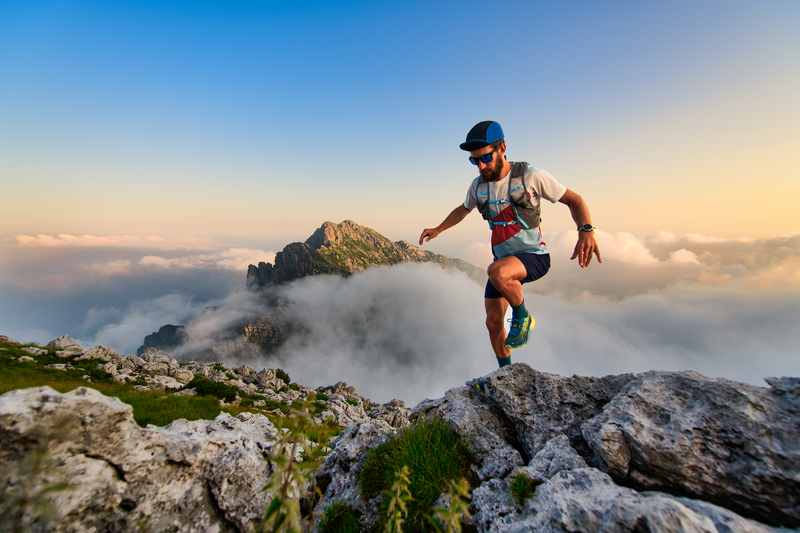
95% of researchers rate our articles as excellent or good
Learn more about the work of our research integrity team to safeguard the quality of each article we publish.
Find out more
CLINICAL TRIAL article
Front. Nutr. , 25 March 2024
Sec. Nutrition and Metabolism
Volume 11 - 2024 | https://doi.org/10.3389/fnut.2024.1384112
This article is part of the Research Topic Collagen Peptides: Production, Bioactivities, and Mechanism of Actions View all 4 articles
Introduction: Collagen peptide supplementation in conjunction with exercise has been shown to improve structural and functional adaptations of both muscles and the extracellular matrix. This study aimed to explore whether specific collagen peptide (SCP) supplementation combined with a concurrent training intervention can improve muscular stress after exercise-induced muscle damage, verified by reliable blood markers.
Methods: 55 sedentary to moderately active males participating in a concurrent training (CT) intervention (3x/week) for 12 weeks were administered either 15 g of SCP or placebo (PLA) daily. Before (T1) and after the intervention (T2), 150 muscle-damaging drop jumps were performed. Blood samples were collected to measure creatine kinase (CK), lactate dehydrogenase (LDH), myoglobin (MYO) and high-sensitivity C-reactive protein (hsCRP) before, after, and at 2 h, 24 h and 48 h post exercise.
Results: A combination of concurrent training and SCP administration showed statistically significant interaction effects, implying a lower increase in the area under the curve (AUC) of MYO (p = 0.004, ηp2 = 0.184), CK (p = 0.01, ηp2 = 0.145) and LDH (p = 0.016, ηp2 = 0.133) in the SCP group. On closer examination, the absolute mean differences (ΔAUCs) showed statistical significance in MYO (p = 0.017, d = 0.771), CK (p = 0.039, d = 0.633) and LDH (p = 0.016, d = 0.764) by SCP supplementation.
Conclusion: In conclusion, 12 weeks of 15 g SCP supplementation combined with CT intervention reduced acute markers of exercise-induced muscle damage and improved post-exercise regenerative capacity, as evidenced by the altered post-exercise time course. The current findings indicate that SCP supplementation had a positive effect on the early phase of muscular recovery by either improving the structural integrity of the muscle and extracellular matrix during the training period or by accelerating membrane and cytoskeletal protein repair.
Clinical trial registration: https://www.clinicaltrials.gov/study/NCT05220371?cond=NCT05220371&rank=1, NCT05220371.
Tissues responsible for proper movement execution (e.g., muscles, tendons) underlie a perpetual process of adaptation in response to specific environmental stimuli. In particular, unaccustomed eccentric exercise is known to induce high mechanical stress, leading to myofibril damage and extracellular matrix (ECM) disruption (1). Many studies have focused on nutritional interventions aimed at improving recovery from locomotor impairments, which are often observed as reductions in functional movement abilities (2–8). In this context, interest in collagen peptide (CP) administration has increased in recent years since regular CP intake has been shown to possibly alleviate joint discomfort, enhance ankle and knee functionality and improve recovery from Achilles tendinopathy, therefore acting as a potential and safe nutritional supplement in sports related injuries (9). Moreover, several short-term studies have reported that CP ingestion is superior to placebo after exercise-induced muscle damage (10–13). Faster restoration of biomechanical parameters such as improved countermovement jump height at 24 h (12) and 48 h (10), improved post-exercise muscle strength at 48 h, and reduced muscle soreness immediately after a muscle-damaging exercise bout (13) have been demonstrated following CP intake in conjunction with exercise. A proteomic analysis illustrated a significantly higher degree of upregulated proteins associated with skeletal muscle fibers, mainly contraction-related changes and structural adaptations to training. This suggests greater changes in collagen-specific proteins after 12 weeks of CP supplementation combined with resistance training (RT) (14). A recent study investigating gene expression patterns following strenuous bouts of knee extensions prior to a single dose of CP showed significant upregulation of the PI3K/Akt and MAPK pathways, both known to be essential for (myofibrillar) protein synthesis (15). The PI3K/Akt pathway may also play a role in connective tissue protein synthesis by mediating TGF-β2 signaling and inducing mRNA expression of COL1A1 & COL1A2 (collagen type 1 genes) in human pigment cells (16). Whether CP supplementation also stimulates connective tissue protein synthesis rates in vivo is currently unknown (17), but seems plausible, as increased collagen concentration was measured in human in vitro-engineered ligament after a single dose of 15 g CP (18).
Recovery from severe lengthening contractions (e.g., squats or drop jumps) also causes biomarkers in serum blood to peak within the following days. Proteins and metabolic-related enzymes such as creatine kinase (CK), lactate dehydrogenase (LDH), myoglobin (MYO), alanine transaminase (ALT), c-reactive protein (CRP) and interleukin-6 (IL-6) have been considered metabolic indicators of muscle damage and concomitant inflammation, both of which occur after strenuous, mainly eccentric exercise (19). However, there are only two studies without exercise intervention that examined recovery-related blood markers after CP supplementation. Neither found differences between CP and placebo after nine and 42 days of supplementation (10, 11). Therefore, the importance of regular, long-term, and at least moderately intense physical activity in combination with CP ingestion may be of interest for proteomic outcomes related to recovery. Furthermore, the CP administered in this study have only been utilized in two long-term investigations without examining blood related recovery parameters so far (20, 21). Thus, the impact of these particular CPs is also noteworthy.
Based on this assumption, the present study aimed to investigate whether 12 weeks of CP supplementation together with a concurrent training intervention would significantly influence exercise-induced muscular stress. In addition, recovery should be confirmed by specific blood parameters. This could indicate either a reduced acute stress response due to improved functional or structural integrity of the muscles. Or, on the other hand, an optimized muscular regenerative capacity that alleviates both myofibrillar damage and inflammation after exercise-induced muscle damage, or both. Therefore, we hypothesized that the increase of biomarkers in blood after a second bout of muscle-damaging exercise would be lower in subjects taking specific collagen peptides (SCP).
The study was a randomized, double-blind, placebo-controlled trial conducted at the University of Vienna, Austria. The study was approved by the Ethics Committee of the University of Vienna (reference no. 00765) and registered at ClinicalTrials.gov (ID: NCT05220371). 75 healthy male participants aged 18–40 years who had not exercised more than 3 hours per week in the preceding months were randomized to the treatment or placebo group after signing a written informed consent. The IPAQ questionnaire quantified individual physical activity levels. Exclusion criteria were a body mass index (BMI) below 18.5 kg/m2 or above 25 kg/m2, unstable weight or dietary behavior, physical complaints related to physical activity, and intake of protein supplements within the 6 months before the start of the study. In addition, subjects with renal, cardiovascular or metabolic diseases were excluded in accordance with American College of Sports Medicine (ACSM) guidelines (22).
The study design (Figure 1) comprised pre-tests (T1) before the training intervention, 12 weeks with daily intake of SCP or PLA with concurrent training (3x/week), and study completion with subsequent post-tests (T2) corresponding to the pre-tests. Subjects were screened 2 weeks before T1. Using a research randomizer1, participants were assigned to the SCP or PLA group after randomization. At baseline, study participants were given a standardized meal (SM) after overnight fasting (7 to 9 a.m.). 1 hour after the SM (“pre”), the first blood samples were collected, followed by the performance of 150 drop jumps. Immediately and 2 hours later, blood samples were collected again (“post,” “2 h”). On the second and third days, subjects arrived at the same time as on day 1, received their SM and waited 1 hour until blood was drawn two more times (“24 h,” “48 h”). Subjects were required to refrain from physically demanding or unaccustomed exercise and alcohol consumption for 48 h before the trials. Only water was allowed during the test procedures except for the SM.
Figure 1. Study design in chronological order. Blood, blood collection; MDIE, muscle damage inducing exercise; DJ, drop jump; CT, concurrent training; SM, standardized meal.
Each blood sample was collected venously via venipuncture and filled into 10 mL serum vacutainers. These were centrifuged at 2500 rpm for 15 min, and the resulting supernatant was pipetted into 2 mL Eppendorf tubes and immediately stored in a refrigerator at -32°C. For analysis purposes, the frozen tubes were transported to a local laboratory and analyzed separately. The Beckman Coulter AU5822 (23) was used for detecting creatine kinase (CK), lactate dehydrogenase (LDH) and myoglobin (MYO). High-sensitivity C-reactive protein (hsCRP) was detected “pre” & “24 h” by immunoturbidimetry (Alinity c CRP Vario Reagent Kit, Abbott Laboratories) with a coefficient of variance (CV) of 12.5%. The “24 h” measurement was chosen due to the high likelihood of CRP levels peaking 1 day after intense exercise (24, 25). As some participants were unable to provide blood at the given times, they were excluded from the data analysis.
The MDIE employed consisted of 150 drop jumps from a 60 cm box. Six sets of 25 jumps were prescribed, with a two-minute rest after each set. Participants had to perform the drop jumps slowly and achieve a knee angle of at least 90° to produce sufficient muscle damage, which has been documented elsewhere (26).
15 g of either a placebo (Silicea) or the test product (a mixture of specific collagen peptides [10 g PeptENDURE® + 5 g TENDOFORTE®], Gelita AG, Eberbach, Germany, Table 1) were administered orally daily for 12 weeks in powder form. Both products were mixed with 500 mL of water and drunk. One half of the SCP and PLA packs had to be taken 1 hour before and the second half instantly after the training session. This administration schedule would result in high blood levels of collagen peptides during and after exercise (27–29). On non-training days, the participants consumed 15 g as a whole at the same time as on exercise days.
A concurrent training (CT) intervention was conducted three times weekly for 12 weeks. The first half of the exercise program consisted of lower body weight exercises (squats, lunges and calf raises in chronological order). Starting with three sets of 20 repetitions for each exercise, five repetitions were added after 4 and 8 weeks. For the last 30 min of the CT, subjects ran at a heart rate determined individually according to the Karvonen formula (30) (training intensity factor between 0.7–0.8; HRmax = 220 – age). Pulse watches were provided for each training session to maintain the pace. After 4 and 8 weeks, the individual training intensity factor was increased by 5%. Recovery was ensured by a rest day between two training sessions. Training sessions were conducted outdoors every Monday, Wednesday and Friday at 7 a.m. and 6 p.m. under the supervision of sport scientists at the Institute of Sport Science in Vienna. Participants were encouraged to exercise regularly, either in the morning or afternoon session. Subjects completing fewer than 26 exercise sessions were eliminated from the study.
The SM (oats and an oat drink) was provided at T1 & T2 on every test day, which participants consumed 2 hours before blood collection. The SM contained 1 g of carbohydrate per kg of body weight. In addition, participants were required to ingest 15 g of PLA or SCP with their meal at T2.
Using household measurements, participants were required to quantify all the beverages and foods they consumed on two weekdays and one weekend day in weeks 1 and 12. Nut.s (nutritional.software, dato Denkwerkzeuge, Vienna) analyzed the amount of total energy intake, carbohydrates, fats and proteins.
IBM SPSS Statistics 23 (IBM SPSS Statistics for Windows, Armonk, NY: IBM Corp.) was used to perform all statistical analyses. Figures were generated by GraphPad Prism 9. The α-level was set to 5%. Blood marker analysis was based on the data listed in Table 2. These data were used to calculate the area under the curve (AUC) with the linear trapezoidal rule for each blood biomarker (31) and the deltas of these AUCs. Normal distribution was demonstrated with the Kolmogorov–Smirnov test. Dependent t-tests elaborated changes over time. Variance of homogeneity was tested with Levene’s test as a prerequisite for the independent t-test. A mixed design ANOVA (generalized linear model with repeated measures) was used for interaction effects, with group (SCP, PLA) as a between-subjects factor and study time (T1, T2) as a within-subjects factor. Partial eta-square (ηp2) was given as effect size (small effect: ηp2 > 0.01, medium effect: ηp2 > 0.06, large effect: ηp2 > 0.14). The effect size in pairwise t-tests was expressed as Cohen’s d (small effect: >0.2, medium effect: >0.5, large effect: >0.8) (32). In the case of a significant Mann–Whitney U test, the effect size was also reported as Cohen’s d/ηp2 [Wolfgang Lenhard & Alexandra (33)]. All tests were two-sided. Due to increased variability in the data sets, outliers were eliminated if they were above the 3rd standard deviation.
After meeting the inclusion criteria and a positive medical examination, 75 participants (initially 76, but one subject declined further participation before day 1) were randomized and assigned to the SCP (n = 37) or PLA group (n = 38) (Figure 2). Participants lost to follow-up were excluded for non-compliance (missing more than 10 sessions) with the training protocol. No adverse events or side effects related to SCP or PLA supplementation were observed. 55 subjects successfully completed the study.
Regarding anthropometric parameters (Table 3), no significant differences were observed for age (p = 0.44), mass (p = 0.35), height (p = 0.51) and BMI (p = 0.7). No significant time effect was calculated for PLA [energy (p = 0.77), protein (p = 0.54), fat (p = 0.44), carbohydrate (p = 0.26)] and SCP [energy (p = 0.09), protein (p = 0.47), fat (p = 0.74), carbohydrate (p = 0.06)] in relation to dietary intake. Similarly, no differences were evident between groups in T1 [energy (p = 0.71), protein (p = 0.72), fat (p = 0.55), carbohydrate (p = 0.89)] as well as in T2 [energy (p = 0.08), protein (p = 0.09), fat (p = 0.08), carbohydrate (p = 0.52)]. In addition, no interaction effect was observed for energy (p = 0.24), protein (p = 0.32), fat (p = 0.36) and carbohydrate intake (p = 0.59).
Descriptive statistics for CK, LDH, MYO and hsCRP are shown in Table 2, which delineates the data used for further analyses. As some blood samples could not be obtained (entirely) from all subjects who completed the study due to malaise and nausea during the process of blood collection, the number of participants differed slightly between each marker. Individuals who failed to furnish blood at a particular time regarding one specific parameter were excluded whereas an exclusion was not obligatory for other parameters, if the amount of collected blood was sufficient for their analysis. Therefore, the following numbers of participants have been analyzed: 45 (CK), 43 (LDH), 44 (MYO) and 40 (hsCRP).
The CK, MYO and hsCRP data were log-transformed due to an initial violation of normal distribution. Significant interaction effects implying a greater reduction in the SCP group were observed in the AUCs of MYO (p = 0.004, ηp2 = 0.184), CK (p = 0.01, ηp2 = 0.145), LDH (p = 0.016 ηp2 = 0.133) but not hsCRP (p = 0.54), as shown in Figure 3. Figures 3, 4 demonstrate the data without transformations.
SCP intake resulted in a significantly higher reduction, visualized by ΔAUCs, in MYO (p = 0.017, d = 0.771/ ηp2 = 0.129), CK (p = 0.039, d = 0.633), LDH (p = 0.016, d = 0.764) but not in hsCRP (p = 0.915) (Figure 4). For MYO and hsCRP, the Mann–Whitney U Test was applied as a non-parametric alternative.
To the best of our knowledge, this is the first study to examine the longer-term impact of a daily intake of specific collagen peptides (SCP) in combination with a 12-week concurrent training (CT) program on muscle stress and recovery-related blood biomarkers. 15 g of SCP significantly decreased the area under the curve (AUC) as well as the ΔAUCs of myoglobin (MYO), creatine kinase (CK) and lactate dehydrogenase (LDH) activity, suggesting improved regenerative capacity within 48 h after muscle damage-inducing bouts of drop jumps.
The results showed better adaptation after eccentric exercise, suggesting a more pronounced repeated bout effect (RBE) after prolonged SCP ingestion. The RBE, a not yet fully understood mechanism that protects tissues involved in muscle lengthening exercise execution from subsequent damage, is thought to be due to neural adaptations, extracellular matrix remodeling, adaptations at the muscle-tendon junction, and inflammation (34).
Severe myofibrillar damage, particularly as it occurs after unaccustomed eccentric exercise bouts, usually results in significant increases in CK, LDH and MYO, which have been extensively used as indirect biomarkers to monitor the extent of acute stress as well as recovery (35). The activity of both cytosolic enzymes (CK, LDH) during a nine (10) and 33-day (13) CP supplementation period was not lower compared to a placebo after muscle-damaging exercise, which could have indicated either reduced acute muscle stress or accelerated reconstruction of damaged tissue. These two studies are the only ones to date that have examined recovery after CP intake by analyzing CK & LDH, albeit in a more short-term manner without prolonged exercise intervention. Therefore, the three-month training intervention in the present study may have improved the remodeling of the force-producing and transmitting tissues (ECM, muscle) with regular SCP administration, reducing the increase in CK & LDH. This hypothesis is supported by the results of a proteomic approach following a 12-week intake of 15 g CP and resistance training (14). The authors identified a 14-fold change in hypoxia-inducible factor alpha 1 (HIF-1α), the alpha subunit of the transcription factor HIF-1, which has shown its contribution to skeletal muscle regeneration in mice (36). HIF-1 exhibits upregulated expression in hypoxic situations, such as exercise, and has been found to play a role in collagen production in rodent tubular epithelial cells (37). Specifically, HIF-1 induces the expression of prolyl and lysyl hydroxylase, two enzymes essential for fiber formation, and promotes proper collagen biogenesis, ECM organization, stiffness, cell adhesion, and motility in human fibroblasts (38). Heat shock protein 90 (HSP90), also upregulated 14-fold in CP in (14), is an HSP chaperone group protein. HSPs are usually overexpressed when exposed to infection, heat-induced stress, hypoxia and thus heat treatment (used as a therapeutic approach), and exercise. Previous studies in mice indicated increased protein levels and markers of muscle hypertrophy/recovery (MHCneo) associated with higher HSP72 levels following downhill running (39). Data from healthy human subjects running downhill for 45 min have shown a negative correlation of ΔMYO and ΔCK along with increasing concentrations of ΔHSP90 (1 h minus baseline) 1 hour after the eccentric endurance bout (40). In addition, Heat shock factor 1 (HSF1), a major transcription factor of HSPs, was also upregulated in CP influenced by HIF-1α in (14), indicating a close interdependence of these proteins (41). It appears that HSPs are involved in protecting cells from damage (42). Still, caution is also warranted when persistently elevated levels are observed, as they indicate chronic inflammation (43). Finally, five and four-fold changes in MAPK and PI3K-Akt pathways have been observed after CP ingestion (14), which are important initiators of signaling cascades leading to protein synthesis. There is little evidence from in-vitro experiments that PI3K-Akt & MAPK may also trigger collagen type I gene expression via TGF-β2 activation (16, 44, 45). TGF-β cytokines generally induce intracellular signaling via specific Smad proteins (Smad2, 3 forming Smad 4 etc.) (46), the latter being responsible for the expression of COL1A2 and COL3A1 (47) which give rise to collagen types I & III, both of which are most abundant in intramuscular connective tissue (48, 49). Given that Smad2, 4 and COL1A1 & COL3A1 mRNA were significantly upregulated 27 (COL1A1) and 30 days after two muscle-damaging bouts of eccentric contractions separated by 27 days (50), the remodeling of muscle ECM, particularly force-bearing proteins such as COL I & III, represents a longer-term process (51). This functional recovery and adaptation process appears to be enhanced by CP supplementation induced by the mechanisms described above.
Based on the CK, LDH & MYO results, the findings suggest increased regenerative capacity throughout ongoing myocellular remodeling. While LDH typically peaks within hours, MYO concentrations and CK activity are highest on the first to third day or, in some cases, even on the fourth day after strenuous exercise, depending on the type of load, muscles used, and individual training history (52–57). Each biomarker leaves its original intracellular location due to cell damage-induced permeability and subsequently enters the bloodstream, where it can be measured in serum/plasma in chronological order (LDH > MYO > CK) (35, 58). In the present study, prolonged SCP intake was shown to ameliorate several cell damage markers and have a beneficial effect on muscle stress response, early-phase muscle recovery, and enhanced RBE. Whether adaptions occurred in surrounding structures such as intramuscular connective tissue and/or muscle fibers per se remains unknown.
Regarding inflammatory parameters, the acute-phase protein C-reactive protein (CRP) is a commonly used marker of local and systemic inflammation. In this trial, there was no significant difference between both groups in terms of hsCRP. Considering the measurement time points (baseline and after 24 h), significant CRP changes were already detected after a marathon race (59), a one-hour strenuous endurance run (52), a 90-min eccentric exercise protocol (60) and 100 drop jumps (61). SCP intake had no apparent significant effect on post-exercise inflammatory status in this study; among other factors, the high CV of 12.5% for hsCRP might be responsible for this.
The choice of concurrent training (CT) as the type of training intervention in the present study was based on the following considerations. In general, CT combines the benefits of both RT and ET, at least in untrained to recreational athletes. CT improves physical fitness, does not impair maximal strength and muscle hypertrophy, and is thus consistent with public health guidelines (62). In contrast, CT limits explosive strength gains compared to resistance-only training, offset in the current study by performing RT before ET (63). In agreement with (64), moderate to high ET intensity was prescribed to benefit cardiorespiratory fitness and muscle strength (64). As a recent systematic review mentioned, protein intake (whey, casein, soy, milk) after an acute CT bout increases myofibrillar protein synthesis to a similar extent as after RT (65). Moreover, phosphorylation of the signaling proteins Akt–mTOR-S6K increased significantly immediately after CT and protein intake compared with placebo, indicating enhanced translation of myofibrillar mRNAs (66). CT plus protein also resulted in an immediate post-exercise increase in specific micro RNAs, suggesting a more myogenic rather than oxidative adaptation, and an effect on longer-term CT responses (65, 67). Whether SCP supplementation in combination with CT also leads to the above results remains unknown but may be of interest for future studies.
The present study also has some limitations. Selecting an exercise that also stressed upper limb muscles (e.g., elbow flexors) would have led to more insightful information about the recovery-related setting of blood markers, as upper and lower body muscles have been shown to elicit a slightly different response to MYO & CK (57, 68). In addition, it has recently been suggested that a 30 g dose of milk protein taken immediately after endurance training efficiently repairs and resynthesizes body proteins (65, 69). Whether the same amount of SCP supplementation over a longer period would result in improved recovery of tissues subjected to exercise-induced muscle damage requires future investigation. To date, there is no clinical evidence or recommendations for effective CP dosing to optimize regenerative capacity. In addition, the effects of SCP supplemented in the present study cannot be generalized to other CP products.
In conclusion, 12 weeks of daily supplementation of 15 g of specific collagen peptides (SCP) in combination with a concurrent training intervention resulted in reduced acute muscle stress response and improvements in early phase recovery, as represented by significantly lower levels of myoglobin (MYO), creatine kinase (CK), and lactate dehydrogenase (LDH) after a second bout of muscle damage-inducing exercise. As this also indicates an enhanced repeated bout effect (RBE), SCPs, together with training, may accelerate repair and reduce cellular damage to the force-producing and transmitting tissues in the first 48 h after eccentric exercise. Future studies should therefore investigate the underlying molecular pathways of myocellular and ECM adaptation after intense muscle-damaging exercises. This may reveal specific target mechanisms affected by SCP intake that could be of significant practical importance in reducing rehabilitation and recovery time and improving RBE.
The raw data supporting the conclusions of this article will be made available by the authors, without undue reservation.
The studies involving humans were approved by Ethics Committee of the University of Vienna (reference no. 00765). The studies were conducted in accordance with the local legislation and institutional requirements. The participants provided their written informed consent to participate in this study.
KB: Writing – original draft, Writing – review & editing. SS: Writing – review & editing. LB: Writing – review & editing. SO: Writing – review & editing. AB: Writing – review & editing. DK: Writing – original draft, Writing – review & editing.
The author(s) declare that financial support was received for the research, authorship, and/or publication of this article. GELITA GmbH, Eberbach Germany, provided the test products (10 g PeptENDURE®, 5 g Tendoforte®) and the placebo. The funder was not involved in the study design, collection, analysis, interpretation of data, the writing of this article or the decision to submit it for publication. All authors declare no other competing interests.
The authors declare that the research was conducted in the absence of any commercial or financial relationships that could be construed as a potential conflict of interest.
All claims expressed in this article are solely those of the authors and do not necessarily represent those of their affiliated organizations, or those of the publisher, the editors and the reviewers. Any product that may be evaluated in this article, or claim that may be made by its manufacturer, is not guaranteed or endorsed by the publisher.
1. Hody, S, Croisier, J-L, Bury, T, Rogister, B, and Leprince, P. Eccentric muscle contractions: risks and benefits. Front Physiol. (2019) 10:536. doi: 10.3389/fphys.2019.00536
2. Bongiovanni, T, Genovesi, F, Nemmer, M, Carling, C, Alberti, G, and Howatson, G. Nutritional interventions for reducing the signs and symptoms of exercise-induced muscle damage and accelerate recovery in athletes: current knowledge, practical application and future perspectives. Eur J Appl Physiol. (2020) 120:1965–96. doi: 10.1007/s00421-020-04432-3
3. König, D, Kohl, J, Jerger, S, and Centner, C. Potential relevance of bioactive peptides in sports nutrition. Nutrients. (2021) 13:3997. doi: 10.3390/nu13113997
4. Markus, I, Constantini, K, Hoffman, JR, Bartolomei, S, and Gepner, Y. Exercise-induced muscle damage: mechanism, assessment and nutritional factors to accelerate recovery. Eur J Appl Physiol. (2021) 121:969–92. doi: 10.1007/s00421-020-04566-4
5. Maughan, RJ, Burke, LM, Dvorak, J, Larson-Meyer, DE, Peeling, P, Phillips, SM, et al. IOC consensus statement: dietary supplements and the high-performance athlete. Int J Sport Nutr Exerc Metab. (2018) 28:104–25. doi: 10.1123/ijsnem.2018-0020
6. Thomas, DT, Erdman, KA, and Burke, LM. American College of Sports Medicine joint position statement. Nutrition and athletic performance. Med Sci Sports Exerc. (2016) 48:543–68. doi: 10.1249/MSS.0000000000000852
7. Tanabe, Y, Fujii, N, and Suzuki, K. Dietary supplementation for attenuating exercise-induced muscle damage and delayed-onset muscle soreness in humans. Nutrients. (2021) 14:70. doi: 10.3390/nu14010070
8. Owens, DJ, Twist, C, Cobley, JN, Howatson, G, and Close, GL. Exercise-induced muscle damage: what is it, what causes it and what are the nutritional solutions? Eur J Sport Sci. (2019) 19:71–85. doi: 10.1080/17461391.2018.1505957
9. Khatri, M, Naughton, RJ, Clifford, T, Harper, LD, and Corr, L. The effects of collagen peptide supplementation on body composition, collagen synthesis, and recovery from joint injury and exercise: a systematic review. Amino Acids. (2021) 53:1493–506. doi: 10.1007/s00726-021-03072-x
10. Clifford, T, Ventress, M, Allerton, DM, Stansfield, S, Tang, JCY, Fraser, WD, et al. The effects of collagen peptides on muscle damage, inflammation and bone turnover following exercise: a randomized, controlled trial. Amino Acids. (2019) 51:691–704. doi: 10.1007/s00726-019-02706-5
11. Lopez, HL, Ziegenfuss, TN, and Park, J. Evaluation of the effects of bio cell collagen, a novel cartilage extract, on connective tissue support and functional recovery from exercise. Integr Med. (2015) 14:30–8. doi: 10.1186/1550-2783-11-S1-P48
12. Prowting, JL, Bemben, D, Black, CD, Day, EA, and Campbell, JA. Effects of collagen peptides on recovery following eccentric exercise in resistance-trained males-a pilot study. Int J Sport Nutr Exerc Metab. (2021) 31:32–9. doi: 10.1123/ijsnem.2020-0149
13. Kuwaba, K, Kusubata, M, Taga, Y, Igarashi, H, Nakazato, K, and Mizuno, K. Dietary collagen peptides alleviate exercise-induced muscle soreness in healthy middle-aged males: a randomized double-blinded crossover clinical trial. J Int Soc Sports Nutr. (2023) 20:2206392. doi: 10.1080/15502783.2023.2206392
14. Oertzen-Hagemann, V, Kirmse, M, Eggers, B, Pfeiffer, K, Marcus, K, De, MM, et al. Effects of 12 weeks of hypertrophy resistance exercise training combined with collagen peptide supplementation on the skeletal muscle proteome in recreationally active men. Nutrients. (2019) 11:1072. doi: 10.3390/nu11051072
15. Centner, C, Jerger, S, Mallard, A, Herrmann, A, Varfolomeeva, E, Gollhofer, S, et al. Supplementation of specific collagen peptides following high-load resistance exercise upregulates gene expression in pathways involved in skeletal muscle signal transduction. Front Physiol. (2022) 13:838004. doi: 10.3389/fphys.2022.838004
16. Yokoyama, K, Kimoto, K, Itoh, Y, Nakatsuka, K, Matsuo, N, Yoshioka, H, et al. The PI3K/Akt pathway mediates the expression of type I collagen induced by TGF-β2 in human retinal pigment epithelial cells. Graefes Arch Clin Exp Ophthalmol. (2012) 250:15–23. doi: 10.1007/s00417-011-1766-x
17. Holwerda, AM, and van Loon, LJC. The impact of collagen protein ingestion on musculoskeletal connective tissue remodeling: a narrative review. Nutr Rev. (2022) 80:1497–514. doi: 10.1093/nutrit/nuab083
18. Shaw, G, Lee-Barthel, A, Ross, ML, Wang, B, and Baar, K. Vitamin C-enriched gelatin supplementation before intermittent activity augments collagen synthesis. Am J Clin Nutr. (2017) 105:136–43. doi: 10.3945/ajcn.116.138594
19. Pérez-Castillo, ÍM, Rueda, R, Bouzamondo, H, López-Chicharro, J, and Mihic, N. Biomarkers of post-match recovery in semi-professional and professional football (soccer). Front Physiol. (2023) 14:1167449. doi: 10.3389/fphys.2023.1167449
20. Balshaw, TG, Funnell, MP, McDermott, E, Maden-Wilkinson, TM, Abela, S, Quteishat, B, et al. The effect of specific bioactive collagen peptides on function and muscle remodeling during human resistance training. Acta Physiol. (2023) 237:e13903. doi: 10.1111/apha.13903
21. Bischof, K, Stafilidis, S, Bundschuh, L, Oesser, S, Baca, A, and König, D. Influence of specific collagen peptides and 12-week concurrent training on recovery-related biomechanical characteristics following exercise-induced muscle damage-a randomized controlled trial. Front Nutr. (2023) 10:1266056. doi: 10.3389/fnut.2023.1266056
22. Riebe, D, Franklin, BA, Thompson, PD, Garber, CE, Whitfield, GP, Magal, M, et al. Updating ACSM's recommendations for exercise preparticipation health screening. Med Sci Sports Exerc. (2015) 47:2473–9. doi: 10.1249/MSS.0000000000000664
23. Zimmerman, MK, Friesen, LR, Nice, A, Vollmer, PA, Dockery, EA, Rankin, JD, et al. Multi-center evaluation of analytical performance of the Beckman coulter AU5822 chemistry analyzer. Clin Biochem. (2015) 48:881–5. doi: 10.1016/j.clinbiochem.2015.06.010
24. Isaacs, AW, Macaluso, F, Smith, C, and Myburgh, KH. C-reactive protein is elevated only in high Creatine kinase responders to muscle damaging exercise. Front Physiol. (2019) 10:86. doi: 10.3389/fphys.2019.00086
25. Kim, HJ, Lee, YH, and Kim, CK. Changes in serum cartilage oligomeric matrix protein (COMP), plasma CPK and plasma hs-CRP in relation to running distance in a marathon (42.195 km) and an ultra-marathon (200 km) race. Eur J Appl Physiol. (2009) 105:765–70. doi: 10.1007/s00421-008-0961-x
26. Clifford, T, Bell, O, West, DJ, Howatson, G, and Stevenson, EJ. The effects of beetroot juice supplementation on indices of muscle damage following eccentric exercise. Eur J Appl Physiol. (2016) 116:353–62. doi: 10.1007/s00421-015-3290-x
27. Iwasaki, Y, Nakatogawa, M, Shimizu, A, Sato, Y, and Shigemura, Y. Comparison of gelatin and low-molecular weight gelatin hydrolysate ingestion on hydroxyproline (Hyp), pro-Hyp and Hyp-Gly concentrations in human blood. Food Chem. (2022) 369:130869. doi: 10.1016/j.foodchem.2021.130869
28. Alcock, RD, Shaw, GC, Tee, N, and Burke, LM. Plasma amino acid concentrations after the ingestion of dairy and collagen proteins, in healthy active males. Front Nutr. (2019) 6:163. doi: 10.3389/fnut.2019.00163
29. Skov, K, Oxfeldt, M, Thøgersen, R, Hansen, M, and Bertram, HC. Enzymatic hydrolysis of a collagen hydrolysate enhances postprandial absorption rate-a randomized controlled trial. Nutrients. (2019) 11:1064. doi: 10.3390/nu11051064
30. Karvonen, MJ, Kentala, E, and Mustala, O. The effects of training on heart rate; a longitudinal study. Ann Med Exp Biol Fenn. (1957) 35:307–15.
31. Yeh, S. T. Using trapezoidal rule for the area under a curve calculation. Proceedings of the 27th annual SAS User Group International (SUGI'02) (2002):1–5. Glaxosmithkline, Collegeville, PA
32. Cohen, J. Statistical power analysis for the behavioral sciences. Hillsdale, NJ: Erlbaum (1988).
33. Lenhard, Wolfgang, and Lenhard, Alexandra. Computation of effect sizes. (2017). Available at: http://www.psychometrica.de/effect_size.html
34. Hyldahl, RD, Chen, TC, and Nosaka, K. Mechanisms and mediators of the skeletal muscle repeated bout effect. Exerc Sport Sci Rev. (2017) 45:24–33. doi: 10.1249/JES.0000000000000095
35. Haller, N, Behringer, M, Reichel, T, Wahl, P, Simon, P, Krüger, K, et al. Blood-based biomarkers for managing workload in athletes: considerations and recommendations for evidence-based use of established biomarkers. Sports Med. (2023) 53:1315–33. doi: 10.1007/s40279-023-01836-x
36. Scheerer, N, Dehne, N, Stockmann, C, Swoboda, S, Baba, HA, Neugebauer, A, et al. Myeloid hypoxia-inducible factor-1α is essential for skeletal muscle regeneration in mice. J Immunol. (2013) 191:407–14. doi: 10.4049/jimmunol.1103779
37. Higgins, DF, Kimura, K, Bernhardt, WM, Shrimanker, N, Akai, Y, Hohenstein, B, et al. Hypoxia promotes fibrogenesis in vivo via HIF-1 stimulation of epithelial-to-mesenchymal transition. J Clin Invest. (2007) 117:3810–20. doi: 10.1172/JCI30487
38. Gilkes, DM, Bajpai, S, Chaturvedi, P, Wirtz, D, and Semenza, GL. Hypoxia-inducible factor 1 (HIF-1) promotes extracellular matrix remodeling under hypoxic conditions by inducing P4HA1, P4HA2, and PLOD2 expression in fibroblasts. J Biol Chem. (2013) 288:10819–29. doi: 10.1074/jbc.M112.442939
39. Touchberry, CD, Gupte, AA, Bomhoff, GL, Graham, ZA, Geiger, PC, and Gallagher, PM. Acute heat stress prior to downhill running may enhance skeletal muscle remodeling. Cell Stress Chaperones. (2012) 17:693–705. doi: 10.1007/s12192-012-0343-5
40. Švec, X, Štorkánová, H, Špiritović, M, Slabý, K, Oreská, S, Pekáčová, A, et al. Hsp 90 as a Myokine: its association with systemic inflammation after exercise interventions in patients with myositis and healthy subjects. Int J Mol Sci. (2022) 23:11451. doi: 10.3390/ijms231911451
41. Szyller, J, and Bil-Lula, I. Heat shock proteins in oxidative stress and ischemia/reperfusion injury and benefits from physical exercises: a review to the current knowledge. Oxidative Med Cell Longev. (2021) 2021:1–12. doi: 10.1155/2021/6678457
42. McGorm, H, Roberts, LA, Coombes, JS, and Peake, JM. Turning up the heat: an evaluation of the evidence for heating to promote exercise recovery, muscle rehabilitation and adaptation. Sports medicine. (2018) 48:1311–28.
43. Krüger, K, Reichel, T, and Zeilinger, C. Role of heat shock proteins 70/90 in exercise physiology and exercise immunology and their diagnostic potential in sports. J Appl Physiol (1985). (2019) 126:916–27. doi: 10.1152/japplphysiol.01052.2018
44. Kimoto, K, Nakatsuka, K, Matsuo, N, and Yoshioka, H. p38 MAPK mediates the expression of type I collagen induced by TGF-beta 2 in human retinal pigment epithelial cells ARPE-19. Invest Ophthalmol Vis Sci. (2004) 45:2431–7. doi: 10.1167/iovs.03-1276
45. Inoue-Mochita, M, Inoue, T, Fujimoto, T, Kameda, T, Awai-Kasaoka, N, Ohtsu, N, et al. p38 MAP kinase inhibitor suppresses transforming growth factor-β2-induced type 1 collagen production in trabecular meshwork cells. PLoS One. (2015) 10:e0120774. doi: 10.1371/journal.pone.0120774
46. Tsukada, S, Westwick, JK, Ikejima, K, Sato, N, and Rippe, RA. SMAD and p38 MAPK signaling pathways independently regulate alpha1(I) collagen gene expression in unstimulated and transforming growth factor-beta-stimulated hepatic stellate cells. J Biol Chem. (2005) 280:10055–64. doi: 10.1074/jbc.M409381200
47. Verrecchia, F, Chu, ML, and Mauviel, A. Identification of novel TGF-beta/Smad gene targets in dermal fibroblasts using a combined cDNA microarray/promoter transactivation approach. J Biol Chem. (2001) 276:17058–62. doi: 10.1074/jbc.M100754200
48. McKee, TJ, Perlman, G, Morris, M, and Komarova, SV. Extracellular matrix composition of connective tissues: a systematic review and meta-analysis. Sci Rep. (2019) 9:10542. doi: 10.1038/s41598-019-46896-0
49. Purslow, PP. The structure and role of intramuscular connective tissue in muscle function. Front Physiol. (2020) 11:495. doi: 10.3389/fphys.2020.00495
50. Hyldahl, RD, Nelson, B, Xin, L, Welling, T, Groscost, L, Hubal, MJ, et al. Extracellular matrix remodeling and its contribution to protective adaptation following lengthening contractions in human muscle. FASEB J. (2015) 29:2894–904. doi: 10.1096/fj.14-266668
51. Mackey, AL, and Kjaer, M. Connective tissue regeneration in skeletal muscle after eccentric contraction-induced injury. J Appl Physiol (1985). (2017) 122:533–40. doi: 10.1152/japplphysiol.00577.2016
52. Reichel, T, Boßlau, TK, Palmowski, J, Eder, K, Ringseis, R, Mooren, FC, et al. Reliability and suitability of physiological exercise response and recovery markers. Sci Rep. (2020) 10:11924. doi: 10.1038/s41598-020-69280-9
53. Kanda, K, Sugama, K, Hayashida, H, Sakuma, J, Kawakami, Y, Miura, S, et al. Eccentric exercise-induced delayed-onset muscle soreness and changes in markers of muscle damage and inflammation. Exerc Immunol Rev. (2013) 19:72–85.
54. Pizza, FX, Davis, BH, Henrickson, SD, Mitchell, JB, Pace, JF, Bigelow, N, et al. Adaptation to eccentric exercise: effect on CD64 and CD11b/CD18 expression. J Appl Physiol. (1996) 80:47–55. doi: 10.1152/jappl.1996.80.1.47
55. Rodenburg, JB, Bär, PR, and De, BRW. Relations between muscle soreness and biochemical and functional outcomes of eccentric exercise. J Appl Physiol. (1993) 74:2976–83. doi: 10.1152/jappl.1993.74.6.2976
56. Callegari, GA, Novaes, JS, Neto, GR, Dias, I, Garrido, ND, and Dani, C. Creatine kinase and lactate dehydrogenase responses after different resistance and aerobic exercise protocols. J Hum Kinet. (2017) 58:65–72. doi: 10.1515/hukin-2017-0071
57. Chen, TC, Yang, T-J, Huang, M-J, Wang, H-S, Tseng, K-W, Chen, H-L, et al. Damage and the repeated bout effect of arm, leg, and trunk muscles induced by eccentric resistance exercises. Scand J Med Sci Sports. (2019) 29:725–35. doi: 10.1111/sms.13388
58. Brancaccio, P, Lippi, G, and Maffulli, N. Biochemical markers of muscular damage. Clin Chem Lab Med. (2010) 48:757–67. doi: 10.1515/CCLM.2010.179
59. Howatson, G, McHugh, MP, Hill, JA, Brouner, J, Jewell, AP, van Someren, KA, et al. Influence of tart cherry juice on indices of recovery following marathon running. Scand J Med Sci Sports. (2010) 20:843–52. doi: 10.1111/j.1600-0838.2009.01005.x
60. Nieman, DC, Zwetsloot, KA, Simonson, AJ, Hoyle, AT, Wang, X, Nelson, HK, et al. Effects of whey and pea protein supplementation on post-eccentric exercise muscle damage: a randomized trial. Nutrients. (2020) 12:2382. doi: 10.3390/nu12082382
61. Hilkens, L, De, BJ, Kretzers, J, Kardinaal, AFM, Floris-Vollenbroek, EG, Scholtens, PAMJ, et al. Whey protein supplementation does not accelerate recovery from a single bout of eccentric exercise. J Sports Sci. (2021) 39:322–31. doi: 10.1080/02640414.2020.1820184
62. Schumann, M, Feuerbacher, JF, Sünkeler, M, Freitag, N, Rønnestad, BR, Doma, K, et al. Compatibility of concurrent aerobic and strength training for skeletal muscle size and function: an updated systematic review and Meta-analysis. Sports Med. (2022) 52:601–12. doi: 10.1007/s40279-021-01587-7
63. Murlasits, Z, Kneffel, Z, and Thalib, L. The physiological effects of concurrent strength and endurance training sequence: a systematic review and meta-analysis. J Sports Sci. (2018) 36:1212–9. doi: 10.1080/02640414.2017.1364405
64. Markov, A, Hauser, L, and Chaabene, H. Effects of concurrent strength and endurance training on measures of physical fitness in healthy middle-aged and older adults: a systematic review with Meta-analysis. Sports Med. (2023) 53:437–55. doi: 10.1007/s40279-022-01764-2
65. Hartono, FA, Martin-Arrowsmith, PW, Peeters, WM, and Churchward-Venne, TA. The effects of dietary protein supplementation on acute changes in muscle protein synthesis and longer-term changes in muscle mass, strength, and aerobic capacity in response to concurrent resistance and endurance exercise in healthy adults: a systematic review. Sports Med. (2022) 52:1295–328. doi: 10.1007/s40279-021-01620-9
66. Camera, DM, West, DWD, Phillips, SM, Rerecich, T, Stellingwerff, T, Hawley, JA, et al. Protein ingestion increases myofibrillar protein synthesis after concurrent exercise. Med Sci Sports Exerc. (2015) 47:82–91. doi: 10.1249/MSS.0000000000000390
67. Camera, DM, Ong, JN, Coffey, VG, and Hawley, JA. Selective modulation of MicroRNA expression with protein ingestion following concurrent resistance and endurance exercise in human skeletal muscle. Front Physiol. (2016) 7:87. doi: 10.3389/fphys.2016.00087
68. Chen, TC, Lin, K-Y, Chen, H-L, Lin, M-J, and Nosaka, K. Comparison in eccentric exercise-induced muscle damage among four limb muscles. Eur J Appl Physiol. (2011) 111:211–23. doi: 10.1007/s00421-010-1648-7
69. Churchward-Venne, TA, Pinckaers, PJM, Smeets, JSJ, Betz, MW, Senden, JM, Goessens, JPB, et al. Dose-response effects of dietary protein on muscle protein synthesis during recovery from endurance exercise in young men: a double-blind randomized trial. Am J Clin Nutr. (2020) 112:303–17. doi: 10.1093/ajcn/nqaa073
Keywords: collagen peptides, muscle damage, concurrent training, recovery, regeneration, creatine kinase, repeated bout effect
Citation: Bischof K, Stafilidis S, Bundschuh L, Oesser S, Baca A and König D (2024) Reduction in systemic muscle stress markers after exercise-induced muscle damage following concurrent training and supplementation with specific collagen peptides – a randomized controlled trial. Front. Nutr. 11:1384112. doi: 10.3389/fnut.2024.1384112
Received: 08 February 2024; Accepted: 15 March 2024;
Published: 25 March 2024.
Edited by:
Guanghua Xia, Hainan University, ChinaReviewed by:
Haohao Shi, Hainan University, ChinaCopyright © 2024 Bischof, Stafilidis, Bundschuh, Oesser, Baca and König. This is an open-access article distributed under the terms of the Creative Commons Attribution License (CC BY). The use, distribution or reproduction in other forums is permitted, provided the original author(s) and the copyright owner(s) are credited and that the original publication in this journal is cited, in accordance with accepted academic practice. No use, distribution or reproduction is permitted which does not comply with these terms.
*Correspondence: Kevin Bischof, a2V2aW4uYmlzY2hvZkB1bml2aWUuYWMuYXQ=
Disclaimer: All claims expressed in this article are solely those of the authors and do not necessarily represent those of their affiliated organizations, or those of the publisher, the editors and the reviewers. Any product that may be evaluated in this article or claim that may be made by its manufacturer is not guaranteed or endorsed by the publisher.
Research integrity at Frontiers
Learn more about the work of our research integrity team to safeguard the quality of each article we publish.