Rikenella and Odoribacter were positively correlated with body weight, while Bifidobacterium and Allobaculum were negatively correlated with body weight. Odoribacter was positively correlated with fat (perirenal + epididymal). norank_f_Clostridiales_vadinBB60_group and Lactobacillus were negatively correlated with TC. Rikenella was positively correlated with TG, while norank_f_Clostridiales_vadinBB60_group was negatively correlated with TG. Bifidobacterium was negatively correlated with LDL-C. Roseburia, Lachnoclostridium, and Faecalibaculum were negatively correlated with HDL-C. Faecalibaculum was positively correlated with LDL-C/HDL-C, whereas Lactobacillus was negatively correlated with LDL-C/HDL-C. Among them, Lactobacillus was one of the differential gut microbiota biomarkers for tomato seed oil. For peony seed oil reflected alleviated hyperlipidemia and hyperglycemia effect on high-fat diet-induced mice (81), Bifidobacterium was positively correlated with Glu, while Prevotellaceae_Prevotella, Prevotella, Parabacteroides, and Paraprevotella were negatively correlated with Glu. Lactobacillus and Prevotella were negatively correlated with TC. Bifidobacterium was positively correlated with TG, Helicobacter was positively correlated with HDL, and Coprococcus was positively correlated with LDL-C. As almond oil displayed anti-diabetes activity in streptozotocin-induced rats (95), Clostridium_sensu_stricto_1 was positively correlated with FBG, MDA, TNF-α, IL-1β, and Keap1 and was negatively correlated with insulin, body weight, SOD, CAT, Nrf2, and HO-1. Ruminococcaceae_UCG-014 was negatively correlated with body weight, SOD, Nrf2, and HO-1. Fusicatenibacter was positively correlated with FBG, MDA, TNF-α, IL-1β, and Keap1, and was negatively correlated with insulin, body weight, SOD, CAT, and Nrf2. Lachnospiraceae_NK4A136_group was positively correlated with SOD and Nrf2 and was negatively correlated with FBG and IL-1β. Bacteroides was positively correlated with insulin, SOD, CAT, Nrf2, and HO-1 and was negatively correlated with FBG and IL-1β.
Overall, remarkable correlations between modulated gut microbiota and changed biochemical indexes were also observed during other oils reflected increase insulin sensitivity and prevent fatty liver, attenuate metabolic syndrome, anti-atherosclerosis, hypocholesterolemic, anti-Alzheimer’s disease, anti-obese, anti-hyperlipidemia, alleviate hyperlipidemia and hyperglycemia, and anti-diabetes effects.
5 Gut microbiota-derived metabolites altered by edible plant oils
5.1 Alterations on SCFAs
Camellia oil increased the levels of propionic acid and butyric acid in feces while exerted Alzheimer’s disease effect on aluminum chloride-induced rats (8). Meanwhile, this oil elevated the amounts of total SCFAs, acetic acid, propionic acid, butyric acid, isobutyric acid, valeric acid, and isovaleric acid in colon contents, while exhibited anti-ulcerative colitis action against dextran sulfate sodium-induced mice (33). The oil reduced the contents of acetic acid, butyric acid, isobutyric acid, isovaleric acid and pentanoic acid in colonic contents, as showed hypolipidemic activity toward high-fat diet-induced mice (39).
Olive oil and flaxseed oil enhanced propionic acid production in plasma while displayed immunoregulation in normal mice (50). Moreover, Flaxseed oil supplementation increased the levels of acetic acid, propionic acid, butyric acid, isobutyric acid, valeric acid, and/or isovaleric acid in feces, while exhibited ameliorating polycystic ovary syndrome (61), anti-hepatocellular carcinoma (18), anti-atherosclerosis (57, 58) and anti-diabetes (55) effects.
Sea buckthorn pulp oil increased the concentrations of acetic acid, butyric acid, and total SCFAs in feces, while exerted immunoenhancement effect on cyclophosphamide-induced mice (70). Sea buckthorn seed oil elevated the levels of acetic acid, propionic acid, butyric acid, and total SCFAs in feces, as exhibited hypocholesterolemic effect against high-cholesterol diet-induced hamsters (69). Coconut oil enhanced the production of propionic acid and butyric acid in feces, while showed anti-obese effect on obese rats (75). Riceberry bran oil aggrandized the levels of butyric acid and valeric acid and reduced that of acetic acid in feces, while showed anti-cancer activity in diethylnitrosamine and 1,2-dimethylhydrazine-induced rats (87). Walnut oil raised the amounts of total SCFAs, acetic acid, propionic acid, and butyric acid in feces, as revealed anti-ulcerative colitis activity against dextran sulfate sodium-induced colitis mice (65). Walnut oil increased the levels of propionic acid, butyric acid, and valeric acid in plasma, while displayed anti-Alzheimer’s disease effect in scopolamine-induced mice (64). Decaisnea insignis seed oil enhanced the concentrations of total SCFAs, acetic acid, propionic acid, and butyric acid in feces, as revealed hepatoprotection in L-carnitine-induced mice (83). Wild melon seed oil increased the levels of total SCFAs, acetic acid, propionic acid, and butyric acid in feces, as showed hypocholesterolemic effect on high-cholesterol diet-induced hamsters (93). Torreya grandis oil elevated the butyric acid concentration in serum, while exerted anti-Alzheimer’s disease action against scopolamine-induced mice (89). Hawthorn seed oil added the contents of total SCFAs, acetic acid, propionic acid, and butyric acid in feces, as revealed hypocholesterolemic effect on high-cholesterol diet-induced hamsters (96). Okra seed oil decreased concentrations of acetic acid, isobutyric acid, and isovaleric acid, and increased that of butyric acid in cecal contents of ethanol-induced mice (92).
5.2 Alterations on other gut microbiota-derived metabolites
Flaxseed oil intervention modulated the microbial bile acids (BAs) metabolism in feces while exhibited anti-atherosclerosis action on high-fat diet-induced ApoE−/− mice, showing increases of lithocholic acid, allocholic acid, glycocholic acid, and taurocholic acid and decreases of allolithocholic acid, isolithocholic acid, 7-ketodeoxycholic acid, β-ursodeoxycholic acid, chenodeoxycholic acid, and hyodeoxycholic acid (58). Flaxseed oil caused a higher conjugated BA concentration and conjugated/free BA ratio in cecal contents in high-fat diet-induced mice (51). Thereinto, the concentrations of several unconjugated BAs, including chenodeoxycholic acid and β-murocholic acid, were higher. Moreover, the concentrations of taurine-conjugated BAs, and in particular taurocholic acid and tauro-ursodesoxycholic acid were increased. Furthermore, the amounts of cholic acid and tauro-β-murocholic acid were elevated. Sacha inchi oil declined primary BAs including cholic acid, glycocholic acid, taurochenodeoxycholic acid, and taurocholic acid in the feces of high-fat diet-induced rats (84). Meanwhile, it significantly modulated the levels of glycerolipids and glycerophospholipids in the liver.
Sea buckthorn seed oil changed the compositions of hepatic fatty acids and fecal fatty acids of high-cholesterol diet-induced hamsters (69). Tomato seed oil reduced saturated fatty acids, monounsaturated fatty acids, and total fatty acid concentrations, and added polyunsaturated fatty acids in the liver and/or feces of high-fat diet-induced mice (94). Okra seed oil increased the levels of pentadecanoic acid, palmitic acid, heptadecanoic acid, linoleic acid, oleic acid, etc., and decreased those of myristic acid and trans-9-octadecenoic acid in serum, while showed hepatoprotection on ethanol-induced mice (92). Coconut oil changed the fatty acid composition of the liver and longissimus dorsi while exhibited regulation of lipid metabolism effect in Holstein male calves (76). Torreya grandis oil supplement generated alterations in fatty acid composition in livers of high-fat diet-induced mice, revealing increments of eicosadienoic acid, sciadonic acid, n-6 PUFA, MUFA, and PUFA, and reductions of arachidonic acid and SFA (88).
About 117 metabolites and 392 metabolites in feces were, respectively, upregulated and downregulated by camellia oil treatment, while it revealed gastroprotection on ethanol-induced mice (41). These metabolites were amino acid and its metabolites, terpenoids, heterocyclic compounds, and lignans compounds, which were significantly enriched in four key metabolic pathways, including biosynthesis of cofactors, metabolic pathway, purine metabolism, and ammonia acid biosynthesis. 159 significantly different metabolites (lipids and lipid-like molecules) in serum metabolites of mice were identified between olive oil-treated group and diabetic group (48). These metabolites were significantly enriched in 18 metabolic pathways. Decaisnea insignis seed oil led to increases in concentrations of squalene, hesperidin, 2-aminophenol, 5-hydroxyindole-3-acetic acid, abietic acid, etc., and decreases in levels of androsterone, triacetin, farnesal, indole-3-acetamide, thymidine-5′-monophosphate dreg pored, etc., in the cecal contents of alcohol-induced mice (82). Moreover, five correlated KEGG pathways were enriched by these metabolites, including steroid hormone biosynthesis, steroid biosynthesis, pyrimidine metabolism, tryptophan metabolism, and tryptophan metabolism pathways. Peony seed oil notably changed 30 metabolites in feces of high-fat diet-induced mice, including organic acids and derivatives, phenylpropanoids and polyketides, benzenoids, organoheterocyclic compounds, lipids and lipid-like molecules, etc. (81).
5.3 Correlations between altered metabolites and modulated gut microbiota
5.3.1 SCFAs and gut microbiota
For camellia oil exhibited anti-Alzheimer’s disease effect on aluminum chloride-induced rats (8), the acetic acid in feces was positively correlated with Prevotellaceae_NK3B31_group, and negatively correlated with f__Ruminococcaceae_Unclassified, Atopostipes, Jeotgalicoccus and Staphylococcus. Meanwhile, the butyric acid in feces was positively correlated with Clostridia_UCG-014 and Lachnoclostridium, and negatively correlated with Blautia, Lachnospiraceae_ND3007_group, f__Butyricicoccaceae_Unclassified, UCG-005, and Christensenellaceae_R-7_group. Additionally, the propionic acid in feces was negatively correlated with Roseburia.
Regarding flaxseed oil exerted anti-hepatocellular carcinoma effect against orthotopic hepatocellular carcinoma mice (18), the acetic acid in feces was negatively correlated with Eubacterium brachy_group. Meanwhile, the butyric acid, isobutyric acid, propionic acid, and isovaleric acid in feces were negatively correlated with Lactococcus. In addition, the valeric acid in feces was negatively correlated with Eubacterium brachy_group, Lactococcus, and Parabacteroides. As flaxseed oil exhibited anti-atherosclerosis action on TMAO-induced ApoE−/− mice (57), the acetic acid in feces was positively correlated with Alistipes, Bifidobacterium, Odoribacter, Parasutterella, and norank Bacteroidales S24-7, and negatively correlated with seven bacteria (Blautia, Clostridium_sensu_stricto_1, Lactococcus, Romboutsia, Roseburia, etc.). The propionic acid in feces was positively correlated with six bacteria (Alistipes, Bifidobacterium, Coriobacteriaceae UCG-002, Odoribacter, Parasutterella, etc.), and negatively correlated with 15 bacteria (Anaerotruncus, Bilophila, Blautia, Clostridium_sensu_stricto_1, Lachnoclostridium, etc.). The butyric acid in feces was positively correlated with Alistipes, Desulfovibrio, Lactobacillus, and Odoribacter, and negatively correlated with six bacteria (Akkermansia, Bilophila, Blautia, Coriobacteriaceae UCG-002, Faecalibaculum, etc.). The valeric acid in feces was positively correlated with six bacteria (Alistipes, Desulfovibrio, Enterorhabdus, Lactobacillus, Odoribacter, etc.), and negatively correlated with Akkermansia, Blautia, Clostridium_sensu_stricto_1, Faecalibaculum, and Roseburia. The total SCFAs in feces were positively correlated with Alistipes, Bifidobacterium, Odoribacter, Parasutterella, and norank Bacteroidales S24-7, and negatively correlated with 12 bacteria (Anaerotruncus, Blautia, Clostridium_sensu_stricto_1, Lachnoclostridium, Lactococcus, etc.). On the other hand, for flaxseed oil revealed anti-atherosclerosis action on high-fat diet-induced ApoE−/− mice (58), the acetic acid and propionic acid in feces were negatively correlated with seven bacteria (Intestinimonas, Oscillibacter, Lachnoclostridium, Bilophila, Enterorhabdus, etc.). The isobutyric acid in feces was negatively correlated with Intestinimonas, Oscillibacter, Blautia, and Bilophila. The isovaleric acid in feces was negatively correlated with Intestinimonas, Blautia, Bilophila, Enterorhabdus, and Negativibacillus. The valeric acid in feces was negatively correlated with eight bacteria (Intestinimonas, Oscillibacter, Blautia, Lachnoclostridium, Bilophila, etc.). For flaxseed oil displayed ameliorating polycystic ovary syndrome activity in letrozole-induced rats (61), the acetic acid in feces was positively correlated with Lactobacillus, Butyrivibrio, Desulfovibrio, and Bifidobacterium, and negatively correlated with Firmicutes/Bacteroidetes ratio. The propionic acid in feces was positively correlated with Lactobacillus, Butyrivibrio, and Desulfovibrio, and negatively correlated with Firmicutes/Bacteroidetes ratio. The butyric acid in feces was positively correlated with Lactobacillus, Allobaculum, Butyrivibrio, and Desulfovibrio, and negatively correlated with Actinobacteria and Bacteroides. The valeric acid in feces was positively correlated with Butyrivibrio, and negatively correlated with Actinobacteria and Bacteroides.
For sea buckthorn seed oil exerted hypocholesterolemic effect against high-cholesterol diet-induced hamsters (69), the acetic acid, propionic acid, valeric acid, and total SCFAs in feces were positively correlated with Parasutterella and Ruminococcaceae_UCG-014, and negatively correlated with Acietatifactor, Lactobacillus, and [Eubacterium]_coprostanoligenes_group. Meanwhile, the acetic acid was negatively correlated with Anaerovorax and Butyricimonas, the propionic acid was negatively correlated with unclassified_f_Ruminococcaceae and Ruminococcaceae_UCG-009, the valeric acid was negatively correlated with unclassified_f_Ruminococcaceae, Coriobacteriaceae_UCG-002, Butyricimonas, and Anaerovorax, and the total SCFAs was negatively correlated with unclassified_f_Ruminococcaceae, Coriobacteriaceae_UCG-002, and Butyricimonas. Moreover, the butyric acid in feces was positively correlated with Parasutterella, and negatively correlated with unclassified_f_Ruminococcaceae, [Eubacterium]_coprostanoligenes_group, Lactobacillus, Butyricimonas, and Acietatifactor. As tomato seed oil exhibited anti-hyperlipidemia activity in high-fat diet-induced mice (94), the acetic acid in feces was positively correlated with Allobaculum, Bifidobacterium, norank_f_Erysipelotrichaceae and unclassified_f_Erysipelotrichaceae, and negatively correlated with Anaerotruncus, Lachnospiraceae_NK4A136_group, Odoribacter, and [Eubacterium]_fissicatena_group. The propionic acid was positively correlated with unclassified_f_Erysipelotrichaceae, and negatively correlated with Lactobacillus, norank_f_Clostridiales_vadinBB60_group, and norank_f_Peptococcaceae. The butyric acid was positively correlated with Ruminiclostridium_5, and negatively correlated with norank_o_Gastranaerophilales. The valeric acid was positively correlated with Bifidobacterium, Pseudomonas, and norank_f_Peptococcaceae, and negatively correlated with [Eubacterium]_fissicatena_group. The total SCFAs were positively correlated with Allobaculum, Bifidobacterium, norank_f_Erysipelotrichaceae, and unclassified_f_Erysipelotrichaceae, and negatively correlated with Anaerotruncus. Among them, Lactobacillus and norank_o_Gastranaerophilales were two differential gut microbiota biomarkers for tomato seed oil. Regarding Decaisnea insignis seed oil displayed hepatoprotection on L-carnitine-induced mice (83), the acetic acid in feces was positively correlated with Alistipes. The propionic acid was negatively correlated with eight bacteria (norank_f_Erysipelotrichaceae, Turicibacter, [Eubacterium]_fissicatena_group, Clostridium_sensu_stricto_1, Faecalibaculum, etc.). The butyric acid was positively correlated with 13 bacteria (unclassified_o_Bacteroidales, norank_o_Mollicutes_RF9, Ruminococcus_1, [Eubacterium]_xylanophilum_group, [Eubacterium]_fissicatena_group, etc.), and negatively correlated with eight bacteria (Alistipes, Lachnospiraceae_NK4A136_group, Odoribacter, Rikenella, Ruminiclostridium, etc.). The isovaleric acid was negatively correlated with norank_f_Ruminococcaceae, Helicobacter, and Clostridium_sensu_stricto_1. The valeric acid was positively correlated with Ruminiclostridium and Prevotellacee_NK3B31_group. For wild melon seeds oil showed hypocholesterolemic effect against high-cholesterol diet-induced hamsters (93), the acetic acid, propionic acid, butyric acid, valeric acid, and total SCFAs in feces were positively correlated with unclassified_p_Firmicutes, nonrank_f_Eubacteriaceae, norank_f__Clostridiales_vadinBB60_group and Ruminococcus_2, and negatively correlated with norank_f_Ruminococcaceae and Escherichia-shigella. In addition, the acetic acid was positively correlated with norank_o_Mollicutes_RF9, Rikenellaceae_RC9_gut_group and Bifidobacterium, and negatively correlated with norank_f_Lachnospiraceae and Ruminiclostridium. The propionic acid was positively correlated with Parasutterella and Bifidobacterium, and negatively correlated with norank_f_Lachnospiraceae. The butyric acid was positively correlated with Parasutterella and Bifidobacterium, and negatively correlated with Coriobacteriaceae_UCG-002 and Blautia. The valeric acid was positively correlated with norank_o_Mollicutes_RF9, Parasutterella, and Candidatus_Saccharimonas. The total SCFAs were positively correlated with Parasutterella and Bifidobacterium and negatively correlated with norank_f_Lachnospiraceae and Blautia. For Torreya grandis oil exhibited anti-Alzheimer’s disease activity on scopolamine-induced mice, the acetic acid in serum was positively correlated with Allobaculum, Parasutterella, and Bifidobacterium (89). For peony seed oil revealed hypocholesterolemic effect toward high-cholesterol diet-induced hamsters (80), the acetic acid and propionic acid were positively correlated with unclassifed_f_Erysipelotrichaceae and Bifidobacterium, and negatively correlated with Ruminiclostridium_9 and norank_o_Gastranaerophilales. In addition, the acetic acid was positively correlated with Anaeroplasm, and negatively correlated with Papillibacter. The butyric acid and valeric acid were positively correlated with Ruminococcus_2 and Bifidobacterium, and the valeric acid was negatively correlated with norank_o_Gastranaerophilales and Butyricimonas. The total SCFAs were positively correlated with unclassifed_f_Erysipelotrichaceae and Bifidobacterium, and negatively correlated with norank_o_Gastranaerophilales and Butyricimonas. For hawthorn seed oil showed hypocholesterolemic effect on high-cholesterol diet-induced hamsters (96), the acetic acid, propionic acid, butyric acid, valeric acid, and total SCFAs in feces were positively correlated with Ruminococcus_2, and negatively correlated with eight bacteria (Ruminiclostridium_9, norank_f_Ruminococcaceae, Desulfovibrio, Butyricimonas, Oscillibacter, etc.). In addition, the acetic acid was negatively correlated with Lachnospiraceae_NK4A136_group, Blautia, and Ruminiclostridium_5. The propionic acid was positively correlated with Faecalibaculum and Anaeroplasm, and negatively correlated with eight bacteria (Lachnospiraceae_NK4A136_group, Peptococcus, norank_o_Gastranaerophilales, Ruminiclostridium, Ruminococcaceae_NK4A214_group, etc.). The butyric acid was positively correlated with Alistipes and Faecalibaculum, and negatively correlated with norank_o_Gastranaerophilales, Ruminococcaceae_NK4A214_group, Peptococcus, Roseburia, and unclassified_f_Christensenellaceae. The valeric acid was positively correlated with Faecalibaculum, and negatively correlated with norank_o_Gastranaerophilales, Ruminococcaceae_NK4A214_group, Peptococcus, Roseburia, and unclassified_f_Christensenellaceae. The total SCFAs were positively correlated with Faecalibaculum and Anaeroplasm, and negatively correlated with seven bacteria (Lachnospiraceae_NK4A136_group, norank_o_Gastranaerophilales, Ruminiclostridium, Peptococcus, Blautia, etc.).
5.3.2 Gut microbiota-derived metabolites and gut microbiota
For camellia oil exhibited gastroprotection on ethanol-induced mice (41), close correlations were found between metabolites and nine bacterial taxa (Rhodobacter, Bosea, Bacteroides, Dorea, Streptococcus, etc.) in feces. Among them, 40 metabolites (5,6-dimethylbenzimidazole, vincamine, gingerenone, amino acid, etc.) were negatively associated with Rhodobacter, Bosea, Bacteroides, and Dorea. Among them, Bosea and Dorea were two of differential gut microbiota biomarkers for camellia oil. Regarding the anti-diabetes effect of olive oil on NOD/LtJ mice (48), the majority of upregulated serum lipid metabolites were positively correlated with seven types of bacteria (Verrucomicrobia, Akkermansia, Cyanobacteria, Bacteroides, Gastranaerophilales, etc.), and negatively correlated with Lachnospira and Eubacterium_xylanophilum_group. Conversely, most decreased serum lipid metabolites were positively correlated with Lachnospira and Eubacterium_xylanophilum_group, and negatively correlated with Lachnoclostridium, Cyanobacteria, Gastranaerophilales, Bacteroides, and Ruminococcaceae_UCG-005. Among them, Akkermansia, Bacteroides, Gastranaerophilales, Lachnoclostridium, Ruminococcaceae_UCG-005, and Eubacterium_xylanophilum_group were differential gut microbiota biomarkers for olive oil. As flaxseed oil exerted anti-atherosclerosis effect against high-fat diet-induced ApoE−/− mice (55), the BAs included LCA, ACA, GCA, and TCA were negatively correlated with seven bacteria (Intestinimonas, Bilophila, Anaerotruncus, Oscillibacter, Negativibacillus, etc.), and alloLCA, isoLCA, 7-ketolca, β-UDCA, CDCA, HDCA, and α-MCA were positively correlated with these bacteria. For flaxseed oil showed regulation of lipid metabolism activity on high-fat diet-induced mice (51), 12-DHCA, TDCA, and DCA were closely correlated with the most bacterial genera, and the most metabolites were positively correlated with Coprobacillus, Adelercreutzia, and Ruminococcus. Among them, Ruminococcus was one of the differential gut microbiota biomarkers for flaxseed oil. For flaxseed oil revealed regulation of lipid metabolism action on Albas cashmere goats (53), significant correlations were observed between blood fatty acid composition and duodenal, ileal, jejunal, cecal, and colonic differential bacteria. Especially, three fatty acids, including c18:3n3, C20:4n6, and c20:5n3, were significantly correlated with differential bacteria ([Eubacterium]_coprostanoligenes_group, Acetitomaculum, Anaerofustis, Brevibacillus, Denitrobacterium, etc.) in duodenum, jejunum, ileum, cecum, and colon.
For sea buckthorn seed oil exerted hypocholesterolemic effect toward high-cholesterol diet-induced hamsters (69), total neutral sterols, coprostanol, coprostanone, cholesterol, dihydrocholesterol, total acidic sterols, lithocholic acid, deoxycholic acid, chenodeoxycholic acid, and cholic acid were significantly correlated with 19 key genera bacteria (norank_f_Bacteroidales_S24-7_group, Acietatifactor, Allobaculum, norank_o_Mollicutes_RF9, Lactobacillus, etc.). At the same time, peony seed oil exhibited hypocholesterolemic effect on high-cholesterol diet-induced hamsters (80), these metabolites were closely related to 27 specific genera bacteria (Allobaculum, Anaeroplasm, Bifidobacterium, Butyricimonas, unclassified_f_Ruminococcaceae, etc.). For hawthorn seed oil showed hypocholesterolemic effect on high-cholesterol diet-induced hamsters (96), these metabolites were obviously correlated with 30 specific genera bacteria (Faecalibaculum, Ruminococcus_2, Peptococcus, unclassifed_f_Christensenellaceae, norank_o_Gastranaerophilales, etc.). For wild melon seeds oil exhibited hypocholesterolemic effect against high-cholesterol diet-induced hamsters (93), these metabolites were notably correlated with 26 specific genera of bacteria (Bifidobacterium, Ruminococcus_2, nonrank_f_Eubacteriaceae, Bilophila, Blautia, etc.). On the other hand, for peony seed oil exerted alleviate hyperlipidemia and hyperglycemia action on high-fat diet-induced mice (81), 12 metabolites (adenosine, choline, β-asarone, glycitein, betaine, etc.) were notably correlated with 12 bacteria (Lactobacillus, Bifidobacterium, Parabacteroides, Coprococcus, Paraprevotella, etc.). For Decaisnea insignis seed oil displayed hepatoprotection against alcohol-induced mice (82), 25 metabolites (asparagine, L-kynurenine, chlorogenic acid, sarcosine, synephrine, etc.) were obviously correlated with 15 bacteria (Desulforibrio, [Eubacterium]_coprostandigenes_group, Akkermansia, Ruminoccaceae_UCG_004, Streptococcus, etc.). For sacha inchi oil reflected hypolipidemic effect on high-fat diet-induced rats (84), significant corrections were found between the primary bile acids (CA, GCA, TCDCA, and TCA) in the metabolisms of glycerolipid and glycerophospholipid and the differential bacteria (Roseburia, Turicibacter, Butyrivibrio, Escherichia, Bacteroides, etc.). For tomato seed oil showed anti-hyperlipidemia activity on high-fat diet-induced mice (94), fecal bile acids (SFA, MUFA, PUFA, and total FA) were closely related to Lactobacillus, Odoribacter, Rikenella, norank_f__Clostridiales_vadinBB60_group and/or [Eubacterium]_fissicatena_group. Among them, Lactobacillus was one of the differential gut microbiota biomarkers for tomato seed oil.
5.4 Correlations between altered metabolites and changed biochemical indexes
In terms of SCFAs, for flaxseed oil exerted anti-diabetes action on streptozotocin-nicotinamide-induced rats (55), the acetic acid was negatively correlated with TNF-α, IL-1β, IL-6, and IL-17A. Meanwhile, the propionic acid and butyric acid were negatively correlated with IL-1β, IL-6, and IL-17A. For flaxseed oil exhibited anti-atherosclerosis activity in high-fat diet-induced ApoE−/− mice (58), the acetic acid and propionic acid were negatively correlated with LPS, TNF-α, IL-1β, and IL-17A in plasma and with TNF-α, IL-1β, and IL-6 in the aorta. The isobutyric acid was positively correlated with IL-10 in the aorta, and negatively correlated with TNF-α and IL-1β in plasma and with TNF-α and IL-6 in the aorta. The isovaleric acid was negatively correlated with TNF-α and IL-1β in plasma and with IL-1β in the aorta. The valeric acid was positively correlated with IL-10 in the aorta, and negatively correlated with LPS, TNF-α, IL-1β, and IL-17A in plasma and with TNF-α, IL-1β, and IL-6 in the aorta.
Regarding other gut microbiota-derived metabolites, olive oil showed anti-diabetes effect on NOD/LtJ mice (48), the increased serum metabolites were positively correlated with islet number, function, and glycemic control, while were negatively correlated with inflammation and cell immunity. For instance, madecassic acid exhibited a positive correlation with islet number but a negative correlation with insulitis, Th1/Th2, MLN_IL-6, AUC area of OGTT and Random blood glucose_15W. Unsaturated fatty acids such as linoleic acid, erucic acid, nervonic acid, and eicosenoic acid were negatively correlated with insulitis and the AUC area of OGTT. Conversely, those decreased lipids, such as oleamide, methyllycaconitine, 5-aminovaleric acid betaine, and ginsenoside f3, were mainly positively correlated with colon_IL-6, insulitis, and AUC area of OGTT. For flaxseed oil exhibited anti-atherosclerosis activity on high-fat diet-induced ApoE−/− mice (58), alloLCA and isoLCA were positively correlated with LPS in plasma, and CDCA and HDCA were positively correlated with TNF-α in aorta. LCA was negatively correlated with IL-1β and IL-6 in the aorta. 7-ketoLCA was positively correlated with LPS in plasma and TNF-α in the aorta. β-UDCA was positively correlated with IL-1β and IL-17A in plasma and aorta. α-MCA was positively correlated with IL-17A in plasma and with IL-1β and IL-17A in the aorta. ACA was negatively correlated with LPS and TNF-α in plasma. GCA was negatively correlated with LPS, TNF-α, and IL-17A in plasma, and with TNF-α in the aorta. TCA was negatively correlated with LPS, TNF-α, and IL-1β in plasma, and with TNF-α and IL-1β in the aorta. For sacha inchi oil exerted hypolipidemic effect against high-fat diet-induced rats (84), CA was positively correlated with Cyp7a1 and Hsd3b7, and negatively correlated with Amacr, Slc27a5, Slc10a1, and FXR. GCA was negatively correlated with Slc27a5, Slc10a1, and Abcc2. TCDCA was negatively correlated with Amacr, Slc27a5, and Slc10a1. TCA was negatively correlated with Slc27a5 and Slc10a1. Meanwhile, lipids (TAG (16:0/16:0/22:4), DG (18:1/20:0), LysoPC (18:2), PC (18:0/20:4), PE (18,1/22:4), etc.) involved in the metabolisms of glycerolipid and glycerophospholipid were significantly correlated with differential genes (Pemt, Crls1, Lipc, Acadm, Scd2, etc.).
6 Conclusion and prospects
Many edible plant oils (especially camellia oil, olive oil, and flaxseed oil) could modulate gut microbiota during their health-promoting effects. Moreover, the modulated gut microbiota was significantly correlated with the changed biochemical indexes. Furthermore, the altered metabolites by edible plant oils were obviously correlated with the modulated gut microbiota and changed biochemical indexes. Overall, it could be speculated that edible plant oils modulate gut microbiota to alter SCFAs and other gut microbiota-derived metabolites, thereby changing biochemical indexes to exhibit health-promoting effects (Figure 5). This review provides theoretical basis for understanding and future research in health-promoting effects of edible plant oils from the perspective of gut microbiota modulation.
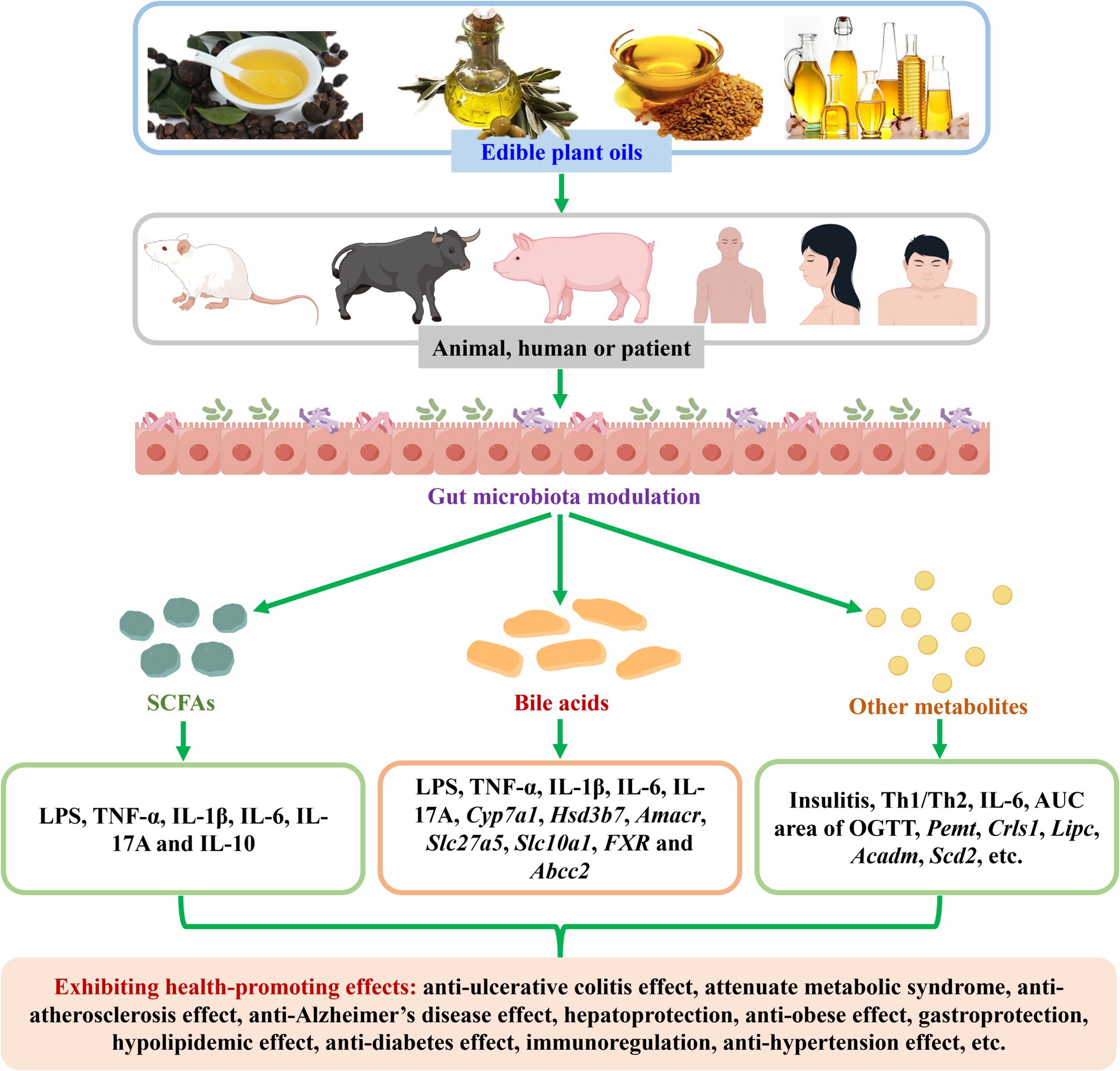
Figure 5. The speculation of “edible plant oils→gut microbiota→metabolites→biochemical indexes→health-promoting effects”.
There are still some questions that remain to be solved: (i) the interactions between edible plant oils and gut microbiota have been rarely studied, and the key bioactive nutrients for edible plant oils in modulating gut microbiota during their health-promoting effects have not been well-ascertained; (ii) which gut microbiota and metabolites are exactly important for edible plant oils in exerting health-promoting effects, and how the screened differential gut microbiota biomarkers along with the altered metabolites mediate the health-promoting effects of edible plant oils, are unknown; (iii) the mechanisms of edible plant oils in modulating gut microbiota, and gut microbiota altering metabolites as well as metabolites changed biochemical indexes have not been investigated; (iv) cohort or clinical studies and the related specific mechanisms concerning gut microbiota modulation of edible plant oils have been rarely uncovered, and the relevant existing researches were absence of longitudinal data.
Author contributions
QZ: Funding acquisition, Investigation, Visualization, Writing – original draft. A-QC: Investigation, Visualization, Writing – original draft. JH: Investigation, Visualization, Writing – original draft. MW: Investigation, Writing – original draft. J-HL: Project administration, Writing – review & editing. AW: Investigation, Writing – original draft. X-YW: Conceptualization, Funding acquisition, Project administration, Supervision, Writing – review & editing.
Funding
The author(s) declare that financial support was received for the research, authorship, and/or publication of this article. This study was financially supported by the Open Project of Key Laboratory of Prevention and Treatment of Cardiovascular and Cerebrovascular Diseases, Ministry of Education (XN202001); Jiangxi Provincial Natural Science Foundation (20232BAB215062 and 20202BABL216081); the Science and Technology Research Project of Jiangxi Provincial Education Department (GJJ190805); and University-Level Scientific Research Projects of Gannan Medical University (QD201913, QD202128, TD202230, and TD2022GR04).
Conflict of interest
The authors declare that the research was conducted in the absence of any commercial or financial relationships that could be construed as a potential conflict of interest.
Publisher’s note
All claims expressed in this article are solely those of the authors and do not necessarily represent those of their affiliated organizations, or those of the publisher, the editors and the reviewers. Any product that may be evaluated in this article, or claim that may be made by its manufacturer, is not guaranteed or endorsed by the publisher.
References
1. Zhou, XR, Liu, Q, and Singh, S. Engineering nutritionally improved edible plant oils. Annu Rev Food Sci Technol. (2023) 14:247–69. doi: 10.1146/annurev-food-052720-104852
2. Zhou, Y, Zhao, WW, Lai, Y, Zhang, BH, and Zhang, DQ. Edible plant oil: global status, health issues, and perspectives. Front Plant Sci. (2020) 11:1315. doi: 10.3389/fpls.2020.01315
3. Wen, CT, Shen, MY, Liu, GY, Liu, XF, Liang, L, Li, YD, et al. Edible vegetable oils from oil crops: preparation, refining, authenticity identification and application. Process Biochem. (2023) 124:168–79. doi: 10.1016/j.procbio.2022.11.017
4. Tian, MK, Bai, YC, Tian, HY, and Zhao, XB. The chemical composition and health-promoting benefits of vegetable oils-a review. Molecules. (2023) 28:6393. doi: 10.3390/molecules28176393
5. Lanza, B, and Ninfali, P. Antioxidants in extra virgin olive oil and table olives: connections between agriculture and processing for health choices. Antioxidants. (2020) 9:41. doi: 10.3390/antiox9010041
6. Zhang, T, Qiu, FC, Chen, L, Liu, RJ, Chang, M, and Wang, XG. Identification and in vitro anti-inflammatory activity of different forms of phenolic compounds in Camellia oleifera oil. Food Chem. (2021) 344:128660. doi: 10.1016/j.foodchem.2020.128660
7. Liu, HC, Zhu, HJ, Xia, H, Yang, X, Yang, LG, Wang, SK, et al. Different effects of high-fat diets rich in different oils on lipids metabolism, oxidative stress and gut microbiota. Food Res Int. (2021) 141:110078. doi: 10.1016/j.foodres.2020.110078
8. Wu, YH, Chen, SY, Yang, JY, Chen, YY, and Yen, GC. Gac (Momordica cochinchinensis Spreng.) oil supplementation mitigates cognitive decline in AlCl3-induced rats through the regulation of Alzheimer's disease markers and autophagy via gut-brain communication. J Funct Foods. (2023) 110:105860. doi: 10.1016/j.jff.2023.105860
9. Moral, R, and Escrich, E. Influence of olive oil and its components on breast cancer: molecular mechanisms. Molecules. (2022) 27:477. doi: 10.3390/molecules27020477
10. Qin, PJ, Shen, JJ, Wei, JG, and Chen, YQ. A critical review of the bioactive ingredients and biological functions of camellia oleifera oil. Curr Res Food Sci. (2024) 8:100753. doi: 10.1016/j.crfs.2024.100753
11. Bilal, RM, Liu, CJ, Zhao, HH, Wang, YZ, Farag, MR, Alagawany, M, et al. Olive oil: nutritional applications, beneficial health aspects and its prospective application in poultry production. Front Pharmacol. (2021) 12:723040. doi: 10.3389/fphar.2021.723040
12. Yang, J, Wen, CT, Duan, YQ, Deng, QC, Peng, DF, Zhang, HH, et al. The composition, extraction, analysis, bioactivities, bioavailability and applications in food system of flaxseed (Linum usitatissimum L.) oil: a review. Trends Food Sci Technol. (2021) 118:252–60. doi: 10.1016/j.tifs.2021.09.025
13. Deen, A, Visvanathan, R, Wickramarachchi, D, Marikkar, N, Nammi, S, Jayawardana, BC, et al. Chemical composition and health benefits of coconut oil: an overview. J Sci Food Agr. (2021) 101:2182–93. doi: 10.1002/jsfa.10870
14. Hsu, CL, and Schnabl, B. The gut-liver axis and gut microbiota in health and liver disease. Nat Rev Microbiol. (2023) 21:719–33. doi: 10.1038/s41579-023-00904-3
15. Perler, BK, Friedman, ES, and Wu, GD. The role of the gut microbiota in the relationship between diet and human health. Annu Rev Physiol. (2023) 85:449–68. doi: 10.1146/annurev-physiol-031522-092054
16. Huang, SY, Sun, HY, Lin, D, Huang, XJ, Chen, RR, Li, ML, et al. Camellia oil exhibits anti-fatigue property by modulating antioxidant capacity, muscle fiber, and gut microbial composition in mice. J Food Sci. (2024) 89:2465–81. doi: 10.1111/1750-3841.16983
17. de Souza, DM, Cavalcante, HC, Dos Santos Lima, M, Alves, AF, da Veiga Dutra, ML, D'Oliveira, AB, et al. Intermittent fasting associated with coconut oil (Cocos nucifera L.) alters gut-liver axis parameters in diet-induced obese rats. Nutrition. (2024) 121:112370. doi: 10.1016/j.nut.2024.112370
18. Liu, J, Li, YW, Shen, WK, Wang, T, Liu, YY, Ma, JB, et al. Dietary supplementation of α-linolenic acid-rich flaxseed oil enhances anti-PD-1 protection against orthotopic hepatocellular carcinoma by reshaping gut homeostasis and improving anti-tumor immunity via gut-liver axis in mice. J Funct Foods. (2024) 116:106157. doi: 10.1016/j.jff.2024.106157
19. Luisi, MLE, Lucarini, L, Biffi, B, Rafanelli, E, Pietramellara, G, Durante, M, et al. Effect of Mediterranean diet enriched in high quality extra virgin olive oil on oxidative stress, inflammation and gut microbiota in obese and normal weight adult subjects. Front Pharmacol. (2019) 10:1366. doi: 10.3389/fphar.2019.01366
20. Pu, S, Khazanehei, H, Jones, PJ, and Khafipour, E. Interactions between obesity status and dietary intake of monounsaturated and polyunsaturated oils on human gut microbiome profiles in the canola oil multicenter intervention trial (COMIT). Front Microbiol. (2016) 7:1612. doi: 10.3389/fmicb.2016.01612
21. Kawamura, A, and Sugita, M. Perilla oil, an omega-3 unsaturated fatty acid-rich oil, enhances diversity of gut microbiota and may relieve constipation in sedentary healthy female: a nonrandomized placebo-controlled pilot study. Die Dermatol. (2023) 2:191–202. doi: 10.3390/dietetics2020015
22. Yang, RN, Zhang, LX, Li, PW, Yu, L, Mao, J, Wang, XP, et al. A review of chemical composition and nutritional properties of minor vegetable oils in China. Trends Food Sci Technol. (2018) 74:26–32. doi: 10.1016/j.tifs.2018.01.013
23. Ye, Z, Xu, YJ, and Liu, YF. Influences of dietary oils and fats, and the accompanied minor content of components on the gut microbiota and gut inflammation: a review. Trends Food Sci Technol. (2021) 113:255–76. doi: 10.1016/j.tifs.2021.05.001
24. Millman, JF, Okamoto, S, Teruya, T, Uema, T, Ikematsu, S, Shimabukuro, M, et al. Extra-virgin olive oil and the gut-brain axis: influence on gut microbiota, mucosal immunity, and cardiometabolic and cognitive health. Nutr Rev. (2021) 79:1362–74. doi: 10.1093/nutrit/nuaa148
25. Gavahian, M, Khaneghah, AM, Lorenzo, JM, Munekata, PE, Garcia-Mantrana, I, Collado, MC, et al. Health benefits of olive oil and its components: impacts on gut microbiota antioxidant activities, and prevention of noncommunicable diseases. Trends Food Sci Technol. (2019) 88:220–7. doi: 10.1016/j.tifs.2019.03.008
26. Gao, L, Jin, LH, Liu, QN, Zhao, KX, Lin, L, Zheng, JY, et al. Recent advances in the extraction, composition analysis and bioactivity of Camellia (Camellia oleifera Abel.) oil. Trends Food Sci Technol. (2023) 143:104211. doi: 10.1016/j.tifs.2023.104211
27. Zhang, F, Zhu, F, Chen, BL, Su, EZ, Chen, YZ, and Cao, FL. Composition, bioactive substances, extraction technologies and the influences on characteristics of Camellia oleifera oil: a review. Food Res Int. (2022) 156:111159. doi: 10.1016/j.foodres.2022.111159
28. Visioli, F, Davalos, A, de Las, L, Hazas, MC, Crespo, MC, and Tomé-Carneiro, J. An overview of the pharmacology of olive oil and its active ingredients. Br J Pharmacol. (2020) 177:1316–30. doi: 10.1111/bph.14782
29. Gorzynik-Debicka, M, Przychodzen, P, Cappello, F, Kuban-Jankowska, A, Marino Gammazza, A, Knap, N, et al. Potential health benefits of olive oil and plant polyphenols. Int J Mol Sci. (2018) 19:686. doi: 10.3390/ijms19030686
30. Tang, ZX, Ying, RF, Lv, BF, Yang, LH, Xu, Z, Yan, LQ, et al. Flaxseed oil: extraction, health benefits and products. Qual Assur Saf Crop. (2021) 13:1–19. doi: 10.15586/qas.v13i1.783
31. Wu, CC, Tung, YT, Chen, SY, Lee, WT, Lin, HT, and Yen, GC. Anti-inflammatory, antioxidant, and microbiota-modulating effects of camellia oil from Camellia brevistyla on acetic acid-induced colitis in rats. Antioxidants. (2020) 9:58. doi: 10.3390/antiox9010058
32. Lee, WT, Tung, YT, Wu, CC, Tu, PS, and Yen, GC. Camellia oil (Camellia oleifera Abel.) modifies the composition of gut microbiota and alleviates acetic acid-induced colitis in rats. J Agric Food Chem. (2018) 66:7384–92. doi: 10.1021/acs.jafc.8b02166
33. Jiang, QH, Jiang, CK, Lu, HL, Zhou, TY, Hu, WJ, Tan, CP, et al. Camellia oil alleviates DSS-induced colitis in mice by regulating the abundance of intestinal flora and suppressing the NF-κB signaling pathway. J Funct Foods. (2023) 108:105777. doi: 10.1016/j.jff.2023.105777
34. Weng, MH, Chen, SY, Li, ZY, and Yen, GC. Camellia oil alleviates the progression of Alzheimer's disease in aluminum chloride-treated rats. Free Radic Biol Med. (2020) 152:411–21. doi: 10.1016/j.freeradbiomed.2020.04.004
35. Guo, PL, Zeng, MN, Cao, B, Liu, M, Zhang, YH, Jia, JF, et al. Camellia oil improves Aβ25-35-induced memory impairment by regulating the composition of the gut microbiota and lipid metabolism in mice. J Funct Foods. (2022) 96:105214. doi: 10.1016/j.jff.2022.105214
36. Chen, SY, Weng, MH, Li, ZY, Wang, GY, and Yen, GC. Protective effects of camellia and olive oils against cognitive impairment via gut microbiota-brain communication in rats. Food Funct. (2022) 13:7168–80. doi: 10.1039/d1fo04418d
37. Huang, TY, Zhou, WK, Ma, XG, Jiang, JH, Zhang, FA, Zhou, W, et al. Oral administration of camellia oil ameliorates obesity and modifies the gut microbiota composition in mice fed a high-fat diet. FEMS Microbiol Lett. (2021) 368:fnab063. doi: 10.1093/femsle/fnab063
38. Waleed, ASA, Huang, TC, Deng, LL, Han, M, Zhao, Y, and Geng, Z. Perilla, sunflower, and tea seed oils as potential dietary supplements with anti-obesity effects by modulating the gut microbiota composition in mice fed a high-fat diet. Eur J Nutr. (2023) 62:2509–25. doi: 10.1007/s00394-023-03155-3
39. Gao, J, Ma, L, Yin, J, Liu, G, Ma, J, Xia, ST, et al. Camellia (Camellia oleifera bel.) seed oil reprograms gut microbiota and alleviates lipid accumulation in high fat-fed mice through the mTOR pathway. Food Funct. (2022) 13:4977–92. doi: 10.1039/d1fo04075h
40. Huang, TY, Jiang, JH, Cao, YJ, Huang, JZ, Zhang, FA, and Cui, GZ. Camellia oil (Camellia oleifera Abel.) treatment improves high-fat diet-induced atherosclerosis in apolipoprotein E (ApoE) −/− mice. Biosci Microbiota Food Health. (2023) 42:56–64. doi: 10.12938/bmfh.2022-005
41. Xu, ZX, Zhang, HM, Yang, YZ, Ma, XY, and Yang, C. Camellia oil (Camellia oleifera Abel.) alleviates gastric injury induced by ethanol associated with modulation of gut microbiota in mice. Oil Crop Sci. (2023) 8:61–71. doi: 10.1016/j.ocsci.2023.02.006
42. Guo, R, Zhu, JY, Chen, L, Li, JM, Ding, QC, Han, Q, et al. Dietary camellia seed oil attenuates liver injury in mice chronically exposed to alcohol. Front Nutr. (2022) 9:1026740. doi: 10.3389/fnut.2022.1026740
43. Zhao, ZH, Shi, AM, Wang, Q, and Zhou, JR. High oleic acid peanut oil and extra virgin olive oil supplementation attenuate metabolic syndrome in rats by modulating the gut microbiota. Nutrients. (2019) 11:3005. doi: 10.3390/nu11123005
44. Prieto, I, Hidalgo, M, Segarra, AB, Martínez-Rodríguez, AM, Cobo, A, Ramírez, M, et al. Influence of a diet enriched with virgin olive oil or butter on mouse gut microbiota and its correlation to physiological and biochemical parameters related to metabolic syndrome. PLoS One. (2018) 13:e0190368. doi: 10.1371/journal.pone.0190368
45. Martínez, N, Prieto, I, Hidalgo, M, Segarra, AB, Martínez-Rodríguez, AM, Cobo, A, et al. Refined versus extra virgin olive oil high-fat diet impact on intestinal microbiota of mice and its relation to different physiological variables. Microorganisms. (2019) 7:61. doi: 10.3390/microorganisms7020061
46. Hidalgo, M, Prieto, I, Abriouel, H, Villarejo, AB, Ramírez-Sánchez, M, Cobo, A, et al. Changes in gut microbiota linked to a reduction in systolic blood pressure in spontaneously hypertensive rats fed an extra virgin olive oil-enriched diet. Plant Foods Hum Nutr. (2018) 73:1–6. doi: 10.1007/s11130-017-0650-1
47. Ma, Y, Li, J, Guo, YJ, Ma, LY, Liu, YX, Kuang, HY, et al. Dietary olive oil enhances the oral tolerance of the food allergen ovalbumin in mice by regulating intestinal microecological homeostasis. J Food Biochem. (2022) 46:e14297. doi: 10.1111/jfbc.14297
48. Wang, Y, Shen, YM, Lu, SP, and Wu, J. EVOO supplement prevents type 1 diabetes by modulating gut microbiota and serum metabolites in NOD mice. Life Sci. (2023) 335:122274. doi: 10.1016/j.lfs.2023.122274
49. Olalla, J, García de Lomas, JM, Chueca, N, Pérez-Stachowski, X, De Salazar, A, Del Arco, A, et al. Effect of daily consumption of extra virgin olive oil on the lipid profile and microbiota of HIV-infected patients over 50 years of age. Medicine (Baltimore). (2019) 98:e17528. doi: 10.1097/MD.0000000000017528
50. Millman, J, Okamoto, S, Kimura, A, Uema, T, Higa, M, Yonamine, M, et al. Metabolically and immunologically beneficial impact of extra virgin olive and flaxseed oils on composition of gut microbiota in mice. Eur J Nutr. (2020) 59:2411–25. doi: 10.1007/s00394-019-02088-0
51. Yang, C, Xu, ZX, Huang, QD, Wang, X, Huang, FH, and Deng, QC. Targeted microbiome metabolomics reveals flaxseed oil supplementation regulated the gut microbiota and farnesoid X receptor pathway in high-fat diet mice. Food Sci Hum Wellness. (2023) 12:2324–35. doi: 10.1016/j.fshw.2023.03.036
52. Liu, SL, Wang, X, Li, YH, Shi, BL, Guo, XY, Zhao, YL, et al. Flaxseed oil and heated flaxseed supplements have different effects on lipid deposition and ileal microbiota in albas cashmere goats. Animals. (2021) 11:790. doi: 10.3390/ani11030790
53. Guo, YM, Liu, SL, Li, YH, Guo, XY, Zhao, YL, Shi, BL, et al. Intestinal microbiota community and blood fatty acid profiles of albas cashmere goats fed with flaxseed oil and whole flaxseed. Animals. (2023) 13:3531. doi: 10.3390/ani13223531
54. Zhang, X, Chen, J, Zhou, SM, Jiang, YR, Wang, Y, and Li, Y. The effect of flaxseed oil after deep frying on lipid metabolism and gut barrier homeostasis. Food Res Int. (2024) 175:113728. doi: 10.1016/j.foodres.2023.113728
55. Zhu, LL, Sha, LP, Li, K, Wang, Z, Wang, T, Li, YW, et al. Dietary flaxseed oil rich in omega-3 suppresses severity of type 2 diabetes mellitus via anti-inflammation and modulating gut microbiota in rats. Lipids Health Dis. (2020) 19:20. doi: 10.1186/s12944-019-1167-4
56. Xia, H, Wang, Y, Shi, XL, Liao, W, Wang, SK, Sui, J, et al. Beneficial effects of dietary flaxseed oil through inflammation pathways and gut microbiota in streptozotocin-induced diabetic mice. Food Secur. (2023) 12:3229. doi: 10.3390/foods12173229
57. He, ZY, Hao, WJ, Kwek, E, Lei, L, Liu, JH, Zhu, HY, et al. Fish oil is more potent than flaxseed oil in modulating gut microbiota and reducing trimethylamine-N-oxide-exacerbated atherogenesis. J Agric Food Chem. (2019) 67:13635–47. doi: 10.1021/acs.jafc.9b06753
58. Li, YW, Yu, Z, Liu, YY, Wang, T, Liu, YJ, Bai, ZX, et al. Dietary α-linolenic acid-rich flaxseed oil ameliorates high-fat diet-induced atherosclerosis via gut microbiota-inflammation-artery axis in ApoE−/− mice. Front Cardiovasc Med. (2022) 9:830781. doi: 10.3389/fcvm.2022.830781
59. Zhang, XX, Wang, H, Yin, PP, Fan, H, Sun, LW, and Liu, YJ. Flaxseed oil ameliorates alcoholic liver disease via anti-inflammation and modulating gut microbiota in mice. Lipids Health Dis. (2017) 16:44. doi: 10.1186/s12944-017-0431-8
60. Sun, XY, Yu, JT, Wang, Y, Luo, JM, Zhang, GG, and Peng, XC. Flaxseed oil ameliorates aging in d-galactose induced rats via altering gut microbiota and mitigating oxidative damage. J Sci Food Agric. (2022) 102:6432–42. doi: 10.1002/jsfa.12010
61. Wang, T, Sha, LP, Li, YW, Zhu, LL, Wang, Z, Li, K, et al. Dietary α-linolenic acid-rich flaxseed oil exerts beneficial effects on polycystic ovary syndrome through sex steroid hormones-microbiota-inflammation axis in rats. Front Endocrinol. (2020) 11:284. doi: 10.3389/fendo.2020.00284
62. Che, LQ, Zhou, Q, Liu, Y, Hu, L, Peng, X, Wu, C, et al. Flaxseed oil supplementation improves intestinal function and immunity, associated with altered intestinal microbiome and fatty acid profile in pigs with intrauterine growth retardation. Food Funct. (2019) 10:8149–60. doi: 10.1039/c9fo01877h
63. Zhou, Q, Ma, L, Zhao, WY, Zhao, W, Han, X, Niu, JH, et al. Flaxseed oil alleviates dextran sulphate sodium-induced ulcerative colitis in rats. J Funct Foods. (2020) 64:103602. doi: 10.1016/j.jff.2019.103602
64. Zheng, JY, Kang, T, Jiang, C, Lin, LK, Gao, L, Jin, LH, et al. Gut microbiome and brain transcriptome analyses reveal the effect of walnut oil in preventing scopolamine-induced cognitive impairment. Food Funct. (2023) 14:9707–24. doi: 10.1039/d3fo01893h
65. Miao, FJ, Shan, CL, Ma, T, Geng, SX, and Ning, DL. Walnut oil alleviates DSS-induced colitis in mice by inhibiting NLRP3 inflammasome activation and regulating gut microbiota. Microb Pathog. (2021) 154:104866. doi: 10.1016/j.micpath.2021.104866
66. Korach-Rechtman, H, Rom, O, Mazouz, L, Freilich, S, Jeries, H, Hayek, T, et al. Soybean oil modulates the gut microbiota associated with atherogenic biomarkers. Microorganisms. (2020) 8:486. doi: 10.3390/microorganisms8040486
67. Patrone, V, Minuti, A, Lizier, M, Miragoli, F, Lucchini, F, Trevisi, E, et al. Differential effects of coconut versus soy oil on gut microbiota composition and predicted metabolic function in adult mice. BMC Genomics. (2018) 19:808. doi: 10.1186/s12864-018-5202-z
68. López-Salazar, V, Tapia, MS, Tobón-Cornejo, S, Díaz, D, Alemán-Escondrillas, G, Granados-Portillo, O, et al. Consumption of soybean or olive oil at recommended concentrations increased the intestinal microbiota diversity and insulin sensitivity and prevented fatty liver compared to the effects of coconut oil. J Nutr Biochem. (2021) 94:108751. doi: 10.1016/j.jnutbio.2021.108751
69. Hao, WJ, He, ZY, Zhu, HY, Liu, JH, Kwek, E, Zhao, YM, et al. Sea buckthorn seed oil reduces blood cholesterol and modulates gut microbiota. Food Funct. (2019) 10:5669–81. doi: 10.1039/c9fo01232j
70. Zhang, J, Zhou, HC, He, SB, Zhang, XF, Ling, YH, Li, XY, et al. The immunoenhancement effects of sea buckthorn pulp oil in cyclophosphamide-induced immunosuppressed mice. Food Funct. (2021) 12:7954–63. doi: 10.1039/d1fo01257f
71. Wang, Z, Zhou, SM, and Jiang, YR. Sea buckthorn pulp and seed oils ameliorate lipid metabolism disorders and modulate gut microbiota in C57BL/6J mice on high-fat diet. Front Nutr. (2022) 9:1067813. doi: 10.3389/fnut.2022.1067813
72. Djurasevic, S, Bojic, S, Nikolic, B, Dimkic, I, Todorovic, Z, Djordjevic, J, et al. Beneficial effect of virgin coconut oil on alloxan-induced diabetes and microbiota composition in rats. Plant Foods Hum Nutr. (2018) 73:295–301. doi: 10.1007/s11130-018-0689-7
73. Rolinec, M, Medo, J, Gábor, M, Miluchová, M, Bíro, D, Šimko, M, et al. The effect of coconut oil addition to feed of pigs on rectal microbial diversity and bacterial abundance. Animals. (2020) 10:1764. doi: 10.3390/ani10101764
74. Ahnan, AD, and Agustinah, W. Effect of virgin coconut oil supplementation on obese rats’ anthropometrical parameters and gut Bacteroidetes and Firmicutes change ratio. Cord. (2016) 32:14–4. doi: 10.37833/cord.v32i1.41
75. Araújo de Vasconcelos, MH, Tavares, RL, Dutra, MLDV, Batista, KS, D'Oliveira, AB, Pinheiro, RO, et al. Extra virgin coconut oil (Cocos nucifera L.) intake shows neurobehavioural and intestinal health effects in obesity-induced rats. Food Funct. (2023) 14:6455–69. doi: 10.1039/d3fo00850a
76. Hu, FM, Piao, MY, Yang, CT, Diao, QY, and Tu, Y. Effects of coconut oil and palm oil on growth, rumen microbiota, and fatty acid profile of suckling calves. Microorganisms. (2023) 11:655. doi: 10.3390/microorganisms11030655
77. Kawamura, A, Nemoto, K, and Sugita, M. Effect of 8-week intake of the n-3 fatty acid-rich perilla oil on the gut function and as a fuel source for female athletes: a randomised trial. Br J Nutr. (2022) 129:1–11. doi: 10.1017/S0007114522001805
78. Tian, Y, Wang, HL, Yuan, FH, Li, N, Huang, Q, He, L, et al. Perilla oil has similar protective effects of fish oil on high-fat diet-induced nonalcoholic fatty liver disease and gut dysbiosis. Biomed Res Int. (2016) 2016:9462571. doi: 10.1155/2016/9462571
79. Wang, F, Zhu, HJ, Hu, MY, Wang, J, Xia, H, Yang, X, et al. Perilla oil supplementation improves hypertriglyceridemia and gut dysbiosis in diabetic KKAy mice. Mol Nutr Food Res. (2018) 62:e1800299. doi: 10.1002/mnfr.201800299
80. Kwek, E, Zhu, HY, Ding, HF, He, ZY, Hao, WJ, Liu, JH, et al. Peony seed oil decreases plasma cholesterol and favorably modulates gut microbiota in hypercholesterolemic hamsters. Eur J Nutr. (2022) 61:2341–56. doi: 10.1007/s00394-021-02785-9
81. Liang, ZY, He, YL, Wei, DF, Fu, PX, Li, YY, Wang, H, et al. Tree peony seed oil alleviates hyperlipidemia and hyperglycemia by modulating gut microbiota and metabolites in high-fat diet mice. Food Sci Nutr. (2024) 12:4421–34. doi: 10.1002/fsn3.4108
82. Liu, XX, Zhao, K, Yang, XB, and Zhao, Y. Gut microbiota and metabolome response of Decaisnea insignis seed oil on metabolism disorder induced by excess alcohol consumption. J Agric Food Chem. (2019) 67:10667–77. doi: 10.1021/acs.jafc.9b04792
83. Zhang, XN, Wu, Q, Zhao, Y, and Yang, XB. Decaisnea insignis seed oil inhibits trimethylamine-N-oxide formation and remodels intestinal microbiota to alleviate liver dysfunction in L-carnitine feeding mice. J Agric Food Chem. (2019) 67:13082–92. doi: 10.1021/acs.jafc.9b05383
84. Li, P, Huang, JZ, Xiao, N, Cai, X, Yang, YY, Deng, JW, et al. Sacha inchi oil alleviates gut microbiota dysbiosis and improves hepatic lipid dysmetabolism in high-fat diet-fed rats. Food Funct. (2020) 11:5827–41. doi: 10.1039/d0fo01178a
85. Lai, MY, Liu, YL, Han, XJ, Chen, YH, Liu, B, and Zeng, F. Three different oils improve HFD-induced lipid metabolism disorders via driving gut microbiota. Eur J Lipid Sci Technol. (2024) 126:2300129. doi: 10.1002/ejlt.202300129
86. Yin, RY, Fu, YX, Yousaf, L, Xue, Y, Hu, JR, Hu, XS, et al. Crude and refined millet bran oil alleviate lipid metabolism disorders, oxidative stress and affect the gut microbiota composition in high-fat diet-induced mice. Int J Food Sci Technol. (2022) 57:2600–10. doi: 10.1111/ijfs.15063
87. Phannasorn, W, Pharapirom, A, Thiennimitr, P, Guo, H, Ketnawa, S, and Wongpoomchai, R. Enriched riceberry bran oil exerts chemopreventive properties through anti-inflammation and alteration of gut microbiota in carcinogen-induced liver and colon carcinogenesis in rats. Cancers. (2022) 14:4358. doi: 10.3390/cancers14184358
88. Wang, H, Li, Y, Wang, R, Ji, HF, Lu, CY, and Su, XR. Chinese Torreya grandis cv. Merrillii seed oil affects obesity through accumulation of sciadonic acid and altering the composition of gut microbiota. Food Sci Hum Wellness. (2022) 11:58–67. doi: 10.1016/j.fshw.2021.07.007
89. Ma, JC, Yuan, T, Gao, YQ, Zeng, XM, Liu, ZG, and Gao, JM. Torreya grandis oil attenuates cognitive impairment in scopolamine-induced mice. Food Funct. (2023) 14:10520–34. doi: 10.1039/d3fo03800a
90. Danneskiold-Samsøe, NB, Andersen, D, Radulescu, ID, Normann-Hansen, A, Brejnrod, A, Kragh, M, et al. A safflower oil based high-fat/high-sucrose diet modulates the gut microbiota and liver phospholipid profiles associated with early glucose intolerance in the absence of tissue inflammation. Mol Nutr Food Res. (2017) 61:1600528. doi: 10.1002/mnfr.201600528
91. Qu, LL, Liu, QQ, Zhang, Q, Tuo, XX, Fan, DD, Deng, JJ, et al. Kiwifruit seed oil prevents obesity by regulating inflammation, thermogenesis, and gut microbiota in high-fat diet-induced obese C57BL/6 mice. Food Chem Toxicol. (2019) 125:85–94. doi: 10.1016/j.fct.2018.12.046
92. Zhang, J, Lu, YL, Yang, XB, and Zhao, Y. Supplementation of okra seed oil ameliorates ethanol-induced liver injury and modulates gut microbiota dysbiosis in mice. Food Funct. (2019) 10:6385–98. doi: 10.1039/c9fo00189a
93. Hao, WJ, Zhu, HY, Chen, JN, Kwek, E, He, ZY, Liu, JH, et al. Wild melon seed oil reduces plasma cholesterol and modulates gut microbiota in hypercholesterolemic hamsters. J Agric Food Chem. (2020) 68:2071–81. doi: 10.1021/acs.jafc.9b07302
94. He, WS, Li, LL, Rui, JX, Li, JJ, Sun, YY, Cui, DD, et al. Tomato seed oil attenuates hyperlipidemia and modulates gut microbiota in C57BL/6J mice. Food Funct. (2020) 11:4275–90. doi: 10.1039/d0fo00133c
95. Liu, R, Shu, Y, Qi, WH, Rao, WL, Fu, ZH, Shi, ZX, et al. Protective effects of almond oil on streptozotocin-induced diabetic rats via regulating Nrf2/HO-1 pathway and gut microbiota. J Food Qual. (2021) 2021:1–14. doi: 10.1155/2021/5599219
96. Kwek, E, Yan, C, Ding, HF, Hao, WJ, He, ZY, Liu, JH, et al. Effects of hawthorn seed oil on plasma cholesterol and gut microbiota. Nutr Metab. (2022) 19:55. doi: 10.1186/s12986-022-00690-4
97. Hidalgo, M, Prieto, I, Abriouel, H, Cobo, A, Benomar, N, Gálvez, A, et al. Effect of virgin and refined olive oil consumption on gut microbiota. Comparison to butter. Food Res Int. (2014) 64:553–9. doi: 10.1016/j.foodres.2014.07.030
98. Yan, XB, Huang, WB, Suo, XX, Pan, SM, Li, T, Liu, H, et al. Integrated analysis of microbiome and host transcriptome reveals the damageprotective mechanism of corn oil and olive oil on the gut health of grouper (♀ Epinephelus fuscoguttatus × ♂ E. Lanceolatu). Int J Biol Macromol. (2023) 253:127550. doi: 10.1016/j.ijbiomac.2023.127550
99. Kwek, E, Yan, C, Ding, HF, Hao, WJ, He, ZY, Ma, KY, et al. Effects of thermally-oxidized frying oils (corn oil and lard) on gut microbiota in hamsters. Antioxidants. (2022) 11:1732. doi: 10.3390/antiox11091732
100. Li, H, Zhu, YY, Zhao, F, Song, SX, Li, YQ, Xu, XL, et al. Fish oil, lard and soybean oil differentially shape gut microbiota of middle-aged rats. Sci Rep. (2017) 7:826. doi: 10.1038/s41598-017-00969-0
Keywords: edible plant oils, gut microbiota, health-promoting effects, metabolites, correlations, nutritional foods
Citation: Zou Q, Chen A-Q, Huang J, Wang M, Luo J-H, Wang A and Wang X-Y (2024) Edible plant oils modulate gut microbiota during their health-promoting effects: a review. Front. Nutr. 11:1473648. doi: 10.3389/fnut.2024.1473648
Edited by:
Ken Ng, The University of Melbourne, AustraliaReviewed by:
Wentong Xue, China Agricultural University, ChinaPeng Wu, First Affiliated Hospital of Zhengzhou University, China
Kaijian Hou, Shantou University, China
Copyright © 2024 Zou, Chen, Huang, Wang, Luo, Wang and Wang. This is an open-access article distributed under the terms of the Creative Commons Attribution License (CC BY). The use, distribution or reproduction in other forums is permitted, provided the original author(s) and the copyright owner(s) are credited and that the original publication in this journal is cited, in accordance with accepted academic practice. No use, distribution or reproduction is permitted which does not comply with these terms.
*Correspondence: Xiao-Yin Wang, xywang@gmu.edu.cn
†These authors share first authorship