- Center for Translational Research in Aging & Longevity, Texas A&M University, College Station, TX, United States
Purpose of review: This study aimed to discuss the use of the pulse stable isotope tracer approach to study changes in metabolism in healthy individuals and critically ill patients.
Recent findings and conclusion: We found that in the postabsorptive state and healthy condition, intracellular protein breakdown and net intracellular protein breakdown, when calculated using the pulse tracer approach, are about double what has previously been reported using the more traditional primed-constant and continuous stable isotope approaches (600 versus 300 grams of protein/day). In critically ill patients, protein breakdown is even higher and calculated to be approximately 900 grams of protein/day, using the pulse tracer approach. Based on these data, we hypothesize that reducing protein breakdown in the postabsorptive state is key when trying to improve the condition of critically ill patients. Moreover, we also used the pulse tracer approach during feeding to better estimate the intracellular metabolic response to feeding. Our first observation is that endogenous protein breakdown does not seem to be reduced during feeding. We also have shown that when consuming a meal with a certain amount of protein, the biological value of that protein meal can be calculated with the pulse tracer approach. In conclusion, using the pulse stable isotope tracer approach to study protein kinetics in the postabsorptive state and during feeding expands our understanding of how dietary proteins can affect human protein metabolism. The intracellular protein synthesis stimulatory effect of a meal is an important factor to consider when calculating the exact protein requirements and needs, particularly in critical illness.
Introduction
Meeting the enhanced needs of critically ill patients through optimal protein intake has been studied for many years. The current protein intake requirements (1) are based on the requirements established for healthy humans, to which a multiplication factor is added. However, protein turnover is highly upregulated in critically ill patients (2–4), suggesting an increased availability of amino acids as more amino acids are released intracellularly and into the circulation from protein breakdown (PB). Therefore, a higher disposal of those amino acids will become available for protein synthesis and other disposal routes. In steady-state conditions, the production of amino acids released from PB is in balance with the disposal of those amino acids.
Stable isotope tracer methodology is often used to measure amino acid kinetics (5). The basic principle is that the dilution measurement of the infused stable isotope amino acid makes it possible to calculate the endogenous substrate production and disposal, and when infusing stable isotopes of essential amino acids, PB can be estimated (6). Protein turnover in healthy individuals and during a variety of disease states, including critical illness, has predominantly been calculated using traditional methods such as primed-constant and continuous infusion of combinations of stable isotope amino acids such as leucine, phenylalanine, and tyrosine (7, 8). This approach requires intravenous infusion of stable amino acid tracers using a calibrated pump and accurate priming of the tracer pool to instantly obtain a tracer steady state, which is not always easy (4).
Recently, we reported a novel pulse tracer approach that enables the calculation of the intracellular production of amino acids (2–4, 9, 10). When using this approach, a pulse of stable isotopes is administered intravenously in a small volume and within 10 s. Measuring the decay of the isotope enrichments in plasma makes it possible to calculate simultaneously both the whole-body production (WBP) rate of these amino acids [is equal to the non-compartmental rate of appearance (Ra) as calculated by the primed-constant and continuous infusion model (7, 8)], and the intracellular production rate (3, 4) from the compartmental analysis. We have used this approach to compare the WBP and intracellular production of amino acids to better understand the balance between protein degradation rate and dietary protein intake (Figure 1).
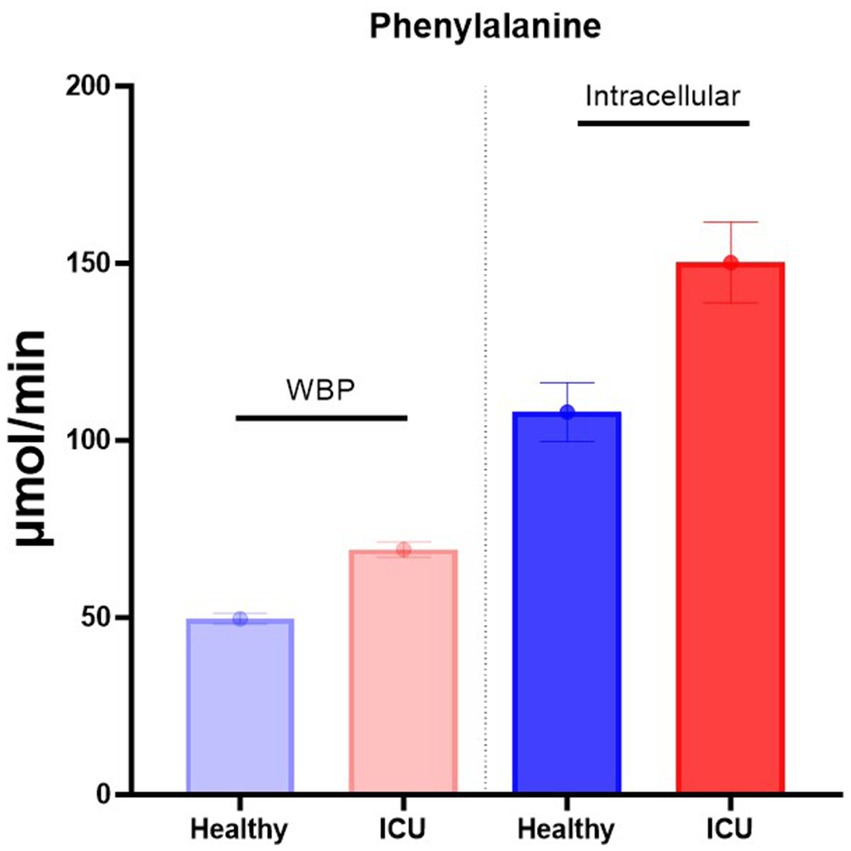
Figure 1. Intracellular phenylalanine production is much higher than WBP. Data are from Deutz et al. (4).
Protein turnover measurement in the post-absorptive state
As critically ill patients can have a wide range of changes in plasma amino acid concentrations (2, 11), correct priming of the pool to obtain instant tracer and tracer product steady state can be very difficult (12). To overcome this, we developed a pulse amino acid tracer approach (9), which does not need an infusion pump or knowledge of the pool sizes, and requires much smaller amounts of tracers than the traditional primed-constant and continuous infusion method. The pulse tracer approach is therefore an easy-to-use method for critically ill patients to study in depth their whole-body amino acid kinetics. Amino acid production can be assessed by calculating the area under the curve (AUC) of the tracer-tracee ratio decay (10) in the measured time period or by fitting the decay with a 2 exponential functions (3).
By measuring the phenylalanine production in healthy and critically ill patients (Figure 1), we can calculate the turnover of protein per gram protein/day/subject (Table 1 and Supplementary Figure S1) (4). As previously reported by others (13), ~300 grams of protein are broken down per day in a healthy individual in the postabsorptive state (4) as measured by the primed-constant and continuous stable isotope infusion protocol. If food only increases protein synthesis (see later), a dietary intake of approximately 75 grams of balanced protein would enhance protein synthesis to 375 grams, which is an ~25% increase.
The leucine/KIC approach (reciprocal model) (6) is often used to better estimate the total PB by assuming that the plasma KIC enrichment represents better the whole-body intracellular enrichment of leucine than the plasma leucine enrichment, as the conversion of leucine to KIC mainly takes place in muscle (14). PB measured with the KIC plasma enrichment is 1.3 times higher than when using the leucine plasma enrichment (15). In contrast, using the pulse approach, PB appears to be 2.6 times higher when calculated with the intracellular appearance of leucine than when calculated with Ra (4). Therefore, using plasma KIC enrichment to better estimate PB is not sufficient to correctly estimate intracellular PB. One of the reasons for this difference could be that it is based on the assumption that the conversion of leucine to KIC takes place in all organs at the same rate. Therefore, although this approach seems better than using plasma enrichment of leucine (6), it does not seem to correctly estimate intracellular PB.
As net PB depends on the careful measurement of oxidation when using the leucine/KIC approach, we and others used the combined phenylalanine/tyrosine approach, which only needs plasma enrichment measurements. The pulse approach with compartmental analysis and the phenylalanine/tyrosine approach, in our opinion, have the advantage of only requiring plasma enrichment measurements to calculate intracellular PB.
However, calculating PB from intracellular production (Table 1) leads to approximately 650 grams/day of PB in the postabsorptive state. In this case, a dietary intake of approximately 75 grams of protein/day would only lead to an 11% increase in protein synthesis. Consequently, if we calculate the net protein loss, which is the difference between PB and synthesis, a net loss of 87 grams of protein/day will take place in a healthy individual when there is no food intake. The net loss would be less if protein synthesis was lower in the absence of protein intake. However, during 12 h fasting (16), PB and oxidation of leucine are not increased, while after 3 days of fasting, protein oxidation is increased by 13% and PB by 30% (17). We therefore conclude that it is likely that the PB rate is not reduced by 24 h of fasting, and thus that our calculations of net loss are probably a good estimation.
In critically ill patients (Table 1), both non-compartmental PB and intracellular PB are increased by approximately 40% as compared to the healthy state. When using the intracellular PB measurement, protein degradation is approximately 900 grams/day and net protein loss is approximately 60 grams/day, which clearly shows that the turnover of protein is substantially increased in relation to the net loss. However, the net loss in critically ill patients is only 6.6% of total PB.
The calculations of net protein synthesis and breakdown are also affected by intracellular appearance. The calculation of phenylalanine hydroxylation as a proxy for oxidation is the enrichment ratio between plasma phenylalanine and the phenylalanine product tyrosine multiplied by the appearance of tyrosine. The conversion of phenylalanine to tyrosine occurs intracellularly, and thus the ratio estimates the correct ratio for both Ra and intracellular appearance calculations. However, the Ra of tyrosine underestimates the intracellular appearance of tyrosine, and therefore, the calculation of net protein synthesis/breakdown is higher than when the Ra of tyrosine is used.
One remarkable observation is that net PB in critically ill patients is not increased but decreased in the postabsorptive state (Table 1 (2–4)). We believe that we should try to interpret this observation physiologically. We hypothesize that reducing net protein loss in ICU patients could be a protective mechanism to reduce protein loss during disease. We also observed the same phenomenon in patients with chronic illnesses or at a higher age (18). Further research is needed to provide a more mechanistic explanation.
According to the calculations of the reduction of net PB in critically ill patients and thus of loss of lean mass, using the compartmental calculations (Table 2), critically ill patients will still lose approximately 400 grams of lean tissue/day (0.8%/day when total lean mass is approximately 50 kg). Others have found that loss of muscle mass, which is approximately 50% of total lean mass (19) in critically ill patients in the ICU is approximately 1%/day (20).
So how can protein loss be attenuated in healthy subjects in the postabsorptive state? We calculated (Table 1) that net protein loss in healthy subjects is approximately 87 grams, indicating that at least 87 grams of dietary amino acids are needed for a healthy subject to become anabolic. When protein is ingested, other factors such as digestion play a leading role in reduced protein efficiency, which may partly explain the higher protein intake advised (21). Reduced digestibility of dietary proteins likely becomes even more important in critically ill patients.
What are the clinical implications of these observations (2–4)? If PB is much higher in critically ill patients than previously thought, the protein synthesis rate will also increase. The energy costs of protein synthesis are approximately 1.3 kcal/gram protein (22), suggesting that these energy costs of critically ill patients are approximately 900 × 1.3 kcal = 1,170 kcal, 65% of the total 1,800 kcal REE we measured in critically ill patients (2). In addition, we suggested that not so much the amount of protein intake needs to be increased in critically ill patients, but that particularly the upregulated PB needs to be reduced (4). Therefore, we hypothesize that critically ill patients need dietary components that can reduce PB. We recently showed a reduction in PB when providing HMB to critically ill patients (23). However, additional research is needed on whether certain dietary amino acids and/or proteins are also able to reduce PB, as we previously showed for arginine in the critically ill (24).
Protein breakdown and synthesis during feeding
During feeding, there is an increase in amino acids released into the circulation, due to enhanced digestion and absorption of the meal-derived amino acids and from amino acids that become available from intracellular PB. The increased appearance of amino acids in the circulation and intracellularly will stimulate the disposal of amino acids (mainly for protein synthesis) and result in an increased intracellular concentration that could reduce PB. Several studies, including our own, have observed a reduced endogenous PB when using the primed-constant and continuous tracer infusion model (7, 8).
One important complicating factor could be the splanchnic extraction of amino acids that could affect the dilution of plasma enrichment. Endogenous PB is the rate of appearance (Ra), corrected for the amount of tracee entering the whole-body pool from nutrition. So we need to establish how much of the meal-derived amino acids are absorbed in the gut. If we assume that absorption is 100% for free dietary amino acids, the amount of nutrition entering the body pool in the mucosa cell needs to be subtracted from the Ra (calculated from the primed-continuous or pulse approach) or the intracellular appearance (from the pulse approach) to estimate endogenous PB.
So how does splanchnic extraction of meal-derived amino acids (e.g., phenylalanine) play a role in calculating endogenous PB? The basic assumption is that when using the Ra, a correction needs to be made for the Ra with the rate of meal-derived phenylalanine, appearing in the hepatic vein (thus post-splanchnic). The misunderstanding with this approach could be that the Ra only represents the PB of non-splanchnic organs and that subtracting the post-splanchnic appearance corrects the Ra on a whole-body level. In actuality, the Ra includes PB in all organs, and thus the absorbed meal-derived amino acids into the mucosa cell should be subtracted to calculate endogenous PB.
So what is the role of the splanchnic extraction measurement with the continuous stable isotope tracer infusion approach during feeding? In our opinion, this calculation is not needed at all. Only the estimation of how many amino acids from food are absorbed should be sufficient (6) to calculate endogenous PB. Using the pulse tracer approach, the same arguments will hold. There is no need to estimate splanchnic extraction to estimate endogenous PB. Therefore, the calculation that was used for many years, Ra = protein synthesis + oxidation = protein breakdown + intake from food remains valid (7, 25, 26). Using the pulse approach, Ra is replaced by the intracellular appearance.
However, it remained unclear whether the proteins in the meal were indeed able to reduce PB. We recently performed a pilot study on 11 human subjects to examine whether intracellular PB (using compartmental analysis) can also be measured in the prandial state (27, 28). For that purpose, we developed a protocol in which nutrition was provided every 20 min as sips containing a mixture of free amino acids, representing the composition of whey protein. Nutrition needs to be given as sips to obtain a steady state influx of dietary amino acids, and we previously observed that using a sip protocol gives comparable information on net protein synthesis and other measures, with some caveats (8). After the steady state was obtained, as verified by adding stable isotopes of amino acids to the sips and measuring the plasma enrichment and concentration of these amino acids, we administered the pulse of stable isotopes as previously conducted in the postabsorptive condition.
We subsequently compared the turnover of phenylalanine (as a measure of PB) obtained by non-compartmental and intracellular (compartmental) analyses in the prandial state (Figure 2, upper panel). Non-compartmental protein breakdown (WBP), measured during feeding (WBPfed), was approximately 50 μmol/min using this approach. When subtracting the WBP when no food was given (WBPfed—fasted), the difference in WBP was approximately 20 μmol/min. As the amount of phenylalanine given enterally was greater than the increase in WBPfed—fasted, the difference became negative (delta endoWBP). This means that there is a reduction in endogenous protein breakdown (endoWBP) when calculating the effect of feeding. A consistent reduction of PB during feeding has previously been observed by us (7, 8) and others (29–31) when WBP was measured using the primed-constant and continuous infusion models.
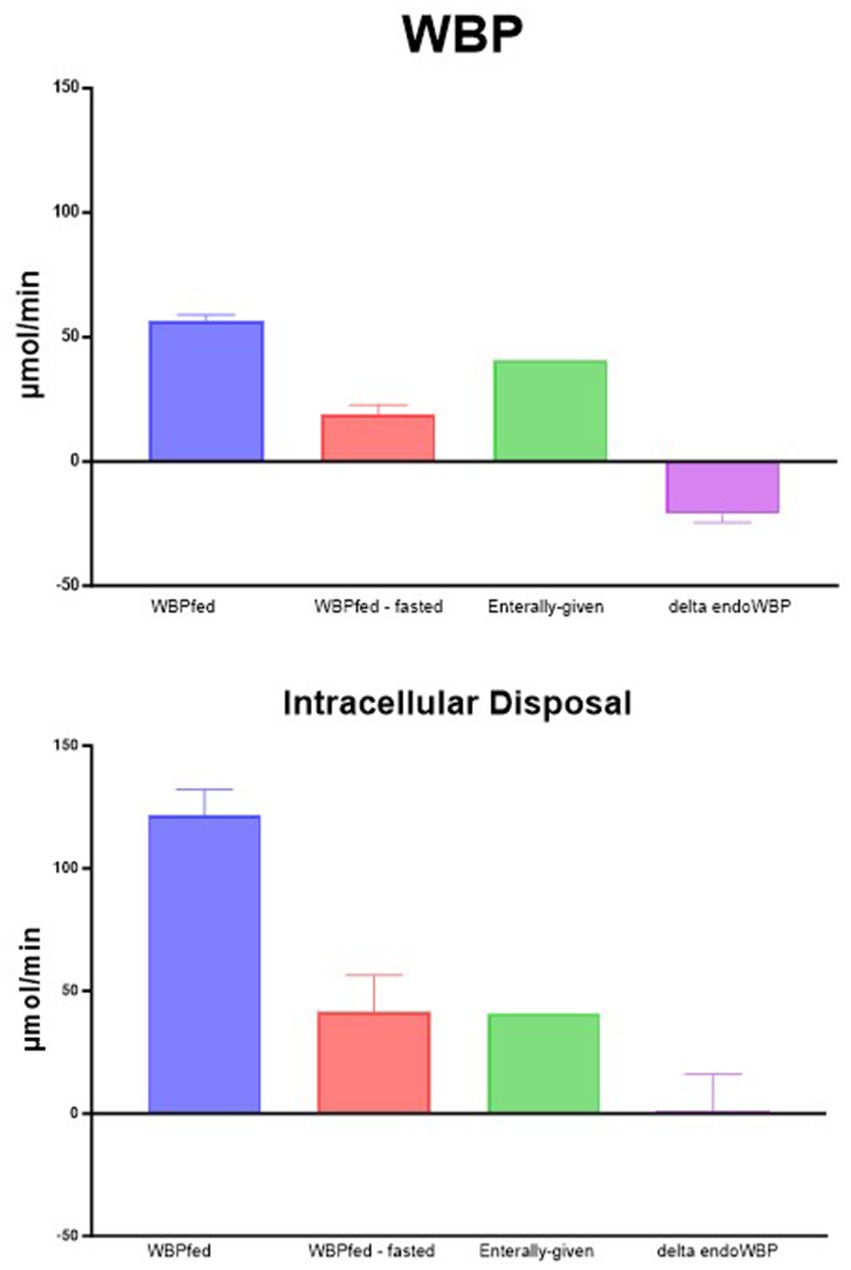
Figure 2. Phenylalanine production. WBPfed is the non-compartmental production during feeding. WBPfed—fasted is when the WBP in the post-absorptive condition is subtracted from the WBP during feeding. Enteral-given is the amount of phenylalanine given with the food. Delta EndoWBP is enterally given and subtracted from WBPfed—fasted, representing the change in the endogenous protein breakdown.
However, as indicated above, the non-compartmental protein breakdown calculation (WBP) underestimates the true intracellular PB, suggesting that our calculation of the intracellular PB provides a better reflection of the true PB. When the same calculations were performed using the intracellular PB approach (Figure 2, lower panel), the amount of phenylalanine given enterally as sips was very well matched with the difference between the intracellular appearance fed and fasted. Therefore, no reduction in PB was observed anymore. Therefore, we believe that feeding does not reduce endogenous PB and that these findings in the past might have likely been caused by the stable isotope tracer model used.
Perspectives and limitations
Our approach of combining sip feeding with the isotope pulse method can be used to measure the intracellular appearance of amino acids from any food protein. The intracellular appearance depends on how many amino acids are left after digestion and absorption of the food protein, the appearance of the plasma pool, and other factors that could have reduced the intracellular appearance and availability. Our pilot study shows that when using a mixture of free dietary amino acids and assuming that the digestion and absorption of free amino acids are not limited, the intracellular appearance of amino acids matches the amount consumed. However, a limitation of our observation is that we still do not know the exact digestion and absorption rates of amino acids.
Furthermore, when consuming a meal with a certain amount of protein, the biological value of that protein in principle can be calculated with our new approach, but this needs more validation studies. The same is true for complex meals with different types of proteins. Protein synthesis stimulatory effects of a meal seem to be an important factor in calculating the exact protein requirements and needs.
Therefore, we propose to check our approach in critically ill patients during feeding to establish which factors affect the anabolic capabilities of certain dietary amino acid mixtures or proteins as it might guide nutritional approaches in the critically ill.
Conclusion
We have provided a new view on protein metabolism in the postabsorptive and fed state when using the pulse stable isotope tracer approach. We have concluded that the estimation of protein turnover with the primed-constant and continuous infusion protocol is too low and healthy human PB is more in the range of 600 grams of protein/day.
We also presented new data that show that during feeding, endogenous PB is likely not reduced by food, but that feeding only stimulates protein synthesis.
Author contributions
ND: Writing – original draft, Writing – review & editing. ME: Writing – original draft, Writing – review & editing.
Funding
The author(s) declare that financial support was received for the research, authorship, and/or publication of this article. Research reported in this publication was partially supported by the National Institute of Health under Grant Number R01HL132887 from the National Heart, Lung, and Blood Institute. The content is solely the responsibility of the authors and it does not necessarily represent the official views of the National Heart, Lung, and Blood Institute or the National Institutes of Health.
Conflict of interest
The authors declare that the research was conducted in the absence of any commercial or financial relationships that could be construed as a potential conflict of interest.
Publisher's note
All claims expressed in this article are solely those of the authors and do not necessarily represent those of their affiliated organizations, or those of the publisher, the editors and the reviewers. Any product that may be evaluated in this article, or claim that may be made by its manufacturer, is not guaranteed or endorsed by the publisher.
Supplementary material
The Supplementary material for this article can be found online at: https://www.frontiersin.org/articles/10.3389/fnut.2024.1388969/full#supplementary-material
SUPPLEMENTARY FIGURE S1 | Graphic representation of the data as shown in Table 1.
Abbreviations
PB, Protein breakdown; WBP, Whole-body production, comparable to the rate of appearance.
References
1. Singer, P, Blaser, AR, Berger, MM, Alhazzani, W, Calder, PC, Casaer, MP, et al. ESPEN guideline on clinical nutrition in the intensive care unit. Clin Nutr. (2019) 38:48–79. doi: 10.1016/j.clnu.2018.08.037
2. Deutz, NEP, Singer, P, Wierzchowska-McNew, RA, Viana, MV, Ben-David, IA, Pantet, O, et al. Comprehensive metabolic amino acid flux analysis in critically ill patients. Clin Nutr. (2021) 40:2876–97. doi: 10.1016/j.clnu.2021.03.015
3. Deutz, NEP, Singer, P, Wierzchowska-McNew, RA, Viana, MV, Ben-David, IA, Pantet, O, et al. Females have a different metabolic response to critical illness, measured by comprehensive amino acid flux analysis. Metabolism. (2023) 142:155400. doi: 10.1016/j.metabol.2023.155400
4. Deutz, NEP, Haines, KL, Wischmeier, PE, and Engelen, M. Amino acid kinetics in the critically ill. Curr Opin Clin Nutr Metab Care. (2024) 27:61–9. doi: 10.1097/MCO.0000000000000995
5. Wolfe, RR, and Chinkes, DL. (2005). Isotope tracers in metabolic research: principles and practice of kinetic analysis. Wiley, New York. 1–274
6. Wolfe, RR, Kim, I-Y, Church, DD, Moughan, PJ, Park, S, and Ferrando, AA. Whole-body protein kinetic models to quantify the anabolic response to dietary protein consumption. Clin Nutr Open Sci. (2021) 36:78–90. doi: 10.1016/j.nutos.2021.02.006
7. Jonker, R, Deutz, NEP, Ligthart-Melis, GC, Zachria, AJ, Veley, EA, Harrykissoon, R, et al. Preserved anabolic threshold and capacity as estimated by a novel stable tracer approach suggests no anabolic resistance or increased requirements in weight stable COPD patients. Clin Nutr. (2019) 38:1833–43. doi: 10.1016/j.clnu.2018.07.018
8. Jonker, R, Deutz, NEP, Harrykissoon, R, Zachria, AJ, Veley, EA, and Engelen, M. A critical evaluation of the anabolic response after bolus or continuous feeding in COPD and healthy older adults. Clin Sci. (2018) 132:17–31. doi: 10.1042/CS20171068
9. Deutz, NEP, Thaden, JJ, Ten Have, GAM, Walker, DK, and Engelen, M. Metabolic phenotyping using kinetic measurements in young and older healthy adults. Metabolism. (2018) 78:167–78. doi: 10.1016/j.metabol.2017.09.015
10. Engelen, M, Ten Have, GAM, Thaden, JJ, and Deutz, NEP. New advances in stable tracer methods to assess whole-body protein and amino acid metabolism. Curr Opin Clin Nutr Metab Care. (2019) 22:337–46. doi: 10.1097/MCO.0000000000000583
11. Mierzchala-Pasierb, M, Lipinska-Gediga, M, Fleszar, MG, Lesnik, P, Placzkowska, S, Serek, P, et al. Altered profiles of serum amino acids in patients with sepsis and septic shock—preliminary findings. Arch Biochem Biophys. (2020) 691:108508. doi: 10.1016/j.abb.2020.108508
12. de Betue, CTI, Garcia Casal, XC, van Waardenburg, DA, Schexnayder, SM, Joosten, KFM, Deutz, NEP, et al. 24-hour protein, arginine and citrulline metabolism in fed critically ill children—a stable isotope tracer study. Clin Nutr. (2017) 36:876–87. doi: 10.1016/j.clnu.2016.12.023
13. Kang, MC. Muscle protein metabolism in critically illness. Surg Metab Nutr. (2020) 11:35–9. doi: 10.18858/smn.2020.11.2.35
14. Ten Have, GAM, Engelen, M, and Deutz, NEP. In-vivo production of branched-chain amino acids, branched-chain keto acids, and beta-hydroxy beta-methylbutyric acid. Curr Opin Clin Nutr Metab Care. (2022) 25:43–9. doi: 10.1097/MCO.0000000000000800
15. Toth, MJ, MacCoss, MJ, Poehlman, ET, and Matthews, DE. Recovery of 13CO2 from infused [1-13C]leucine and [1,2-13C2]leucine. Am J Physiol Endocrinol Metab. (2001) 281:E233–41. doi: 10.1152/ajpendo.2001.281.2.E233
16. Kurpad, AV, Regan, MM, Raj, T, Maruthy, K, Gnanou, J, and Young, VR. Intravenously infused 13C-leucine is retained in fasting healthy adult men. J Nutr. (2002) 132:1906–8. doi: 10.1093/jn/132.7.1906
17. Nair, KS, Woolf, PD, Welle, SL, and Matthews, DE. Leucine, glucose, and energy metabolism after 3 days of fasting in healthy human subjects. Am J Clin Nutr. (1987) 46:557–62. doi: 10.1093/ajcn/46.4.557
18. Cruthirds, CL, Deutz, NEP, Harrykissoon, R, Zachria, AJ, and Engelen, M. A low postabsorptive whole body protein balance is associated with markers of poor daily physical functioning in chronic obstructive pulmonary disease. Clin Nutr. (2022) 41:885–93. doi: 10.1016/j.clnu.2022.02.018
19. Zhu, K, Wactawski-Wende, J, Ochs-Balcom, HM, Lamonte, MJ, Hovey, KM, Evans, W, et al. The association of muscle mass measured by D3-creatine dilution method with dual-energy X-ray absorptiometry and physical function in postmenopausal women. J Gerontol A. (2021) 76:1591–9. doi: 10.1093/gerona/glab020
20. Fazzini, B, Markl, T, Costas, C, Blobner, M, Schaller, SJ, Prowle, J, et al. The rate and assessment of muscle wasting during critical illness: a systematic review and meta-analysis. Crit Care. (2023) 27:2. doi: 10.1186/s13054-022-04253-0
21. Heyland, DK, Patel, J, Compher, C, Rice, TW, Bear, DE, Lee, ZY, et al. The effect of higher protein dosing in critically ill patients with high nutritional risk (EFFORT protein): an international, multicentre, pragmatic, registry-based randomised trial. Lancet. (2023) 401:568–76. doi: 10.1016/S0140-6736(22)02469-2
22. Aoyagi, Y, Tasaki, I, Okumura, J-I, and Muramatsu, T. Energy COST of whole-body protein synthesis measured in V1VO in chicks. Comp Biochem Physiol. (1988) 91:765–8. doi: 10.1016/0300-9629(88)90962-0
23. Viana, MV, Becce, F, Pantet, O, Schmidt, S, Bagnoud, G, Thaden, JJ, et al. Impact of β-hydroxy-β-methylbutyrate (HMB) on muscle loss and protein metabolism in critically ill patients: a RCT. Clin Nutr. (2021) 40:4878–87. doi: 10.1016/j.clnu.2021.07.018
24. Luiking, YC, Poeze, M, and Deutz, NE. Arginine infusion in patients with septic shock increases nitric oxide production without haemodynamic instability. Clin Sci. (2015) 128:57–67. doi: 10.1042/CS20140343
25. Matthews, DE, Bier, DM, Rennie, MJ, Edwards, RH, Halliday, D, Millward, DJ, et al. Regulation of leucine metabolism in man: a stable isotope study. Science. (1981) 214:1129–31. doi: 10.1126/science.7302583
26. Waterlow, JC. Whole-body protein turnover in humans—past, present, and future. Annu Rev Nutr. (1995) 15:57–92. doi: 10.1146/annurev.nu.15.070195.000421
27. Knezek, SB, Engelen, MP, Perez, CN, Have, GAT, Thaden, JJ, and Deutz, NE. Meal anabolic capacity, measured by either non-or compartmental analysis after pulse tracer administration, is not different between HMB or LEU added to an essential amino acid mixture in older adults. Clin Nutr ESPEN. (2023) 58:694. doi: 10.1016/j.clnesp.2023.09.782
28. Knezek, SB, Engelen, MP, Perez, CN, Have, GAT, Thaden, JJ, and Deutz, NE. Increasing intake of free essential amino acids twofold leads to doubling of the anabolic response in healthy older adults without reducing protein breakdown. Clin Nutr ESPEN. (2023) 58:718–9. doi: 10.1016/j.clnesp.2023.09.840
29. Holwerda, AM, Paulussen, KJM, Overkamp, M, Goessens, JPB, Kramer, IF, Wodzig, W, et al. Dose-dependent increases in whole-body net protein balance and dietary protein-derived amino acid incorporation into myofibrillar protein during recovery from resistance exercise in older men. J Nutr. (2019) 149:221–30. doi: 10.1093/jn/nxy263
30. Trommelen, J, Kouw, IWK, Holwerda, AM, Snijders, T, Halson, SL, Rollo, I, et al. Presleep dietary protein-derived amino acids are incorporated in myofibrillar protein during postexercise overnight recovery. Am J Physiol Endocrinol Metab. (2018) 314:E457–67. doi: 10.1152/ajpendo.00273.2016
Keywords: amino acids, critically ill, ICU, stable isotopes, nutrition
Citation: Deutz NEP and Engelen MPKJ (2024) Compartmental analysis: a new approach to estimate protein breakdown and meal response in health and critical illness. Front. Nutr. 11:1388969. doi: 10.3389/fnut.2024.1388969
Edited by:
Paul Moughan, Massey University, New ZealandReviewed by:
Kenji Nagao, Ajinomoto, JapanRobert R. Wolfe, University of Arkansas for Medical Sciences, United States
Copyright © 2024 Deutz and Engelen. This is an open-access article distributed under the terms of the Creative Commons Attribution License (CC BY). The use, distribution or reproduction in other forums is permitted, provided the original author(s) and the copyright owner(s) are credited and that the original publication in this journal is cited, in accordance with accepted academic practice. No use, distribution or reproduction is permitted which does not comply with these terms.
*Correspondence: Nicolaas E. P. Deutz, bmVwLmRldXR6QGN0cmFsLm9yZw==