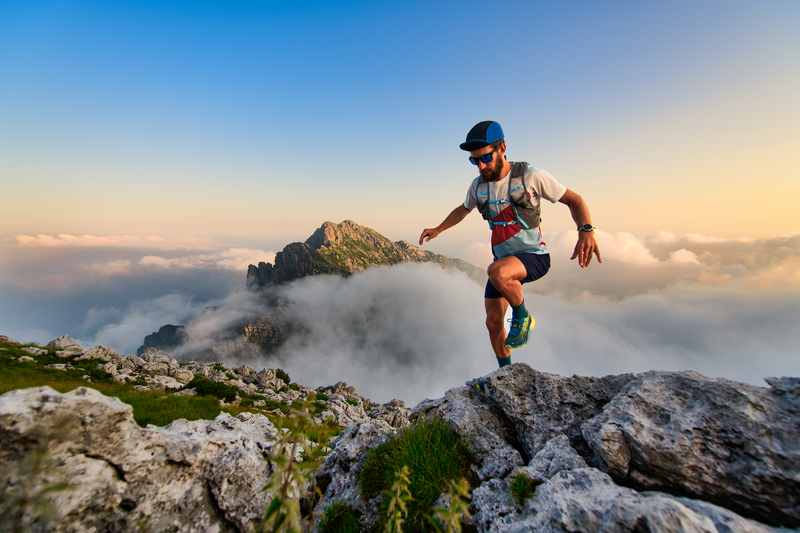
94% of researchers rate our articles as excellent or good
Learn more about the work of our research integrity team to safeguard the quality of each article we publish.
Find out more
ORIGINAL RESEARCH article
Front. Nutr. , 26 June 2024
Sec. Nutrition and Metabolism
Volume 11 - 2024 | https://doi.org/10.3389/fnut.2024.1383658
Background: High dietary protein intake exacerbates proteinuria in individuals with diabetic kidney disease (DKD). However, studies on the impacts of low protein diet (LPD) on DKD have yielded conflicting results. Furthermore, patient compliance to continuous protein restriction is challenging.
Objective: The current study aims to investigate the effects of intermittent protein restriction (IPR) on disease progression of DKD.
Methods: Diabetic KK-Ay mice were used in this study. For the IPR treatment, three consecutive days of LPD were followed by four consecutive days of normal protein diet (NPD) within each week. For early intervention, mice received IPR before DKD onset. For late intervention, mice received IPR after DKD onset. In both experiments, age-matched mice fed continuous NPD served as the control group. Kidney morphology, structure and function of mice in different groups were examined.
Results: Intermittent protein restriction before DKD onset ameliorated pathological changes in kidney, including nephromegaly, glomerular hyperfiltration, tubular injuries and proteinuria, without improving glycemic control. Meanwhile, IPR initiated after DKD onset showed no renoprotective effects despite improved glucose homeostasis.
Conclusion: Intermittent protein restriction before rather than after DKD onset protects kidneys, and the impacts of IPR on the kidneys are independent of glycemic control. IPR shows promise as an effective strategy for managing DKD and improving patient compliance.
Diabetic kidney disease (DKD), which progresses from an initially elevated glomerular filtration rate (GFR) to albuminuria and reduced GFR, is the leading cause of end-stage renal disease (ESRD) and the strongest predictor of mortality in diabetic patients (1–4). It is estimated that 783 million adults worldwide will have diabetes by 2045. With up to 40% of individuals with type 2 diabetes developing DKD, the incidence of DKD will continue to be an unmet clinical need (5, 6).
High protein intake is a well-established risk factor for the progression of DKD. In DKD, high protein intake can lead to significant increases in renal blood flow and GFR, which are thought to precede the loss of nephron units (7–12). Such increases can be prevented by protein restriction (PR) (13–16). In individuals with DKD, low protein intake may slow the progression of DKD, delay the initiation of renal replacement therapy and reduce urinary albumin excretion (17–28). It has been suggested that people with stage 3 to 5 of DKD should aim for a reduced dietary protein intake (DPI) of 0.6 to 0.8 g/kg body weight (BW) per day (29, 30).
Despite the potential benefits, patient compliance with continuous PR limits its application (19). There was an inverse relationship between patient compliance and urinary albumin excretion (28). More than a third of individuals do not have good compliance with continuous PR (20, 24). This brings intermittent protein restriction (IPR) intervention into consideration. An intermittent protein-restricted, calorie-restricted diet, known as the fasting mimicking diet (FMD), improved patient compliance and reduced dietary fatigue (31, 32). IPR has been shown to benefit insulin sensitivity similarly to continuous PR (33). It remains unclear whether IPR can provide beneficial effects on the progression of DKD.
Early intervention of DKD is crucial for improving renal outcomes. The rate of progression to ESRD in diabetic individuals with macroalbuminuria is about 14 times faster than that in those with other kidney diseases. The risk of all-cause mortality increases significantly as DKD progresses (34). Medication initiated after stage 3 or 4 of DKD may not slow disease progression as effectively as that started at the onset of microalbuminuria (35–37). Furthermore, in diabetic individuals, PR yields better results when implemented in the early stages of kidney disease or even before its onset (38, 39). Therefore, early detection and intervention is urgently needed to delay the onset and progression of DKD.
In this study, we used the KK-Ay mice, which closely mimic type 2 diabetic kidney disease, to investigate the effects of IPR on renal morphology, structure and function in DKD. The results showed that IPR before but not after the onset of DKD attenuated DKD progression.
The study was conducted according to the guidelines of the Declaration of Helsinki, and approved by the Committee on Ethics in the Care and Use of Laboratory Animals of Chu Hsien-I Memorial Hospital, Tianjin Medical University (Approval No. DXBYY-IACUC-2022070, approved on 1 March 2022). Nine-week-old male KK-Ay mice, which spontaneously develop diabetes, were obtained from HFK Bioscience Co. Ltd., Beijing. The animals were maintained in specific-pathogen-free, temperature-controlled (23 ± 1°C) facilities with optimal humidity, and a 12-h light/dark cycle at the Tianjin Key Laboratory of Metabolic Diseases, Chu Hsien-I Memorial Hospital and Tianjin Institute of Endocrinology, Tianjin Medical University.
The normal protein diet (NPD) and the specialized low protein diet (LPD) based on the NPD were obtained from HFK Bioscience Co. Ltd., Beijing (Table 1). The NPD provided 16.46% of calories from protein, 37.89% of calories from carbohydrates, and 45.65% of calories from fat. The LPD provided 5% of calories from protein. The reduced calories from protein in LPD were replaced by calories from carbohydrates, while calories from fat were held fixed, making the two diets isocaloric (4.25 kcal/g). In the IPR group, mice were fed LPD for three consecutive days followed by NPD for four consecutive days in each week, as previously described (33). For early intervention, mice were randomized to receive immediate intervention before the onset of DKD. For late intervention, mice received intervention after the onset of DKD when their urinary albumin to creatinine ratio (ACR) exceeded 300 mg/g in the absence of elevated serum creatinine levels. In both experiments, age-matched mice fed continuous NPD served as controls. All mice had ad libitum access to water and food except as indicated in the procedures below.
Mice were fasted for 6 h before blood glucose was measured. Plasma insulin levels were measured using a murine enzyme-linked immunosorbent assay (ELISA) kit from Jiangsu Meimian Industrial Co., Ltd., Jiangsu, China. The homeostasis model assessment of insulin resistance (HOMA-IR) was calculated.
After overnight fasting, a single dose of 1 g/kg BW glucose was injected intraperitoneally and blood glucose levels were measured at various times. The area under the curve (AUC) is then developed to quantify the overall increase in blood glucose during the test.
Immediately after anesthesia, whole blood was collected from the hearts of mice and placed in EDTA-K2-treated tubes. The blood was then centrifuged at 3,500 rpm for 10 min to separate the plasma supernatant, which was stored at −80°C for further experiments. The levels of plasma urea nitrogen, albumin, triglycerides (TG), total cholesterol (TC) and urinary retinol binding protein (RBP) were measured. Plasma levels of low-density lipoprotein cholesterol (LDL-C), creatinine and cystatin C were determined using murine ELISA kits (Jiangsu Meimian Industrial Co., Ltd.). The levels of urinary albumin, creatinine, Kim-1, NGAL, podocin and nephrin were also measured using ELISA kits from Jiangsu Meimian Industrial Co., Ltd.
Body composition analysis in mice was performed using quantitative magnetic resonance (QMR).
Mice were weighed and anesthetized with isoflurane. They were then injected retro-orbitally with FITC-labeled inulin (2 μL/g of BW). Blood samples were taken from the tail vein at various times. After centrifugation, the plasma supernatant was transferred to EP tubes and diluted with HEPES buffer (pH 7.4). The fluorescence intensity of the diluted plasma samples was measured using a microplate reader. Glomerular filtration rate (GFR) was calculated using GraphPad Prism software version 9.4.1.
After sacrifice, unilateral mouse kidney tissues were fixed in 4% paraformaldehyde and embedded in paraffin. Tissue sections were stained with hematoxylin and eosin (HE), Masson’s trichrome (MT) and periodic acid-Schiff (PAS). Tissue morphological changes were observed under an inverted microscope and photographed with the attached camera. Each staining was performed on 5 independent slides.
High-throughput RNA sequencing (RNA-seq) method was used by LC Bio Technology CO., Ltd. (Hangzhou, China) to obtain gene expression profiles in our study. Total RNA was extracted from kidney tissues and subjected to mRNA enrichment, cDNA library construction and Illumina sequencing. The resulting reads were aligned to the reference genome and gene expression levels were quantified using Fragments Per Kilobase of exon model per Million mapped fragments (FPKM). Differential gene expression analysis was performed to identify significant changes. Pathway and functional enrichment analyses were performed using the Gene Ontology (GO) and Kyoto Encyclopedia of Genes and Genomes (KEGG) databases in R, with statistical significance determined using the hypergeometric test (p-value < 0.05).
The antibody against E-cadherin was purchased from Proteintech (Wuhan, China). Antibodies to ZO-1 and occludin were purchased from Abmart (Abmart Shanghai Co., Ltd., Shanghai, China). The antibody against TGF-β was purchased from PTMab (Jingjie PTM BioLab Co., Ltd., Hangzhou, China). The immunoblotting protocol used in this study has been described previously (40). ImageJ software was used for image analysis.
For ZO-1 immunohistochemical staining, kidney sections were incubated in a humidified chamber with anti-ZO-1 antibody (catalog number ab221546, Abcam) and secondary antibody. The sections were then stained with a chromogenic substrate and hematoxylin (Beijing Solarbio Science & Technology Co., Ltd.). Multiple images (3 to 13) per section were captured using an Olympus BX51 microscope (Olympus Corporation, Tokyo, Japan).
All data are expressed as mean ± standard error of the mean (SEM). Statistical analysis was performed using an unpaired t-test where appropriate. Data that did not follow a normal distribution were tested by the nonparametric Mann–Whitney U test.
We first investigated the effects of IPR intervention after the onset of DKD (IPRa). Seventeen-week-old KK-Ay mice with elevated urinary albumin excretion above 300 mg/g creatinine without elevated serum creatinine levels (not shown) were treated with either continuous NPD or IPR for 22 weeks (Figure 1A). Relative lean body mass and serum albumin levels were not reduced after IPR intervention. These results suggested that IPR did not cause malnutrition in mice (Figures 1B, C). Interestingly, while calories from carbohydrates were used to replace calories from protein in LPD with a higher glycemic index, IPR initiated after the onset of DKD reduced body weight gain and improved glucose tolerance and insulin resistance (Figures 1D–G).
Figure 1. The beneficial metabolic effects of intermittent protein restriction (IPR) after the onset of diabetic kidney disease (DKD). (A) Protocol for investigating the influence of IPR after the onset of DKD on the renal outcomes in KK-Ay mice. (B) Lean mass adjusted by body weight (BW). (C) Serum albumin change after a 6-h fasting. (D) Changes of body weight during the experiment period. (E) Daily food consumption of every mouse during the experiment period. (F) Changes in blood glucose levels over time following intraperitoneal glucose injection. And area under the curve (AUC) of the intraperitoneal glucose tolerance test (IPGTT) was calculated. (G) Graphical representation of the homeostasis model assessment of insulin resistance (HOMA-IR) index. Data are represented as mean ± SEM (n = 6). #p < 0.05, ns indicates a non-significant p-value. Two groups of mice were included: diabetic male KK-Ay mice receiving a continuous normal protein diet (CONa), and mice receiving IPR intervention (IPRa). IPRa versus CONa, by unpaired t-tests.
Despite the beneficial metabolic effects, kidney weight, kidney index and GFR measured by serum FITC-labeled inulin concentrations were increased but not decreased in IPRa mice, compared with those in control group (Figures 2A, B). Mesangial matrix expansion and interstitial collagen deposition were more evident in IPRa mice than those in the control group (Figures 2C, D). Glomerular size was not significantly changed (Figure 2D). Podocin and nephrin are major protein components of the podocyte slit diaphragm, an important part of the glomerular filtration barrier (GFB). The urinary levels of podocin and nephrin were not reduced by IPR (Figure 2E). The excretion of urinary albumin, immunoglobulin G (IgG) and transferrin (TF) was not reduced following IPR (Figure 2F). Tubular injury markers including urinary neutrophil gelatinase-associated lipocalin (NGAL), kidney injury molecule-1 (Kim-1) and retinol binding protein (RBP) in the urine were not changed by IPR (Figure 2G). Serum cystatin C, urea nitrogen and creatinine levels were not decreased (Figure 2H). Together, these results showed that IPR starting after the onset of DKD failed to improve renal outcomes, regardless of improved glycemic control.
Figure 2. IPR after the onset of DKD did not improve renal outcomes. (A) Kidney weight and kidney index calculated as the kidney weight adjusted for BW after 22-week dietary intervention. (B) Concentrations of serum FITC-labeled inulin at various time points after retro-orbital injection. And glomerular filtration rate (GFR) was calculated based on the clearance of serum FITC-labeled inulin. (C) Representative images of PAS and MT staining in renal tubules (scale bar, 100 μm). (D) Representative images of H&E and PAS staining in glomeruli (scale bar, 50 μm), and quantitative analysis of glomerular size (25 glomeruli per mouse were analyzed, n = 6). (E) Urinary podocin and nephrin excretion levels. (F) Urinary albumin to creatinine ratio, immunoglobulin G (IgG) and transferrin (TF) excretion levels. (G) Levels of urinary neutrophil gelatinase-associated lipocalin (NGAL), kidney injury molecule-1 (Kim-1) and retinol binding protein (RBP) excretion. (H) Plasma cystatin C, urea nitrogen and creatinine levels after IPR intervention. Data are represented as mean ± SEM (n = 6). #p < 0.05, ##p < 0.01, ns indicates a non-significant p-value. IPRa versus CONa.
To determine whether IPR starting before the onset of DKD (IPRb) could slow DKD progression, KK-Ay mice at nine weeks of age that did not develop proteinuria were treated with either continuous NPD or IPR for 22 weeks (Figure 3A). In IPRb mice, kidney weight, kidney index and urinary albumin excretion (Figures 3B–D) were significantly reduced, compared with those in NPD-treated mice. Notably, despite the reduction in kidney volume, serum urea nitrogen and creatinine levels did not increase significantly (Figure 3E). Similarly, there was no difference in serum cystatin C levels between the IPRb group and the control group (Figure 3E). Together, these results demonstrated the protective effects of initiating IPR before the onset of early DKD.
Figure 3. IPR before the onset of DKD improved renal outcomes. (A) Protocol for investigating the influence of IPR before the onset of DKD on the renal outcomes in KK-Ay mice. (B) Weight of kidneys. (C) Kidney index. (D) Urinary albumin to creatinine ratio after 22-week diet intervention. (E) Serum cystatin C, urea nitrogen and creatinine levels at the end of intervention. Data are represented as mean ± SEM (n = 6). *p < 0.05, ns indicates a non-significant p-value. Two groups of mice were included: mice fed a continuous normal protein diet (CONb), and mice fed IPR (IPRb) before DKD onset. IPRb versus CONb, by unpaired t-tests.
Given that the impairment of glomerular filtration barrier, manifested by protein leakage from the bloodstream into the urine, is a strong risk factor for the progression of DKD to ESRD, we investigated the effects of IPRb on the glomeruli. The clearance of serum FITC-labeled inulin, the gold standard of kidney function, was used to measure GFR. In IPRb mice, measured GFR, glomerular volumes and mesangial matrix expansion were reduced compared with those in control mice (Figures 4A, B). Urinary podocin, nephrin, IgG, and TF levels were decreased by IPR started before the onset of DKD (Figures 4C, D). In the tubules, pathological changes such as infiltration of inflammatory cells and deposition of collagen fibers in the peritubular region were evident in the DKD mice (Figure 4E), which were attenuated by IPRb. The excretion of NGAL and RBP in the urine was reduced in IPRb group as well (Figure 4F). Together, these results highlighted the beneficial effects of IPRb in attenuating both glomerular and tubular damages.
Figure 4. IPR before the onset of DKD protected both glomeruli and tubules. (A) Concentrations of FITC-labeled inulin at different time after retro-orbital injection. GFR was calculated. (B) Representative images of glomeruli stained with H&E and PAS (scale bar, 50 μm). Quantitative analysis of glomerular size was performed (25 glomeruli per mouse, n = 6). (C) Levels of urinary podocin and nephrin excretion. (D) Levels of urinary IgG and TF excretion. (E) Representative images of renal tubules stained with PAS and MT (scale bar, 100 μm). (F) Levels of urinary NGAL, Kim-1 and RBP excretion. Data are represented as mean ± SEM (n = 6). *p < 0.05, **p < 0.01, ns indicates a non-significant p-value. IPRb versus CONb.
Given the importance of glycemic control in slowing the progression of DKD, we tested whether the renoprotective effects of IPR before DKD onset resulted from glycemic control. IPR initiated before DKD onset did not result in decreased lean body mass or serum albumin levels (Figures 5A, B). Glucose tolerance and insulin resistance were not improved by IPRb (Figures 5C, D). Furthermore, IPR started before DKD onset did not reduce body weight gain or food intake (Figure 5E). Taken together, these findings suggested that the beneficial effects of IPRb on the kidneys were independent of glycemic control.
Figure 5. IPR before the onset of DKD did not improve glycemic control. (A) Lean mass adjusted by BW. (B) Serum albumin changes after a 6-h fasting. (C) Dynamic changes in blood glucose levels after intraperitoneal glucose injection and AUC of the IPGTT. (D) Graphical representation of the HOMA-IR index. (E) Body weight change and daily food consumption of every mouse throughout the study duration. Data are represented as mean ± SEM (n = 6). *p < 0.05, ns indicates a non-significant p-value, IPRb versus CONb.
To investigate the potential mechanism underlying early IPR’s beneficial effects, we performed transcriptomic analysis of kidney tissues. Differentially expressed genes were particularly enriched in cell-cell junction assembly (GO: 0007043), cadherin binding (GO: 0045296), and adherens junction (GO: 0005912) (Figure 6A). Thyroid hormone synthesis (mmu04918) and TGF-beta signaling pathway (mmu04350) were identified as significantly differentially expressed pathways by KEGG pathway enrichment analysis. All genes mapped to these KEGG pathways were upregulated in the IPRb group, compared with those in the control group (Figure 6B). Selected results from the profile analyses were verified by Western blot, confirming the upregulation of ZO-1, occludin and E-cadherin, and the suppression of TGF-β (Figure 6C). ZO-1 upregulation was further supported by immunohistochemical staining (Figure 6D). On the other hand, IPR started after the onset of DKD did not alter the protein expression of ZO-1, occludin, E-cadherin, and TGF-β (Figure 6E). Immunohistochemical staining for ZO-1 in kidney tissues showed no significant differences. Together, these results suggested that early IPR intervention before DKD onset improved renal outcomes through protection of cell-cell junction.
Figure 6. Cell-cell junction was improved by early IPR initiated before DKD onset. (A) GO analysis of differentially expressed genes in kidney tissues of which IPR started before DKD onset, focusing on biological processes. (B) KEGG analysis of pathways involved of kidney tissues. (C) Representative immunoblotting images illustrating the expression levels of ZO-1, E-cadherin, occludin, TGF-β and β-actin in kidney tissues of which IPR started before DKD onset (n = 6). Quantitative analysis of each protein expression level is presented, normalized to β-actin expression levels. (D) Immunostaining of ZO-1 in kidney tissues of which IPR started before or after DKD onset, with a scale bar representing 100 μm. (E) Representative immunoblotting images illustrating the expression levels of ZO-1, E-cadherin, occludin, TGF-β and β-actin in kidney tissues of which IPR started after DKD onset (n = 6). Quantitative analysis of each protein expression level is presented, normalized to β-actin expression levels. Data presented as mean ± SEM. Statistical significance indicated by #p < 0.05, while ns indicates a non-significant p-value. IPRa versus CONa. *p < 0.05, ns indicates a non-significant p-value. IPRb versus CONb.
In the current study, we found that IPR before but not after DKD onset attenuated its progression. IPR before DKD onset ameliorated pathophysiological changes of DKD without improving glycemic control. On the other hand, IPR administered after the onset of DKD showed no significant renal protection despite the improved glucose homeostasis.
A study by Kitada et al. (41) showed improved kidney function when IPR was administered in advanced DKD. In contrast, the levels of serum creatinine and cystatin C were not significantly increased in mice when IPR started after DKD onset in the current study, but GFR was elevated, suggesting that different stages of DKD were involved. And in that study, the intervention was performed with an alternation between a high protein diet and LPD, different from the transition from NPD to LPD used in the current study. This diet difference may contribute to the different effects of IPR on renal outcomes.
Age-related protein absorption may play a role in the different effects of IPRa and IPRb on DKD. Despite the consensus that LPD benefits stages 3 to 5 DKD, our results showed that IPR started after DKD onset at the age of 17 weeks did not improve renal outcomes, in agreement with a study by Meloni et al. (39). IPR initiated at 9 weeks of age before the onset of DKD ameliorated the pathophysiological changes of DKD. This is consistent with a previous report suggesting that people under 65 years of age may benefit from LPD, whereas the older groups may not (42). Age-related malabsorption of proteins and amino acids can lead to malnutrition (43). The benefits of LPD may potentially be compromised by the adverse effects of malnutrition in older animals.
The lack of protection against DKD in the IPRa group could also be due to the short duration of the intervention. Studies have shown that the short-term effects of treatments, including PR, may differ from the long-term benefits on the progression of kidney disease (44). In the modification of diet in renal disease (MDRD) trial, long but not short LPD treatment showed a significant effect of PR in slowing GFR decline (45, 46). It is possible that extending the duration of IPRa intervention could show protective effects on DKD. Further research is needed to assess the long-term effects of IPRa.
The pathological changes of DKD at early or late stage may also attribute to the different effects of IPRa and IPRb. In the early stages of DKD, albuminuria is likely resulted from a local intrarenal hemodynamic effect rather than structural changes in the kidney. Early dietary intervention may reduce albuminuria and delay disease progression. In the late stages of DKD, the progressive structural damage becomes irreversible. Interventions at this advanced stage are less effective than early interventions (36, 47). This may partly explain the differences observed in the current study between early and late IPR intervention. Levels of albuminuria were significantly higher when IPRa was initiated than those when IPRb was initiated.
The amount of food taken by the control group and the IPR group showed no significant differences during LPD and NPD feeding periods, supporting lower total protein intake in the IPR-treated group. The reduced protein intake was further supported by the reduction in serum urea nitrogen levels in IPR group. Of note, there was no decrease in plasma albumin levels or lean mass after long-term IPR. These results suggested that the IPR approach effectively reduces protein intake while mitigating the risk of malnutrition associated with prolonged implementation of LPD (48).
IPR has been proposed as a potential strategy to improve glucose homeostasis (33), which is crucial for the treatment of DKD. Interestingly, our results showed that implementation of IPR before the onset of DKD slowed DKD progression without improving glycemic control. On the other hand, IPR after DKD onset showed no protective effect on DKD progression while improving glucose homeostasis. The improvement in glycemic control occurred despite the higher glycemic index of LPD. These results suggested that the effects of IPR on renal outcomes and glucose homeostasis were not correlated (49) and early IPR protected kidney through glycemia-independent mechanism.
Cell-cell junctions are important components for the integrity of GFB to prevent serum protein leakage into the urine (50). The upregulated expression levels of intracellular junction proteins ZO-1, occludin, and E-cadherin may partly contribute to the improved renal outcomes after IPR initiated before DKD onset. IPR initiated after DKD onset did not improve intracellular junctions, which may partly explain the lack of improvement of renal outcomes. The TGF-β signaling pathway is closely associated with intracellular junctions (51). The decrease in TGF-β expression levels was observed in the mice treated with IPR before the onset of DKD, suggesting that the TGF-β signaling pathway may be involved in the beneficial effects.
In conclusion, our research suggested that IPR held great promise as an effective strategy for the management of DKD independently of glycemic control. Importantly, early rather than late IPR intervention provided significant renal protection while mitigating the risk of malnutrition. The different renal outcomes of IPR intervention started at different time underscored the importance of bringing forward the timing of PR intervention to slow the progression of DKD.
The raw sequence data reported in this paper have been deposited in the NCBI Short Read Archive (SRA) with bioproject accession number (PRJNA1123475). The raw data supporting the conclusions of this article are available upon request from the corresponding author, without undue reservation.
The animal study was approved by the Committee on Ethics in the Care and Use of Laboratory Animals of Chu Hsien-I Memorial Hospital, Tianjin Medical University. The study was conducted in accordance with the local legislation and institutional requirements.
XP: Formal analysis, Investigation, Writing – original draft. ML: Writing – review & editing. YW: Writing – review & editing. WF: Writing – review & editing. YH: Writing – review & editing. YK: Writing – review & editing. YL: Resources, Writing – review & editing. XZ: Writing – review & editing. CS: Conceptualization, Writing – review & editing. HS: Conceptualization, Writing – review & editing. YY: Conceptualization, Formal analysis, Investigation, Writing – review & editing.
The authors declare that financial support was received for the research, authorship, and/or publication of this article. This work was supported by the National Key Research and Development Program of China (2019YFA0802500), the National Natural Science Foundation of China (92057107, 32200965, and 32371234), Collaborative Innovation Program of Shanghai Municipal Health Commission (2020CXJQ01), the Tianjin Key Medical Discipline (Specialty) Construction Project (TJYXZDXK-032A), the Tianjin Natural Science Foundation (Grant Nos. 21JCQNJC00460 and 22JCQNJC01330), the Special Foundation for Beijing Tianjin Hebei Basic Research Cooperation (J210008, 21JCZXJC00170, and H2021202008), and the Scientific Research Funding of Tianjin Medical University Chu Hsien-I Memorial Hospital (Nos. ZXY-ZDSYS2021-4, ZXY-ZDSYSZD2023-1, ZXY-YJJ2020-5, and ZXY-ZDSYSZD2021-2).
The authors declare that the research was conducted in the absence of any commercial or financial relationships that could be construed as a potential conflict of interest.
All claims expressed in this article are solely those of the authors and do not necessarily represent those of their affiliated organizations, or those of the publisher, the editors and the reviewers. Any product that may be evaluated in this article, or claim that may be made by its manufacturer, is not guaranteed or endorsed by the publisher.
1. Foley R, Collins A. End-stage renal disease in the United States: An update from the united states renal data system. J Am Soc Nephrol. (2007) 18:2644–8. doi: 10.1681/asn.2007020220
2. Alicic R, Rooney M, Tuttle K. Diabetic kidney disease: Challenges, progress, and possibilities. Clin J Am Soc Nephrol. (2017) 12:2032–45. doi: 10.2215/cjn.11491116
3. Pereira P, Carrageta D, Oliveira P, Rodrigues A, Alves M, Monteiro M. Metabolomics as a tool for the early diagnosis and prognosis of diabetic kidney disease. Med Res Rev. (2022) 42:1518–44. doi: 10.1002/med.21883
4. Raes A, Donckerwolcke R, Craen M, Hussein M, Vande Walle J. Renal hemodynamic changes and renal functional reserve in children with type I diabetes mellitus. Pediatr Nephrol. (2007) 22:1903–9. doi: 10.1007/s00467-007-0502-6
5. Saeedi P, Petersohn I, Salpea P, Malanda B, Karuranga S, Unwin N, et al. Global and regional diabetes prevalence estimates for 2019 and projections for 2030 and 2045: Results from the international diabetes federation diabetes Atlas, 9(th) Edition. Diabetes Res Clin Pract. (2019) 157:107843. doi: 10.1016/j.diabres.2019.107843
6. Sun H, Saeedi P, Karuranga S, Pinkepank M, Ogurtsova K, Duncan B, et al. IDF diabetes atlas: Global, regional and country-level diabetes prevalence estimates for 2021 and projections for 2045. Diabetes Res Clin Pract. (2022) 183:109119. doi: 10.1016/j.diabres.2021.109119
7. Ronco C, Brendolan A, Bragantini L, Chiaramonte S, Fabris A, Feriani M, et al. Renal functional reserve in pregnancy. Nephrol Dial Transplant. (1988) 3:157–61.
8. Bosch J, Saccaggi A, Lauer A, Ronco C, Belledonne M, Glabman S. Renal functional reserve in humans. Effect of protein intake on glomerular filtration rate. Am J Med. (1983) 75:943–50. doi: 10.1016/0002-934390873-2
9. Sharma A, Mucino M, Ronco C. Renal functional reserve and renal recovery after acute kidney injury. Nephron Clin Pract. (2014) 127:94–100. doi: 10.1159/000363721
10. Armenta A, Madero M, Rodriguez-Iturbe B. Functional reserve of the kidney. Clin J Am Soc Nephrol. (2022) 17:458–66. doi: 10.2215/cjn.11070821
11. De Nicola L, Keiser J, Blantz R, Gabbai F. Angiotensin ii and renal functional reserve in rats with goldblatt hypertension. Hypertension. (1992) 19:790–4. doi: 10.1161/01.hyp.19.6.790
12. Tuttle K, Bruton J, Perusek M, Lancaster J, Kopp D, DeFronzo R. Effect of strict glycemic control on renal hemodynamic response to amino acids and renal enlargement in insulin-dependent diabetes mellitus. N Engl J Med. (1991) 324:1626–32. doi: 10.1056/nejm199106063242304
13. Smadel J, Farr L. The effect of diet on the pathological changes in rats with nephrotoxic nephritis. Am J Pathol. (1939) 15:199–216.5. doi: 10.1111/j.1753-4887.1988.tb05436.x
14. Castellino P, Coda B, DeFronzo R. Effect of amino acid infusion on renal hemodynamics in humans. Am J Physiol. (1986) 251:F132–40. doi: 10.1152/ajprenal.1986.251.1.F132
15. Viberti G, Bognetti E, Wiseman M, Dodds R, Gross J, Keen H. Effect of protein-restricted diet on renal response to a meat meal in humans. Am J Physiol. (1987) 253:F388–93. doi: 10.1152/ajprenal.1987.253.3.F388
16. Brenner B, Meyer T, Hostetter T. Dietary protein intake and the progressive nature of kidney disease: The role of hemodynamically mediated glomerular injury in the pathogenesis of progressive glomerular sclerosis in aging, renal ablation, and intrinsic renal disease. N Engl J Med. (1982) 307:652–9. doi: 10.1056/nejm198209093071104
17. Pedrini M, Levey A, Lau J, Chalmers T, Wang P. The effect of dietary protein restriction on the progression of diabetic and nondiabetic renal diseases: A meta-analysis. Ann Intern Med. (1996) 124:627–32. doi: 10.7326/0003-4819-124-7-199604010-00002
18. Nezu U, Kamiyama H, Kondo Y, Sakuma M, Morimoto T, Ueda S. Effect of low-protein diet on kidney function in diabetic nephropathy: Meta-analysis of randomised controlled trials. BMJ Open. (2013) 3:e002934. doi: 10.1136/bmjopen-2013-002934
19. Pan Y, Guo L, Jin H. Low-protein diet for diabetic nephropathy: A meta-analysis of randomized controlled trials. Am J Clin Nutr. (2008) 88:660–6. doi: 10.1093/ajcn/88.3.660
20. Barsotti G, Cupisti A, Barsotti M, Sposini S, Palmieri D, Meola M, et al. Dietary treatment of diabetic nephropathy with chronic renal failure. Nephrol Dial Transplant. (1998) 13:49–52. doi: 10.1093/ndt/13.suppl_8.49
21. Brouhard B, LaGrone L. Effect of dietary protein restriction on functional renal reserve in diabetic nephropathy. Am J Med. (1990) 89:427–31. doi: 10.1016/0002-934390370-s
22. Velázquez López L, Sil Acosta M, Goycochea Robles M, Torres Tamayo M, Castañeda Limones R. Effect of protein restriction diet on renal function and metabolic control in patients with type 2 diabetes: A randomized clinical trial. Nutr Hosp. (2008) 23:141–7.
23. Walker J, Bending J, Dodds R, Mattock M, Murrells T, Keen H, et al. Restriction of dietary protein and progression of renal failure in diabetic nephropathy. Lancet. (1989) 2:1411–5. doi: 10.1016/s0140-673692032-1
24. Zeller K, Whittaker E, Sullivan L, Raskin P, Jacobson H. Effect of restricting dietary protein on the progression of renal failure in patients with insulin-dependent diabetes mellitus. N Engl J Med. (1991) 324:78–84. doi: 10.1056/nejm199101103240202
25. Raal F, Kalk W, Lawson M, Esser J, Buys R, Fourie L, et al. Effect of moderate dietary protein restriction on the progression of overt diabetic nephropathy: A 6-Mo prospective study. Am J Clin Nutr. (1994) 60:579–85. doi: 10.1093/ajcn/60.4.579
26. Hansen H, Tauber-Lassen E, Jensen B, Parving H. Effect of dietary protein restriction on prognosis in patients with diabetic nephropathy. Kidney Int. (2002) 62:220–8. doi: 10.1046/j.1523-1755.2002.00421.x
27. Pijls L, de Vries H, Donker A, van Eijk J. The effect of protein restriction on albuminuria in patients with type 2 diabetes mellitus: A randomized trial. Nephrol Dial Transplant. (1999) 14:1445–53. doi: 10.1093/ndt/14.6.1445
28. Dullaart R, Beusekamp B, Meijer S, van Doormaal J, Sluiter W. Long-term effects of protein-restricted diet on albuminuria and renal function in IDDM patients without clinical nephropathy and hypertension. Diabetes Care. (1993) 16:483–92. doi: 10.2337/diacare.16.2.483
29. Kidney Disease: Improving Global Outcomes (KDIGO) Diabetes Work Group. KDIGO 2020 clinical practice guideline for diabetes management in chronic kidney disease. Kidney Int. (2020) 98:S1–115. doi: 10.1016/j.kint.2020.06.019
30. Ikizler T, Cuppari L. The 2020 updated KDOQI clinical practice guidelines for nutrition in chronic kidney disease. Blood Purif. (2021) 50:667–71. doi: 10.1159/000513698
31. Brandhorst S, Longo V. Protein quantity and source, fasting-mimicking diets, and longevity. Adv Nutr. (2019) 10:S340–50. doi: 10.1093/advances/nmz079
32. Wei S, Zhao J, Bai M, Li C, Zhang L, Chen Y. Comparison of glycemic improvement between intermittent calorie restriction and continuous calorie restriction in diabetic Mice. Nutr Metab (Lond). (2019) 16:60. doi: 10.1186/s12986-019-0388-x
33. Wei S, Li C, Luo X, Yang L, Yu L, Wang Q, et al. Intermittent protein restriction protects islet β cells and improves glucose homeostasis in diabetic mice. Sci Bull. (2022) 67:733–47. doi: 10.1016/j.scib.2021.12.024
34. KDOQI. KDOQI clinical practice guidelines and clinical practice recommendations for diabetes and chronic kidney disease. Am J Kidney Dis. (2007) 49:S12–154. doi: 10.1053/j.ajkd.2006.12.005
35. Romagnani P, Remuzzi G, Glassock R, Levin A, Jager K, Tonelli M, et al. Chronic kidney disease. Nat Rev Dis Prim. (2017) 3:17088. doi: 10.1038/nrdp.2017.88
36. Schievink B, Kröpelin T, Mulder S, Parving H, Remuzzi G, Dwyer J, et al. Early renin-angiotensin system intervention is more beneficial than late intervention in delaying end-stage renal disease in patients with type 2 diabetes. Diabetes Obes Metab. (2016) 18:64–71. doi: 10.1111/dom.12583
37. Tonelli M, Wiebe N, Richard J, Klarenbach S, Hemmelgarn B. Characteristics of adults with type 2 diabetes mellitus by category of chronic kidney disease and presence of cardiovascular disease in Alberta Canada: A cross-sectional study. Can J Kidney Health Dis. (2019) 6:2054358119854113. doi: 10.1177/2054358119854113
38. American Diabetes Association. Nutritional recommendations and principles for individuals with diabetes mellitus: 1986. Diabetes Care. (1987) 10:126–32. doi: 10.2337/diacare.10.1.126
39. Meloni C, Morosetti M, Suraci C, Pennafina M, Tozzo C, Taccone-Gallucci M, et al. Severe dietary protein restriction in overt diabetic nephropathy: Benefits or risks? J Ren Nutr. (2002) 12:96–101. doi: 10.1053/jren.2002.31762
40. Xu D, Wang Z, Zhang Y, Jiang W, Pan Y, Song B, et al. Paqr3 modulates cholesterol homeostasis by anchoring scap/srebp complex to the golgi apparatus. Nat Commun. (2015) 6:8100. doi: 10.1038/ncomms9100
41. Kitada M, Ogura Y, Suzuki T, Monnno I, Kanasaki K, Watanabe A, et al. Cyclic and intermittent very low-protein diet can have beneficial effects against advanced diabetic nephropathy in wistar fatty (Fa/Fa) rats, an animal model of type 2 diabetes and obesity. Nephrology. (2017) 22:1030–4. doi: 10.1111/nep.13152
42. Levine M, Suarez J, Brandhorst S, Balasubramanian P, Cheng C, Madia F, et al. Low protein intake is associated with a major reduction in IGF-1, cancer, and overall mortality in the 65 and younger but not older population. Cell Metab. (2014) 19:407–17. doi: 10.1016/j.cmet.2014.02.006
43. Castellino P, Giordano M, de Pascale E, Solini A. Derangements in protein metabolism induced by type I diabetes mellitus. Miner Electrolyte Metab. (1998) 24:41–6. doi: 10.1159/000057349
44. Belledonne M. Short-term effects of protein intake, blood pressure, and antihypertensive therapy on glomerular filtration rate in the modification of diet in renal disease study. J Am Soc Nephrol. (1996) 7:2097–109. doi: 10.1681/asn.V7102097
45. Klahr S, Levey A, Beck G, Caggiula A, Hunsicker L, Kusek J, et al. The effects of dietary protein restriction and blood-pressure control on the progression of chronic renal disease. N Engl J Med. (1994) 330:877–84. doi: 10.1056/nejm199403313301301
46. Kasiske B, Lakatua J, Ma J, Louis TA. A meta-analysis of the effects of dietary protein restriction on the rate of decline in renal function. Am J Kidney Dis. (1998) 31:954–61. doi: 10.1053/ajkd.1998.v31.pm9631839
47. Palmer A, Annemans L, Roze S, Lamotte M, Lapuerta P, Chen R, et al. Cost-effectiveness of early irbesartan treatment versus control (standard antihypertensive medications excluding ace inhibitors, other angiotensin-2 receptor antagonists, and dihydropyridine calcium channel blockers) or late irbesartan treatment in patients with type 2 diabetes, hypertension, and renal disease. Diabetes Care. (2004) 27:1897–903. doi: 10.2337/diacare.27.8.1897
48. Noce A, Vidiri M, Marrone G, Moriconi E, Bocedi A, Capria A, et al. Is low-protein diet a possible risk factor of malnutrition in chronic kidney disease patients? Cell Death Discov. (2016) 2:16026. doi: 10.1038/cddiscovery.2016.26
49. Trepanowski J, Kroeger C, Barnosky A, Klempel M, Bhutani S, Hoddy K, et al. Effect of alternate-day fasting on weight loss, weight maintenance, and cardioprotection among metabolically healthy obese adults: A randomized clinical trial. JAMA Intern Med. (2017) 177:930–8. doi: 10.1001/jamainternmed.2017.0936
50. Kocylowski M, Aypek H, Bildl W, Helmstädter M, Trachte P, Dumoulin B, et al. A slit-diaphragm-associated protein network for dynamic control of renal filtration. Nat Commun. (2022) 13:6446. doi: 10.1038/s41467-022-33748-1
Keywords: diabetic kidney disease, intermittent protein restriction, low protein diet, early intervention, cell-cell junction
Citation: Peng X, Liu M, Wu Y, Fan W, Hou Y, Kong Y, Liu Y, Zhang X, Shan C, Sun H and Yang Y (2024) Intermittent protein restriction before but not after the onset of diabetic kidney disease attenuates disease progression in mice. Front. Nutr. 11:1383658. doi: 10.3389/fnut.2024.1383658
Received: 07 February 2024; Accepted: 10 June 2024;
Published: 26 June 2024.
Edited by:
Marija Takic, University of Belgrade, SerbiaReviewed by:
Yan Chen, University of Chinese Academy of Sciences, ChinaCopyright © 2024 Peng, Liu, Wu, Fan, Hou, Kong, Liu, Zhang, Shan, Sun and Yang. This is an open-access article distributed under the terms of the Creative Commons Attribution License (CC BY). The use, distribution or reproduction in other forums is permitted, provided the original author(s) and the copyright owner(s) are credited and that the original publication in this journal is cited, in accordance with accepted academic practice. No use, distribution or reproduction is permitted which does not comply with these terms.
*Correspondence: Chunyan Shan, Y2h1bnlhbnNoYW5AaG90bWFpbC5jb20=; Haipeng Sun, c3VuLmhhaXBlbmdAdG11LmVkdS5jbg==; Yanhui Yang, eWFuZ3lhbmh1aUB0bXUuZWR1LmNu
Disclaimer: All claims expressed in this article are solely those of the authors and do not necessarily represent those of their affiliated organizations, or those of the publisher, the editors and the reviewers. Any product that may be evaluated in this article or claim that may be made by its manufacturer is not guaranteed or endorsed by the publisher.
Research integrity at Frontiers
Learn more about the work of our research integrity team to safeguard the quality of each article we publish.